DOI:
10.1039/D3AN01252B
(Critical Review)
Analyst, 2023,
148, 5805-5821
Recent advances in the modification of electrodes for trace metal analysis: a review†
Received
21st July 2023
, Accepted 25th August 2023
First published on 25th August 2023
Abstract
This review paper summarizes the research published in the last five years on using different compounds and/or materials as modifiers for electrodes employed in trace heavy metal analysis. The main groups of modifiers are identified, and their single or combined application on the surface of the electrodes is discussed. Nanomaterials, film-forming substances, and polymers are among the most used compounds employed mainly in the modification of glassy carbon, screen-printed, and carbon paste electrodes. Composites composed of several compounds and/or materials have also found growing interest in the development of modified electrodes. Environmentally friendly substances and natural products (mainly biopolymers and plant extracts) have continued to be included in the modification of electrodes for trace heavy metal analysis. The main analytical performance parameters of the modified electrodes as well as possible interferences affecting the determination of the target analytes, are discussed. Finally, a critical evaluation of the main findings from these studies and an outlook discussing possible improvements in this area of research are presented.
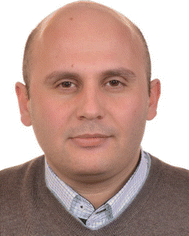 Klodian Xhanari | Klodian Xhanari graduated from the University of Tirana, with a Diploma in General Chemistry in 2004. In 2011, he received his Ph.D. in Chemical Engineering from the Norwegian University of Science and Technology in Trondheim, Norway. From October 2015 to July 2016, he pursued his postdoctoral studies at the Laboratory for Analytical Chemistry and Industrial Analysis at the University of Maribor, Slovenia. Since 2012 he has been a full-time lecturer/researcher at the Faculty of Natural Sciences, University of Tirana, Albania. In 2021 he received the Associate Professor title from the University of Tirana. His research interests include corrosion and corrosion protection, electroanalysis, and surface characterization. |
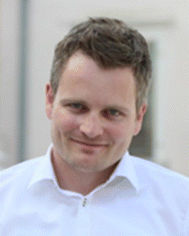 Matjaž Finšgar | Matjaž Finšgar was a researcher at Jožef Stefan Institute (2006–2010). He earned his Ph.D. in Analytical Chemistry from the University of Ljubljana (2010). He continued his post-doctorate studies at BASF SE, Ludwigshafen, Germany (2011–2012). Later, he was a researcher at the University of Maribor (2012–2015). He was appointed as a professor at the University of Maribor in 2015. Now he is the head of the Laboratory for Analytical Chemistry and Industrial Analysis. His expertise includes analytical chemistry, electroanalysis, surface analysis, and corrosion studies. |
1. Introduction
This work presents the recent developments in the research published in the last five years (i.e., 2018–2022, including the research published so far in 2023) on the modification of different types of electrodes used in trace heavy metal determination. Literature research on this area showed a large number of publications published in the last five years. As shown in Fig. 1a, there has been continuously increased interest in the research community throughout these years (i.e., an increased number of publications in 2018–2020) on the use of different types of modifiers. The decline in the research work published in 2021 and 2022 can be attributed to the slowdown from the pandemic. The focus of this review paper is to discuss the use of nanomaterials, film-forming substances (to obtain metallic or polymeric film-modified electrodes), polymers, metal- and covalent–organic frameworks, as well as environmentally friendly substances and natural products for the modification of different types of electrodes employed in trace heavy metal determination. To the best of the authors’ knowledge, no comprehensive review of the research work published in this area in the last five years has been presented.
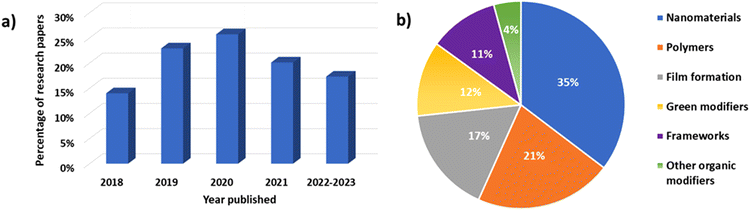 |
| Fig. 1 Distribution of the research work (presented in this review) published in the last five years (i.e., 2018–2022, including the research work published so far in 2023) on modifications of electrodes used in trace heavy metal analysis based on (a) the year of publication and (b) the type of electrode modifier used. | |
Heavy metals are naturally occurring metals and metalloids with a relatively high atomic number (higher than 20) and density (higher than 5 g cm−3).1,2 Some of these elements (i.e., Cu, Fe, Zn, and Fe) in small quantities are considered essential and can be part of enzymatic and metabolic mechanisms in different systems. Other metals, such as Cd, Hg, Pb, and As are not essential and show high toxicity even at lower concentrations (i.e., at ppb levels).2 The toxicity of these heavy metals combined with their ability to bioaccumulate and the fact that they are nondegradable present a significant threat not only to the environment (i.e., water, soil, and air), but also to human health.1–4
Determination of trace level concentrations of heavy metals present in different matrixes (mainly in water) has been a continuous challenge. Several spectroscopic techniques, among which atomic absorption spectroscopy (AAS) and inductively coupled plasma mass spectrometry (ICP-MS), have been first employed for trace level analysis of heavy metals.5,6 However, electrochemical techniques have been found to be more attractive in this area as they provide high analytical performance (in terms of selectivity, precision, and accuracy) at a much lower cost compared with spectroscopic techniques, using portable instrumentation which allows in situ analysis.7–10
Voltammetric methods, especially stripping techniques, mainly anodic stripping voltammetry (ASV) combined with square-wave and differential pulse excitation signals i.e., SWASV and DPASV, respectively, have very low detection limits.11 In the first step of ASV (the pre-concentration step), the metal ions are electrodeposited (reduced) from the solution on the surface of the electrode at an applied potential. In the second step (stripping step), the potential is swept in the anodic direction, and at a specific potential, the electrodeposited metal is oxidized and stripped out of the electrode into the solution. The current flowing in this step is proportional to the concentration of the metal present.
Different types of mercury electrodes (i.e., hanging mercury drop electrodes, dropping mercury electrodes, and mercury film electrodes) have been first employed in trace metal analysis.7,9,12,13 Although the mercury electrodes have, among others, high sensitivity and reproducibility, due to mercury's toxicity and its ability to accumulate, they are no longer desirable for this determination.7,14 Glassy carbon electrodes (GCEs) and carbon paste electrodes (CPEs) have been extensively employed in the determination of several heavy metals in various environments. In addition to the most common electrodes mentioned above, several other approaches have also been used to develop electrodes for trace metal analysis, including 3D printed electrodes and electrochemical paper-based analytical devices (ePADs).15–20 The ease of fabrication onto paper combined with the possibility of performing highly selective determination with very low detection limits of target analytes, using miniaturized electrodes, renders ePADs very attractive in trace metal analysis under both static and hydrodynamic conditions.17,18,20,21 In addition, these devices are non-expensive and easily disposable.20 Screen-printing technology is one of the most common methods for ePAD manufacturing, with screen-printed electrodes (SPEs) being among the most used electrodes. 3D printing technology allows the low cost, in-house manufacturing of highly reproducible electrodes with customizable geometries using a wide range of materials.15,16,19,22 Photopolymerization, extrusion, powder bed fusion and material jetting are the most used methods for the fabrication of 3D printed electrodes.15,16 In order to increase charge transfer and to obtain reproducible active surfaces, different types of surface activations are sought for 3D printed electrodes, including metal deposition, and chemical and electrochemical treatments.16
However, independent of their type, in order to improve the sensitivity and/or selectivity of all types of electrodes, further modifications are always sought. As shown in Fig. 1b, a wide range of different materials and/or compounds, including metallic and polymeric film-forming substances, various types of nanomaterials (i.e., nanoparticles (NPs), nanotubes, nanocomposites, and nanosheets), metal- and covalent–organic frameworks, polymers, and environmentally friendly modifiers (i.e., biopolymers, plant extracts, natural products, etc.) have been employed.
In the following sections, the main materials and/or compounds are discussed (grouped based on their type), along with the explanation of their use in combination with other modifiers to obtain the modified electrodes for trace heavy metal analysis. This review will first discuss the modification of electrodes using different types of nanomaterials, followed by film-formation. Next, the role of polymers, metal- and covalent–organic frameworks, other organic compounds, as well as environmentally friendly compounds, and natural products as electrode modifiers will be discussed. In addition, for each of the main groups of the modifiers mentioned above, the main analytical performance parameters of the modified electrodes (including the limit of detection (LOD) and linear concentration range) and the respective testing conditions, as well as possible interferences affecting their sensitivity are presented in Tables S1–S6 in the ESI.† The application of the modified electrodes for trace metal analysis in real samples will also be discussed.
2. Nanomaterials as electrode modifiers
The combination of a large specific surface area and high conductivity renders nanomaterials (NMs) very attractive as electrode modifiers in trace heavy metal analysis. Five main types of NMs, i.e., graphene, graphene oxide (GO) and reduced graphene oxide (rGO),22–92 NPs,23–25,27,31,56,57,59,64,67,70,83,86,87,93–138 single and multiwalled carbon nanotubes (SWCNTs and MWCNTs),32,83,89,98,99,101,113,129,133,139–162 nanosheets,34,85,107,111,139,162–166 and nanofibers,167–169 have been employed in the modification of the electrodes used in the determination of heavy metals. Nanocubes, nanoflakes, nanospheres, and nanodiamonds75,170–175 are among the other types of NMs used in the modification of the electrodes. Moreover, a poly(melamine)/grafitic-C3N4 nanonetwork,176 NiCo2O4 nanocages,177 Co3O4 nanopolyhedrons,88 and N,S-dual-doped carbon/sepiolite clay hybrid nanostructures178 were also used in the modification of various types of electrodes.
NMs have been primarily applied on the electrodes in combination with other modifiers (including through the formation of composites).22–25,27,30–32,34,46,47,50,54,59,67,70,72,75,83,85,86,88,89,92–102,107,113,115,117,118,122–126,129–131,135,136,138–156,160,162,168,175,179–189 However, in most of these studies, due to the lack of discussing the sensing mechanism of these nanocomposite-modified electrodes, there is no clear understanding of the influence of different components on the sensitivity and/or selectivity of the electrodes. Apart from the combination of different NMs (usually a combination of NPs and MWCNTs),83,98,99,101,107,113,115,128,129,133,139,162 compounds and/or materials belonging to the other main groups of electrode modifiers have also been combined with NMs to modify the electrodes. As a result, many of the studies reported on the use of nanocomposites are further discussed in sections 3–7. Several studies also reported on the use of NMs as sole electrode modifiers.105,109,112,114,119,121,127,134,137,161,163,165,166,170,172,173,190 Table S1 in the ESI† summarizes the main findings of the research studies published in the last five years on using different NMs to determine trace heavy metals. The parameters related to the analytical performance of the modified electrodes with composites comprised of other main group modifiers are presented in Tables S2–S6 in the ESI.†
Carbon-based nanomaterials, including graphene, GO, rGO, carbon nanotubes (CNTs), carbon nanofibers, and carbon nanosheets, are widely used in the modification of electrodes. In addition to the above-mentioned properties, carbon-based NMs are easy to apply, low cost, and abundant materials which can be further functionalized and serve as hosts for other compounds/materials (mainly NPs).191,192 These materials are mostly used either in combination with other modifiers,24,30,34,47,54,56,57,59,64,67,70,75,85,86,88,107,113,115,129,147,149,160,162 or functionalized with other compounds.146,148,158,159,167
In general, organic functionalization (i.e., oxygen and amino functionalization) and doping (mainly with nitrogen, boron, sulphur, and phosphorus) are the two main strategies used to improve the catalytic activity and increase active sites (affecting in this manner the electron-transfer process) and the hydrophilic nature of the carbon NMs.73,85,147,193,194 Co-doping rGO with either nitrogen and sulphur67,178 or nitrogen and bismuth195 has also been reported. In order to overcome the insolubility and their tendency to agglomerate, as well as to improve their biocompatibility, CNTs are subjected to covalent functionalization (acid treatment) and non-covalent functionalization by polymers, surfactants, or aromatic compounds.32,98,101,142,148,154,158 However, the use of CNTs also faces challenges related to their synthesis (i.e., cost and specific route) as well as the need for binders to improve their mechanical stability.196
Metals (i.e., Ag, Au, Bi, Pd, Pt, Pb, and Sn),23–25,56,57,59,64,70,83,86,87,93–95,97–99,101,102,104–106,108,110,113–115,117,120,121,123,125–127,129,132,134,138 metal oxides and mixed metal oxides (i.e., SiO2, SnO2, Fe3O4, Mn3O4, Cu2O/ZnO, FeAl2O4, MgFe2O4, ZnFe2O4, and NiFe2O4)27,31,67,96,100,103,109,112,118,122,124,130,131,133,135–137,188,197,198 are the most commonly used NPs in the modification of the electrodes. In addition, clinoptilolite (a naturally occurring zeolite containing Si and Al) NPs were ion exchanged in a Fe(II) solution, and the modified CPE obtained was used in the determination of Sn2+.199 Several research groups have also reported on the synthesis of magnetic NMs (mainly NPs as part of nanocomposites) and their use as electrode modifiers.26,30,96,100,132,137,182,188,200Fig. 2 presents the preparation of magnetic NiFe2O4-NPs and polypyrrole (PPy) nanocomposites used in the modification of a GCE for the determination of Pb2+ in tap, lake, and river water samples.96 Although the performance of the NM-modified electrodes has been mainly tested in real water samples, several studies reported on their use in heavy metal determination in other samples, including, among others, body fluids31,57,88,163,173,190 and cosmetic product190 samples. Moreover, Das et al.31 successfully employed a bimetal oxide (Bi2O3/Fe2O3)-NP decorated GO modified PGE for the determination of Cd2+ in a wide range of real samples, including food (potato, lemon, and apple), soil, biological samples (blood serum and urine), and water (tap, drinking and chemical laboratory wastewater) samples.
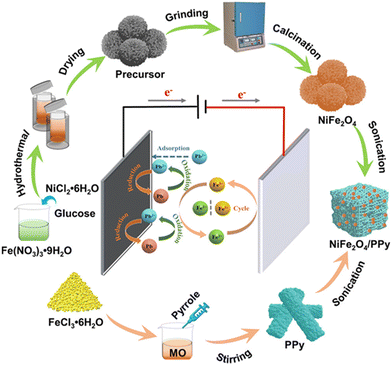 |
| Fig. 2 Schematic illustration of the preparation process of the NiFe2O4/PPy nanocomposite.96 Reprinted from Y. Wang, Z. Nie, X. Li, R. Wang, Y. Zhao, and H. Wang, PPy-functionalized NiFe2O4 nanocomposites toward highly selective Pb2+ electrochemical sensing, ACS Sustainable Chem. Eng., 10(18), 6082–6093, Copyright (2022) American Chemical Society. | |
In addition to the widely used electrodeposition, reduction, and hydrothermal methods, the synthesis of NPs from environmentally friendly sources (i.e., waste and plant extracts as well as mango seed kernels) has been reported,23,119–122,125,127 and will be further discussed in section 7.
3. Polymers as electrode modifiers
Polymers’ high conductivity and porosity, combined with the presence of several functional groups and their ability to be further functionalized, make polymer-modified electrodes very attractive in trace heavy metal determination. Table S2 in the ESI† summarizes the main findings of the research studies published in the last five years reporting on the use of different polymer modifiers for various types of electrodes employed in the determination of trace heavy metals.
Although the vast majority of the studies reported on the combined use of polymers and other materials and/or molecules to modify the electrodes,22,23,26,29,32,35,37,38,41,45,61,69,71–73,77,80,84,87,89,91,92,94,96–102,106,122–124,131,141–144,175,176,182–184,186,187,197,200–221 there is also a significant number of studies on polymers as sole modifiers.174,185,222–232 These exceptions include, among others, the pure polymer film-modified electrodes discussed in section 4. A combination of two polymers (i.e., conductive polydopamine (PDA) doped PPy hydrogel) as modifiers of an SPCE for the determination of Pb2+ has also been reported.232
Biopolymers (mainly chitosan and different forms of cellulose)35,37,61,69,71–73,87,89,92,123,124,175,186,187,197,200–205 (further discussed in section 7) and Nafion (a sulfonated tetrafluoroethylene-based fluoropolymer–copolymer)24,62,108,113,149,154,155,179,200,233–241 are by far most frequently used as polymeric components of the nanocomposites. Moreover, other conducting polymers such as polyaniline (PANI),23,38,99,102,122,144,183,206–209,220 PPy,32,77,80,96,101,210–212,214,216 and poly(3,4-ethylenedioxythiophene) (PEDOT) derivatives,58,174,217 as well as PDA,98,182 have also been part of the nanocomposites used to modify electrodes for trace heavy metal analysis. In addition, nanocomposites comprised of ion imprinted polymers (IIPs)29,41,84,87,100,187,214,219 as well as ion imprinted NPs206,215 have also been reported as modifiers. Fig. 3 shows the preparation steps for an ion imprinted (L-cysteine) and ball milling biochar (BBC) obtained from the high temperature pyrolysis of sugarcane-modified GCE used in the individual determination of Pb2+ and Cd2+ in tap, lake, and ground water samples.219 The authors reported that low LOD values of 5.86 fM and 0.883 aM for Pb2+ and Cd2+, respectively, were achieved with the modified electrode.
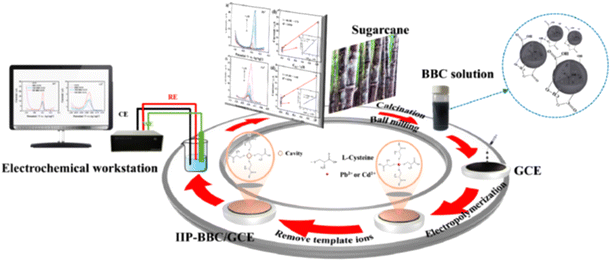 |
| Fig. 3 Schematic diagram for the preparation of the ion imprinted BBC electrode (IIP-BBC/GCE) and application for the detection of heavy metal ions219 (this figure was published in D. Mao, J. Hu, P. Duan, C. Qin, Y. Piao, Ultrasensitive and highly reusable electrochemical sensor with ion imprinted nanobiochar, Sens. Actuators, B, 371, 132490, Copyright Elsevier (2022)). | |
Nandy et al.97 synthesized a block copolymer from poly(methyl methacrylate) and poly(2-acrylamido-2-methylpropane sulfonic acid) and, in combination with Ag-NPs used it to modify a GCE for the determination of Hg2+. Different forms of carbon-containing materials (i.e., graphene, GO, rGO, CNTs, and g-C3N4),29,32,38,45,80,87,91,98,99,101,141,142,144,176,183,184,206 NPs and nanoflakes,23,87,94,96–99,101,102,124,176,182,200,218 and MOFs208,209,213,216 were combined with the polymers and biopolymers to obtain the modified electrodes. Chelating agents, such as 2,2′,2′′,2′′′-(ethane-1,2-diyldinitrilo)tetraacetic acid (EDTA) and L-cysteine, have also been included in these polymer-containing nanocomposites.207,214,220 Meanwhile, Fernando et al.142 reported on the combination of a chelating polymer (i.e., poly(4-vinyl pyridine)) with MWCNTs and poly(ethylene terephthalate) as modifiers of an Au electrode for Cu2+ determination in tap, lake, and ocean water samples. Apart from different types of real water samples, few studies reported on polymer-modified electrodes for heavy metal determination in food and beverages100,185,242 and body fluid samples.106,185,209
In situ electrochemical and oxidative polymerization,38,80,174,176,211,214,219 as well as co-precipitation polymerization,26,100,215 are among the methods used to prepare the nanocomposites. Suspensions of these nanocomposites in different solvents (including ethanol, isopropanol, N,N-dimethylformamide, and water) were dropcast on the surface of the electrodes. In the case of modified CPEs, the polymers were mixed with graphite and other additives to form the paste.
4. Film-forming substances as electrode modifiers
The development of film-coated electrodes presented an excellent alternative to the mercury electrodes in trace heavy metal analysis.7,243 Since the first Bi-coated carbon electrode proposed by Wang et al.,244 several mainly metallic (including Sb-, Sn-, Pb-, and Cu-film electrodes) and other film-coated electrodes have been employed in the determination of various heavy metals.7,12,243 In the last five years, the research was focused on preparing two main types of film electrodes, i.e., metallic-25,40,50,83,181,197,233,235,245–273 and polymeric-film electrodes.77,91,94,95,141,210,217,220,223–230,274–276 However, the combination of metallic (Bi) and polymer films (i.e., PPy and aminonaphthalenesulphonic acid derivatives), in addition to other materials, was also used to modify GCEs for the determination of Pb2+ and Cd2+.216,221 Moreover, the development of a bimetallic (Hg–Bi)-film incorporated with a poly(1,2-diaminoanthraquinone) modified GCE for individual and simultaneous determination of Pb2+, Cd2+, and Zn2+ was reported.277 Oghli and Soleymanpour218 reported on combining poly-L-cysteine (a conducting polymer) and a Fe3O4-NPs film to modify a pencil graphite electrode (PGE) for the determination of Hg2+, Cu2+, and Cd2+. Furthermore, carbon and several of its forms (i.e., diamond, graphdiyne, rGO, and g-C3N4) have also been coated on different types of film electrodes.76,83,93,139,246,278Fig. 4 presents the formation of a dendritic bismuth and graphdiyne (Bi/GDY) film on the surface of a GCE used for the simultaneous determination of Zn2+, Cd2+, and Pb2+ in real seawater samples.246 The authors attributed the low LODs obtained (i.e., 0.115 nM, 0.171 nM, and 0.146 nM for Zn2+, Cd2+, and Pb2+, respectively) to the synergistic effect of the Bi-film and the GDY. Table S3 in the ESI† summarizes the main findings of the research studies published in the last five years regarding the use of different types of film electrodes in the determination of trace heavy metals.
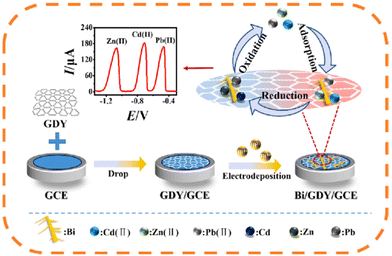 |
| Fig. 4 The preparation of the Bi/GDY/GCE and the application for simultaneous detection of three heavy metal ions246 (this figure was published in Y. Ai, L. Yan, S. Zhang, X. Ye, Y. Xuan, S. He, X. Wang, W. Sun, Ultra-sensitive simultaneous electrochemical detection of Zn(II), Cd(II) and Pb(II) based on the bismuth and graphdiyne film modified electrode, Microchem. J., 184, 108186, Copyright Elsevier (2023)). | |
Bismuth film electrodes (BiFEs) are among the most employed electrodes in the determination of trace heavy metals.25,40,50,83,181,197,233,235,245–260 In addition to its low toxicity and the ability to form alloys with other metals, bismuth has a wide potential range, which allows BiFEs to have comparable analytical performance to the mercury electrodes in trace heavy metal analysis.7,243,244 The film can be electrochemically deposited directly on the electrode surface from the solution containing both the modifier and the analytes (in situ preparation) or through film deposition before the electrode is introduced in the sample solution (ex situ preparation). In the studies published in the last five years, bismuth was almost always in situ electrodeposited on the electrode surface, with only a few exceptions.197,248 Trace heavy metal analysis has been performed either with just BiFEs247,248,252,253,255–257,260 or with electrodes modified with a Bi-film combined with other materials and/or compounds, including with different NMs,25,50,83,181,256 Nafion and GO,40,83,233,235,250 as well as doping of the Bi-films with boron or indium.245,259 Various other metals, including Sb,261–264 Cu,265–267 Au,268 Pb,269 and Hg,260 have also been electrodeposited on different types of electrodes. Moreover, complexing agents such as 8-hydroxyquinoline,251 nioxime,265 and cupferron269 have been added during the in situ Bi-, Pb-, and Cu-film modification of GCEs. Next, the combination of two or three metals such as Bi–Sn, Bi–Cu, Bi–Hg, and Bi–Sn–Sb has also been reported for the modification of GCEs used in the determination of Pb2+, Cd2+, and Zn2+.270–272,277 The main advantage of these types of electrodes is their increased sensitivity compared with single metal film electrodes. Different Bi
:
Cu, Bi
:
Sn, and Bi
:
Sn
:
Sb ratios (for a total mass concentration of either 0.5 or 1.0 mg L−1) have been employed to in situ modify GCEs.270–272 No direct correlation between the Bi to metal ratio and the LOD or linear concentration range of all the analytes of interest has been observed in these studies.
Polymer film electrodes are obtained primarily by electropolymerization of the monomers on the electrode surface94,141,223–230,274,275 However, Yi et al.91 drop-coated the surface of the GCE with a suspension of poly(L-glutamic acid) and GO in N,N-dimethylformamide, and used the modified film electrode for simultaneous determination of Cu2+, Cd2+, and Hg2+. Aminoanthraquinone141,229,230,274 and PPy derivative polymers,77,210,216,223 are among the most used conductive polymers to coat the electrodes. The modified electrodes are obtained either by pure polymer film-formation,223–230 or by combining the polymers with other materials and/or compounds.91,94,95,141,210,220,274,276
5. Metal- and covalent–organic frameworks as electrode modifiers
Table S4 in the ESI† summarizes the main findings of the research studies published in the last five years regarding the use of different metal–organic frameworks (MOFs) and covalent–organic frameworks (COFs) as modifiers for various types of electrodes employed in the determination of trace heavy metals. Several MOFs42,53,116,150–154,164,177,201,208,209,212,216,236,237,279–297 and COFs198,298–302 have been employed as modifiers for different types of electrodes in combination with other materials. MOFs are 1D, 2D, or 3D network like structures, highly ordered crystalline materials formed by self-assembly of metal ions or clusters and organic ligands through coordination bonds.303 A similar type of structure is observed in the case of COFs, but instead of the metal COFs they contain light elements (such as C, H, O, N, or B atoms) connected with the rest with strong covalent bonds.304 Both types of materials possess a large specific surface area, high and tunable porosity, and, most importantly, can be easily functionalized or combined with other materials. These properties make these materials suitable for applications in several areas, including as modifiers for different types of electrodes.303,304
However, two of the main drawbacks of MOFs and COFs in trace heavy metal determination are their low conductivity and low stability in water. To increase the charge transfer (i.e., to facilitate the electron transfer through the electrode surface to or from the electroactive species in solution) and to improve their mechanical strength, MOFs have been combined with carbon-containing materials, such as graphene (mainly rGO),42,53,285 MWCNTs,150,152–154 mesoporous carbon,282 carbon black,236 and expanded graphite.286 The addition of conducting polymers (mainly PANI and PPy)208,209,212,213,216 to MOFs has resulted in higher conductivity and stability in water. The development of core–shell structures has been reported to not only increase the stability, but also improve the LOD of the modified electrodes used for the determination of Pb2+, Cd2+, and Cu2+ ions.150,209 These structures are formed through either the integration of two MOFs (i.e., NH2-UiO-66 and ZIF-8 to form NU66@Z8, shown in Fig. 5),150 or the combination of a MOF (i.e., NH2-UiO-66) and self-doped PANI nanofibers.209 The functionalized NU66@Z8 (shown in Fig. 5) was applied on the GCE and the modified electrode was used in the simultaneous determination of Pb2+ and Cu2+ in tap water of laboratory, soil, and Chinese cabbage samples.150
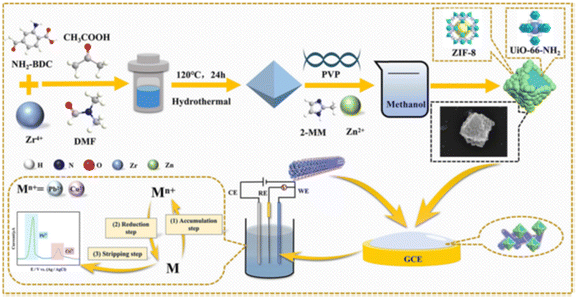 |
| Fig. 5 The synthesis route of NU66@Z8 and the construction of the working electrode150 (this figure was published in R. Tan, P. Jiang, C. Pan, J. Pan, N. Gao, Z. Cai, F. Wu, G. Chang, A. Xie, Y. He, Core–shell architectured NH2-UiO-66@ZIF-8/multi-walled carbon nanotubes nanocomposite-based sensitive electrochemical sensor towards simultaneous determination of Pb2+ and Cu2+, Microchim. Acta, 190, 30, Copyright Springer (2023)). | |
Moreover, the integration of Bi in the form of films or encapsulated in the mesoporous structure of MOFs and COFs, has also been reported.198,216,295 Liu et al.216 and Sarvestani et al.198 used an in situ formed Bi-film and MOFs or COFs to modify a GCE surface for the determination of Pb2+. Few studies reported on the use of newly synthesized MOFs and COFs as sole electrode modifiers.284,288,291,302
Zeolitic imidazolate frameworks (ZIFs),154,177,201,236,279,284,286 amino-functionalized Zr-based MOFs (Universitet i Oslo (UiO) type, i.e., NH2-UiO-66),42,53,151,152,209,216,283,285 and Materials of Institute Lavoisier (MILs),164,212,295 are among the main types of MOFs used as electrode modifiers. The presence of amino groups or imidazole in MOFs facilitates the formation of coordination bonds with heavy metals, improving the adsorption ability of the analytes and thus improving the analytical performance of the electrodes. The majority of COFs used were prepared by amine–aldehyde condensation. The metals incorporated in the MOF structures include Zr, Zn, Cu, Cr, Bi, Fe, V, Co, Mn, Ca, and lanthanides. However, bimetallic ZIF-type MOFs (i.e., Zn and Ni236 and Fe and Co284) have also been reported as modifiers for GCEs. Moreover, ZIF-8 modified with mesoporous carbons served as the substrate for the in situ growth of MIL-100(Fe), and the obtained hybrid material from the combination of two MOFs was used to modify the GCE for the simultaneous determination of Cd2+ and Pb2+.282 In addition, MOFs (i.e., ZIF-67) can serve as precursors for the synthesis of 3D structures, such as NiCo2O4 nanocages, employed to modify the GCE, enabling Hg2+ determination.177
Dropcasting dispersions of the MOFs in various solvents (including ethanol, N,N-dimethylformamide, and isopropanol) on the pretreated surfaces of the electrodes, followed by drying at room temperatures, is generally the main mechanism for applying these frameworks. Although GCEs are by far the most commonly used substrates for the application of different types of MOFs and COFs,53,116,150,152–154,177,198,201,216,236,237,279–290,298,299 several other types of electrodes have also been modified, including CPEs,291,292,301 SPCEs,42,209 gold electrodes,212,293 as well as PGEs, SPEs, and carbon cloth electrodes (CCEs).208,294,295,298 In addition, Yang et al.296 and Han et al.300 reported on the attachment of a mercaptan functionalized Zr-based MOF as well as a 1,4-benzenedithiol-2,5-diamino-hydrochloride and 1,3,5-triformylbenzene condensed COF to a 3D-structure kenaf stem derived carbon. These combined electrodes have been employed in either the simultaneous determination of Cd2+, Pb2+, Cu2+, and Hg2+ or for the determination of Hg2+ individually. The authors reported that the integrated electrode has better analytical performance compared with the GCE modified electrode.296 In addition to different real water samples, modified electrodes have also been successfully employed in the determination of heavy metals in food,42,53,116,151,236,293 spices,198,302 and body fluid208,209 samples.
6. Other organic compounds as electrode modifiers
In addition to the above-mentioned main groups of materials and/or compounds, in the last five years, the research community has also reported on the inclusion of calixarene-based compounds,117,118,281,305–309 ionic liquids (ILs),55,155,168,258 and Schiff bases156,310,311 as electrode modifiers for trace heavy metal analysis. Table S5 in the ESI† summarizes the main findings of the research studies published in the last five years reporting on the use of other organic compounds as modifiers for various types of electrodes employed in the determination of trace heavy metals.
Calix[n]arenes are macrocyclic compounds containing several phenolic units (n represents the number of phenolic units).312 Their cavity-containing, cup-shaped structure with both polar and nonpolar groups, combined with the ability to form complexes with cations, in addition to their ease of synthesis and functionalization, makes these compounds attractive in trace metal analysis. In the last five years calix[4]arene-functionalized compounds have been used mainly as sole electrode modifiers. However, their use in combination with NPs (i.e., Au- and Mn3O4-NPs),117,118 as well as with a MOF281 was also reported. Calix[4]arene-functionalized compounds were mostly used to modify GCEs (in addition to the SPCE and Au electrode) employed in the individual or simultaneous determination of Hg2+, Pb2+, Cd2+, Zn2+, and Cu2+ in different real water samples (including wastewater, river, drinking, and deionized water)117,118,305–307,309 and commercial oysters, clams, and mussel samples.308
Imidazolium-155,168 and pyridinium-based55,258 ILs are also among the organic compounds used to modify the electrodes. These ILs were always applied in combination with other materials and/or compounds (i.e., nanofibers, SWCNTs, Bi and Fe3O4-NPs, and L-cysteine). Apart from the conventional electrodes (GCE, SPE, and CPE), a pyridinium-based IL (i.e., N-butylpyridinium hexafluorophosphate) in combination with poly-L-cysteine has been used to modify a laser-engraved electrode (LEGE) as shown in Fig. 6. The authors have attributed the increased sensitivity of the modified LEGE to the combination of the good conductivity of the IL and the complexing ability of poly-L-cysteine. The modified electrodes have been successfully employed in the individual or simultaneous determination of Pb2+ and Cd2+ in real water55,168 and soil extract155,258 samples.
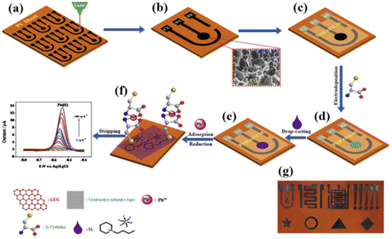 |
| Fig. 6 Schematic of the fabrication process of LEG micro-patterned electrodes for the electrochemical sensing of lead ions. (a) Fabrication of LEGE arrays on a PI sheet by direct laser engraving. (b) Three-electrode system of the LEGE. (c) Selective surface passivation of LEGE areas by a parafilm to define the WE area. (d) L-Cysteine was polymerized on the electrode to form the PLC/LEGE. (e) IL-functionalized PLC/LEGE. (f) Selective electrochemical detection of Pb2+. (g) Optical image of different micro-patterned electrode arrays on a PI sheet55 (this figure was published in Z. Lu, X. Lin, J. Zhang, W. Dai, B. Liu, G. Mo, J. Ye, J. Ye, Ionic liquid/poly-L-cysteine composite deposited on flexible and hierarchical porous laser-engraved graphene electrode for high-performance electrochemical analysis of lead ion, Electrochim. Acta, 295, 514–523, Copyright Elsevier (2019)). | |
The ability of the Schiff bases to form complexes with metal ions through the lone pair electrons of O and N atoms in their structure makes this type of compound of interest potent in the modification of electrodes for trace metal analysis. Schiff bases have been used either as sole modifiers310,311 or applied in combination with MWCNTs156 on the surface of CPEs. The modified electrodes have been successfully employed mostly in the determination of Pb2+ and Hg2+ in diverse samples, including real water,310,311 food,310 tobacco,310,311 and algae156 samples.
7. Environmentally friendly compounds and natural products as electrode modifiers
In the last five years, several research groups have continued to explore the inclusion of various environmentally friendly (green) modifiers in the development of new electrodes for trace metal analysis.23,35,37,61,66,69,71–73,87,89,92,104,110,120–127,157,175,186,187,197,201–205,219,289,293,313–321 Table S6 in the ESI† summarizes the main findings of the research studies published in the last five years reporting on the use of different green modifiers for various types of electrodes employed in the determination of trace heavy metals.
A wide range of green modifiers has been employed, including biopolymers,35,37,61,69,71–73,87,89,92,123,124,175,186,187,197,201–205 plant extracts,23,66,104,120–122,125,127,313 natural products,126,219,314–318 biomass,157,289,319 and DNA.293,320,321 It is important to emphasize that all the different green modifiers have been either added to or combined with other modifiers to achieve the desired electroanalytical performance of the newly developed electrodes through the synergistic effect. Various other modifiers (discussed in sections 2–6) have been combined with the green modifiers to obtain the final modified electrodes. Moreover, Wong et al.175 reported on combining two green modifiers, chitosan, and biochar (i.e., carbon NPs with a large surface area and temperature dependent hydrophobicity), which in addition to nanodiamonds were used to modify a GCE for Pb2+ and Cd2+ determination in river water samples. Low LODs (i.e., 0.056 μM and 0.110 μM for Pb2+ and Cd2+, respectively) were achieved with the modified electrode.175
In addition to the above-mentioned green modifiers, few research groups reported on environmentally friendly approaches to obtain the modified electrodes. The determination of Pb2+ and Cd2+ in either various vegetables65 or herbal food supplements239 was carried out with a multilayer graphene paste electrode modified with activated carbon produced from coconut husk or using the graphite extracted from waste zinc–carbon batteries combined with Bi-NPs, MWCNTs, and Nafion, respectively. Pristine blast furnace slag322 has also been used to modify a GCE for the determination of Pb2+ and Cu2+. However, the determination of 1 mM of the analytes was significantly influenced by the presence of 2 mM Cd2+, Zn2+, and Hg2+.
Biopolymers are by far the most used green modifiers for the various types of electrodes used in trace heavy metal analysis. This is due to the fact that apart from being biocompatible, biodegradable, nontoxic, and relatively inexpensive, they combine high mechanical strength and water permeability, good adherence to surfaces, good film-forming ability, and high hydrophilicity with the ability to form chelates with transition metal ions.123 These compounds are rich in functional groups such as hydroxyl, carboxyl, and sulfonic groups, which bind the analytes and help facilitate the combination of different modifiers.69,71,72,92 Chitosan,35,37,61,73,87,89,123,124,175,186,187,197,201 modified cellulose, bacterial cellulose and carboxymethyl cellulose (CMC),69,71,202,203 lignosulfonate,92 chitin,204 lignin,205 and κ-carrageenan72 are among the biopolymers used to modify the electrodes.
In most studies, plant extracts have been used as precursors of NPs (mainly Ag-NPs)23,104,120,121,125,127 to modify electrodes for the determination of Pb2+, Cd2+, and Hg2+ ions. The synthesis and characteristics of Ag-NPs, as well as the performance of the obtained Ag-NPs modified electrode, were found to be pH dependent.127 Okpara et al.122 synthesized metal oxide NPs from orange and lemon peel extracts and used them to modify an SPCE to determine Cd2+ and Hg2+ ions. Meanwhile, the powder obtained from Eichhornia crassipes root extract was incorporated into a CPE and used for Pb2+ and Cd2+ determination.313
Natural products such as spent coffee grounds,314,315Bigarreau Burlat kernel shells,316 walnut shells,317 tapioca flour,126 mango seed kernels,318 sugarcane,219 and waste pomelo peels110 have also been used as green modifiers, with and without pretreatment. The spent coffee grounds were first treated with citric acid to increase the carboxyl groups present, and then, in addition to other modifiers, were used to prepare the modified CPE employed for the determination of Cd2+ and Pb2+.314 Spent coffee grounds,315 walnut shells,317 and sugarcane219 were subjected to pyrolysis to obtain biochar, which, combined with other compounds, was used to modify GCEs or CPEs for the determination of Cu2+, Pb2+, Cd2+, and Hg2+ ions. No prior chemical pretreatment of Bigarreau Burlat kernels shells316 and mango seed kernels318 was reported. Zhang et al.110 obtained porous activated carbon from waste pomelo peels through a KOH activation method followed by carbonization at 700 °C. The porous activated carbon was then, in combination with Pd-NPs used to modify a GCE for the simultaneous determination of Cd2+, Pb2+, and Cu2+.
Dali et al.157 modified a GCE with a mixture of functionalized CNTs and filamentous fungi (Trichoderma asperellum) and used it to determine Cd2+ and Pb2+. The filamentous fungi can excrete organic acids which bind heavy metals. Hordeum vulgare L. dust (biomass residue released by brewing industries) activated using Na2CO3 was used to modify a CPE for the determination of Cd2+, Pb2+, and Hg2+.319
In all these studies, the authors claim the use of either environmentally friendly components or approaches for the modification of the electrodes, but no studies on the toxicity, biodegradability, and/or biocompatibility of the obtained composites used for electrode modification are presented.
8. Conclusions and outlook
The large number of publications reported in the last five years on modifications of electrodes for trace metal analysis emphasizes even more the continuous and growing interest in this area of research. A wide range of compounds and/or materials have been employed as modifiers, including nanomaterials, metallic and polymeric film-formation substances, metal– and covalent–organic frameworks, polymers, and environmentally friendly compounds and natural products (i.e., biopolymers, plant extracts, etc.).
The vast majority of the studies published in the last five years reported on the use of different types of nanomaterials (mainly carbon-based nanomaterials and NPs) as electrode modifiers. Polymers (including biopolymers) have found growing interest in modifying the electrodes through film-formation, as sole modifiers, or in combination with other compounds/materials. Although film electrodes have been among the first alternatives to replace mercury electrodes for stripping analysis of heavy metals, even in the last five years, film formation has continued to be one of the most used alternatives for electrode modification. In addition to pure metallic and polymeric film-modified electrodes, there are a growing number of reports on the combination of different materials and/or compounds as composite materials.
The formation of these composites is not only seen in the case of film-modified electrodes, but also with other main groups of modifiers discussed above. This is, of course, a desired outcome as it increases the possibility of developing new electrodes and/or leads to their improved sensitivity and analytical performance. However, many of these studies do not report on the contribution of the components of these composites to the analytical performance of the obtained modified electrode. It would be of great interest to the research community that the authors present the mechanism behind heavy metal determination with the modified electrodes. The latter is rarely discussed. Moreover, in some cases, the number of components involved combined with the procedures used to prepare the composites and/or apply them on the surface of the electrodes makes it difficult to scale the production of these electrodes. In several studies, the authors claim that the development of environmentally friendly electrodes is either due to the modifiers used or the preparation and modification procedure. However, no evaluation of toxicity has been presented in these studies. This is important, especially in the combination of several modifiers to form composites. Environmentally friendly compounds and natural products have also been used in the modification of electrodes, either included in the composite materials or as precursors to other materials (mainly NPs) used to modify the electrodes.
The modifications have been mostly applied to GCEs, followed by SPEs and CPEs. In addition, the modification of several other electrodes, including PGEs, Au electrodes, stainless steel electrodes, and indium tin oxide electrodes, has been reported. Only a few studies conducted in the last five years reported on the modification of 3D printed electrodes. This can be attributed to the pretreatment methods needed and the available materials that can be used to overcome the poor conductivity of such electrodes. In that respect, the modification of these types of electrodes presents a challenging area of research. However, due to their ease of preparation and modification, low cost, and portability, in combination with their high sensitivity and various applications in real samples, it would be of great interest for future research to focus more on the modification of SPEs and CPEs.
The modifiers’ application on the electrode surface is mainly done through drop-casting. The modified electrodes have been successfully employed in the individual or simultaneous determination of heavy metals, primarily in diverse water samples (i.e., tap, river, lake, sea, bay, spring, mountain, ground, pond, well, and reservoir water) as well as in wastewater, body fluids, and in food and drinks. There are only a few reports on using the obtained modified electrodes for heavy metal determination in soil samples.
The research work published in the last five years has been focused on the determination of the most common and most problematic heavy metals (i.e., Hg2+, Pb2+, Cd2+, Cu2+, etc.). Other analytes of interest included Zn2+, Cr2+, Cr3+, Cr6+, and Tl+. Most of these studies reported the development of electrodes for multiple ion determination (mostly the simultaneous determination of Pb2+ and Cd2+). However, in some cases, when the modified electrodes can be used in the determination of multiple ions, the authors reported only on the individual determination of the respective heavy metals. The simultaneous determination of several heavy metals is of great importance and is always sought, especially when analyzing complex samples.
Acetate and phosphate buffers are by far the most employed supporting electrolytes in these studies. The pH of these supporting electrolytes varied in the range of 2.0–6.6 for the acetate buffers and 3.5–12.0 for the phosphate buffers. Other solutions, including acids (i.e., HCl and H2SO4 in the 0.01–1 M and 0.1 or 0.5 M concentration range, respectively), Britton–Robinson buffer (mainly at pH = 2.0–4.0), and 0.01 or 0.1 M ammonia buffer solution (pH = 8.5 or 9.2), are also reported. 2-[4-(2-Hydroxyethyl)-1-piperazinyl]-ethanesulfonic acid (pH = 6.5 or 7.0) and 3-[(2-mercapto-vinyl)-hydrazono]-1,3-dihydro-indol-2-one (pH = 9–10) are among the organic compounds employed as supporting electrolytes for the simultaneous determination of Pb2+, Cd2+, Zn2+, and Cu2+.
The stability, reproducibility, and selectivity of the modified electrodes in the presence of possible interferents are important factors for their application in real sample analysis. The majority of studies do not report on electrode stability. The influence of a wide range of possible interferents on the selectivity of the modified electrodes has been studied. These interferents vary from the main cations and anions (i.e., Zn2+, Ni2+, Co2+, Fe2+, Fe3+, Cu2+, Ca2+, Mg2+, Mn2+, NH4+, Na+, K+, Cl−, NO3−, and SO42−) to organic compounds (mainly surfactants) within 1–10
000 times the concentration of the analytes. Moreover, depending on the type of the sample analyzed, the possible interference of cationic and anionic surfactants (i.e., sodium dodecyl sulfate, cetyltrimethylammonium bromide, and Triton X-100) and other organic compounds (mainly citric, uric, tannic and ascorbic acids) has also been investigated. However, in the vast majority of reports, the interference effect is not studied for all the possible interferents that can be found in real sample analysis other than in certified reference materials. The most commonly reported interference is from Cu2+ and Hg2+ in the determination of Pb2+ and Cd2+, respectively. The addition of ferrocyanide decreased the interference effect of the Cu2+ ions.
Frequently, the authors report on one-by-one optimization of the experimental parameters. The problem with such optimization (often first the accumulation time and then the deposition time, followed by the solution composition) is that the optimum conditions in most cases cannot be met. The determination of the optimal deposition potential at a fixed accumulation time in a certain solution would result in the local improvement of e.g., sensitivity. However, when the authors fix the so-called optimized deposition potential, the other parameters change. As a result, this procedure does not lead to the optimum conditions but only to local improvements. Moreover, the authors frequently considered an increase in sensitivity as the optimization criterion. Nevertheless, the optimization of sensitivity usually leads to a narrower linear concentration range (which is of course not desired) and a different precision and accuracy of the method. The best optimization criteria should simultaneously increase the width of the linear concentration range, the sensitivity (slope of the calibration curve), the precision (the lowest RSD is desired), the highest accuracy (recovery closest to 100% is desired), and to achieve the lowest LOD and limit of quantification (LOQ) possible.
Finally, increased attention needs to be devoted to the reporting of the analytical parameters of the modified electrodes. Often authors are not consistent when reporting significant figures (number of digits in a value). For example, different numbers of digits are used to report the linear concentration range (e.g., 0.1–1 nM or 31.25–2000 μg L−1 or 0.02–2 ppb) and recovery (e.g., 98.02–105.0% or 94.2–106%). Moreover, some authors report concentrations that have a high degree of uncertainty (e.g., 0.289 μg L−1), and therefore are not acceptable to the analytical chemistry community.
Abbreviations
AAS | Atomic absorption spectroscopy |
ABS | Acetate buffer solution |
BiFE | Bismuth film electrode |
CCE | Carbon cloth electrode |
CMC | Carboxymethyl cellulose |
CNT | Carbon nanotube |
COF | Covalent–organic framework |
CPE | Carbon paste electrode |
EDTA | 2,2′,2′′,2′′′-(Ethane-1,2-diyldinitrilo)tetraacetic acid |
GCE | Glassy carbon electrode |
GO | Graphene oxide |
IIP | Ion imprinted polymer |
ICP MS | Inductively coupled plasma mass spectrometry |
LOD | Limit of detection |
LOQ | Limit of quantification |
MOF | Metal–organic framework |
MILs | Materials of Institute Lavoisier |
MWCNTs | Multiwalled carbon nanotubes |
NMs | Nanomaterials |
NPs | Nanoparticles |
PANI | Polyaniline |
PBS | Phosphate buffer solution |
PDA | Polydopamine |
PEDOT | Poly(3,4-ethylenedioxythiophene) |
PGE | Pencil graphite electrode |
PPy | Polypyrrole |
rGO | Reduced graphene oxide |
SPE | Screen-printed electrode |
SWASV | Square wave anodic stripping voltammetry |
SWCNTs | Single walled carbon nanotubes |
UiO | Universitetet i Oslo |
ZIFs | Zeolitic imidazolate frameworks |
Conflicts of interest
There are no conflicts to declare.
Acknowledgements
The financial support received from the Slovenian Research Agency for program P2-0118 and projects J1-4416 and NK-0001 is hereby gratefully acknowledged.
References
-
S. Mishra, R. N. Bharagava, N. More, A. Yadav, S. Zainith, S. Mani and P. Chowdhary, in Environmental Biotechnology: For Sustainable Future, ed. R. C. Sobti, N. K. Arora and R. Kothari, Springer Singapore, Singapore, 2019, pp. 103–125, DOI:10.1007/978-981-10-7284-0_5.
- X. Wu, S. J. Cobbina, G. Mao, H. Xu, Z. Zhang and L. Yang, Environ. Sci. Pollut. Res., 2016, 23, 8244–8259 CrossRef CAS PubMed.
- Z. Rahman and V. P. Singh, Environmental Monitoring and Assessment, 2019, 191, 419 CrossRef PubMed.
- M. S. Sankhla, M. Kumari, M. Nandan, R. Kumar and A. Prashant, Int. J. Curr. Microbiol. App. Sci., 2016, 5, 759–766 CrossRef CAS.
- M. He, L. Huang, B. Zhao, B. Chen and B. Hu, Anal. Chim. Acta, 2017, 973, 1–24 CrossRef CAS PubMed.
- H. Mohammed, S. Sadeek, A. R. Mahmoud and D. Zaky, Microchem. J., 2016, 128, 1–6 CrossRef CAS.
- C. Ariño, N. Serrano, J. M. Díaz-Cruz and M. Esteban, Anal. Chim. Acta, 2017, 990, 11–53 CrossRef PubMed.
- S.-H. Chen, Y.-X. Li, P.-H. Li, X.-Y. Xiao, M. Jiang, S.-S. Li, W.-Y. Zhou, M. Yang, X.-J. Huang and W.-Q. Liu, TrAC, Trends Anal. Chem., 2018, 106, 139–150 CrossRef CAS.
- G. M. S. Alves, L. S. Rocha and H. Soares, Talanta, 2017, 175, 53–68 CrossRef CAS PubMed.
- J. Holmes, P. Pathirathna and P. Hashemi, TrAC, Trends Anal. Chem., 2019, 111, 206–219 CrossRef CAS.
- H. Han and D. Pan, Trends Environ. Anal. Chem., 2021, 29, e00119 CrossRef CAS.
- A. Nsabimana, S. A. Kitte, T. H. Fereja, M. I. Halawa, W. Zhang and G. Xu, Curr. Opin. Electrochem., 2019, 17, 65–71 CrossRef CAS.
- M. Cuartero, Sens. Actuators, B, 2021, 334, 129635 CrossRef CAS.
- Minamata Convention on Mercury, https://www.mercuryconvention.org/.
- A. Abdalla and B. A. Patel, Annu. Rev. Anal. Chem., 2021, 14, 47–63 CrossRef CAS PubMed.
- J. Muñoz and M. Pumera, ChemElectroChem, 2020, 7, 3404–3413 CrossRef.
- J. Mettakoonpitak, K. Boehle, S. Nantaphol, P. Teengam, J. A. Adkins, M. Srisa-Art and C. S. Henry, Electroanalysis, 2016, 28, 1420–1436 CrossRef CAS.
- R. Ding, Y. H. Cheong, A. Ahamed and G. Lisak, Anal. Chem., 2021, 93, 1880–1888 CrossRef CAS PubMed.
- K. Y. Lee, A. Ambrosi and M. Pumera, Electroanalysis, 2017, 29, 2444–2453 CrossRef CAS.
- W. Mazurkiewicz, M. Podrażka, E. Jarosińska, K. K. Valapil, M. Wiloch, M. Jönsson-Niedziółka and E. W. Nery, ChemElectroChem, 2020, 7, 2939–2956 CrossRef CAS.
- L. A. Pradela-Filho, W. B. Veloso, I. V. S. Arantes, J. L. M. Gongoni, D. M. de Farias, D. A. G. Araujo and T. R. L. C. Paixão, Microchim. Acta, 2023, 190, 179 CrossRef CAS PubMed.
- J. G. Walters, S. Ahmed, I. M. Terrero Rodríguez and G. D. O'Neil, Electroanalysis, 2020, 32, 859–866 CrossRef CAS.
- K. A. Alamry, A. Khan and M. A. Hussein, Synth. Met., 2022, 285, 117023 CrossRef CAS.
- I. Albalawi, A. Hogan, H. Alatawi, S. Alsefri and E. Moore, Sens. Actuators, B, 2023, 380, 133273 CrossRef CAS.
- A. F. Al-Hossainy, A. A. I. Abd-Elmageed and A. T. A. Ibrahim, Arabian J. Chem., 2019, 12, 2853–2863 CrossRef CAS.
- M. Baghayeri, H. Alinezhad, M. Fayazi, M. Tarahomi, R. Ghanei-Motlagh and B. Maleki, Electrochim. Acta, 2019, 312, 80–88 CrossRef CAS.
- S. R. Bhardiya, A. Asati, H. Sheshma, A. Rai, V. K. Rai and M. Singh, Sens. Actuators, B, 2021, 328, 129019 CrossRef CAS.
- C.-C. Bi, X.-X. Ke, X. Chen, R. Weerasooriya, Z.-Y. Hong, L.-C. Wang and Y.-C. Wu, Anal. Chim. Acta, 2020, 1100, 31–39 CrossRef CAS PubMed.
- J. Chen, Y. Chen and Y. Liang, Diamond Relat. Mater., 2021, 119, 108591 CrossRef CAS.
- Z. Dahaghin, P. A. Kilmartin and H. Z. Mousavi, J. Mol. Liq., 2018, 249, 1125–1132 CrossRef CAS.
- T. R. Das and P. K. Sharma, Microchem. J., 2019, 147, 1203–1214 CrossRef CAS.
- B. Fall, A. K. D. Diaw, M. Fall, M. L. Sall, M. Lo, D. Gningue-Sall, M. O. Thotiyl, H. J. Maria, N. Kalarikkal and S. Thomas, Mater. Today Commun., 2021, 26, 102005 CrossRef CAS.
- X. Fang, X. Chen, Y. Liu, Q. Li, Z. Zeng, T. Maiyalagan and S. Mao, ACS Appl. Nano Mater., 2019, 2, 2367–2376 CrossRef CAS.
- R. Fu, P. Yu, M. Wang, J. Sun, D. Chen, C. Jin and Z. Li, Microchem. J., 2020, 157, 105076 CrossRef CAS.
- C. Guo, C. Wang, H. Sun, D. Dai and H. Gao, RSC Adv., 2021, 11, 29590–29597 RSC.
- B. Habibi, S. Pashazadeh, L. A. Saghatforoush and A. Pashazadeh, J. Electroanal. Chem., 2021, 888, 115210 CrossRef CAS.
- X. J. Han, Z. C. Meng, H. F. Zhang and J. B. Zheng, Microchim. Acta, 2018, 185, 274 CrossRef PubMed.
- F. Hanif, A. Tahir, M. Akhtar, M. Waseem, S. Haider, M. F. Aly Aboud, I. Shakir, M. Imran and M. F. Warsi, Synth. Met., 2019, 257, 116185 CrossRef CAS.
- F. E.-T. Heakal, M. E. B. Mohamed and M. M. Soliman, Microchem. J., 2020, 155, 104691 CrossRef CAS.
- X. Hou, B. Xiong, Y. Wang, L. Wang and H. Wang, Sensors, 2020, 20, 1322 CrossRef CAS PubMed.
- J. Hu, M. Sedki, Y. Shen, A. Mulchandani and G. Gao, Sens. Actuators, B, 2021, 346, 130474 CrossRef CAS.
- D. Huo, Y. Zhang, N. Li, W. Ma, H. Liu, G. Xu, Z. Li, M. Yang and C. Hou, Anal. Bioanal. Chem., 2022, 414, 1575–1586 CrossRef CAS PubMed.
- H. D. Kiliç and H. Kizil, Anal. Bioanal. Chem., 2019, 411, 8113–8121 CrossRef PubMed.
- E. B. Kim, M. Imran, A. Umar, M. S. Akhtar and S. Ameen, Adv. Compos. Hybrid Mater., 2022, 5, 1582–1594 CrossRef CAS.
- E.-B. Kim, M. Imran, A. Umar, M. S. Akhtar and S. Ameen, Adv. Compos. Hybrid Mater., 2022, 5, 1582–1594 CrossRef CAS.
- P. Lei, Y. Zhou, S. Zhao, C. A. Dong and S. M. Shuang, J. Hazard. Mater., 2022, 435, 129036 CrossRef CAS PubMed.
- G. L. Li, X. M. Qi, Y. Xiao, Y. C. Zhao, K. H. Li, Y. H. Xia, X. Wan, J. T. Wu and C. Yang, Nanomaterials, 2022, 12, 3317 CrossRef CAS PubMed.
- L. Li, Y. Qiu, Y. Feng, Y. Li, K. Wu and L. Zhu, J. Electroanal. Chem., 2020, 865, 114121 CrossRef CAS.
- F. Liendo, A. P. de la Vega, M. J. Aguirre, F. Godoy, A. A. Martí, E. Flores, J. Pizarro and R. Segura, Food Chem., 2021, 130676 Search PubMed.
- X. Lin, Z. Lu, Y. Zhang, B. Liu, G. Mo, J. Li and J. Ye, Microchim. Acta, 2018, 185, 438 CrossRef PubMed.
- S. Liu, T. Wu, F. Li, Q. Zhang, X. Dong and L. Niu, Anal. Methods, 2018, 10, 1986–1992 RSC.
- S. X. Liu, X. N. Liu, B. Z. Xie, X. Liu and H. B. Hu, J. Electrochem. Soc., 2022, 169, 077509 CrossRef CAS.
- M. Lu, Y. Deng, Y. Luo, J. Lv, T. Li, J. Xu, S.-W. Chen and J. Wang, Anal. Chem., 2019, 91, 888–895 CrossRef CAS PubMed.
- Y. Lu, X. Liang, J. Xu, Z. Zhao and G. Tian, Sens. Actuators, B, 2018, 273, 1146–1155 CrossRef CAS.
- Z. Lu, X. Lin, J. Zhang, W. Dai, B. Liu, G. Mo, J. Ye and J. Ye, Electrochim. Acta, 2019, 295, 514–523 CrossRef CAS.
- S. Ma, D. Pan, H. Wei, N. Wang, F. Pan and Q. Kang, Microchem. J., 2019, 151, 104210 CrossRef CAS.
- S. Ma, Q. Zhang, J. Zhu, H. Shi, K. Zhang and Y. Shen, Sens. Actuators, B, 2021, 345, 130383 CrossRef CAS.
- L. A. Machhindra and Y.-K. Yen, Chemosensors, 2022, 10, 209 CrossRef CAS.
- J. Mei, Z. Ying, W. Sheng, J. Chen, J. Xu and P. Zheng, Chem. Pap., 2020, 74, 1027–1037 CrossRef CAS.
- S. H. Mnyipika, T. S. Munonde and P. N. Nomngongo, Membranes, 2021, 11, 517 CrossRef CAS PubMed.
- Z. Mo, H. Liu, R. Hu, H. Gou, Z. Li and R. Guo, Ionics, 2018, 24, 1505–1513 CrossRef CAS.
- L. D. Nguyen, T. C. D. Doan, T. M. Huynh, D. M. T. Dang and C. M. Dang, Microchem. J., 2021, 169, 106627 CrossRef CAS.
- L. D. Nguyen, T. C. D. Doan, T. M. Huynh, V. N. P. Nguyen, H. H. Dinh, D. M. T. Dang and C. M. Dang, Sens. Actuators, B, 2021, 345, 130443 CrossRef CAS.
- A. Nourbakhsh, M. Rahimnejad, M. Asghary and H. Younesi, Microchem. J., 2022, 175, 107137 CrossRef CAS.
- S. T. Palisoc, L. C. D. Estioko and M. T. Natividad, Mater. Res. Express, 2018, 5, 085035 CrossRef.
- S. T. Palisoc, R. I. M. Vitto, M. G. Noel, K. T. Palisoc and M. T. Natividad, Sci. Rep., 2021, 11, 1394 CrossRef CAS PubMed.
- J. X. Pang, H. H. Fu, W. W. Kong, R. Jiang, J. H. Ye, Z. Y. Zhao, J. Hou, K. S. Sun, Y. Zheng and L. Chen, Chem. Eng. J., 2022, 433, 133854 CrossRef CAS.
- J. Pei, X. Yu, Z. Zhang, J. Zhang, S. Wei and R. Boukherroub, Appl. Surf. Sci., 2020, 527, 146761 CrossRef CAS.
- T. Priya, N. Dhanalakshmi, V. Karthikeyan and N. Thinakaran, J. Electroanal. Chem., 2019, 833, 543–551 CrossRef CAS.
- T. Priya, N. Dhanalakshmi, S. Thennarasu, V. Karthikeyan and N. Thinakaran, Chem. Phys. Lett., 2019, 731, 136621 CrossRef CAS.
- T. Priya, N. Dhanalakshmi, S. Thennarasu and N. Thinakaran, Carbohydr. Polym., 2018, 197, 366–374 CrossRef CAS PubMed.
- T. Priya, N. Dhanalakshmi, S. Thennarasu and N. Thinakaran, Carbohydr. Polym., 2018, 182, 199–206 CrossRef CAS PubMed.
- Q. X. Ran, F. F. Sheng, G. R. Chang, M. Zhong and S. X. Xu, Microchem. J., 2022, 175, 107138 CrossRef CAS.
- P. Ruengpirasiri, E. Punrat, O. Chailapakul and S. Chuanuwatanakul, Electroanalysis, 2017, 29, 1022–1030 CrossRef CAS.
- S. Sahoo and A. K. Satpati, Surf. Interfaces, 2021, 24, 101096 CrossRef CAS.
- D. Shi, W. Wu and X. Li, Sens. Bio-Sens. Res., 2021, 34, 100464 CrossRef.
- Y. Song, C. Bian, J. Hu, Y. Li, J. Tong, J. Sun, G. Gao and S. Xia, J. Electrochem. Soc., 2019, 166, B95–B102 CrossRef CAS.
- P. Sun, K. Xu, S. Guang and H. Xu, Microchem. J., 2021, 162, 105883 CrossRef CAS.
- G. Suppan, M. Briones-Macias, E. Pazmino-Arias and C. Zamora-Ledezma, Phys. Status Solidi B, 2021, 258, 2000515 CrossRef CAS.
- V. Suvina, S. M. Krishna, D. H. Nagaraju, J. S. Melo and R. G. Balakrishna, Mater. Lett., 2018, 232, 209–212 CrossRef CAS.
- R. Tekenya, K. Pokpas, N. Jahed and E. I. Iwuoha, Anal. Lett., 2019, 52, 373–398 CrossRef CAS.
- C. T. Wang, W. S. Chen, K. H. Fan, C. Y. Chiang and C. W. Wu, J. Solid State Electrochem., 2022, 26, 2699–2711 CrossRef CAS.
- H. Wang, Y. Yin, G. Zhao, F. Bienvenido, I. M. Flores-Parrad, Z. Q. Wang and G. Liu, Int. J. Agric. Biol. Eng., 2019, 12, 194–200 Search PubMed.
- J. Wang, J. Hu, S. Hu, G. Gao and Y. Song, Sensors, 2020, 20, 1004 CrossRef CAS PubMed.
- J. Wang, P. Yu, K. Kan, H. Lv, Z. Liu, B. Sun, X. Bai, J. Chen, Y. Zhang and K. Shi, Chem. Eng. J., 2021, 420, 130317 CrossRef CAS.
- W.-J. Wang, X.-Y. Lu, F.-Y. Kong, H.-Y. Li, Z.-X. Wang and W. Wang, Microchem. J., 2022, 175, 107078 CrossRef CAS.
- S. Wu, K. Li, X. Dai, Z. Zhang, F. Ding and S. Li, Microchem. J., 2020, 155, 104710 CrossRef CAS.
- F. Xie, Z. Y. Song, M. Yang, W. C. Duan, Y. N. Quan, X. J. Huang, W. Q. Liu and P. H. Xie, Anal. Chim. Acta, 2022, 1200, 339607 CrossRef CAS PubMed.
- Z. Xu, X. Fan, Q. Ma, B. Tang, Z. Lu, J. Zhang, G. Mo, J. Ye and J. Ye, Mater. Chem. Phys., 2019, 238, 121877 CrossRef CAS.
- Y. Ye, L. Wang, K. Liu and J. Li, Microchem. J., 2020, 155, 104749 CrossRef CAS.
- W. Yi, Z. He, J. Fei and X. He, RSC Adv., 2019, 9, 17325–17334 RSC.
- L. Yu, Q. Zhang, B. Yang, Q. Xu, Q. Xu and X. Hu, Sens. Actuators, B, 2018, 259, 540–551 CrossRef CAS.
- H. Yin, H. He, T. Li, M. Hu, W. Huang, Z. Wang, X. Yang, W. Yao, F. Xiao, Y. Wu and Y. Sun, Anal. Chim. Acta, 2023, 1239, 340730 CrossRef CAS PubMed.
- K. M. Hassan, G. M. Elhaddad and M. AbdelAzzem, Microchim. Acta, 2019, 186, 440 CrossRef PubMed.
- I. Sadok and K. Tyszczuk-Rotko, J. Electroanal. Chem., 2018, 808, 204–210 CrossRef CAS.
- Y. Wang, Z. G. Nie, X. Y. Li, R. Wang, Y. Zhao and H. Wang, ACS Sustainable Chem. Eng., 2022, 10, 6082–6093 CrossRef CAS.
- K. Nandy, A. Srivastava, S. Afgan, R. Kumar, D. K. Yadav and V. Ganesan, Polym. Bull., 2022, 80, 4061–4083 CrossRef.
- M. Nodehi, M. Baghayeri and A. Kaffash, Chemosphere, 2022, 301, 134701 CrossRef CAS PubMed.
- Y. Shao, Y. Dong, L. Bin, L. Fan, L. Wang, X. Yuan, D. Li, X. Liu and S. Zhao, Microchem. J., 2021, 170, 106726 CrossRef CAS.
- Z. Dahaghin, P. A. Kilmartin and H. Z. Mousavi, Food Chem., 2020, 303, 125374 CrossRef CAS PubMed.
- L. Oularbi, M. Turmine and M. El Rhazi, Synth. Met., 2019, 253, 1–8 CrossRef CAS.
- Z. Lu, W. Dai, B. Liu, G. Mo, J. Zhang, J. Ye and J. Ye, J. Colloid Interface Sci., 2018, 525, 86–96 CrossRef CAS PubMed.
- P. Sengupta, K. Pramanik and P. Sarkar, Sens. Actuators, B, 2021, 329, 129052 CrossRef CAS.
- J. Lalmalsawmi, Zirlianngura, D. Tiwari, S.-M. Lee and D.-J. Kim, J. Electroanal. Chem., 2021, 115578, DOI:10.1016/j.jelechem.2021.115578.
- D. Kong, J. Yao, X. Li, J. Luo and M. Yang, Ecotoxicol. Environ. Saf., 2020, 204, 111107 CrossRef CAS PubMed.
- H. A. El-Raheem, R. Y. A. Hassan, R. Khaled, A. Farghali and I. M. El-Sherbiny, Microchem. J., 2020, 155, 104765 CrossRef CAS.
- J. Han, R. Fu, C. Jin, Z. Li, M. Wang, P. Yu and Y. Xie, Microchem. J., 2020, 152, 104356 CrossRef CAS.
- S. Palisoc, V. A. Sow and M. Natividad, Anal. Methods, 2019, 11, 1591–1603 RSC.
- S. N. Aurun Kumar, S. Ashoka and M. Pandurangappa, Mater. Res. Express, 2019, 6, 125049 CrossRef.
- T. Zhang, H. Jin, Y. Fang, J. Guan, S. Ma, Y. Pan, M. Zhang, H. Zhu, X. Liu and M. Du, Mater. Chem. Phys., 2019, 225, 433–442 CrossRef CAS.
- M. Wang, R. Fu, C. Jin, Z. Li, J. Sun, P. Yu and M. You, Measurement, 2019, 140, 548–556 CrossRef.
- N. S. A. Kumar, S. Ashoka and P. Malingappa, J. Environ. Chem. Eng., 2018, 6, 6939–6946 CrossRef.
- S. T. Palisoc, M. T. Natividad, N. De Jesus and J. Carlos, Sci. Rep., 2018, 8, 17445 CrossRef PubMed.
- M. G. Trachioti, J. Hrbac and M. I. Prodromidis, Sens. Actuators, B, 2018, 260, 1076–1083 CrossRef CAS.
- M. Jiang, H.-R. Chen, S.-S. Li, R. Liang, J.-H. Liu, Y. Yang, Y.-J. Wu, M. Yang and X.-J. Huang, Environ. Sci.: Nano, 2018, 5, 2761–2771 RSC.
- S. Singh, A. Numan, Y. Zhan, V. Singh, T. Van Hung and N. D. Nam, J. Hazard. Mater., 2020, 399, 123042 CrossRef CAS PubMed.
- C. J. Mei, N. A. Yusof and S. A. Alang Ahmad, Chemosensors, 2021, 9, 157 CrossRef CAS.
- P. S. Adarakatti, A. Siddaramanna and P. Malingappa, Anal. Methods, 2019, 11, 813–820 RSC.
- A. M. A. Rahim and E. M. M. Mahmoud, Anal. Sci., 2023, 39, 179–190 CrossRef CAS PubMed.
- J. Lalmalsawmi, D. Tiwari and D. J. Kim, J. Electroanal. Chem., 2022, 918, 116490 CrossRef CAS.
- P. Kumar, P. K. Sonkar, K. N. Tiwari, A. K. Singh, S. K. Mishra, J. Dixit, V. Ganesan and J. Singh, Environ. Sci. Pollut. Res., 2022, 29, 79995–80004 CrossRef CAS PubMed.
- E. C. Okpara, O. E. Fayemi, E. S. M. Sherif, P. S. Ganesh, B. E. K. Swamy and E. E. Ebenso, Sens. Bio-Sens. Res., 2022, 35, 100476 CrossRef.
- J.-H. Hwang, D. Fox, J. Stanberry, V. Anagnostopoulos, L. Zhai and W. H. Lee, Micromachines, 2021, 12, 649 CrossRef PubMed.
- P. Sanchayanukun and S. Muncharoen, Talanta, 2020, 217, 121027 CrossRef CAS PubMed.
- S. R. Dash, S. S. Bag and A. K. Golder, Sens. Actuators, B, 2020, 314, 128062 CrossRef CAS.
- M. Y. Pudza, Z. Z. Abidin, S. Abdul-Rashid, F. M. Yasin, A. S. M. Noor and J. Abdullah, Environ. Sci. Pollut. Res., 2020, 27, 13315–13324 CrossRef CAS PubMed.
- J. Bastos-Arrieta, A. Florido, C. Pérez-Ràfols, N. Serrano, N. Fiol, J. Poch and I. Villaescusa, Nanomaterials, 2018, 8, 946 CrossRef PubMed.
- M. Malakootian, S. Hamzeh and H. Mahmoudi-Moghaddam, Microchem. J., 2020, 158, 105194 CrossRef CAS.
- C. Yıldız, D. E. Bayraktepe, Z. Yazan and M. Önal, J. Electroanal. Chem., 2022, 910, 116205 CrossRef.
- G. L. Li, X. M. Qi, G. Q. Zhang, S. L. Wang, K. H. Li, J. T. Wu, X. Wan, Y. Liu and Q. Li, Microchem. J., 2022, 179, 107515 CrossRef CAS.
- B. Maleki, M. Baghayeri, M. Ghanei-Motlagh, F. M. Zonoz, A. Amiri, F. Hajizadeh, A. Hosseinifar and E. Esmaeilnezhad, Measurement, 2019, 140, 81–88 CrossRef.
- H. Filik and A. A. Avan, Food Chem., 2019, 292, 151–159 CrossRef CAS PubMed.
- W. Wu, M. Jia, Z. Zhang, X. Chen, Q. Zhang, W. Zhang, P. Li and L. Chen, Ecotoxicol. Environ. Saf., 2019, 175, 243–250 CrossRef CAS PubMed.
- J. Tu, Y. Gan, T. Liang, H. Wan and P. Wang, Sens. Actuators, B, 2018, 272, 582–588 CrossRef CAS.
- Y. Pu, Y. Wu, Z. Yu, L. Lu and X. Wang, Talanta Open, 2021, 3, 100024 CrossRef.
- S. Krishnan, S. Chatterjee, A. Solanki, N. Guha, M. K. Singh, A. K. Gupta and D. K. Rai, ACS Appl. Nano Mater., 2020, 3, 11203–11216 CrossRef CAS.
- C. Fan, L. Chen, R. Jiang, J. Ye, H. Li, Y. Shi, Y. Luo, G. Wang, J. Hou and X. Guo, ACS Appl. Nano Mater., 2021, 4, 4026–4036 CrossRef CAS.
- X.-Y. Xiao, S.-H. Chen, S.-S. Li, J. Wang, W.-Y. Zhou and X.-J. Huang, Environ. Sci.: Nano, 2019, 6, 1895–1908 RSC.
- H. Y. Lv, Z. Y. Teng, S. C. Wang, K. Feng, X. L. Wang, C. Y. Wang and G. X. Wang, Sens. Actuators, B, 2018, 256, 98–106 CrossRef CAS.
- K. Y. Chen, J. K. Li, L. X. Zhang, R. R. Xing, T. F. Jiao, F. M. Gao and Q. M. Peng, Nanotechnology, 2018, 29, 445603 CrossRef PubMed.
- A. G. Ali, M. F. Altahan, A. M. Beltagi, A. A. Hathoot and M. Abdel-Azzem, RSC Adv., 2022, 12, 4988–5000 RSC.
- P. U. A. I. Fernando, E. Alberts, M. W. Glasscott, A. Netchaev, J. D. Ray, K. Conley, R. Patel, J. Fury, D. Henderson, L. C. Moores and G. K. Kosgei, ACS Omega, 2021, 6, 5158–5165 CrossRef CAS PubMed.
- A. Seifi, A. Afkhami and T. Madrakian, J. Appl. Electrochem., 2022, 52, 1513–1523 CrossRef CAS.
- M. H. Motaghedifard, S. M. Pourmortazavi and S. Mirsadeghi, Sens. Actuators, B, 2021, 327, 128882 CrossRef CAS.
- T. L. Hai, L. C. Hung, T. T. B. Phuong, B. T. T. Ha, B.-S. Nguyen, T. D. Hai and V.-H. Nguyen, Microchem. J., 2020, 153, 104456 CrossRef CAS.
- Mounesh and K. R. V. Reddy, Microchem. J., 2021, 160, 105610 CrossRef CAS.
- D. Qin, X. Mamat, Y. Li, X. Hu, H. Cheng and G. Hu, Microchem. J., 2020, 153, 104299 CrossRef CAS.
- T. Priya, N. Dhanalakshmi, S. Thennarasu, S. Pulikkutty, V. Karthikeyan and N. Thinakaran, Food Chem., 2020, 317, 126430 CrossRef CAS PubMed.
- G. Zhao and G. Liu, Sens. Actuators, B, 2019, 288, 71–79 CrossRef CAS.
- R. Tan, P. Jiang, C. Pan, J. Pan, N. Gao, Z. Cai, F. Wu, G. Chang, A. Xie and Y. He, Mikrochim. Acta, 2022, 190, 30 CrossRef PubMed.
- X. Wang, Y. Xu, Y. Li, Y. Li, Z. Li, W. Zhang, X. Zou, J. Shi, X. Huang, C. Liu and W. Li, Food Chem., 2021, 357, 129762 CrossRef CAS PubMed.
- Z. Lu, W. Zhao, L. Wu, J. He, W. Dai, C. Zhou, H. Du and J. Ye, Sens. Actuators, B, 2021, 326, 128957 CrossRef CAS.
- S. Singh, A. Numan, H. H. Somaily, M. M. A. Dawsari, M. H. S. Alqarni, A. Alam and P. Kumar, J. Environ. Chem. Eng., 2021, 9, 106534 CrossRef CAS.
- Y. Zhang, H. Yu, T. Liu, W. Li, X. Hao, Q. Lu, X. Liang, F. Liu, F. Liu, C. Wang, C. Yang, H. Zhu and G. Lu, Anal. Chim. Acta, 2020, 1124, 166–175 CrossRef CAS PubMed.
- G. Zhao and G. Liu, J. Appl. Electrochem., 2019, 49, 609–619 CrossRef CAS.
- A. Rezaei, M. A. Tarighat and K. Mohammadi, J. Mater. Sci.: Mater. Electron., 2019, 30, 13347–13359 CrossRef CAS.
- M. Dali, K. Zinoubi, A. Chrouda, S. Abderrahmane, S. Cherrad and N. Jaffrezic-Renault, J. Electroanal. Chem., 2018, 813, 9–19 CrossRef CAS.
- J. Y. Ma and X. P. Hong, Desalin. Water Treat., 2022, 252, 140–147 CrossRef CAS.
- Z. L. Li, X. R. Liu and S. Y. Li, Iran. J. Chem. Chem. Eng., 2022, 41, 1528–1537 CAS.
- G. S. Ustabasi, I. Yilmaz, M. Ozcan and E. Cetinkaya, Electroanalysis, 2022, 34, 1237–1244 CrossRef CAS.
- V. Anjuran and V. Chinnapiyan, J. Anal. Chem., 2019, 74, 276–285 CrossRef.
- M. Ramalingam, V. K. Ponnusamy and S. N. Sangilimuthu, Microchim. Acta, 2019, 186, 69 CrossRef PubMed.
- G. Boopathy, M. Govindasamy, M. Nazari, S. F. Wang and M. J. Umapathy, J. Electrochem. Soc., 2019, 166, E761–E770 CrossRef.
- S. Chen, J. Yu, Z. Chen, Z. Huang and Y. Song, Anal. Methods, 2021, 13, 5830–5837 RSC.
- K. Zhao, L. Y. Ge and G. Lisak, Environ. Pollut., 2022, 307, 119524 CrossRef CAS PubMed.
- Y. Zhang, Y. Qin, L. Jiao, H. Wang, Z. Wu, X. Wei, Y. Wu, N. Wu, L. Hu, H. Zhong, W. Gu and C. Zhu, Anal. Chim. Acta, 2022, 1235, 340510 CrossRef CAS PubMed.
- S. Singh, A. Pankaj, S. Mishra, K. Tewari and S. P. Singh, J. Environ. Chem. Eng., 2019, 7, 103250 CrossRef CAS.
- L. Oularbi, M. Turmine, F. E. Salih and M. El Rhazi, J. Environ. Chem. Eng., 2020, 8, 103774 CrossRef CAS.
- G. Zhu, J. Su, B. Zhang and J. Liu, J. Electroanal. Chem., 2021, 882, 114976 CrossRef CAS.
- A. Yamuna, C.-Y. Hong, S.-M. Chen, T.-W. Chen, E. A. Alabdullkarem, M. Soylak, M. M. Al-Anazy, M. A. Ali and X. Liu, J. Electroanal. Chem., 2021, 887, 115159 CrossRef CAS.
- Y.-F. Sun, J.-J. Li, F. Xie, Y. Wei and M. Yang, Sens. Actuators, B, 2020, 320, 128355 CrossRef CAS.
- L. Durai and S. Badhulika, Mater. Sci. Eng., C, 2020, 112, 110865 CrossRef CAS PubMed.
- L. Durai and S. Badhulika, Sens. Actuators Rep., 2022, 4, 100097 CrossRef.
- Z. P. Zhong, A. Ali, R. Jamal, R. Simayi, L. Xiang, S. Ding and T. Abdiryim, J. Electroanal. Chem., 2018, 822, 112–122 CrossRef CAS.
- A. Wong, P. A. Ferreira, A. M. Santos, F. H. Cincotto, R. A. B. Silva and M. D. P. T. Sotomayor, Microchem. J., 2020, 158, 105292 CrossRef CAS.
- M. Eswaran, P.-C. Tsai, M.-T. Wu and V. K. Ponnusamy, J. Hazard. Mater., 2021, 418, 126267 CrossRef CAS PubMed.
- L. Li, Y. Feng, Y. Qiu, Y. Li, K. Wu and L. Zhu, Microchem. J., 2020, 155, 104762 CrossRef CAS.
- M. Ghanei-Motlagh and M. Baghayeri, Mater. Chem. Phys., 2022, 285, 126127 CrossRef CAS.
- D. Valera, L. Fernández, G. González, H. Romero, O. Martínez and P. J. Espinoza-Montero, Heliyon, 2021, 7, e07120 CrossRef CAS PubMed.
- H. Xiao, W. Wang, S. Pi, Y. Cheng and Q. Xie, Sens. Actuators, B, 2020, 317, 128202 CrossRef CAS.
- Y. Yao, H. Wu and J. Ping, Food Chem., 2019, 274, 8–15 CrossRef CAS PubMed.
- L. Wang, T. Lei, Z. Ren, X. Jiang, X. Yang, H. Bai and S. Wang, J. Electroanal. Chem., 2020, 864, 114065 CrossRef CAS.
- M. R. Mahmoudian, Y. Alias, P. M. Woi, R. Yousefi and W. J. Basirun, Adv. Powder Technol., 2020, 31, 3372–3380 CrossRef CAS.
- M. R. Mahmoudian, W. J. Basirun, Y. Alias and P. MengWoi, J. Environ. Chem. Eng., 2020, 8, 104204 CrossRef CAS.
- L. Samandari, A. Bahrami, M. Shamsipur, L. Farzin and B. Hashemi, Int. J. Environ. Anal. Chem., 2019, 99, 172–186 CrossRef CAS.
- P. Pathak, J.-H. Hwang, R. H. T. Li, K. L. Rodriguez, M. M. Rex, W. H. Lee and H. J. Cho, Sens. Actuators, B, 2021, 344, 130263 CrossRef CAS.
- S. Wu, X. Dai, T. Cheng and S. Li, Carbohydr. Polym., 2018, 195, 199–206 CrossRef CAS PubMed.
- M. Dib, A. Moutcine, H. Ouchetto, A. Chtaini, A. Hafid and M. Khouili, Inorg. Chem. Commun., 2021, 131, 108624 CrossRef CAS.
- H. Zheng, L. Ntuli, M. Mbanjwa, N. Palaniyandy, S. Smith, M. Modibedi, K. Land and M. Mathe, Electrocatalysis, 2019, 10, 149–155 CrossRef CAS.
- P. Swetha, J. Chen, A. S. Kumar and S.-P. Feng, J. Electroanal. Chem., 2020, 878, 114657 CrossRef CAS.
- A. Numan, A. A. S. Gill, S. Rafique, M. Guduri, Y. Zhan, B. Maddiboyina, L. Li, S. Singh and N. N. Dang, J. Hazard. Mater., 2021, 409, 124493 CrossRef CAS PubMed.
-
A. Lochab, R. Sharma, S. Kumar and R. Saxena, Preston, England, 2020 Search PubMed.
- A. R. Cherian, L. Benny, A. George, A. Varghese and G. Hegde, J. Nanostruct. Chem., 2022, 12, 441–466 CrossRef CAS.
- P. Yan, S. Zhang, C. Zhang, Z. Liu and A. Tang, Microchim. Acta, 2019, 186, 706 CrossRef PubMed.
- C.-T. Wang, W.-S. Chen, K.-H. Fan, C.-Y. Chiang and C.-W. Wu, J. Solid State Electrochem., 2022, 26, 2699–2711 CrossRef CAS.
- N. Baig, M. Sajid and T. A. Saleh, TrAC, Trends Anal. Chem., 2019, 111, 47–61 CrossRef CAS.
- M. Somkid and P. Chooto, J. Electrochem. Sci. Eng., 2019, 9, 153–164 CrossRef CAS.
- M. R. J. Sarvestani, T. Madrakian and A. Afkhami, Talanta, 2022, 250, 123716 CrossRef PubMed.
- N. Pourshirband and A. Nezamzadeh-Ejhieh, Solid State Sci., 2020, 99, 106082 CrossRef CAS.
- P. Wei, Z. Li, X. Zhao, R. Song and Z. Zhu, J. Taiwan Inst. Chem. Eng., 2020, 113, 107–113 CrossRef CAS.
- Y. R. Chu, F. Gao and Q. X. Wang, J. Electroanal. Chem., 2019, 835, 293–300 CrossRef CAS.
- H. Ajab, A. A. A. Khan, M. S. Nazir, A. Yaqub and M. A. Abdullah, Environ. Res., 2019, 176, 108563 CrossRef CAS PubMed.
- H. Ajab, J. O. Dennis and M. A. Abdullah, Int. J. Biol. Macromol., 2018, 113, 376–385 CrossRef CAS PubMed.
- V. K. Singh, C. S. Kushwaha and S. K. Shukla, Int. J. Biol. Macromol., 2020, 147, 250–257 CrossRef CAS PubMed.
- O. Ifguis, A. Moutcine, C. Laghlimi, Y. Ziat, R. Bouhdadi, A. Chtaini, A. Moubarik and M. Mbarki, J. Anal. Methods Chem., 2022, 2022, 5348246 Search PubMed.
- M. Ardalani, M. Shamsipur and A. Besharati-Seidani, J. Electroanal. Chem., 2020, 879, 114788 CrossRef CAS.
- M. A. Deshmukh, R. Celiesiute, A. Ramanaviciene, M. D. Shirsat and A. Ramanavicius, Electrochim. Acta, 2018, 259, 930–938 CrossRef CAS.
- E. Roy, S. Pal, C. W. Kung, S. S. Yu, A. Nagar and C. H. Lin, ChemistrySelect, 2022, 7, e202203574 CrossRef CAS.
- X. Zhao, W. Bai, Y. Yan, Y. Wang and J. Zhang, J. Electrochem. Soc., 2019, 166, B873–B880 CrossRef CAS.
- M. A. Deshmukh, G. A. Bodkhe, S. Shirsat, A. Ramanavicius and M. D. Shirsat, Front. Chem., 2018, 6, 451 CrossRef CAS PubMed.
- H. Zhang, Y. Li, Y. Zhang, J. Wu, S. Li and L. Li, J. Electron. Mater., 2023, 52, 1819–1828 CrossRef CAS.
- N. Wang, W. Zhao, Z. Shen, S. Sun, H. Dai, H. Ma and M. Lin, Sens. Actuators, B, 2020, 304, 127286 CrossRef CAS.
- W. Zhang, S. Fan, X. Li, S. Liu, D. Duan, L. Leng, C. Cui, Y. Zhang and L. Qu, Microchim. Acta, 2019, 187, 69 CrossRef PubMed.
- Z. Ait-Touchente, H. E. E. Y. Sakhraoui, N. Fourati, C. Zerrouki, N. Maouche, N. Yaakoubi, R. Touzani and M. M. Chehimi, Appl. Sci., 2020, 10, 7010 CrossRef CAS.
- Z. Dahaghin, P. A. Kilmartin and H. Z. Mousavi, J. Electroanal. Chem., 2018, 810, 185–190 CrossRef CAS.
- Y. Liu, C. W. Chang, Q. Xue, R. Wang, L. X. Chen, Z. Y. Liu and L. He, Diamond Relat. Mater., 2022, 130, 109477 CrossRef CAS.
- J. M. S. Alshawi, M. Q. Mohammed, H. F. Alesary, H. K. Ismail and S. Barton, ACS Omega, 2022, 7, 20405–20419 CrossRef CAS PubMed.
- A. H. Oghli and A. Soleymanpour, Microchim. Acta, 2022, 189, 121 CrossRef PubMed.
- D. Mao, J. Hu, P. Duan, C. Qin and Y. Piao, Sens. Actuators, B, 2022, 371, 132490 CrossRef CAS.
- M. A. Deshmukh, H. K. Patil, G. A. Bodkhe, M. Yasuzawa, P. Koinkar, A. Ramanaviciene, M. D. Shirsat and A. Ramanavicius, Sens. Actuators, B, 2018, 260, 331–338 CrossRef CAS.
- A. Yifru, G. Dare, T. B. Demissie, S. Mehretie and S. Admassie, Heliyon, 2021, 7, e08215 CrossRef CAS PubMed.
- S. Di Masi, A. Pennetta, A. Guerreiro, F. Canfarotta, G. E. De Benedetto and C. Malitesta, Sens. Actuators, B, 2020, 307, 127648 CrossRef.
- A. Poudel, G. S. S. Sunder, A. Rohanifar, S. Adhikari and J. R. Kirchhoff, J. Electroanal. Chem., 2022, 911, 116221 CrossRef CAS.
- M. T. T. Nguyen, H. L. Nguyen and D. T. Nguyen, Adv. Polym. Technol., 2021, 2021, 6637316 CrossRef.
- M. Palanna, S. Aralekallu, C. P. K. Prabhu, V. A. Sajjan, Mounesh and L. K. Sannegowda, Electrochim. Acta, 2021, 367, 137519 CrossRef CAS.
- L. Liv and N. Nakibolu, Anal. Lett., 2020, 53, 1155–1175 CrossRef CAS.
- R. Manikandan and S. S. Narayanan, Ionics, 2019, 25, 6083–6092 CrossRef CAS.
- T. M. Lima, P. I. Soares, L. A. do Nascimento, D. L. Franco, A. C. Pereira and L. F. Ferreira, Microchem. J., 2021, 168, 106406 CrossRef CAS.
- E. A. Shalaby, A. M. Beltagi, A. A. Hathoot and M. A. Azzem, Electroanalysis, 2022, 34, 523–534 CrossRef CAS.
- K. M. Hassan, S. E. Gaber, M. F. Altahan and M. A. Azzem, Electroanalysis, 2018, 30, 1155–1162 CrossRef CAS.
- C. Bayraktar, H. Cankurtaran and B. F. Senkal, Int. J. Environ. Anal. Chem., 2018, 98, 889–906 CrossRef CAS.
- J. Zhong, H. Zhao, Y. Cheng, T. Feng, M. Lan and S. Zuo, J. Electroanal. Chem., 2021, 902, 115815 CrossRef CAS.
- I. Albalawi, A. Hogan, H. Alatawi and E. Moore, Sens. Bio-Sens. Res., 2021, 34, 100454 CrossRef.
- Y. Chen, D. Zhang, D. Wang, L. Lu, X. Wang and G. Guo, Talanta, 2019, 202, 27–33 CrossRef CAS PubMed.
- N. Colozza, I. Cacciotti, D. Moscone and F. Arduini, Electroanalysis, 2020, 32, 345–357 CrossRef CAS.
- D. Li, X. Qiu, H. Guo, D. Duan, W. Zhang, J. Wang, J. Ma, Y. Ding and Z. Zhang, Environ. Res., 2021, 202, 111605 CrossRef CAS PubMed.
- Y. Li, T. Xia, J. Zhang, Y. Cui, B. Li, Y. Yang and G. Qian, J. Solid State Chem., 2019, 275, 38–42 CrossRef CAS.
- L. D. Nguyen, T. M. Huynh, T. S. V. Nguyen, D. N. Le, R. Baptist, T. C. D. Doan and C. M. Dang, J. Electroanal. Chem., 2020, 873, 114396 CrossRef CAS.
- S. Palisoc, R. I. M. Vitto and M. Natividad, Sci. Rep., 2019, 9, 18491 CrossRef CAS PubMed.
- L. Y. Yu, Q. Zhang, B. R. Yang, Q. Xu and X. Y. Hu, Sens. Actuators, B, 2018, 259, 540–551 CrossRef CAS.
- Y. Zhang, C. Li, Y. Su, W. Mu and X. Han, Inorg. Chem. Commun., 2020, 111, 107672 CrossRef CAS.
- G. Li, T. Belwal, Z. Luo, Y. Li, L. Li, Y. Xu and X. Lin, Food Chem., 2021, 356, 129632 CrossRef CAS PubMed.
- K. Tyszczuk-Rotko, Curr. Opin. Electrochem., 2019, 17, 128–133 CrossRef CAS.
- J. Wang, J. Lu, S. B. Hocevar, P. A. M. Farias and B. Ogorevc, Anal. Chem., 2000, 72, 3218–3222 CrossRef CAS PubMed.
- L. R. G. Silva, Y. S. Mutz, J. S. Stefano, C. A. Conte-Junior and R. d. Q. Ferreira, J. Food Compos. Anal., 2022, 110, 104564 CrossRef CAS.
- Y. Ai, L. Yan, S. Zhang, X. Ye, Y. Xuan, S. He, X. Wang and W. Sun, Microchem. J., 2023, 184, 108186 CrossRef CAS.
- S. E. D. Bahinting, A. P. Rollon, S. Garcia-Segura, V. C. C. Garcia, B. M. B. Ensano, R. R. M. Abarca, J.-J. Yee and M. D. G. de Luna, Sensors, 2021, 21, 1811 CrossRef CAS PubMed.
- H. Li, J. Zhao, S. Zhao and G. Cui, Microchem. J., 2021, 168, 106390 CrossRef CAS.
- C. Rojas-Romo, M. E. Aliaga, V. Arancibia and M. Gomez, Microchem. J., 2020, 159, 105373 CrossRef CAS.
- W. Seanghirun, K. Samoson, S. Cotchim, S. Kongkaew and W. Limbut, Microchem. J., 2020, 157, 104958 CrossRef CAS.
- N. M. Thanh, N. D. Luyen, T. T. T. Toan, N. H. Phong and N. Van Hop, J. Anal. Methods Chem., 2019, 2019, 4593135 Search PubMed.
- K. Domańska and K. Tyszczuk-Rotko, Anal. Chim. Acta, 2018, 1036, 16–25 CrossRef PubMed.
- E. A. Al-Harbi and M. S. El-Shahawi, Electroanalysis, 2018, 30, 1829–1838 CrossRef CAS.
- B. Petovar, K. Xhanari and M. Finšgar, Anal. Chim. Acta, 2018, 1004, 10–21 CrossRef CAS PubMed.
- Q. M. Jiang, M. R. Zhang, F. Hou, Z. G. Wang, S. H. Zhang, Y. Chen, L. Q. Luo and G. B. Pan, J. Electroanal. Chem., 2018, 809, 105–110 CrossRef CAS.
- G. Zhao, H. Wang and G. Liu, RSC Adv., 2018, 8, 5079–5089 RSC.
- K. C. Bedin, E. Y. Mitsuyasu, A. Ronix, A. L. Cazetta, O. Pezoti and V. C. Almeida, Int. J. Anal. Chem., 2018, 2018, 1473706 CrossRef PubMed.
- H. Wang, G. Zhao, Y. Yin, Z. Wang and G. Liu, Sensors, 2018, 18, 6 CrossRef PubMed.
- R. Z. Ouyang, L. N. Xu, H. F. Wen, P. H. Cao, P. P. Jia, T. Lei, X. Zhou, M. Tie, X. L. Fu, Y. F. Zhao, H. Z. Chang and Y. Q. Miao, Int. J. Electrochem. Sci., 2018, 13, 1423–1440 CrossRef CAS PubMed.
- A. Sánchez-Calvo, M. C. Blanco-López and A. Costa-García, Biosensors, 2020, 10, 52 CrossRef PubMed.
- S. Christidi, A. Chrysostomou, A. Economou, C. Kokkinos, P. R. Fielden, S. J. Baldock and N. J. Goddard, Sensors, 2019, 19, 4809 CrossRef PubMed.
- M. Finšgar, K. Xhanari and B. Petovar, J. Electrochem. Soc., 2019, 166, H108–H118 CrossRef.
- K. Xhanari, A. Ramot, B. Petovar and M. Finšgar, 7th International Conference on Advanced Materials and Systems, 2018, 483–488 Search PubMed.
- M. Finšgar, D. Majer, U. Maver and T. Maver, Sensors, 2018, 18, 3976 CrossRef PubMed.
- N. I. Hrastnik, V. Jovanovski and S. B. Hočevar, Sens. Actuators, B, 2020, 307, 127637 CrossRef.
- M. Finšgar, K. Xhanari and B. Petovar, Microchem. J., 2019, 147, 863–871 CrossRef.
- V. Jovanovski and N. I. Hrastnik, Microchem. J., 2019, 146, 895–899 CrossRef CAS.
- D. Lu, C. Sullivan, E. M. Brack, C. P. Drew and P. Kurup, IEEE Sens. J., 2021, 21, 2485–2494 CrossRef CAS PubMed.
- J. Wasąg and M. Grabarczyk, Talanta, 2021, 233, 122565 CrossRef PubMed.
- M. Finšgar and L. Kovačec, Microchem. J., 2020, 154, 104635 CrossRef.
- M. Finšgar and K. Jezernik, Sensors, 2020, 20, 3921 CrossRef PubMed.
- M. Finšgar, B. Petovar and K. Vodopivec, Microchem. J., 2019, 145, 676–685 CrossRef.
- C. Yıldız, D. E. Bayraktepe and Z. Yazan, Monatsh. Chem., 2021, 152, 1527–1537 CrossRef.
- M. F. Altahan, A. G. Ali, A. A. Hathoot and M. Abdel-Azzem, J. Electrochem. Soc., 2020, 167, 127510 CrossRef CAS.
- R. C. Urban, J. R. Romero and M. L. A. M. Campos, Anal. Methods, 2018, 10, 2056–2063 RSC.
- A. Toghan, M. Abd-Elsabour and A. M. Abo-Bakr, Sens. Actuators, A, 2021, 322, 112603 CrossRef CAS.
- K. M. Hassan, S. E. Gaber, M. F. Altahan and M. A. Azzem, Sens. Bio-Sens. Res., 2020, 29, 100369 CrossRef.
- L. Wu, X. H. Liu, X. Yu, S. J. Xu, S. X. Zhang and S. M. Guo, Materials, 2022, 15, 6013 CrossRef CAS PubMed.
- R. H. Huang, Z. Ke, H. W. Sun, D. F. Zhang, J. Zhu, S. X. Zhou, W. Y. Li, Y. T. Li, C. Q. Wang, X. X. Jia, T. Wagberg and G. Z. Hu, Anal. Chim. Acta, 2022, 1228, 340309 CrossRef CAS PubMed.
- S. Theerthagiri, P. Rajkannu, P. S. Kumar, P. Peethambaram, C. Ayyavu, R. Rasu and D. Kannaiyan, Food Chem. Toxicol., 2022, 167, 113313 CrossRef CAS PubMed.
- F.-F. Wang, C. Liu, J. Yang, H.-L. Xu, W.-Y. Pei and J.-F. Ma, Chem. Eng. J., 2022, 438, 135639 CrossRef CAS.
- Y. Zhu, S. Zhou, J. Zhu, P. Wang, X. Wang, X. Jia, T. Wågberg and G. Hu, Ecotoxicol. Environ. Saf., 2022, 243, 113987 CrossRef CAS PubMed.
- J. Ru, X. Wang, X. Cui, F. Wang, H. Ji, X. Du and X. Lu, Talanta, 2021, 234, 122679 CrossRef CAS PubMed.
- X. Chen, J.-X. Zhao, J.-W. Wang, Y. Liu, L.-C. Wang, R. Weerasooriya and Y.-C. Wu, Electrochim. Acta, 2021, 387, 138539 CrossRef CAS.
- X. Wang, Y. Qi, Y. Shen, Y. Yuan, L. Zhang, C. Zhang and Y. Sun, Sens. Actuators, B, 2020, 310, 127756 CrossRef CAS.
- L. Ma, X. Zhang, M. Ikram, M. Ullah, H. Wu and K. Shi, Chem. Eng. J., 2020, 395, 125216 CrossRef CAS.
- S. Sakthinathan, P. Tamizhdurai, A. Ramesh, T.-W. Chiu, V. L. Mangesh, S. Veerarajan and K. Shanthi, Microporous Mesoporous Mater., 2020, 292, 109770 CrossRef CAS.
- W. Ye, Y. Li, J. Wang, B. Li, Y. Cui, Y. Yang and G. Qian, J. Solid State Chem., 2020, 281, 121032 CrossRef CAS.
- C. Xu, J. Liu, Y. Bi, C. Ma, J. Bai, Z. Hu and M. Zhou, Anal. Chim. Acta, 2020, 1116, 16–26 CrossRef CAS PubMed.
- J.-Q. Niu, W.-T. An, X.-J. Zhang, Y.-Y. Ma and Z.-G. Han, Chem. Eng. J., 2021, 418, 129408 CrossRef CAS.
- B. Niu, B. Yao, M. Zhu, H. Guo, S. Ying and Z. Chen, J. Electroanal. Chem., 2021, 886, 115121 CrossRef CAS.
- O. A. Fouad, A. E. Ali, G. G. Mohamed and N. F. Mahmoud, Microchem. J., 2022, 175, 107228 CrossRef CAS.
- H. Liu, J. Wang, H. Jin, M. Wei, W. Ren, Y. Zhang, L. Wu and B. He, Sens. Actuators, B, 2021, 329, 129215 CrossRef CAS.
- A. D. Pournara, A. Margariti, G. D. Tarlas, A. Kourtelaris, V. Petkov, C. Kokkinos, A. Economou, G. S. Papaefstathiou and M. J. Manos, J. Mater. Chem. A, 2019, 7, 15432–15443 RSC.
- E. Shi, G. Yu, H. Lin, C. Liang, T. Zhang, F. Zhang and F. Qu, Microchim. Acta, 2019, 186, 451 CrossRef PubMed.
- H. Yang, C. Peng, J. Han, Y. Song and L. Wang, Sens. Actuators, B, 2020, 320, 128447 CrossRef CAS.
- J. Wan, Y. Shen, L. Xu, R. Xu, J. Zhang, H. Sun, C. Zhang, C. Yin and X. Wang, J. Electroanal. Chem., 2021, 895, 115374 CrossRef CAS.
- C. Wang, L. Pei, R. Chen, Y. Zhu and J. Su, Ionics, 2022, 28, 4025–4033 CrossRef CAS.
- L. Pei, J. Su, H. Yang, Y. Wu, Y. Du and Y. Zhu, Microporous Mesoporous Mater., 2022, 333, 111742 CrossRef CAS.
- J. Han, J. Yu, Y. Guo, L. Wang and Y. Song, Sens. Actuators, B, 2020, 321, 128498 CrossRef CAS.
- T. Zhang, C. W. Gao, W. Huang, Y. L. Chen, Y. Wang and J. M. Wang, Talanta, 2018, 188, 578–583 CrossRef CAS PubMed.
- M. R. J. Sarvestani, T. Madrakian and A. Afkhami, Food Chem., 2023, 402, 134246 CrossRef PubMed.
- N. Kajal, V. Singh, R. Gupta and S. Gautam, Environ. Res., 2022, 204, 112320 CrossRef CAS PubMed.
- H. R. Abuzeid, A. F. M. El-Mahdy and S.-W. Kuo, Giant, 2021, 6, 100054 CrossRef CAS.
- S. Sultan, A. Shah, B. Khan, R. Qureshi, J. I. Al-Mutawah, M. R. Shah and A. H. Shah, J. Electrochem. Soc., 2019, 166, B1719–B1726 CrossRef CAS.
- L. Liu, K. Zhang and Y. Wei, New J. Chem., 2019, 43, 1544–1550 RSC.
- M. B. Kocer, Z. O. Erdogan, M. Oguz, S. Kucukkolbasi and M. Yilmaz, Org. Commun., 2019, 12, 160–168 CrossRef CAS.
- J. Pizarro, E. Flores, V. Jimenez, T. Maldonado, C. Saitz, A. Vega, F. Godoy and R. Segura, Sens. Actuators, B, 2019, 281, 115–122 CrossRef CAS.
- S. Aziz, R. Zawawi and S. A. A. Ahmad, Electroanalysis, 2018, 30, 533–542 CrossRef.
- E. Tesfaye, B. S. Chandravanshi, N. Negash and M. Tessema, Sens. Bio-Sens. Res., 2022, 38, 100520 CrossRef.
- S. E. Hashemi, M. Payehghadr, Z. Es'haghi and H. Kargar, Int. J. Environ. Anal. Chem., 2019, 99, 1148–1163 CrossRef CAS.
- C. J. Mei and S. A. A. Ahmad, Arabian J. Chem., 2021, 14, 103303 CrossRef.
- T. Jackfama, M. Moyo, T. Nharingo, M. Shumba and J. Okonkwo, Int. J. Environ. Anal. Chem., 2019, 99, 47–60 CrossRef CAS.
- J. Estrada-Aldrete, J. M. Hernández-López, A. M. García-León, J. M. Peralta-Hernández and F. J. Cerino-Córdova, J. Electroanal. Chem., 2020, 857, 113663 CrossRef CAS.
- M. Z. M. Mendonça, F. M. de Oliveira, J. M. Petroni, B. G. Lucca, R. A. B. da Silva, V. L. Cardoso and E. I. de Melo, J. Appl. Electrochem., 2023, 53, 1461–1471 CrossRef.
- L. Hermouche, Y. Aqil, K. Abbi, Y. El Hamdouni, F. Ouanji, S. El Hajjaji, M. El Mahi, E. m. Lotfi and N. Labjar, Chem. Data Collect., 2021, 32, 100642 CrossRef CAS.
- Y. El Hamdouni, S. El Hajjaji, T. Szabo, L. Trif, I. Felhosi, K. Abbi, N. Labjar, L. Harmouche and A. Shaban, Arabian J. Chem., 2022, 15, 104252 CrossRef CAS.
- A. M. A. Rahim and E. M. M. Mahmoud, Anal. Sci., 2023, 39, 179–190 CrossRef CAS PubMed.
- L. G. Djemmoe, E. Njanja, F. M. M. Tchieno, D. T. Ndinteh, P. G. Ndungu and I. K. Tonle, Int. J. Environ. Anal. Chem., 2020, 100, 1429–1445 CrossRef CAS.
- C. Pal and S. Majumder, Mater. Today: Proc., 2020, 29, 1129–1131 CrossRef CAS.
- W. C. Zhang, P. Zhang, Y. Liang, W. Cheng, L. F. Li, H. M. Wang, Z. D. Yu, Y. Liu and X. W. Zhang, RSC Adv., 2022, 12, 13448–13455 RSC.
- A. Mourya, S. K. Sinha and B. Mazumdar, Microchem. J., 2019, 147, 707–716 CrossRef CAS.
|
This journal is © The Royal Society of Chemistry 2023 |
Click here to see how this site uses Cookies. View our privacy policy here.