DOI:
10.1039/D1QI00857A
(Research Article)
Inorg. Chem. Front., 2022,
9, 179-185
Self-reconstruction of cationic activated Ni-MOFs enhanced the intrinsic activity of electrocatalytic water oxidation†
Received
12th July 2021
, Accepted 9th November 2021
First published on 16th November 2021
Abstract
Metal–organic frameworks (MOFs) have emerged as alternative OER catalysts due to their alkaline hydrolysis from high “molecular-scale pores” to high “nano-scale porosity” in alkaline solution, and then in situ self-reconstruction from MOFs to metal hydroxides. Herein, quantitative iron ions are spontaneously adsorbed on the surface of Ni-MOFs with negative charge effect and are directly used as OER catalysts. Based on the cation regulation and the in situ alkaline hydrolysis of MOFs in 1.0 M KOH solution, the multilayer bimetallic nickel irons hydroxide with enriched active sites were intended to be the real active phase. At the same time, the introduction of iron cation will also produce a bimetallic synergy, thus achieving superior OER catalytic activity (η50 = 280 mV). This novel strategy provides an avenue for the exploration of catalytic mechanisms and directional design of MOF-based catalysts.
Introduction
Oxygen evolution reaction (OER) is an immensely significant electrochemical half-reaction due to the irreplaceable role in efficient energy conversion and storage technologies, such as water electrolysis and rechargeable metal–air batteries.1–3 Nevertheless, the sluggish dynamics is still a bottleneck problem to be tackled, which makes it one of the most challenging puzzles.4–6 Comparatively, the traditional Ru-based and Ir-based catalysts show satisfactory catalytic activity, although the scarcity and high cost of these metals still restrict their industrialization.7,8 Therefore, the design and development of a new, high-efficient, and robust transition-metal OER electrocatalyst is an intelligent strategy to reduce the cost of catalysis at the industrial level.9,10
Controlling the electronic structure of the active center and its adsorption energy with oxygen-containing intermediates is an effective strategy to achieve excellent water oxidation performance, as OER is a typical surface reaction, which is composed of adsorbed reactants.11–13 Doping, etching, and ion substitution are common methods to adjust the electronic structure of catalysts. Due to the synergistic effect between two different metal atoms in regulating the electronic performance of a catalyst, the preparation of bimetallic electrocatalysts by the cation doping technology has attracted extensive attention.14–17
Metal–organic frameworks (MOFs) have gained considerable attention in the OER field for their periodic arrangement, unsaturated coordination active centers, and the ultrahigh surface area.18–20 In recent years, MOFs usually provide a sterling platform for preparing numerous transition metal-based oxy-hydroxides (MOOH/M(OH)x, M = Fe, Co, Ni, V, etc.) owing to their alkaline hydrolysis in lye. These MOOH/M(OH)x have been considered as the most effective electrocatalysts due to their inherent layered structure and the ability to offer more active sites than intrinsic hydroxides.14,21–24 For instance, Zhang et al. reported an excellent NiFe Prussian blue analogue, and proved that the in situ formed NiOOH2−x containing Ni4+ is the real active center by Operando X-ray spectroscopy.23 Bu et al. reported the ligand exchange phenomenon of MOFs in the KOH electrolytes owing to its poor stability, and the obtained bimetallic hydroxide from mixed two MOFs shows excellent OER activity.25 Unfortunately, the construction of bimetallic MOFs often leads to the difference of physical properties such as crystallinity and morphology, resulting in more variables affecting the catalytic activity.
With the above results in mind, NF-n (n = 5, 10, 20, 50) electrocatalysts were synthesized through electrostatic interaction between Fe ions and Ni-MOFs during preparing the working electrode and were directly employed in electrochemical OER. The optimized catalyst (NF-10) produces the best water oxidation activity (η50 = 280 mV) and satisfactory electrochemical stability, superior to other samples with different Fe-doping concentrations. A series of characterization displays that the nickel iron hydroxide (NF-LDH) formed by the ion exchange of Ni-MOFs-Fe in an alkaline solution was the real active species. To the best of our current knowledge, the cation held Ni-MOF strategy in our study is the most convenient and fascinating way to obtain NF-LDH. Additionally, cationic Fe can be directly introduced in the preparation of an MOF anode without additional operation. The excellent performance benefits from the multi-layered structure enrich active sites, and bimetallic synergistic effect. This straightforward method of obtaining a bimetallic LDH catalyst opens up a new perspective for the direction of electrochemistry.
Results and discussion
Structural characterization
The synthetic routes of the NF-10 are shown in Scheme 1. First, lamellated Ni-MOFs were prepared by the hydrothermal method reported in the literature.25 The characteristic peaks in X-ray diffraction (XRD) pattern are consistent with the previously reported single-crystal data (CCDC: 638866), indicating the successful synthesis of Ni-MOFs (Fig. 1a) and the layered morphology of as-synthesized Ni-MOFs can be discerned commendably by scanning electron microscopy (SEM) (Fig. 1c). In addition, zeta potential data of Ni-MOFs shows a negative effect of −14.67 mV. As expected, Ni-MOFs-Fe was obtained skillfully by mixing 10 mM Fe3+ with Ni-MOFs under ultrasonic irradiation. The negative charge of Ni-MOFs-Fe (−5.78 mV) is smaller than that of Ni MOFs, which indicates that Fe3+ can be uniformly adsorbed on Ni-MOF nanosheets through electrostatic interaction after a simple ultrasonic treatment. Significantly, the XRD pattern of Ni-MOFs-Fe shows that the intervention of Fe3+ has an unobservable change of the structure of Ni-MOFs (Fig. 1a). SEM and transmission electron microscopy (TEM) images of Ni-MOFs-Fe unveils the original laminated morphology with minor changes except for the edges of the nonrigid nanosheets crooked spontaneously (Fig. 1d and 2c). Subsequently, the SEM element mapping confirmed the uniform distribution of F, O, Ni and Fe on the entire Ni-MOFs-Fe surface in which the F element comes from binder Nafion (Fig. 1e).
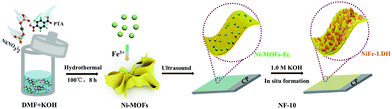 |
| Scheme 1 Synthesis procedure of NF-10 electrocatalysts. | |
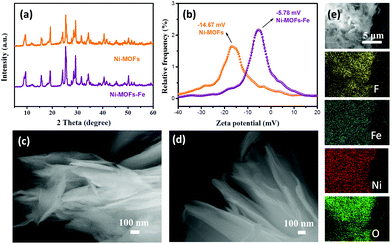 |
| Fig. 1 (a) XRD patterns and (b) zeta potential of Ni-MOFs and Ni-MOFs-Fe. SEM images of (c) Ni-MOFs and (d) Ni-MOFs-Fe. (e) SEM images (5 μm scale) and the corresponding mapping of Ni-MOFs-Fe. | |
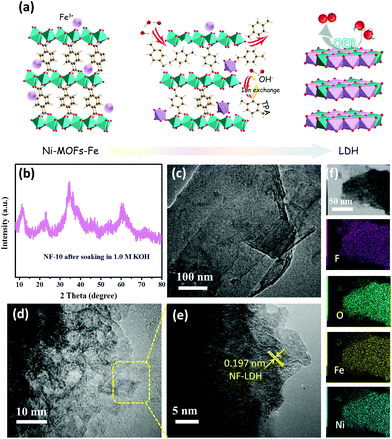 |
| Fig. 2 (a) Sketch of the evolution of the ion exchange mechanism. (b) XRD patterns of Ni-MOFs-Fe after soaked in 1.0 M KOH. (c) TEM image of Ni-MOFs-Fe. (d and e) HR-TEM images with different magnification and (f) its mapping of Ni-MOFs-Fe after soaked in 1.0 M KOH. | |
In order to further verify the self-reconstruction of the optimized catalyst in the test environment, a series of ex situ characterizations were carried out. The XRD characteristic peaks confirm the formation of NF-LDH (Fig. 2b) once soaking Ni-MOFs-Fe in the alkaline electrolyte solution,26 suggesting the occurrence of an alkaline hydrolysis of the target material. The specific alkaline hydrolysis mechanism is shown in Fig. 2a, while the hydroxyl ions in the electrolyte replaces the terephthalic acid ligands (TPA) in Ni-MOFs, and then react Ni2+ of Ni-MOFs and Fe3+ to form NF-LDH in situ. As a real active species, NF-LDH will participate in the formation of active intermediates in the OER reaction, thus accelerating the whole reaction process, which corroborates with the extant literature.25,27 As illustrated in Fig. 2d and S1,† the details morphology of Ni-MOFs-Fe after soaked in 1.0 M KOH were characterized by TEM and the flocculent nanosheet structure was determined, which is more conducive to expose the active sites and accelerate the OER process. Further high-resolution TEM image (Fig. 2e) shows the uniform lattice spacing is 0.197 nm, which matches the (018) surface of NF-LDH well. The TEM and corresponding mapping images of NF-LDH show that all Ni, Fe, and O elements are evenly distributed in the catalyst, and likewise, element F comes from perfluorosulfonic acid in Nafion (Fig. 2f). Therefore, in fact, Ni-MOFs-Fe is only an intermediate state of working electrode preparation engineering. In order to track the catalyst characteristics under different test environments, the working electrode loaded with Ni-MOFs-Fe (10 mM) or soaked in 1.0 M KOH are all defined as NF-10 (Scheme 1). For comparison purposes, a mixture solution containing 10 mM Fe3+ and Ni-MOFs was directly dispersed in 1.0 KOH, respectively. Then, the Ni(OH)2 (named as N-1) (PDF # 73-1520)28 and the FeOOH (labled as F-1) (PDF # 29-0713)29 are obtained (Fig. S2 and 3†), which further confirmed the alkali hydrolysis effect.
Electrochemical measurements
In 1.0 M KOH electrolyte, the electrode potentials (E° vs. RHE) of oxygen evolution (+0.40 V) and hydrogen evolution (−0.83 V) are found in the following eqn (1)–(3):30 | 4OH− → O2 + 2H2O + 4e− E° = +0.40 V | (1) |
| 4H2O + 4e → 2H2 + 4OH− E° = −0.83 V | (2) |
| 2H2O → O2 + 2H2 E° = +1.23 V | (3) |
Firstly, the water oxidation performance of NF-n was evaluated by linear sweep voltammetry (LSV) (Fig. S4a†), and NF-10 displays the minimum overpotential. In contrast, N-1, F-1, RuO2, and CP (carbon paper) were also carried out with LSV (Fig. 3a). The bulge of the oxidation peak observed at about 1.38 V/1.45 V in Ni-containing samples is considered to be of Ni2+ → Ni3+via the deprotonating of lattice hydroxide (–OH) to oxidizing group (–O) according to eqn (4).23,31
| Ni(OH)2 + OH− ⇄ NiOOH + e− + H2O | (4) |
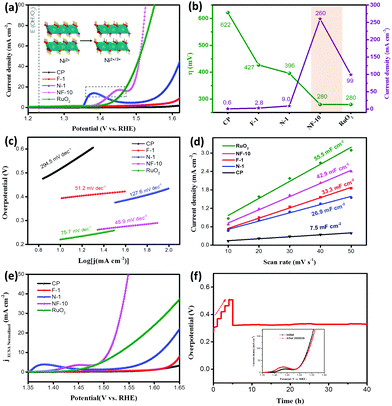 |
| Fig. 3 Electrochemical characterization of N-1, F-1, NF-10, RuO2 and CP. (a) LSV polarization curves. (b) Comparison of overpotential (at 50 mA cm−2) and current density of different catalysts at 1.55 V (vs. RHE). (c) Tafel plots. (d) Double-layer capacitance determined by the CV curves. (e) LSV curves normalized by ECSA. (f) V–T curve of FN-10 and LSVs before and after 20 000th CV cycles (inset). | |
The optimized NF-10 has the minimum value of η50 (the current density is 50 mA cm−2) of 280 mV, which is smaller than the contrast sample N-1 (396 mV), F-1 (427 mV), CP (622 mV), and other NF-n samples, respectively (Fig. 3a, b and Fig. S4a†), and shows similar electrochemical performance as precious metal RuO2 (280 mV). As expected, NF-10 has the minimum overpotential accompanied by the fastest current rise, that is, it has the fastest dynamic characteristics. Specifically, NF-10 has the minimum value of the Tafel slope (48.9 mV dec−1), which are 2.3, 26.8, 78.7 and 245.6 mV dec−1 lower than F-1 (51.2 mV dec−1), RuO2 (75.7 mV dec−1), N-1 (127.6 mV dec−1), CP (294.5 mV dec−1), and other NF-n samples, respectively (Fig. 3c and Fig. S4b†). At the fixed voltage (1.55 V, vs. RHE), NF-10 also displays the highest current density (260 mA cm−2), which are 2.6, 28.9, 92.8 and 433.3 times that of RuO2 (99.1 mA cm−2), N-1 (9.0 mA cm−2), F-1 (2.8 mA cm−2), and CP (0.6 mA cm−2), respectively (Fig. 3a and b). Moreover, electrochemically active surface area (ECSA) is used to characterize the number of electrochemically active sites and is proportional to the capacitance of the electric double layer (Cdl).32–34Cdl curves are calculated from the cyclic voltammetry (CV) curves at different scanning speeds in the non-Faradaic range (Fig. S5a–h†). The value of NF-10 is better than that of N-1, F-1 and CP (Fig. 3d), indicating that there are more activation sites in the optimized catalyst (Fig. 3d and Fig. S5i†). The introduction of Fe ions increases the ECSA and the bimetallic synergistic effect between Fe and Ni, forming higher valence Fe4+/Ni4+, which effectively improves the catalytic activity.35,36 Notably, when the iron content is in a certain range (n from 0 to 10), the performance of the catalyst shows an increasing trend. When the iron content continues to increase (n from 10 to 50), the catalytic activity decreases to a certain extent, which may be mainly due to the production of FeOOH with less contribution to the activity during the formation of NF-LDH due to sufficient Fe3+.37 As reported in the literature, not all active sites are really catalytic sites for the OER reaction.25,38 To reveal the intrinsic catalytic performance of NF-10 in-depth, the LSV curves were normalized by ECSA (Fig. 3e). Not surprisingly, NF-10 showed the minimum overpotential as usual, which was superior to other catalysts and commercial RuO2, illustrating that the NF-10 catalyst has excellent electrocatalytic intrinsic activity. Since durability is also a key parameter to evaluate the OER activity of the catalyst,39 electrochemical stability test was also explored as displayed in Fig. 3f. Multistep curve indicated that a negligible change in voltage was shown after the 40 h OER test (Fig. 3f). The current density remained almost unchanged after the electrode was cycled 20
000 times at 1.50–1.55 V (vs. RHE) (inset), indicating its good recoverability benefitted from its novel structural characteristics, such as pliability and toughness of two-dimensional nanosheets.7 In addition, after the OER test, the well-distributed elements and multi-level lamellar structure of NF-10 is completely maintained substantiating the satisfactory stability of the catalyst (Fig. 4c–e). All the above electrochemical data analysis results show that the cation regulation strategy has been proven to enhance OER performance.
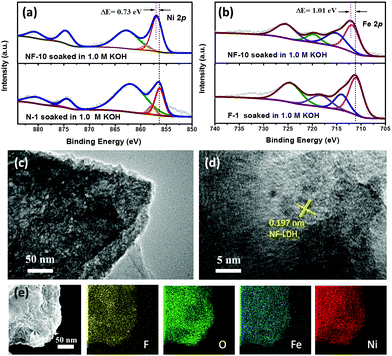 |
| Fig. 4 (a) Ni 2p and (b) Fe 2p XPS spectra of NF-10, N-1 and F-1 after soaked in 1.0 M KOH. (c) TEM, (d) HR-TEM images, and TEM mapping (e) of NF-10 after OER test. | |
Chemical valence research
To further elucidate the influences of Fe-doping and its doping effect on the surface chemical properties and the chemical state of NF-10, X-ray photoelectron spectroscopy (XPS) was carried out (Fig. 4a, b and Fig. S6†). The XPS survey spectra (Fig. S6c†) demonstrate the presence of Fe, Ni and O in NF-10, which align with the SEM results (Fig. 1e). Ni 2p and Fe 2p core-level spectra were recorded to discuss the effect of atom doping on another metal's electronic structures. As displayed in Fig. 4a, two distinct characteristic peaks at positions 856.9 and 874.8 eV belong to Ni 2p3/2 and Ni 2p1/2, respectively,40,41 and are accompanied by their respective satellite peaks at positions 862.8 and 880.8 eV, respectively. In particular, the peak at 858.9 eV indicates the appearance of the Ni3+ oxidation state, which was attributed to the crystal and amorphous interface formed by the interaction between Ni atom and OH−.42,43 Compared with pure N-1, the binding energy position shifts to the positive direction (0.73 eV), indicating oxidation state is increased by the loss of more electrons under the regulation of cation.44 In the Fe 2p spectra (Fig. 4b), the peaks at above 712.1 and 725.5 eV are attributed to Fe 2p3/2 and Fe 2p1/2, respectively. The Fe3+ oxidation state appears at 719.9 eV and is accompanied by more than one valence state of Fe3+ (715.5 eV). The Fe3+ peak of NF-10 is shifted by 1.01 eV compared to F-1, demonstrating strong electronic interaction between Fe and Ni metals.25 Therefore, the electronic environment modulated by the introduction of cations into N-1 favors the formation of metal high valence active species in NF-LDH-based electrocatalysts. These active species can accelerate the nucleophile addition of OH− and H2O on the electrocatalyst surface, enhance the absorption of reaction intermediates (*OH, *OOH and *O), and further enhance its electrocatalytic OER performance.45 Furthermore, the Ni 2p spectrum after OER testing observed a significant increase in the number of Ni3+ oxides, which is usually considered to be the active center of OER (Fig. S6a†).46,47 Moreover, as the OER proceeds, the Fe 2p binding energy shifts significantly negatively, while the Ni 2p binding energy of Ni2+ remains almost unchanged (Fig. S6b†). Thus, the iron position is most likely to be the hydroxyl receptor, which accelerates the OER by discharge and desorption, consistent with the catalyst reported earlier.35,48
Conclusions
In summary, NF-n (n = 5, 10, 20, 50) was creatively constructed during the preparation of an anode electrode using the cation-regulated Ni-MOFs, and their reactivity as electrochemistry OER catalysts was further investigated. In particular, due to the alkaline hydrolysis reaction of low stability Ni-MOFs modified by Fe ions, the as-prepared catalyst materials undergo self-reconstruction under the test environment, and the in situ formation of NF-LDH is supposed as the actual catalytic active phase to drive the OER process. The optimized NF-10 electrocatalyst shows superb electrocatalytic performances towards OER (η50 = 280 mV) and the current density at 1.55 V (vs. RHE) achieves about 26 times and about 78 times increases compared to those of N-1 and F-1, respectively. In addition, NF-10 exhibits a tiny Tafel slope of 48.9 mV dec−1 and shows excellent electrochemical stability over 40 h with negligible voltage decay. This brand-new proof-of-concept methodology cation regulation strategy is an effective strategy to construct excellent OER electrocatalysts. It provides a new perspective for further understanding of electrocatalysis from the electronic and atomic levels.
Experimental
Synthesis of Ni-MOFs
Ni-MOFs was synthesized according to the previous literature with a little modification:25,49 1.0 mmol 1,4-dicarboxybenzene (C8H6O4, 166 mg) and 0.325 mmol Ni(NO3)2·6H2O (95 mg) were successively dissolved in 20 mL N,N-dimethylformamide and was continuously stirred at 25 °C. Then, 2 mL of 0.2 M potassium hydroxide (KOH) was added dropwise to the above-mixed solution and then continuously stirred for 30 min. Finally, the solution was heated at 100 °C for 8 h in a covered polytetrafluoroethylene reactor (Anhui Kemi Machinery Technology Co., Ltd). The centrifuged product were collected were washed twice with DMF and ethanol, respectively, and then vacuum dried at 60 °C for 12 h.
Synthesis of NF-n (n = 5, 10, 20, 50), N-1
NF-10 was obtained during the preparation of an anode electrode without introducing any additional operation. 3 mg Ni-MOFs and 20 μL Nafion (5.0 wt%) were dispersed in the mixture solution (Vdistilled water
:
Vethanol = 2
:
3) containing 10 mM FeCl3·6H2O, and then ultrasonically treated for 10 min to form a light green slurry. The catalyst slurry is dripped onto the carbon paper (CP, 1 cm × 1 cm, loading 1 mg cm−2) to form a working electrode (Ni-MOF-Fe, NF-10). Other contrast slurry with different ion concentrations of Fe (0, 5, 20, and 50 mM) is named N-1, NF-5, NF-20, and NF-50, respectively.
Synthesis of NF-LDH and F-1
The as-prepared working electrode NF-10 was directly used as an OER catalyst and immersed in 1.0 KOH for 10 min entitled as NF-LDH. To obtain F-1, a mixture solution (Vdistilled water
:
Vethanol = 2
:
3) containing 10 mM FeCl3·6H2O was directly immersed in 1.0 KOH for 10 min, and the centrifuged product was collected and washed twice with H2O and ethanol, and vacuum dried at 50 °C for 4 h. Then, 3 mg powder and 20 μl Nafion were dispersed in a mixture solution (Vdistilled water
:
Vethanol = 2
:
3), and ultrasonically treated for 10 min to form a light yellow slurry. The catalyst slurry is dripped onto the CP to form a working electrode (F-1).
Electrochemical measurements
Three electrodes were used to test the electrochemical performance at 25 °C. Among them, Ag/AgCl (1.0 M potassium chloride solution) was used as a reference electrode, 1 × 1 cm2 platinum mesh electrode was used as counter electrode, and the chemical workstation model was Chenhua 760E. All the data of electrochemical polarization curves have been corrected (iR = 95%) and converted to the potential relative to the hydrogen standard electrode: | ERHE = EAg/AgCl + 0.059 × pH + 0.2223 V | (1) |
ECSA and normalized current density were calculated according to following equation:
| 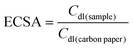 | (2) |
| 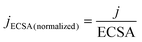 | (3) |
C
dl is the double-layer capacitance, calculated from CV tests at different scan rates, and j is the current density.
Conflicts of interest
There are no conflicts to declare.
Acknowledgements
Our project was financially supported by Tianjin Undergraduate Innovative Research program (202010055420), the MOE (IRT13R30) and the NCC fund (grant NCC2020PY02).
Notes and references
- X. Liu, L. Wang, P. Yu, C. Tian, F. Sun, J. Ma, W. Li and H. Fu, A stable bifunctional catalyst for rechargeable zinc-air batteries: iron-cobalt nanoparticles embedded in a nitrogen-doped 3D carbon matrix, Angew. Chem., Int. Ed., 2018, 57, 16166–16170 CrossRef CAS.
- S. Dou, L. Tao, J. Huo, S. Wang and L. Dai, Etched and doped Co9S8/graphene hybrid for oxygen electrocatalysis, Energy Environ. Sci., 2016, 9, 1320–1326 RSC.
- D. A. Kuznetsov, M. A. Naeem, P. V. Kumar, P. M. Abdala, A. Fedorov and C. R. Muller, Tailoring lattice oxygen binding in ruthenium pyrochlores to enhance oxygen evolution activity, J. Am. Chem. Soc., 2020, 142, 7883–7888 CrossRef CAS PubMed.
- H. Zhang, X. Wang, Z. Yang, S. Yan, C. Zhang and S. Liu, Space-confined synthesis of lasagna-like N-doped graphene-wrapped copper-cobalt sulfides as efficient and durable electrocatalysts for oxygen reduction and oxygen evolution reactions, ACS Sustainable Chem. Eng., 2019, 8, 1004–1014 CrossRef.
- H. Yan, Y. Xie, A. Wu, Z. Cai, L. Wang, C. Tian, X. Zhang and H. Fu, Anion–modulated HER and OER activities of 3D Ni-V–based interstitial compound heterojunctions for high–efficiency and stable overall water splitting, Adv. Mater., 2019, 31, 1901174 CrossRef.
- D. Friebel, M. W. Louie, M. Bajdich, K. E. Sanwald, Y. Cai, A. M. Wise, M. J. Cheng, D. Sokaras, T. C. Weng, R. Alonso-Mori, R. C. Davis, J. R. Bargar, J. K. Norskov, A. Nilsson and A. T. Bell, Identification of highly active Fe sites in (Ni,Fe)OOH for electrocatalytic water splitting, J. Am. Chem. Soc., 2015, 137, 1305–1313 CrossRef CAS PubMed.
- X. Wang, H. Zhang, Z. Yang, C. Zhang and S. Liu, Ultrasound-treated metal-organic framework with efficient electrocatalytic oxygen evolution activity, Ultrason. Sonochem., 2019, 59, 104714 CrossRef CAS PubMed.
- Z. Xue, K. Liu, Q. Liu, Y. Li, M. Li, C. Y. Su, N. Ogiwara, H. Kobayashi, H. Kitagawa, M. Liu and G. Li, Missing-linker metal-organic frameworks for oxygen evolution reaction, Nat. Commun., 2019, 10, 5048 CrossRef PubMed.
- K. He, T. Tadesse Tsega, X. Liu, J. Zai, X. H. Li, X. Liu, W. Li, N. Ali and X. Qian, Utilizing the space–charge region of the FeNi–LDH/CoP p–n junction to promote performance in oxygen evolution electrocatalysis, Angew. Chem., Int. Ed., 2019, 58, 11903–11909 CrossRef CAS PubMed.
- M. Ahmad, B. Xi, Y. Gu and S. Xiong, N-Doped carbon coated NiCo2O4 nanorods for efficient electrocatalytic oxygen evolution, Inorg. Chem. Front., 2021, 8, 3740–3747 RSC.
- Z. Qiu, C. W. Tai, G. A. Niklasson and T. Edvinsson, Direct observation of active catalyst surface phases and the effect of dynamic self-optimization in NiFe-layered double hydroxides for alkaline water splitting, Energy Environ. Sci., 2019, 12, 572–581 RSC.
- M. Cabán-Acevedo, M. L. Stone, J. Schmidt, J. G. Thomas, Q. Ding, H. C. Chang, M. L. Tsai, J. H. He and S. Jin, Efficient hydrogen evolution catalysis using ternary pyrite-type cobalt phosphosulphide, Nat. Mater., 2015, 14, 1245–1251 CrossRef.
- G. Yang, J. Zhu, P. Yuan, Y. Hu, G. Qu, B. A. Lu, X. Xue, H. Yin, W. Cheng, J. Cheng, W. Xu, J. Li, J. Hu, S. Mu and J. N. Zhang, Regulating Fe-spin state by atomically dispersed Mn-N in Fe-N-C catalysts with high oxygen reduction activity, Nat. Commun., 2021, 12, 1734 CrossRef CAS.
- J. Zhang, R. Cui, C. Gao, L. Bian, Y. Pu, X. Zhu, X. a. Li and W. Huang, Electrocatalytic water splitting: cation–modulated HER and OER activities of hierarchical VOOH hollow architectures for high–efficiency and stable overall water splitting, Small, 2019, 15, 1970255 CrossRef.
- B. Zhang, Y. Zheng, T. Ma, C. Yang, Y. Peng, Z. Zhou, M. Zhou, S. Li, Y. Wang and C. Cheng, Designing MOF nanoarchitectures for electrochemical water splitting, Adv. Mater., 2021, 33, e2006042 CrossRef PubMed.
- S. Zhao, C. Tan, C. T. He, P. An, F. Xie, S. Jiang, Y. Zhu, K. H. Wu, B. Zhang, H. Li, J. Zhang, Y. Chen, S. Liu, J. Dong and Z. Tang, Structural transformation of highly active metal-organic framework electrocatalysts during the oxygen evolution reaction, Nat. Energy, 2020, 5, 881–890 CrossRef CAS.
- S. Pan, X. Mao, J. Yu, L. Hao, A. Du and B. Li, Remarkably improved oxygen evolution reaction activity of cobalt oxides by an Fe ion solution immersion process, Inorg. Chem. Front., 2020, 7, 3327–3339 RSC.
- P. Q. Liao, J. Q. Shen and J. P. Zhang, Metal-organic frameworks for electrocatalysis, Coord. Chem. Rev., 2018, 373, 22–48 CrossRef CAS.
- S. Zhao, Y. Wang, J. Dong, C. T. He, H. Yin, P. An, K. Zhao, X. Zhang, C. Gao and L. Zhang, Ultrathin metal-organic framework nanosheets for electrocatalytic oxygen evolution, Nat. Energy, 2016, 1, 1–10 CrossRef.
- J. Li, W. Huang, M. Wang, S. Xi, J. Meng, K. Zhao, J. Jin, W. Xu, Z. Wang, X. Liu, Q. Chen, L. Xu, X. Liao, Y. Jiang, K. A. Owusu, B. Jiang, C. Chen, D. Fan, L. Zhou and L. Mai, Low-crystalline bimetallic metal–organic framework electrocatalysts with rich active sites for oxygen evolution, ACS Energy Lett., 2018, 4, 285–292 CrossRef.
- Y. Cui, Y. Xue, R. Zhang, J. Zhang, X. a. Li and X. Zhu, Vanadium-cobalt oxyhydroxide shows ultralow overpotential for the oxygen evolution reaction, J. Mater. Chem. A, 2019, 7, 21911–21917 RSC.
- B. Patil, B. Satılmış, M. A. Khalily and T. Uyar, Atomic layer deposition of NiOOH/Ni(OH)2 on PIM-1-based N-doped carbon nanofibers for electrochemical water splitting in alkaline medium, ChemSusChem, 2019, 12, 1469–1477 CrossRef CAS PubMed.
- X. Su, Y. Wang, J. Zhou, S. Gu, J. Li and S. Zhang, Operando spectroscopic identification of active sites in NiFe prussian blue analogues as electrocatalysts: activation of oxygen atoms for oxygen evolution reaction, J. Am. Chem. Soc., 2018, 140, 11286–11292 CrossRef CAS PubMed.
- Z. Xiao, Y. Mei, S. Yuan, H. Mei, B. Xu, Y. Bao, L. Fan, W. Kang, F. Dai, R. Wang, L. Wang, S. Hu, D. Sun and H. C. Zhou, Controlled hydrolysis of metal-organic frameworks: hierarchical Ni/Co-layered double hydroxide microspheres for high-performance supercapacitors, ACS Nano, 2019, 13, 7024–7030 CrossRef CAS PubMed.
- M. Liu, L. Kong, X. Wang, J. He and X. H. Bu, Engineering bimetal synergistic electrocatalysts based on metal–organic frameworks for efficient oxygen evolution, Small, 2019, 15, 1903410 CrossRef CAS PubMed.
- M. Laipan, R. Zhu, J. Zhu and H. He, Visible light assisted fenton-like degradation of orange II on Ni3Fe/Fe3O4 magnetic catalyst prepared from spent FeNi layered double hydroxide, J. Mol. Catal. A: Chem., 2016, 415, 9–16 CrossRef CAS.
- J. Huang, Y. Li, R. K. Huang, C. T. He, L. Gong, Q. Hu, L. Wang, Y. T. Xu, X. Y. Tian and S. Y. Liu, Electrochemical exfoliation of pillared–layer metal-organic framework to boost the oxygen evolution reaction, Angew. Chem., 2018, 130, 4722–4726 CrossRef.
- X. Li, R. Ding, W. Shi, Q. Xu, D. Ying, Y. Huang and E. Liu, Hierarchical porous Co(OH)F/Ni(OH)2: a new hybrid for supercapacitors, Electrochim. Acta, 2018, 265, 455–473 CrossRef CAS.
- W. Li, S. a. He, X. Wang, Q. Ma and C. h. Zhao, A BiOCl/β–FeOOH heterojunction for HER photocatalytic performance under visible–light illumination, Int. J. Energy Res., 2019, 43, 2162–2171 CrossRef CAS.
- A. Sivanantham, P. Ganesan, A. Vinu and S. Shanmugam, Surface activation and reconstruction of non-oxide-based catalysts through in situ electrochemical tuning for oxygen evolution reactions in alkaline media, ACS Catal., 2019, 10, 463–493 CrossRef.
- M. Görlin, J. H. Stenlid, S. Koroidov, H. Y. Wang, M. Börner, M. Shipilin, A. Kalinko, V. Murzin, O. V. Safonova and M. Nachtegaal, Key activity descriptors of nickel-iron oxygen evolution electrocatalysts in the presence of alkali metal cations, Nat. Commun., 2020, 11, 1–11 Search PubMed.
- X. Wang, M. Liu, H. Zhang, S. Yan, C. Zhang and S. Liu, Spontaneous migration induced Co nanokarstcave encapsulated in N-doped carbon hybrids for efficient oxygen electrocatalyst, Nano Res., 2021, 14, 4569–4576 CrossRef CAS.
- M. Liu, L. Kong, X. Wang, J. He, J. Zhang, J. Zhu and X. H. Bu, Deciphering of advantageous electrocatalytic water oxidation behavior of metal-organic framework in alkaline media, Nano Res., 2021, 14, 4680–4688 CrossRef CAS.
- X. Wang, M. Liu, H. Yu, H. Zhang, S. Yan, C. Zhang and S. Liu, Oxygen-deficient 3D-ordered multistage porous interfacial catalysts with enhanced water oxidation performance, J. Mater. Chem. A, 2020, 8, 22886–22892 RSC.
- J. Y. Chen, L. Dang, H. Liang, W. Bi, J. B. Gerken, S. Jin, E. E. Alp and S. S. Stahl, Operando analysis of NiFe and Fe oxyhydroxide electrocatalysts for water oxidation: detection of Fe4+ by Mossbauer spectroscopy, J. Am. Chem. Soc., 2015, 137, 15090–15093 CrossRef CAS PubMed.
- H. S. Ahn and A. J. Bard, Surface Interrogation Scanning Electrochemical Microscopy of Ni1−xFexOOH (0<x < 0.27) Oxygen Evolving Catalyst: Kinetics of the “fast” Iron Sites, J. Am. Chem. Soc., 2016, 138, 313–318 CrossRef CAS PubMed.
- S. Klaus, Y. Cai, M. W. Louie, L. Trotochaud and A. T. Bell, Effects of Fe electrolyte impurities on Ni(OH)2/NiOOH structure and oxygen evolution activity, J. Phys. Chem. C, 2015, 119, 7243–7254 CrossRef CAS.
- S. Jin, Are metal chalcogenides, nitrides, and phosphides oxygen evolution catalysts or bifunctional catalysts?, ACS Energy Lett., 2017, 2, 1937–1938 CrossRef CAS.
- B. Zhang, X. Zheng, O. Voznyy, R. Comin, M. Bajdich, M. García-Melchor, L. Han, J. Xu, M. Liu and L. Zheng, Homogeneously dispersed multimetal
oxygen-evolving catalysts, Science, 2016, 352, 333–337 CrossRef CAS PubMed.
- X. Kong, C. Zhang, S. Y. Hwang, Q. Chen and Z. Peng, Free–standing holey Ni(OH)2 nanosheets with enhanced activity for water oxidation, Small, 2017, 13, 1700334 CrossRef.
- P. Wei, Z. Hao, Y. Yang, M. Liu, H. Zhang, M.-R. Gao and S.-H. Yu, Unconventional dual-vacancies in nickel diselenide-graphene nanocomposite for high-efficiency oxygen evolution catalysis, Nano Res., 2020, 13, 3292–3298 CrossRef CAS.
- Y. Zhao, X. Jia, G. Chen, L. Shang, G. I. Waterhouse, L. Z. Wu, C. H. Tung, D. O'Hare and T. Zhang, Ultrafine NiO nanosheets stabilized by TiO2 from monolayer NiTi-LDH precursors: an active water oxidation electrocatalyst, J. Am. Chem. Soc., 2016, 138, 6517–6524 CrossRef CAS PubMed.
- H. Wu, X. Lu, G. Zheng and G. W. Ho, Topotactic engineering of ultrathin 2D nonlayered nickel selenides for full water electrolysis, Adv. Energy Mater., 2018, 8, 1702704 CrossRef.
- B. Q. Li, S. Y. Zhang, B. Wang, Z. J. Xia, C. Tang and Q. Zhang, A porphyrin covalent organic framework cathode for flexible Zn-air batteries, Energy Environ. Sci., 2018, 11, 1723–1729 RSC.
- L. Fan, P. Zhang, B. Zhang, Q. Daniel, B. J. Timmer, F. Zhang and L. Sun, 3D core-shell NiFeCr catalyst on a Cu nanoarray for water oxidation: synergy between structural and electronic modulation, ACS Energy Lett., 2018, 3, 2865–2874 CrossRef CAS.
- F. Hu, S. Zhu, S. Chen, Y. Li, L. Ma, T. Wu, Y. Zhang, C. Wang, C. Liu and X. Yang, Amorphous metallic NiFeP: a conductive bulk material achieving high activity for oxygen evolution reaction in both alkaline and acidic media, Adv. Mater., 2017, 29, 1606570 CrossRef.
- H. Liang, F. Meng, M. Caban-Acevedo, L. Li, A. Forticaux, L. Xiu, Z. Wang and S. Jin, Hydrothermal Continuous Flow Synthesis and Exfoliation of NiCoLayered Double Hydroxide Nanosheets for Enhanced OxygenEvolution Catalysis, Nano Lett., 2015, 15, 1421–1427 CrossRef CAS.
- S. Yin, W. Tu, Y. Sheng, Y. Du, M. Kraft, A. Borgna and R. Xu, A Highly Efficient Oxygen Evolution Catalyst Consisting of Interconnected Nickel-Iron-Layered Double Hydroxide and Carbon Nanodomains, Adv. Mater., 2018, 30, 1705106 CrossRef PubMed.
- Y. Yan, P. Gu, S. Zheng, M. Zheng, H. Pang and H. Xue, Facile synthesis of an accordion-like Ni-MOF superstructure for high-performance flexible supercapacitors, J. Mater. Chem. A, 2016, 4, 19078–19085 RSC.
Footnote |
† Electronic supplementary information (ESI) available. See DOI: 10.1039/d1qi00857a |
|
This journal is © the Partner Organisations 2022 |
Click here to see how this site uses Cookies. View our privacy policy here.