DOI:
10.1039/C8AN01908H
(Paper)
Analyst, 2019,
144, 439-447
A two-photon ratiometric fluorescent probe for highly selective sensing of mitochondrial cysteine in live cells†
Received
5th October 2018
, Accepted 29th October 2018
First published on 1st November 2018
Abstract
We report herein a two-photon ratiometric fluorescent probe (DNEPI) for mitochondrial cysteine (Cys) detection on the basis of a merocyanine (compound 1) as the two-photon fluorophore and a 2,4-dinitrobenzensulfonyl (DNBS) unit as the biothiol reaction site. Upon reaction with Cys in DMSO/PBS (1/1, v/v), DNEPI showed a distinct ratiometric fluorescence emission characteristic (F583 nm/F485 nm) linearly proportional to Cys concentrations over the range of 2–10 μM, which was attribute to the enhanced intramolecular charge transfer (ICT) effect by cleavage of the sulfonic acid ester bond of DNEPI to release compound 1. More importantly, the probe could detect Cys with a fast response time (within 2 min) and the detection limit was quantitatively calculated as 0.29 μM. Furthermore, DNEPI not only exhibited high selectivity toward Cys over other similar biothiols, including homocysteine (Hcy) and glutathione (GSH), but also displayed significant mitochondrial-targeting ability, which were favorable for mitochondrial Cys-selective imaging. Subsequently, application of DNEPI to Cys imaging in live cells was successfully achieved by two-photon fluorescence microscopy, suggesting that the probe proposed here could be used to monitor mitochondrial Cys concentration changes in live cells with negligible interference from other biological thiols.
1. Introduction
Mitochondria are vital organelles in most eukaryotic cells, which produce reactive oxygen species (ROS), and their oxidative damage is closely related to cell apoptosis.1 In order to avoid oxidative stress damage, a significant number of free radical scavengers, such as mitochondrial cysteine (Cys), a crucial antioxidant reservoir, play essential roles in maintaining ROS homeostasis and exerting protective effects against oxidative damage.2 In addition, Cys has been associated with the process of mitochondrial protein turnover.2h,3 Therefore, it is crucial to develop mitochondrial Cys probes and to apply them to monitor mitochondrial Cys level in living cells.
Of the popular techniques reported, fluorescent probes have attracted much more attention owing to their non-invasiveness, simple operation, fast response time, excellent sensitivity and real-time detection. So far, a number of fluorescent probes for biothiol detection have been developed on the basis of different mechanisms,4 such as Michael addition to α,β-unsaturated carbonyl compounds,4,5 thiol-halogen/aromatic nucleophilic substitution,4,6 –CHO attached fluorophores,4,7 de-protection of 2,4-dinitrobenzenesulfonyl (DNBS)-protected fluorophores,4,8 and demetallation of copper complexes.4,9 Unfortunately, most of the reported fluorescent probes are under one-photon excitation, of which applications are limited in short excitation wavelengths (<500 nm) with regard to deep-tissue imaging owing to the shallow penetration depth (<100 μm).4d,e,10 In contrast, two-photon microscopy (TPM), which employs two near-infrared (NIR) photons as the excitation source, can offer several advantages, including higher spatial resolution, lower phototoxicity, longer observation time and greater tissue penetration depths (>500 μm).4d,e,11 Thus, to facilitate the use of TPM in biomedical research, a wider variety of efficient and application-specific two-photon (TP) fluorescent probes are still strongly needed for various applications. However, till now, only a limited number of TP probes have been designed for intracellular biothiol imaging.4d,e,12 Huang and co-workers reported the first example of a turn-on TP probe for TPM imaging of Cys/Hcy, based on the highly nucleophilic ability of Cys/Hcy to electron-deficient 8-oxoacenaphthopyrrole derivative.12a However, the probe was limited in its ability to target mitochondria. Cho's group designed a TP probe (ASS) for thiols derived from use of 2-methylamino-6-acetylnaphthalene as the fluorophore and a disulfide group as the reaction site for the thiols.12c Colocalization experiments revealed that the probe existed predominately in the mitochondria. However, the probe still shows single-emission intensity changes, which are easily influenced by environmental factors, such as photo bleaching, cell autofluorescence, inhomogeneous cellular distribution, probe distribution, and instrumental performance. However, ratiometric measurement could endow a self-calibration effect for most of the aforementioned interferences, and thus provide a more accurate analysis. Subsequently, the same group developed a reaction-based ratiometric TP probe (SSH-Mito), which consisted of three components: BTDAN as the TP reporter, a disulfide bond as the thiol reaction site, and TPP as the mitochondria-targeting moiety.12d Nevertheless, all these TP probes failed in discriminating mitochondrial Cys over homocysteine (Hcy) and glutathione (GSH) owing to their similar structures and comparable reactivity. Recently, our group designed and synthesized a ratiometric mitochondrial Cys-selective TP probe (ASMI) on the basis of a merocyanine as the fluorophore and an acrylate moiety as the biothiol reaction site, which has been successfully applied to ratiometrically image and detect mitochondrial Cys in live cells without interference from other biological thiols.12i Nevertheless, progress in this field is still strongly hindered. Therefore, it is urgent and challenging to develop novel ratiometric Cys-selective TP probes for monitoring mitochondrial Cys levels in live cells.
In this contribution, we report a two-photon ratiometric fluorescent probe (DNEPI) for mitochondrial Cys-selective detection in live cells. To the best of our knowledge, it is difficult to design ratiometric probes owing to the strong electron-withdrawing effect of the 2,4-dinitrobenzensulfonyl (DNBS) group. However, by combining a merocyanine (compound 1) as the two-photon fluorophore and DNBS as the biothiol reaction site, DNEPI is prepared as the first mitochondria-targeted ratiometric TP probe based on the DNBS group (Scheme 1). The mechanism may be attributed to the enhanced intramolecular charge transfer (ICT) effect by Cys-promoted cleavage of the sulfonic acid ester bond of DNEPI, causing the release of compound 1 and thereby pronouncing red-shift properties in both absorption and emission spectra. More importantly, the probe can detect Cys with a fast response time (within 2 min) and the detection limit was quantitatively calculated as 0.29 μM. Furthermore, DNEPI exhibits high selectivity toward Cys over Hcy and GSH, and displays significant mitochondrial-targeting ability, which are favorable for mitochondrial Cys-selective imaging. Subsequently, application of DNEPI to Cys imaging in live cells was successfully achieved by two-photon fluorescence microscopy, suggesting that the probe proposed here can be used to monitor mitochondrial Cys concentration changes in live cells.
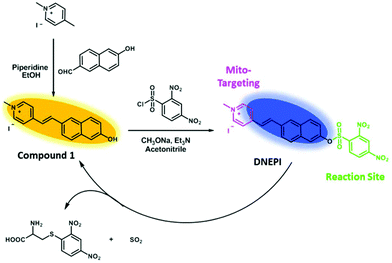 |
| Scheme 1 Synthetic scheme for DNEPI probe and the proposed sensing mechanism of DNEPI with Cys. | |
2. Experimental section
2.1 Materials and apparatus
Unless otherwise stated, all chemicals and solvents were of analytical grade and were used without further purification. 1,4-Dimethylpyridinium iodide and piperidine were purchased from Sigma-Aldrich. 6-Hydroxy-2-naphthaldehyde and 2,4-dinitrobenzenesulfonyl chloride was commercially available from J&K Chemical (Shanghai) Ltd. 3-(4,5-Dimethylthiazol-2-yl)-2,5-diphenyltetrazolium bromide (MTT) was obtained from Thermo-Fisher Biochemical Products (Beijing) Co., Ltd. All other chemicals were bought from Beijing Chemical Reagent Company.
NMR spectra were recorded on a Bruker AVANCE III HD instrument with tetramethylsilane (TMS) as the internal standard in deuterated dimethyl sulfoxide (DMSO-d6) or deuterium oxide (D2O) as the solvent with 600 MHz for 1H NMR and 150 MHz for 13C NMR. LC-MS was done using an Agilent Accurate-Mass-Q-TOF MS 6520 system equipped with an electrospray ionization (ESI) source (Agilent, USA). UPLC-HRMS was carried out on a Q-Exactive high resolution mass spectrometry (Thermo Scientific) with an electrospray ionization (HESI) source. Deionized water was acquired with a Milli-Q water purification system (Millipore). The pH value was obtained using a Beckman Φ50 pH meter (Shanghai LeiCi Device Works, Shanghai, China). The UV-visible spectra were taken on a UV-vis spectrophotometer (Beijing Purkinje General Instrument Co., LTD, Beijing, China). Fluorescence spectra were obtained using a FLS-920 Edinburgh Fluorescence Spectrophotometer (Edinburgh Co., Ltd, England) equipped with a xenon discharge lamp using a 1 ml Fluor Micro Cell. Two-photon fluorescence images were taken using an Olympus FV1000-MPE multiphoton laser scanning confocal microscope (Olympus Co., Ltd, Japan).
2.2 Synthesis and characterization
2.2.1 Synthesis of 4-(2-(6-hydroxy-2-naphthalenyl)ethenyl)-1-methylpyridinium iodide (1).
A mixture of 2.35 g (10 mmol) of 1,4-dimethylpyridinium iodide, 2.58 g (15 mmol) of 6-hydroxy-2-naphthaldehyde and 0.5 mL of piperidine was refluxed in 30 mL of anhydride ethanol for 24 h. The reaction was cooled to room temperature. Then a yellow powder (2.72 g, 7 mmol) was obtained by column chromatography using ethyl dichloromethane/methanol (10/1, v/v) as the eluent with a yield of 70%. 1H NMR (DMSO-d6, 600 MHz), δ (ppm): 10.01 (s, 1H), 8.76 (d, J = 6.7 Hz, 2H), 8.45–8.01 (m, 4H), 7.87–7.69 (m, 3H), 7.50 (d, J = 16.3 Hz, 1H), 7.26–7.11 (m, 2H), 4.23 (s, 3H). 13C NMR (101 MHz, DMSO-d6) δ (ppm): 156.95, 152.66, 144.80, 141.12, 135.67, 130.44, 130.11, 129.66, 127.41, 126.99, 123.91, 123.19, 121.79, 119.43, 109.12, 46.77. LC-MS m/z: [M+] calculated for C18H16NO+, 262.1226; measured, 262.1222.
2.2.2 Synthesis of 4-(2-(6-(2,4-dinitrophenylsulfonyloxy)-2-naphthalenyl)ethenyl)-1-methylpyridinium iodide (DNEPI).
Under N2 atmosphere, a mixture of 1.00 g (2.56 mmol) of 1,4-dimethylpyridineiodide and 0.14 g (2.56 mmol) of sodium methanolate in 50 mL of anhydride methanol was stirred for 4 h at 60 °C. The reaction was cooled to room temperature and the precipitate was collected by filtration, affording a blue solid. Then 1 g (2.43 mmol) of blue solid, 0.78 g (2.92 mmol) of 2,4-dinitrobenzenesulfonyl chloride and 0.26 g of (2.43 mmol) trimethylamine were dissolved in 100 ml of anhydride acetonitrile and heated at 60 °C for 2 h. After evaporation of the solvent, the crude product was purified by silica gel chromatography (CH2Cl2 to CH2Cl2/MeOH (95/5, v/v) to afford a pale yellow powder (1.05 g, 2.1.4 mmol) with a yield of 84%. 1H-NMR (DMSO-d6, 600 MHz) δ 9.16 (d, J = 2.0 Hz, 1H), 8.89 (d, J = 6.5 Hz, 1H), 8.58 (dd, 2H), 8.40 (dd, J = 8.6, 2.1 Hz, 2H), 8.34–8.23 (m, 2H), 8.16 (d, J = 16.2 Hz, 1H), 8.12–8.00 (m, 3H), 7.86 (s, 1H), 7.69 (d, J = 16.4 Hz, 1H), 7.40 (d, J = 8.8 Hz, 1H), 4.27 (s, 3H) ppm. 13C NMR (DMSO-d6, 150 MHz) δ 152.05, 151.47, 148.06, 147.30, 146.86, 145.15, 144.63, 139.80, 133.74, 133.50, 131.80, 131.33, 130.64, 129.19, 128.90, 127.42, 125.54, 124.95, 124.49, 123.60, 121.05, 119.77, 118.22, 46.90 ppm. LC-MS m/z: [M+] calculated for C24H18N3O7S+, 492.0860; measured, 492.0857.
2.3 UV-vis and fluorescence measurements
Doubly distilled water was used to prepare all the aqueous solutions. Stock solutions of DNEPI and compound 1 (1.0 mM) were prepared in DMSO, and the solutions for spectroscopic determination were obtained by diluting the stock solution to a final concentration of 10 μM with DMSO/PBS (1/1, v/v) at pH 7.4. Stock solutions (0.1 M) of the analytes (including biothiols, Na2S, amino acids and ions) were prepared in doubly distilled water. For optical measurements, 2 mL of DNEPI and compound 1 solution were poured into a quartz optical cell of 1 cm optical path length each time. The fluorescent or UV-vis spectra were then recorded upon addition of various analytes. Excitation and emission bandwidths are both set at 2.0 nm, and the excitation wavelength is 370 nm. All spectroscopic experiments are carried out at room temperature.
2.4 Quantum yield measurements
The quantum yields of the probes were measured according to the following equation:
Φx = Φst(Dx/Dst)(Ast/Ax)(ηx2/ηst2) |
where Φst is the reported quantum yield of the standard, D is the area under the emission spectrum, A is the absorbance at the excitation wavelength and η is the refractive index of the solvent used. The subscript x denotes unknown and the subscript st means standard. We chose quinine sulfate solution in 0.1 M H2SO4,12i and perylene in cyclohexane13 as the standards for DNEPI and compound 1, respectively.
2.5 DFT calculations
The calculations in this work were performed with the Gaussian 09 program package. The geometry optimizations and electronic structure calculations were investigated with density functional theory (DFT) at the B3LYP/6-311+G (d, p) level. In each optimization, the vibrational frequencies were calculated and the results showed that all optimized structures were stable geometric structures.
2.6 Cell cytotoxicity assay
The cellular cytotoxicity of DNEPI and compound 1 that formed after reacting with Cys toward the human hepatocellular carcinoma cell line (SMMC7721 cells) was evaluated using the standard MTT assay.14 Cells at a density of 1 × 105 cells per mL were seeded into a 96 well microplate to a total volume of 200 μL per well in a 5% CO2 95% air incubator. After 24 h, different concentrations of DNEPI and their reaction with Cys ([DNEPI] = 0, 1, 5, 10, 15 and 20 μM; [Cys] = 100 μM) were incubated with SMMC7721 cells for 3 h in fresh medium. After washing the cells with PBS (pH 7.4) 3 times, 100 μL of MTT solution (5 mg ml−1 in PBS) was added into each well for another 4 h. Then 150 μL of DMSO was added into each well to dissolve the intracellular blue-violet formazan crystals. The absorbance of the samples was measured at 490 nm wavelength with a microplate reader. The cell viability was calculated using the following equation:
% viability = [∑(Ai/Acontrol × 100)]/n |
where Ai is the absorbance of different probe concentrations of 0, 1, 5, 10, 15 and 20 μM. Acontrol is the average absorbance of the control well in which the probe was absent, and n (= 3) is the number of data points.
2.7 Two-photon excited fluorescent imaging in cells
HeLa cells were grown on a 35 mm diameter round glass Petri dish at a density of 1 × 105 and maintained in an incubator at 37 °C in a humidified atmosphere containing 5% CO2 for 24–36 h. HeLa cells were cultured in RPMI 1640 medium supplemented with 10% heat-inactivated fetal bovine serum (FBS), 100 U mL−1 penicillin, and 100 U mL−1 gentamicin. For colocalization experiments, DNEPI dissolved in DMSO (0.5 μL, 10 mM) was added to the cell medium (500 μL) at a final concentration of 10 μM for 10 min, and then cells were washed with (pH 7.4) 3 times. 1 μM MitoTracker Red was then added and co-incubated for another 30 min, and cell imaging was then carried out after washing cells with PBS 3 times. Then fluorescence images were acquired using an Olympus FV1000-MPE multiphoton laser scanning confocal microscope with the yellow channel (Ex = 750 nm, Em = 510–610 nm) for DNEPI, the green channel (Ex = 580 nm, Em = 620–660 nm) for MitoTracker Red.
For the two-photon ratiometric fluorescent imaging experiments, the SMMC7721 cells were first washed with PBS (pH 7.4), then incubated with DNEPI, N-ethylmaleimide (NEM), thiols (Cys, Hcy or GSH) and H2O2 in an incubator at 37 °C, and then washed with PBS (pH 7.4) 3 times. Then the two-photon fluorescent images were acquired using an Olympus FV1000-MPE multiphoton laser scanning confocal microscope with the blue channel (Ex = 750 nm, Em = 400–500 nm) and the yellow channel (Ex = 750 nm, Em = 510–610 nm), respectively.
3. Results and discussion
3.1 Design and synthesis of the probe DNEPI
As an overall strategy, the merocyanine dye (compound 1) is chosen as a satisfactory fluorophore not only owing to its excellent two-proton property, but also for its strong intramolecular charge transfer (ICT) with “push–pull” substituent pairs. On the other hand, 2,4-dinitrobenzensulfonyl (DNBS) is used as a biothiol reaction site, which is linked to the fluorophore to prepare the probe DNEPI. Owing to its strong electron-withdrawing property, DNBS can block the ICT process of the merocyanine dye. Cys-promoted cleavage of the sulfonic acid ester bond of DNEPI led to the release of the compound 1, increasing the “push–pull” character of the dye and resulting in alteration in the absorption and emission spectra. The synthesis route for DNEPI is displayed in Scheme 1. 1,4-Dimethylpyridinium iodide was first condensed with 6-hydroxy-2-naphthaldehyde by piperidine catalysis, giving compound 1. Reaction of 1 and 2,4-dinitrobenzenesulfonyl chloride in the presence of trimethylamine afforded DNEPI, which was fully characterized by 1H NMR, 13C NMR (Fig. S1, ESI†), and LC-MS analysis (Fig. S2, ESI†). The data obtained were consistent with the proposed structures.
3.2 UV-vis and fluorescence measurements
In the first step we tested the time-dependent sensing behavior of DNEPI for Cys, as a representative biothiol, in DMSO/PBS (1/1, v/v, pH 7.4). As seen in the fluorescence spectra (Fig. 1a and Fig. S3, ESI†), DNEPI itself displayed a very faint blue fluorescent color (inset of Fig. 1a, under irradiation with a hand-help 365 nm lamp) with a fluorescence maximum emission at 485 nm. Nevertheless, the addition of 100 μM Cys to DNEPI solution (10 μM) induced fast and distinct fluorescence quenching at 485 nm with a concomitant increase in fluorescence intensity at 583 nm. Accordingly, a well-defined isoemission point at 502 nm was noticed and the fluorescent color changed to bright yellow (inset of Fig. 1a). Furthermore, the emission intensity attained saturation within only 2 min, indicating a faster response of the reaction as compared to those previously reported. Meanwhile, as shown in the UV-vis spectra (Fig. S4, ESI†), there was a gradual decrease in the absorption at 352 nm (ε = 4.335 × 104 M−1 cm−1), and a simultaneously progressive increase in the absorption at 392 nm (ε = 4.110 × 104 M−1 cm−1) with the addition of Cys. As a consequence, a clear isosbestic point was also noted at 370 nm, and an obvious color change in the solution from colorless to yellow occurred, which could be observed easily by the naked eye (inset of Fig. S4a, ESI†).
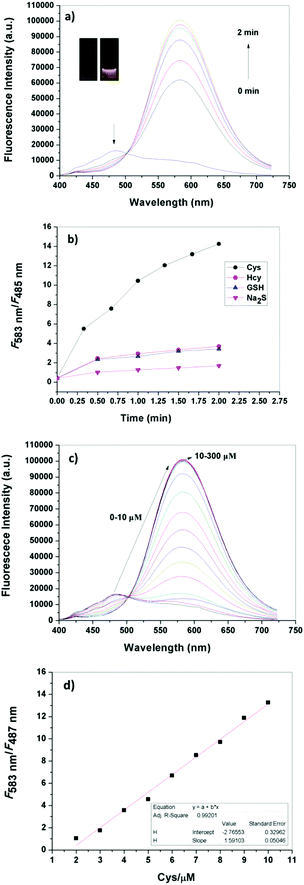 |
| Fig. 1 (a) Fluorescence spectral changes of DNEPI (10 μM) against time in the presence of Cys (100 μM) in DMSO/PBS (1/1, v/v, pH 7.4). Inset: Photograph of DNEPI solution in the absence or presence of Cys under a UV light. (b) Fluorescent kinetics of DNEPI (10 μM) with 100 μM of Cys, Hcy, GSH and Na2S in DMSO/PBS (1/1, v/v, pH 7.4) within 2 min. (c) Fluorescence responses of DNEPI (10 μM) with different concentrations of Cys (0–300 μM) in DMSO/PBS (1/1, v/v, pH 7). (d) A linear relationship of the fluorescence ratio (F583 nm/F485 nm) with Cys concentration in the range of 2–10 μM. | |
DNEPI showed similar optical changes to Hcy, GSH, and Na2S (Fig. S5, ESI†), but with different reactivity characteristics. Herein the time-dependent fluorescence ratio (F583 nm/F485 nm) changes of DNEPI with Cys, Hcy, GSH, and Na2S were also determined. As shown in Fig. 1b and Fig. S6 (ESI†), under the same reaction conditions, DNEPI displayed a more than 34-fold enhancement in the fluorescence intensity ratio (F583 nm/F485 nm) within 2 min in the presence of 10 equiv. of Cys, whereas no more than 8-fold fluorescence enhancement was observed in other biothiols. The time-dependent fluorescent sensing behavior of the probe to Cys, Hcy and GSH was determined in DMSO/PBS (1/99, v/v, pH 7.4), which is more close to cell culture conditions. Although the kinetic properties are solvent dependent, our probe also showed a much higher reactivity resulting in a fast and distinct fluorescence ratio change for Cys in DMSO/PBS (1/99, v/v, pH 7.4) (Fig. S7†). The results indicated that DNEPI exhibited a much higher reactivity, resulting in its potential for application for selective detection of Cys in biosystems.
Moreover, we evaluated the capability of DNEPI for quantitative detection of Cys. The fluorescence and UV-vis responses of DNEPI (10 μM) with different concentrations of Cys (0–300 μM) are shown in Fig. 1c and Fig. S8 (ESI†). The fluorescence ratio (F583 nm/F485 nm) was found to be linearly proportional to the Cys concentration in the range of 2–10 μM, according to the linear regression equation: F583 nm/F485 nm = 1.591 − 2.766CCys with a linear coefficient (R2) of 0.992 (Fig. 1d). More interestingly, the corresponding detection limit based on the IUPAC definition (CDL = 3 Sb m−1) was 0.29 μM from 10 blank solutions, which indicated that DNEPI was more sensitive than most of the reported biothiol probes. Therefore, we think that DNEPI has the potential to ratiometrically image Cys in living cells. In addition, the optical properties of DNEPI and compound 1 in different solvents are summarized in Table S1 (ESI†). The fluorescence lifetime of DNEPI in DMSO/PBS (1/1, v/v, pH 7.4) was determined as 6.28 ns (Fig. S9†).
3.3 Mechanism of probe DNEPI responding to Cys
To explore the fluorescence response mechanism, the interaction between DNEPI and Cys was systematically monitored by UPLC-MS. As shown in Fig. S10 (ESI†), the signal corresponding to DNEPI around 3.0 min decreased markedly, concomitant with a new peak at 2.2 min appearing and increasing gradually over time, which represented the main reaction product corresponding to compound 1. This result was further confirmed by mass spectra analysis. The signal at 3.0 min (m/z 492.0857, Fig. S2, ESI†) and the new peak at 2.2 min (m/z 262.1222, Fig. S2, ESI†) showed characteristic mass information for DNEPI and compound 1, respectively. Further characterization showed that another new peak at m/z 288.0279 could be assigned to the by-product (Fig. S2, ESI†). On the basis of the above observations, we proposed that the fluorescence reaction of DNEPI with Cys in the present system may proceed through the following route (Scheme 1): the strong nucleophilic substitution of Cys promoted the cleavage of the sulfonic acid ester bond of DNEPI, causing the release of compound 1 and thereby pronouncing Cys-dependent red-shift properties in both the absorption and emission spectra.
To obtain more detailed insights into the mechanism, we carried out density functional theory (DFT) calculations program to elucidate the mechanism of the spectral changes that accompany the formation of the putative compound 1 after the addition of Cys to DNEPI. These were implemented at the B3LYP/6-311G (d) level using Gaussian 09 program. As shown in Fig. 2, the HOMO in DNEPI and compound 1 were localized mainly on the merocyanine chromophore core. However, these two species are different at the level of the HOMO/LUMO. The energies of the HOMO and LUMO of compound 1 are much higher than those of DNEPI. More importantly, DFT calculations indicate that the HOMO–LUMO energy gap of compound 1 (2.4169 eV) is significantly lower than that of DNEPI (2.5900 eV), which leads us to predict the recovery of the D–π–A feature of compound 1 and the red-shift character by Cys-promoted cleavage in absorption. Furthermore, the π-electrons of the HOMO and LUMO in compound 1 are more resided on the merocyanine chromophore core, which actually translated to the red-shift change in the emission.15 Thus, the concordance supports the proposed intramolecular charge transfer sensing mechanism for DNEPI.
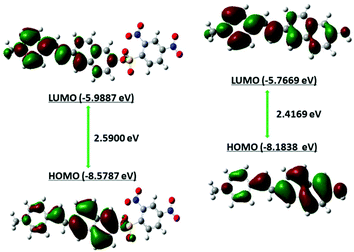 |
| Fig. 2 The frontier molecular orbitals (MOs) of: DNEPI (left two: LUMO and HOMO) and compound 1 (right two: LUMO and HOMO). The energy levels of the MOs are shown (eV). Calculations are calculated at the B3LYP/6-311G (d) level using Gaussian 09 program. | |
3.4 Effect of pH, selectivity and photostability of probe DNEPI toward Cys
To verify whether probe DNEPI is suitable for physiological detection, we evaluated the effect of pH on the fluorescence of DNEPI in the absence or presence of Cys. As shown in Fig. S11 (ESI†), the fluorescence ratio (F583 nm/F485 nm) of DNEPI showed no significant change within the pH range of 3.0–9.0, indicating that the free probe was very stable over a relatively wide pH range. Interestingly, when DNEPI was treated with Cys, there was a gradual ratio enhancement with pH value greater than 5, reaching a maximum around physiological pH of 7.4. These results indicate that DNEPI is able to work within a wide pH range and works the best at physiological pH for responding to Cys, which holds promise for biological applications. Similarly, the best physiological pH for DNEPI responding to Hcy, GSH and Na2S was also pH 7.4 for biological applications.
High selectivity toward Cys over other species is a very important feature to evaluate the performance of the fluorescent probe DNEPI. Subsequently, selectivity experiments were also conducted in the presence of biological thiols, such as Hcy, GSH and Na2S, different amino acids and essential ions. As depicted in Fig. 3, the fluorescence ratio (F583 nm/F485 nm) of DNEPI increased more than 34-fold upon the addition of Cys; in contrast, DNEPI displayed a weak response towards Hcy, GSH and Na2S, which indicated that DNEPI was able to selectively detect cellular Cys from other biological thiols. Moreover, the intensity ratio change was found to be insignificant for different amino acids (Asp, Val, Phe, Tyr, Ala, Ser, Leu, Arg, Pro, Thr, Glu, Try, Iso, Lys, Gly), and essential ions (K+, Na+, Ca2+, Mg2+, Al3+, Zn2+, Cl−, Cu2+, Fe3+, Br−, SO32−, SO42−, CO32−, Ac−, NO3−, NO2−). Therefore, the probe DNEPI is highly selective towards Cys without any interference from other biologically relevant analytes. Furthermore, the photostability of probe DNEPI was studied by continuously monitoring the fluorescence intensity at 485 nm over a period of 2 h. Fig. S12† shows the time course of the fluorescence intensity of the probe in DMSO
:
PBS buffer (1/1 v/v) at pH 7.4 at room temperature, indicating that the probe possesses good photostability.
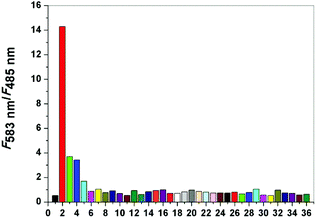 |
| Fig. 3 Fluorescence responses of DNEPI (10 μM) toward thiols and Na2S (100 μM), different amino acids and essential ions (500 μM) after 2 min in DMSO/PBS (1/1, v/v, pH 7.4). (1) Blank; (2) Cys; (3) Hcy; (4) GSH; (5) Na2S; (6) Asp; (7) Val; (8) Phe; (9) Tyr; (10) Ala; (11) Ser; (12) Leu; (13) Arg; (14) Pro; (15) Thr; (16) Glu; (17); Try; (18) Iso; (19) Lys; (20) Gly; (21) K+; (22) Na+; (23) Ca2+; (24) Mg2+; (25) Al3+; (26) Zn2+; (27) Cl−; (28) Cu2+; (29) Fe3+; (30) Br−; (31) SO32−; (32) SO42−; (33) CO32−; (34) Ac−; (35) NO3−; (36) NO2−. | |
3.5 Cell cytotoxicity assay
Since DNEPI is highly selective towards Cys, it is possible to explore its application in intracellular Cys imaging. Before cell imaging, a MTT assay is crucial to evaluate the cytotoxicity of DNEPI in the absence or presence of Cys. Fig. S13 (ESI†) depicts the viability of SMMC7721 cells at various probe concentrations (from 0 to 20 μM) in the absence or presence of Cys. The results demonstrated that more than 90% of cells are viable, showing the low toxicity of DNEPI and compound 1 formed in the presence of Cys under the experimental conditions (10 μM), and inferring their potential application in intracellular imaging of live cells.
3.6 Two-photon excited fluorescence imaging in live cells
To explore the potential of the probe for two-photon excited imaging applications, the two-photon action cross sections (Φσ) of DNEPI and its released fluorophore, compound 1, formed after reaction with Cys were determined by the two-photon induced fluorescence method using a femtosecond pulsed laser in the range of 680–960 nm with fluorescein as the reference. Fig. S14† shows the two-photon action cross section excitation spectra of DNEPI and compound 1. The Φσmax of DNEPI and compound 1 were determined to be 0.65 GM and 5.33 GM (1 GM = 10−50 cm4 s per photo per molecule) in DMSO
:
H2O (1
:
1), respectively.
Since DNEPI is a positively charged fluorophore, it would have a high tendency to accumulate in the mitochondria of a cell owing to the negative membrane potential caused by the proton gradient across the inner mitochondrial membrane.15 To confirm whether DNEPI could specifically stain the mitochondria, a co-localization experiment was conducted with HeLa cells stained with DNEPI and MitoTracker Red, a commercially available one-photon probe for mitochondria targeting.16 As demonstrated in Fig. 4c, the TPM pseudo-color image of DNEPI (Fig. 4a) overlapped well with the OPM red image of MitoTracker Red (Fig. 4b). The average Pearson's co-localization coefficient (A), which described the correlation of the intensity distribution between the two channels of DNEPI with MitoTracker Red, was 0.94 (Fig. 4e). Moreover, the changes in the intensity profile of linear regions of interest (DNEPI and MitoTracker Red co-staining) tended toward synchronization (Fig. 4f). There results indicate that DNEPI has the ability of targeting mitochondria in living cells.
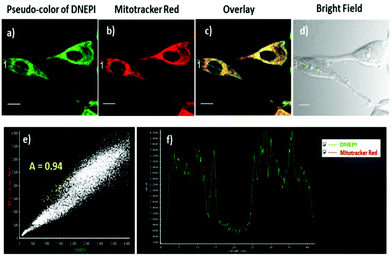 |
| Fig. 4 Two-photon pseudo-colour confocal fluorescence image of 10 μM DNEPI (a) and one-photon image of MitoTracker Red (1 μM) (b) costained in HeLa cells. (c) Overlay image. (d) Bright field image. (e) The correlation of DNEPI and MitoTracker Red intensities (A = 0.94). (f) Intensity profile of linear regions across HeLa cells. The wavelengths for two-photon and one-photon excitation were 750 and 580 nm, respectively, and the corresponding emissions were collected at 510–610 nm (DNEPI) and 620–660 nm (MitoTracker Red). Scale bar: 10 μm. | |
With its intrinsic ability to target mitochondria confirmed, DNEPI was further used as a ratiometric two-photon excited fluorescent probe, and the two-photon images of the SMMC7721 cells stained with DNEPI were monitored with the blue channel (Ex = 750 nm, Em = 400–500 nm) and the yellow channel (Ex = 750 nm, Em = 510–610 nm). As can be seen from Fig. 5a, the DNEPI-stained SMMC7721 cells exhibited almost no two-photon excited fluorescence (TPEF) in the blue channel and bright fluorescence in the yellow channel, resulting from the presence of endogenous Cys in living cells.
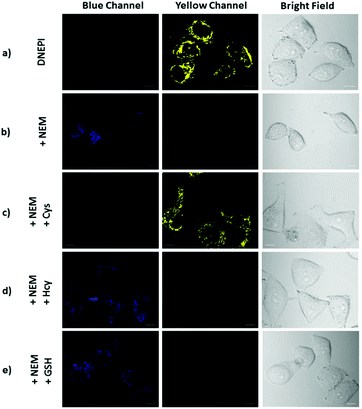 |
| Fig. 5 Two-photon confocal microscopy fluorescence images of biothiols in SMMC7721 cells. (a) SMMC7721 cells stained with DNEPI (10 μM) for 30 min. (b) SMMC7721 cells pretreated with NEM (1.0 mM) for 30 min and then stained with DNEPI (10 μM) for 30 min. (c–e) SMMC7721 cells pretreated with NEM (1.0 mM) for 30 min and then stained with Cys, Hcy, and GSH (100 μM) for 30 min, and finally stained with DNEPI (10 μM) for 30 min. From left to right: Blue channel: Em = 400–500 nm; yellow channel: Em = 510–610 nm; bright field overlay. λex = 750 nm. Scale bar: 10 μm. | |
To assess the potential selective response of DNEPI toward Cys over Hcy and GSH, a series of control experiments were performed. When the SMMC7721 cells were pretreated with N-ethylmaleimide (NEM, 1.0 mM) for 30 min to remove endogenous thiols prior to incubation with DNEPI,17 a weak TPEF in the blue channel was observed, whereas no emission was detected in the yellow channel (Fig. 5b), suggesting that thiol species were completely depleted by NEM. Then with treatment of Cys, Hcy and GSH to the NEM-pretreated SMMC7721 cells for 30 min, prior to imaging with DNEPI, marked variations of fluorescence responses in the two channels were observed. As predicted, the blue TPEF and sharp yellow TPEF were enhanced (Fig. 5c, yellow channel), after the addition of Cys. By contrast, addition of Hcy could only induce a very faint yellow TPEF emission (Fig. 5d, yellow channel), and the treatment of GSH hardly afforded any yellow TPEF emission (Fig. 5d and e). These results accorded well with the ratiometric fluorescence behavior in Fig. 1 and DNEPI could be used as a novel ratiometric two-photon fluorescent sensing probe for selective bioimaging of mitochondrial Cys in living cells with negligible interference from other biological thiols.
It has been reported that mitochondria regulate the cellular redox state and the intracellular concentration of Cys is closely related to the level of oxidative stress.1,2 Thus, we evaluated the response ability of DNEPI to the change of the Cys concentration in SMMC7721 cells by altering the redox balance with H2O2. Compared with those without H2O2 pretreatment (Fig. 6a), H2O2 (200 μM) incubation of the DNEPI-loaded SMMC7721 cells displayed a distinct TPEF emission quenching in the yellow channel accompanied by a weak increase in the blue TPEF (Fig. 6b), implying that the decrease of the intracellular Cys concentration was induced by H2O2 oxidation of Cys. Thus, DNEPI could be used to monitor the level of intracellular Cys.
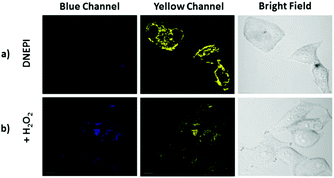 |
| Fig. 6 Two-photon confocal microscopic fluorescence images of Cys in SMMC7721 cells. (a) SMMC7721 cells stained with DNEPI (10 μM) for 30 min. (b) SMMC7721 cells pretreated with H2O2 (200 μM) for 30 min and then stained with incubated with DNEPI (10 μM) for 30 min. From left to right: Blue channel: Em = 400–500 nm; yellow channel: Em = 510–610 nm; bright field overlay. λex = 750 nm. Scale bar: 10 μm. | |
4. Conclusion
In summary, a novel ratiometric TP probe (DNEPI) was developed for two-photon excited cellular imaging of mitochondrial cysteine on the basis of the strong nucleophilic substitution of Cys promoting the cleavage of the sulfonic acid ester bond of DNEPI. The probe exhibited high selectivity toward Cys over other biothiols (including Hcy and GSH). More importantly, the probe could detect Cys with a fast response time (within 2 min) and the detection limit was quantitatively calculated as 0.29 μM. Furthermore, this probe also displayed low cytotoxicity and excellent biocompatibility, which were favorable for intracellular imaging. Subsequently, application of DNEPI to Cys imaging in live cells was successfully achieved by two-photon fluorescence microscopy, indicating that the probe had significant mitochondrial-targeting ability and could be used to monitor Cys concentration change in live cells. Therefore, this ratiometric two-photon fluorescent sensing probe proposed here has great potential for application to investigating and monitoring changes in intracellular Cys levels in living biological systems with negligible interference from other biological thiols.
Conflicts of interest
There are no conflicts to declare.
Acknowledgements
This work was supported by the National Natural Science Foundation of China (No. 21475080, 21575084 and 21874087), the Natural Sciences Foundation of Shanxi (No. 2016021036), and the Scientific and Technological Innovation Programs of Higher Education Institutions in Shanxi. We appreciate the Translational Medicine Research Center (Shanxi Medical University, Taiyuan, China) for kindly providing us with SMMC7721 cells and HeLa cells.
Notes and references
-
(a) H. M. McBride, M. Neuspiel and S. Wasiak, Curr. Biol., 2006, 16, R551 CrossRef CAS PubMed;
(b) T. Finkel, M. Serrano and M. A. Blasco, Nature, 2007, 448, 767 CrossRef CAS PubMed;
(c) B. Levine and G. Kroemer, Cell, 2008, 132, 27 CrossRef CAS PubMed.
-
(a) J. S. Armstrong, M. Whiteman, H. Yang, D. P. Jones and P. Sternberg, Invest. Ophthalmol. Visual Sci., 2004, 45, 4183 CrossRef PubMed;
(b) P. Nagy and M. T. Ashby, J. Am. Chem. Soc., 2007, 129, 14082 CrossRef CAS PubMed;
(c) M. Mancuso, D. Orsucci, A. Logerfo, A. Rocchi, L. Petrozzi, C. Nesti, F. Galetta, G. Santoro, L. Murri and G. Siciliano, J. Neurol., 2010, 257, 774 CrossRef CAS PubMed;
(d) S. R. Danielson, J. M. Held, M. Oo, R. Riley, B. W. Gibson and J. K. Andersen, J. Biol. Chem., 2011, 286, 7601 CrossRef CAS PubMed;
(e) C. A. Piantadosi, Biochim. Biophys. Acta, 2012, 1820, 712 CrossRef CAS PubMed;
(f) C. E. Paulsen and K. S. Carroll, Chem. Rev., 2013, 113, 4633 CrossRef CAS PubMed;
(g) R. J. Mailloux, S. L. McBride and M. E. Harper, Trends Biochem. Sci., 2013, 38, 592 CrossRef CAS PubMed;
(h) D. W. Bak and E. Weerapana, Mol. BioSyst., 2015, 11, 678 RSC.
-
(a) N. Mesecke, N. Terziyska, C. Kozany, F. Baumann, W. Neupert, K. Hell and J. M. Herrmann, Cell, 2005, 121, 1059 CrossRef CAS PubMed;
(b) C. P. Lee, M. Wirtz and R. Hell, Plant Cell Physiol., 2014, 55, 64 CrossRef CAS PubMed;
(c) P. Bragoszewski, M. Wasilewski, P. Sakowska, A. Gornicka, L. Bottinger, J. Qiu, N. Wiedemann and A. Chacinska, Proc. Natl. Acad. Sci. U. S. A., 2015, 112, 7713 CrossRef CAS PubMed.
-
(a) X. Chen, Y. Zhou, X. Peng and J. Yoon, Chem. Soc. Rev., 2010, 39, 2120 RSC;
(b) M. E. Jun, B. Roy and K. H. Ahn, Chem. Commun., 2011, 47, 7583 RSC;
(c) Y. Zhou and J. Yoon, Chem. Soc. Rev., 2012, 41, 52 RSC;
(d) D. Kim, H. G. Ryu and K. H. Ahn, Org. Biomol. Chem., 2014, 12, 4550 RSC;
(e) H. M. Kim and B. R. Cho, Chem. Rev., 2015, 115, 5014 CrossRef CAS PubMed;
(f) Z. Xu and L. Xu, Chem. Commun., 2016, 52, 1094 RSC.
-
(a) J. Guy, K. Caron, S. Dufresne, S. W. Michnick, W. G. Skene and J. W. Keillor, J. Am. Chem. Soc., 2007, 129, 11969 CrossRef CAS PubMed;
(b) L. Yi, H. Li, L. Sun, L. Liu, C. Zhang and Z. Xi, Angew. Chem., Int. Ed., 2009, 48, 4034 CrossRef CAS PubMed;
(c) H. S. Jung, J. H. Han, T. Pradhan, S. Kim, S. W. Lee, J. L. Sessler, T. W. Kim, C. Kang and J. S. Kim, Biomaterials, 2012, 33, 945 CrossRef CAS PubMed;
(d) H. S. Jung, T. Pradhan, J. H. Han, K. J. Heo, J. H. Lee, C. Kang and J. S. Kim, Biomaterials, 2012, 33, 8495 CrossRef CAS PubMed;
(e) X. Zhou, X. Jin, G. Sun, D. Li and X. Wu, Chem. Commun., 2012, 48, 8793 RSC;
(f) X. Zhou, X. Jin, G. Sun and X. Wu, Chem. – Eur. J., 2013, 19, 7817 CrossRef CAS PubMed;
(g) Q. Q. Wu, Z. F. Xiao, X. J. Du and Q. H. Song, Chem. – Asian J., 2013, 8, 2564 CrossRef CAS PubMed;
(h) Y. Yue, F. Huo, P. Ning, Y. Zhang, J. Chao, X. Meng and C. Yin, J. Am. Chem. Soc., 2017, 139, 3181 CrossRef CAS PubMed;
(i) P. S. Zhang, H. Wang, Y. X. Hong, M. L. Yu, R. J. Zeng, Y. F. Long and J. Chen, Biosens. Bioelectron., 2018, 99, 318 CrossRef CAS PubMed;
(j) H. Wang, P. S. Zhang, Y. Tian, Y. Zhang, H. P. Yang, S. Chen, R. J. Zeng, Y. F. Long and J. Chen, Anal. Bioanal. Chem., 2018, 410, 4379 CrossRef CAS PubMed.
-
(a) W. Jiang, Q. Fu, H. Fan, J. Ho and W. Wang, Angew. Chem., Int. Ed., 2007, 46, 8445 CrossRef CAS PubMed;
(b) B. Tang, Y. Xing, P. Li, N. Zhang, F. Yu and G. Yang, J. Am. Chem. Soc., 2007, 129, 11666 CrossRef CAS PubMed;
(c) J. Zhang, A. Shibata, M. Ito, S. Shuto, Y. Ito, B. Mannervik, H. Abe and R. Morgenstern, J. Am. Chem. Soc., 2011, 133, 14109 CrossRef CAS PubMed;
(d) J. Shao, H. Sun, H. Guo, S. Ji, J. Zhao, W. Wu, X. Yuan, C. Zhang and T. D. James, Chem. Sci., 2012, 3, 1049 RSC;
(e) R. Wang, L. Chen, P. Liu, Q. Zhang and Y. Wang, Chem. – Eur. J., 2012, 18, 11343 CrossRef CAS PubMed;
(f) M. H. Lee, J. H. Han, P. S. Kwon, S. Bhuniya, J. Y. Kim, J. L. Sessler, C. Kang and J. S. Kim, J. Am. Chem. Soc., 2012, 134, 1316 CrossRef CAS PubMed;
(g) Z. Guo, S. W. Nam, S. Park and J. Yoon, Chem. Sci., 2012, 3, 2760 RSC;
(h) L. Y. Niu, Y. S. Guan, Y. Z. Chen, L. Z. Wu, C. H. Tung and Q. Z. Yang, Chem. Commun., 2013, 49, 1294 RSC;
(i) S. Y. Lim, K. H. Hong, D. I. Kim, H. Kwon and H. J. Kim, J. Am. Chem. Soc., 2014, 136, 7018 CrossRef CAS PubMed;
(j) J. Liu, Y. Q. Sun, Y. Huo, H. Zhang, L. Wang, P. Zhang, D. Song, Y. Shi and W. Guo, J. Am. Chem. Soc., 2014, 136, 574 CrossRef CAS PubMed;
(k) Y. Liu, D. H. Yu, S. S. Ding, Q. Xiao, J. Guo and G. Q. Feng, ACS Appl. Mater. Interfaces, 2014, 6, 17543 CrossRef CAS PubMed;
(l) C. Han, H. Yang, M. Chen, Q. Q. Su, W. Feng and F. Y. Li, ACS Appl. Mater. Interfaces, 2015, 7, 27968 CrossRef CAS PubMed;
(m) X. He, X. Wu, W. Shi and H. Ma, Chem. Commun., 2016, 52, 9410 RSC;
(n) H. Zhang, R. Liu, J. Liu, L. Li, P. Wang, S. Q. Yao, Z. Xu and H. Sun, Chem. Sci., 2016, 7, 256 RSC;
(o) C. Y. Kim, H. J. Kang, S. J. Chung, H. K. Kim, S. Y. Na and H. J. Kim, Anal. Chem., 2016, 88, 7178 CrossRef CAS PubMed;
(p) K. Umezawa, M. Yoshida, M. Kamiya, T. Yamasoba and Y. Urano, Nat. Chem., 2017, 9, 279 CrossRef CAS PubMed;
(q) Z. H. Fu, X. Han, Y. L. Shao, J. G. Fang, Z. H. Zhang, Y. W. Wang and Y. Peng, Anal. Chem., 2017, 89, 1937 CrossRef CAS PubMed;
(r) Y. K. Yue, F. J. Huo, X. Q. Li, Y. Wen, T. Yi, J. Salamanca, J. O. Escobedo, R. M. Strongin and C. X. Yin, Org. Lett., 2017, 19, 82 CrossRef CAS PubMed;
(s) L. W. He, X. L. Yang, K. X. Xu, X. Q. Kong and W. Y. Lin, Chem. Sci., 2017, 8, 6257 RSC;
(t) L. W. He, X. L. Yang, K. X. Xu and W. Y. Lin, Anal. Chem., 2017, 89, 9567 CrossRef CAS PubMed.
-
(a) K. S. Lee, T. K. Kim, J. H. Lee, H. J. Kim and J. I. Hong, Chem. Commun., 2008, 46, 6173 RSC;
(b) H. L. Li, J. L. Fan, J. Y. Wang, M. Z. Tian, J. J. Du, S. G. Sun, P. P. Sun and X. J. Peng, Chem. Commun., 2009, 39, 5904 RSC;
(c) M. M. Hu, J. L. Fan, H. L. Li, K. D. Song, S. Wang, G. H. Cheng and X. J. Peng, Org. Biomol. Chem., 2011, 9, 980 RSC;
(d) X. Yang, Y. Guo and R. Strongin, Angew. Chem., Int. Ed., 2011, 50, 10690 CrossRef CAS PubMed;
(e) P. Wang, J. Liu, X. Lv, Y. L. Liu, Y. Zhao and W. Guo, Org. Lett., 2012, 14, 520 CrossRef CAS PubMed;
(f) D. Gong, Y. Tian, C. Yang, A. Iqbal, Z. Wang, W. Liu, W. Qin, X. Zhu and H. Guo, Biosens. Bioelectron., 2016, 85, 178 CrossRef CAS PubMed.
-
(a) H. Maeda, H. Matsuno, M. Ushida, K. Katayama, K. Saeki and N. Itoh, Angew. Chem., Int. Ed., 2005, 117, 2982 CrossRef;
(b) J. Y. Shao, H. M. Guo, S. M. Ji and J. Z. Zhao, Biosens. Bioelectron., 2011, 26, 3012 CrossRef CAS PubMed;
(c) L. Yuan, W. Lin, S. Zhao, W. S. Gao, B. Chen, L. W. He and S. S. Zhu, J. Am. Chem. Soc., 2012, 134, 13510 CrossRef CAS PubMed;
(d) J. Shao, H. Sun, H. Guo, S. Ji, J. Zhao, W. Wu, X. Yuan, C. Zhang and T. D. James, Chem. Sci., 2012, 3, 1049 RSC;
(e) J. Lu, Y. Song, W. Shi, X. Li and H. Ma, Sens. Actuators, B, 2012, 161, 615 CrossRef CAS;
(f) X. D. Liu, R. Sun, J. F. Ge, Y. J. Xu, Y. Xu and J. M. Lu, Org. Biomol. Chem., 2013, 11, 4258 RSC;
(g) M. Wei, P. Yin, Y. Shen, L. Zhang, J. Deng, S. Xue, H. Li, B. Guo, Y. Zhang and S. Yao, Chem. Commun., 2013, 49, 4640 RSC;
(h) D. Su, C. L. Teoh, S. Sahu, R. K. Das and Y. T. Chang, Biomaterials, 2014, 35, 6078 CrossRef CAS PubMed;
(i) J. Yin, Y. Kwon, D. Kim, D. Lee, G. Kim, Y. Hu and J. Yoon, J. Am. Chem. Soc., 2014, 136, 5351 CrossRef CAS PubMed;
(j) H. Chen, Y. H. Tang, M. G. Ren and W. Y. Lin, Chem. Sci., 2016, 7, 1896 RSC;
(k) X. Zhu, Y. Li, W. Zan, J. Zhang, Z. Chen, X. Liu, F. Qi, X. Yao, X. Zhang and H. Zhang, Photochem. Photobiol. Sci., 2016, 15, 412 RSC;
(l) X. Dai, T. Zhang, J. Y. Miao and B. X. Zhao, Sens. Actuators, B, 2016, 223, 274 CrossRef CAS;
(m) Q. K. Gao, W. Z. Zhang, B. Song, R. Zhang, W. H. Guo and J. L. Yuan, Anal. Chem., 2017, 89, 4517 CrossRef CAS PubMed;
(n) X. L. Liu, B. Song, H. Ma, Z. X. Tang and J. L. Yuan, J. Mater. Chem. B, 2018, 6, 1844 RSC.
-
(a) H. S. Jung, J. H. Han, Y. Habata, C. Kang and J. S. Kim, Chem. Commun., 2011, 47, 5142 RSC;
(b) W. Hao, A. McBride, S. McBride, J. P. Gao and Z. Y. Wang, J. Mater. Chem., 2011, 21, 1040 RSC;
(c) Y. Hu, C. H. Heo, G. Kim, E. J. Jun, J. Yin, H. M. Kim and J. Yoon, Anal. Chem., 2015, 87, 3308 CrossRef CAS PubMed.
-
(a) W. R. Zipfel, R. M. Williams, R. Christie, A. Y. Nikitin, B. T. Hyman and W. W. Webb, Proc. Natl. Acad. Sci. U. S. A., 2003, 100, 7075 CrossRef CAS PubMed;
(b) Y. Y. Ma, Y. P. Zhao, R. Guo, L. L. Zhu and W. Y. Lin, J. Mater. Chem. B, 2018, 6, 6212 RSC;
(c) R. Guo, J. L. Yin, Y. Y. Ma, Q. A. Wang and W. Y. Lin, J. Mater. Chem. B, 2018, 6, 2894 RSC;
(d) M. G. Ren, K. Zhou, L. W. He and W. Y. Lin, J. Mater. Chem. B, 2018, 6, 1716 RSC.
-
(a) M. D. Cahalan, I. Parker, S. H. Wei and M. J. Miller, Nat. Rev. Immunol., 2002, 2, 872 CrossRef CAS PubMed;
(b) W. R. Zipfel, R. M. Williams and W. W. Webb, Nat. Biotechnol., 2003, 21, 1369 CrossRef CAS PubMed;
(c) F. Helmchen and W. Denk, Nat. Methods, 2005, 2, 932 CrossRef CAS PubMed;
(d) S. Kim, Q. D. Zheng, G. S. He, D. J. Bharali, H. E. Pudavar, A. Baev and P. N. Prasad, Adv. Funct. Mater., 2006, 16, 2317 CrossRef CAS;
(e) T. Terai and T. Nagano, Curr. Opin. Chem. Biol., 2008, 12, 515 CrossRef CAS PubMed;
(f) M. Pawlicki, H. A. Collins, R. G. Denning and H. L. Anderson, Angew. Chem., Int. Ed., 2009, 48, 3244 CrossRef CAS PubMed;
(g) K. Y. Liu, X. Q. Kong, Y. Y. Ma and W. y. Lin, Nat. Protoc., 2018, 13, 1020 CrossRef CAS PubMed;
(h) Y. j. Zuo, T. X. Yang, Y. Zhang, Z. M. Gou, M. G. Tian, X. Q. Kong and W. Y. Lin, Chem. Sci., 2018, 9, 2774 RSC;
(i) K. Y. Liu, X. Q. Kong, Y. Y. Ma and W. Y. Lin, Angew. Chem., Int. Ed., 2017, 56, 13489 CrossRef CAS PubMed; P. S. Zhang, H. Wang, D. Zhang, X. Y. Zeng, R. J. Zeng, L. H. Xiao, H. W. Tao, Y. F. Long, P. G. Yi and J. Chen, Sens. Actuators, B, 2018, 255, 2223 Search PubMed.
-
(a) M. Zhang, M. Yu, F. Li, M. Zhu, M. Li, Y. Gao, L. Li, Z. Liu, J. Zhang, D. Zhang, T. Yi and C. Huang, J. Am. Chem. Soc., 2007, 129, 10322 CrossRef CAS PubMed;
(b) X. Zhang, X. Ren, Q. Xu, K. P. Loh and Z. Chen, Org. Lett., 2009, 11, 1257 CrossRef CAS PubMed;
(c) J. H. Lee, C. S. Lim, Y. S. Tian, J. H. Han and B. R. Cho, J. Am. Chem. Soc., 2010, 132, 1216 CrossRef CAS PubMed;
(d) C. S. Lim, G. Masanta, H. J. Kim, J. H. Han, H. M. Kim and B. R. Cho, J. Am. Chem. Soc., 2011, 133, 11132 CrossRef CAS PubMed;
(e) Z. Yang, N. Zhao, Y. Sun, F. Miao, Y. Liu, X. Liu, Y. Zhang, W. Ai, G. Song, X. Shen, X. Yu, J. Sun and W. Wong, Chem. Commun., 2012, 48, 3442 RSC;
(f) L. Yuan, W. Lin, H. Chen, S. Zhu and L. He, Angew. Chem., Int. Ed., 2013, 52, 10018 CrossRef CAS PubMed;
(g) Y. Hu, C. H. Heo, G. Kim, E. J. Jun, J. Yin, H. M. Kim and J. Yoon, Anal. Chem., 2015, 87, 3308 CrossRef CAS PubMed;
(h) X. D. Guo, X. Zhang, S. Q. Wang, S. Y. Li, R. Hua, Y. Li and G. Q. Yang, Anal. Chim. Acta, 2015, 81, 869 Search PubMed;
(i) W. F. Niu, L. Guo, Y. H. Li, S. M. Shuang, C. Dong and M. S. Wong, Anal. Chem., 2016, 88, 1908 CrossRef CAS PubMed; P. Yue, X. L. Yang, P. Ning, X. G. Xi, H. Z. Yu, Y. Feng, R. Shao and X. G. Meng, Talanta, 2018, 178, 24 Search PubMed;
(j) J. Y. Wang, Z. R. Liu, M. G. Ren, X. Q. Kong and W. Y. Lin, J. Mater. Chem. B, 2017, 5, 134 RSC.
-
(a) A. S. Brown, L. M. Bernal, T. L. Micotto, E. L. Smith and J. N. Wilson, Org. Biomol. Chem., 2011, 9, 2142 RSC;
(b) E. A. Mensah, W. G. Gonzalez, J. Miksovska and J. N. Wilson, J. Phys. Chem. A, 2012, 116, 12470 CrossRef PubMed.
- T. Mosmann and J. Immunol, Methods, 1983, 65, 55 CAS.
-
(a) L. Deng, W. Wu, H. Guo, J. Zhao, S. Ji, X. Zhang, X. Yuan and C. Zhang, J. Org. Chem., 2011, 76, 9294 CrossRef CAS PubMed;
(b) Q. X. Han, Z. H. Shi, X. L. Tang, L. Z. Yang, Z. L. Mou, J. Li, J. M. Shi, C. Y. Chen, W. Liu, H. Yang and W. S. Liu, Org. Biomol. Chem., 2014, 12, 5023 RSC.
- L. F. Yousif, K. M. Stewart and S. O. Kelley, ChemBioChem, 2009, 10, 1939 CrossRef CAS PubMed.
- C. R. Yellaturu, M. Bhanoori, I. Neeli and G. N. Rao, J. Biol. Chem., 2002, 277, 40148 CrossRef CAS PubMed.
Footnotes |
† Electronic supplementary information (ESI) available. See DOI: 10.1039/c8an01908h |
‡ These authors contributed equally to this work. |
|
This journal is © The Royal Society of Chemistry 2019 |
Click here to see how this site uses Cookies. View our privacy policy here.