DOI:
10.1039/D3TC04511K
(Paper)
J. Mater. Chem. C, 2024,
12, 2953-2960
Ionic liquid-assisted growth of high-quality methylammonium lead bromide single crystals for photodetection applications†
Received
8th December 2023
, Accepted 18th January 2024
First published on 20th January 2024
Abstract
The control of nucleation temperature and growth kinetics during inverse temperature crystallization (ITC) is critical for achieving high-quality perovskite single crystals (SCs) for various optoelectronic applications. Here, we show that the addition of a 1-butyl-3-methylimidazolium bromide (BMIB) ionic liquid to the methylammonium lead bromide (MAPbBr3) precursor solution not only reduces the nucleation temperature from 80 °C to 60 °C but also substantially improves the crystal quality and optoelectronic properties. Specifically, MAPbBr3 SCs grown in the presence of BMIB exhibit better crystallinity with reduced lattice strain, nonradiative recombination and trap density compared to the MAPbBr3 SCs grown by the conventional ITC method at 80 °C. The high quality of the BMIB-based MAPbBr3 SCs enabled us to build an efficient planar-structured photodetector with high responsivity to green light (530 nm). This study shows that the ionic liquid-assisted growth of perovskite SCs has a significant influence on the crystal properties, which is beneficial for optoelectronic SC-based devices.
1. Introduction
Over the last decade, hybrid organic–inorganic lead halide perovskites (LHPs) have revolutionized the scenario of the existing semiconductor technologies due to their broad flexibility of material composition, easy bandgap tunability and outstanding optoelectronic properties.1–4 So far, most high-efficiency LHP-based optoelectronic devices are based on polycrystalline thin films, which suffer from high defect density formed by rapid crystallization processes and severe instabilities arising from large grain boundaries.5–8 In turn, LHPs in the form of single crystals (SCs) have been demonstrated to surmount these challenges and provide better stability, longer carrier diffusion length, lower trap density and higher charge mobility than polycrystalline thin films.9–13 Recently, the free-standing perovskite SCs have become a convenient platform for the next-generation optoelectronic devices including photodetectors (PDs),14–18 electronic eyes,19 image sensing in the medical field,20 light emitting diodes (LEDs),21,22 X-ray imaging,23–25 and field effect transistors (FETs).26
So far, various solution-based methods such as antisolvent vapor-assisted crystallization, slow evaporation, top-seed solution method and inverse temperature crystallization (ITC) have been adopted to grow high-quality LHP SCs with different compositions and shapes.27–31 Among them, the ITC method is particularly attractive, which is used for the rapid growth of high-quality and shape-controlled perovskite SCs within minutes by reduced solubility in different solutions at a range of elevated temperatures.29,32,33 However, the rapid crystal growth kinetics of the ITC method at high temperatures can also lead to the formation of small crystals and affect the crystal quality.34 The as-formed small crystals can further serve as seed nucleation sites for the growth of larger SCs. This seed-assisted growth has been widely employed in the literature as a method to yield large and high-quality LHP SCs.35–37 Recently, Zia et al. reported the seed-assisted ITC method, which reduces the crystallization temperature from 80 °C to 65 °C for methylammonium lead bromide (MAPbBr3) crystal growth.38 This low-temperature seed-assisted method plays a positive role in the optical and photodetector properties of MAPbBr3 SCs. However, this requires a two-step crystallization process, which increases the complexity and cost of the overall process. To address this issue, changing the crystallization environment was proposed as an efficient method to produce large LHP SCs with high quality at low temperatures. For example, the addition of formic acid39–41 or 3-(decyldimethylammonio)-propane-sulfonate inner salt (DPSI)42 to a perovskite precursor solution facilitates the rapid growth of high-quality SCs with reduced defect density and enables crystallization at lower temperatures without the help of the seed crystals. Recent works revealed that the addition of ionic liquids (ILs) to the perovskite precursor solution improves the crystallization propensity for the acquisition of high-quality polycrystalline perovskite films with a low number of defects.43,44 Due to the hybrid structures of anions and cations, ILs can form strong hydrogen and coordination bonds in solution with the perovskite components, modifying the crystallization kinetics.45,46 However, to the best of our knowledge, the use of ILs for the controlled growth of LHP SCs has not been reported.
Here, we show that the addition of a 1-butyl-3-methylimidazolium bromide (BMIB) IL to the MAPbBr3 precursor solution is promising for the growth of high-quality MAPbBr3 SCs and for reducing the nucleation temperature from 80 °C to 60 °C. First, the effect of various BMIB concentrations on the nucleation time, crystallization rate and growth temperature of MAPbBr3 SCs was examined. It was found that the presence of an optimized amount of BMIB IL facilitated the self-assembly and crystallization process via the formation of PbBr2–ionic liquid complexes, which reduced the crystallization kinetics for the growth of large SCs. X-ray diffraction (XRD) analysis revealed a reduced lattice strain in MAPbBr3 SCs grown in the presence of BMIB compared to the MAPbBr3 SCs obtained using the conventional ITC method at 80 °C. In addition, the BMIB-based MAPbBr3 SC exhibits better optical and electrical properties, which further confirms their high quality and potential for utilization in photodetection. A planar-structured PD based on a BMIB-based MAPbBr3 SC exhibits responsivity and EQE as high as 4.81 A W−1 and 1124% under green light (530 nm) at 2 V bias, respectively. These values are much better than those of the device based on the MAPbBr3 SC formed by the conventional ITC method at 80 °C.
2. Results and discussion
The molecular structure of the ionic liquid (BMIB) used in this study is shown in Fig. S1 (ESI†). MAPbBr3 SCs were grown using the ITC method, as previously reported.47,48 For this conventional nucleation growth, a 1.2 M MAPbBr3 precursor solution in DMF was heated to a temperature of 80 °C at a ramp of 20 °C h−1. It was observed that almost 30 min were required to start the nucleation process at 80 °C, and SCs with the desired dimensions of ∼4 × 4 × 2 mm3 were observed after continuing crystallization for 4 h (Fig. 1a and Fig. S2, ESI†). We also tested the crystallization process of a 1.2 M MAPbBr3 precursor solution at a lower temperature (60 °C); however, at this temperature, SCs were not observed even after 24 h. This is consistent with the work of Liu et al., where ∼18 days were required to reach sufficient supersaturation for the nucleation and growth of MAPbBr3 SCs at a lower temperature of 60 °C.49 For crystal growth with the IL additive, BMIB was added to the perovskite precursor solution at various concentrations (1, 3, 5 and 10 mol%) (Fig. 1b). The temperature for crystallization was set as 60 °C using a ramp of 20 °C h−1. The nucleation time (after reaching 60 °C) varied with increasing the concentration of BMIB, as shown in Table S1 (ESI†). It was observed that SCs with desired dimensions were obtained from the precursor solution with 5 mol% BMIB after growing for 12 h (Fig. S3, ESI†). With lower concentrations of BMIB (1 mol% and 3 mol%), only small crystallites were formed after 12 h due to the very slow crystallization rate (Fig. S4, ESI†). However, increasing the BMIB concentration to 10 mol% failed to initiate the nucleation at 60 °C.
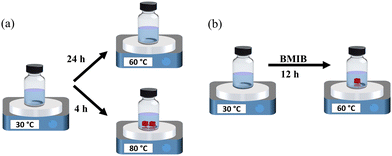 |
| Fig. 1 Schematic of the crystallization procedure of the MAPbBr3 SC (a) without and (b) with BMIB. | |
It is known that the growth of SCs is generally controlled by the diffusion rate of the solute in the solution and the solute deposition rate on the crystal surface.38 The diffusion rate in solution exponentially increases with increasing the temperature according to eqn (1):
| 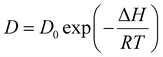 | (1) |
where
D is the diffusion rate, Δ
H is the diffusion activation rate,
D0 is the diffusion constant,
T is the solution temperature and
R is the gas constant. Therefore, a high diffusion rate at high temperatures (80 °C) can lead to the formation of SCs with defects and imperfections.
29,50 To control the crystal growth quality at low temperatures, it is important to find a balance between the diffusion rate and solute deposition rate. The addition of 5% BMIB to the MAPbBr
3 precursor solution helped to overcome the nucleation energy for the formation of nuclei at a low temperature of 60 °C and assisted in growing crystals below the supersaturation limit. To further investigate the effect of 5 mol% of BMIB on the crystallization rate, we attempted the crystallization of MAPbBr
3 SCs with and without the BMIB additive at 80 °C (Fig. S5, ESI
†). The time required to start the nucleation process for the precursor solution with BMIB was around ∼60 min, which is much longer than the nucleation time (∼30 min) for the precursor solution without BMIB. The prolonged nucleation process in the precursor solution with BMIB could be attributed to the interactions between the BMIB and perovskite component, which restrict the diffusion of ions and affect the growth rate of MAPbBr
3 SCs (
vide infra). To compare the crystallization rate, we continued the crystallization process for the next 6 h after the nucleation process. As seen in Table S2 (ESI
†), the addition of BMIB to the perovskite solution decreases the growth rate and allows control over the formation of SCs in the ITC process.
The change in the crystal growth kinetics of the MAPbBr3 SC by the addition of BMIB could affect its crystal structure and quality. To confirm this, we first checked whether BMIB existed in the bulk and/or at the surface of the MAPbBr3 SC using liquid-state 1H NMR spectroscopy. As shown in Fig. S6 (ESI†), the lack of characteristic signals of BMIB in the respective NMR spectra indicates that this additive does not exist in the crystal structure. However, BMIB molecules may interact with the perovskite component (PbBr2) in the precursor solution via hydrogen bonding interactions51 and influence the crystallization process by decreasing the solute diffusion rate.38,50 Fig. S7 (ESI†) shows the 1H NMR spectrum of the simple reaction between BMIB and PbBr2 in a 1
:
1 molar ratio. As seen, the peak belonging to BMIB at 9.18 ppm shifts toward the high field region to 9.11 ppm after the addition of PbBr2, which suggests the formation of salt [BMI]PbBr3, as revealed by Zhang et al.51 XRD analysis was further conducted to study the effect of BMIB on the crystal structure and crystallinity of the MAPbBr3 SC. The XRD patterns of the maximal facet for both MAPbBr3 SCs showed characteristic peaks along (100), (200), and (300) planes, confirming their high purity (Fig. 2a). However, the much stronger intensities of these peaks for the BMIB-based MAPbBr3 SC suggests superior crystallinity. Furthermore, the full width at half-maximum (FWHM) analysis of the three major peaks can provide the lattice strain using the tangent formula (see Supplementary Note 1, ESI†).52 The FWHM and lattice strain values of both SCs are summarized in Table S3 (ESI†). As seen, low FWHM was found for the BMIB-based MAPbBr3 SC, which indicates a reduced lattice strain within this SC. The reduced lattice strain is beneficial for decreasing ion migration within the perovskite crystal and nonradiative recombination processes.38,53
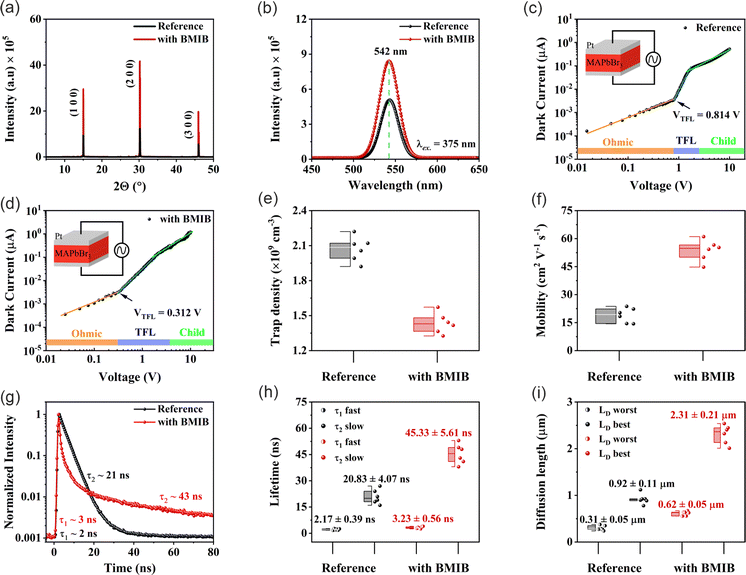 |
| Fig. 2 (a) XRD patterns and (b) steady-state photoluminescence spectra of the reference and BMIB-based MAPbBr3 SCs. Pulsed voltage SCLC I–V measurement curves for all three regimes of the (c) reference and (d) BMIB-based MAPbBr3 SCs. (e) Trap density (η), (f) mobility (μ) and (g) time-resolved photoluminescence (TRPL) of the reference and BMIB-based MAPbBr3 SCs. (h) Carrier lifetime and (i) diffusion length (LD) statistics for each type of studied SCs. | |
The trap states located within the band gap of perovskite materials play a critical role of undesirable obstacles in all photophysical applications.31 To shed more light on the energy states and defects, photoluminescence (PL) measurements were performed on both types of SCs. As shown in Fig. 2b, the PL spectra exhibit a sharp peak at 542 nm (2.29 eV) with no shift in the peak position, which is consistent with the literature.38,50 Notably, the BMIB-based MAPbBr3 SC exhibited an enhanced PL intensity compared to the reference MAPbBr3 SC, which indicates suppression of the nonradiative recombination in the modified SC. These results further support our findings from the XRD analysis.
Next, we investigated the charge transport and electronic properties of these SCs using the space charge-limited current (SCLC) method.31,54 The values of conductivity (σ), trap density (ηtrap) and mobility (μ) were calculated by recording the dark current of the Pt/SC/Pt hole-only devices with a vertical geometry. The pulsed voltage sweep SCLC method was used to minimize the ion migration effect during the measurement.55Fig. 2c and d displays the dark I–V characteristics of the reference and BMIB-based MAPbBr3 SCs. Both dark I–V curves exhibit three distinct regions marked as ohmic, trap-filling and child regions. The conductivity (σ) of both types of SCs was calculated from the ohmic region, and the BMIB-based MAPbBr3 SC shows a higher value of σ than the reference MAPbBr3 SC (Fig. S8, ESI†). For the trap-filling region and considering a linear dependence between ηtrap and the trap-filled limit voltage (VTFL), the hole densities (ηh) are calculated using eqn (2):31
| 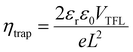 | (2) |
where
ε,
L,
ε0 and
e are the dielectric constant, thickness of the crystals, vacuum permittivity and elementary charge, respectively. To minimize the error in the measurement, we tested six separate reference and BMIB-based MAPbBr
3 SCs. The average
ηtrap was found to be 2.07 ± 0.11 × 10
9 cm
−3 for the reference MAPbBr
3 SC, while the values of
ηtrap decreased by ∼40% and were equal to 1.2 ± 0.08 × 10
9 cm
−3 for the BMIB-based MAPbBr
3 SC (
Fig. 2e). The carrier mobility (
μ) for holes in the SCs studied can also be calculated from the trap-filled SCLC regions of hole-only devices using the Mott–Gurney law
(3):
|  | (3) |
where
Jd and
V are the dark current density and the applied bias, respectively. As expected, the BMIB-based MAPbBr
3 SC exhibits a high average
μ of 63.9 ± 5.4 cm
2 V
−1 s
−1, which is more than two times higher than
μ calculated for the reference MAPbBr
3 SC (23.8 ± 3.4 cm
2 V
−1 s
−1) (
Fig. 2f). The ultralow trap density and high carrier mobility indicate the excellent quality of the BMIB-based MAPbBr
3 SC.
To study the recombination dynamics of the photoexcited species, both SCs were investigated by time-resolved PL (TRPL) spectroscopy. We fitted the decay curves using the biexponential decay with a fast component (τ1) and a slow component (τ2). The fast (τ1) and slow (τ2) decay components are associated with the nonradiative recombination arising from the surface defects and bulk radiative recombination, respectively.56 As shown in Fig. 2g, the BMIB-based MAPbBr3 SC (τ1 = 3 ns and τ2 = 43 ns) exhibits a longer carrier lifetime than the reference MAPbBr3 SC (τ1 = 2 ns and τ2 = 21 ns). As can be seen, the fast and slow components are longer for the BMIB-based MAPbBr3 SC, which suggests reduced surface and bulk nonradiative recombination in this type of crystal. It can be observed that the difference between the carrier lifetimes is very small, and the values change with changes in the measurement position, excitation wavelength, crystalline plane, and measurement time.57–59 Therefore, we investigated three individual crystals for each type of sample and collected time-resolved PL (TRPL) decay for a longer decay time scale to calculate the diffusion length (LD) (Fig. S9, ESI†). The fitted decay curves show three decay components (two fast components and one slow component). The statistical distributions of the average fast and slow components for each crystal are shown in Fig. 2h. As can be seen, the average fast component is almost the same for both types of SCs. The slow component is longer for the BMIB-based MAPbBr3 SC, which suggests a reduced bulk nonradiative recombination in this type of crystal. Based on the calculated μ and τ, the diffusion length (LD) for each SC can be estimated using eqn (4):29
| 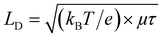 | (4) |
where
kB and
T are the Boltzmann constant and the temperature, respectively. The average
LD values based on the fast and slow components of the carrier lifetime are 1.72 ± 0.18 μm and 4.46 ± 0.47 μm for the BMIB-based MAPbBr
3 SC, while those for the reference MAPbBr
3 SC are 2.88 ± 0.15 μm and 9.74 ± 0.52 μm respectively (
Fig. 2i). The values of
LD in the BMIB-based MAPbBr
3 SC are ∼2 times higher than those of reference MAPbBr
3 SC, which supports its better quality and optoelectronic properties.
For high-performance optoelectronic devices, especially in SC-based PDs, the quality of the active material and its optoelectronic properties play a significant role. Having in hand the high-quality MAPbBr3 SC, we fabricated planar-type PDs by depositing Pt electrodes on the surfaces of reference and BMIB-based MAPbBr3 SCs according to our previous study (for the device fabrication, see the Experimental section).47 First, we collected the I–V characteristics under dark conditions, as shown in Fig. 3a. As can be seen, BMIB-based PD exhibits a higher dark current (Id) than that of the reference PD. The observed higher Id may primarily suggest the presence of a high defect density in the BMIB-based MAPbBr3 SC.38,50 However, our results reveal that the values of ηtrap are lower in the BMIB-based MAPbBr3 SC than in the reference MAPbBr3 SC (Fig. 2e). Thus, we attributed the higher Id to the high conductivity and hole mobility of the BMIB-based MAPbBr3 SC. The quality of the PDs was further verified by analyzing the photocurrent under light and an applied bias. The photocurrent (Iph) increases with increasing bias for each type of PD, and the BMIB-based PD exhibits a higher Iph than the reference PD.
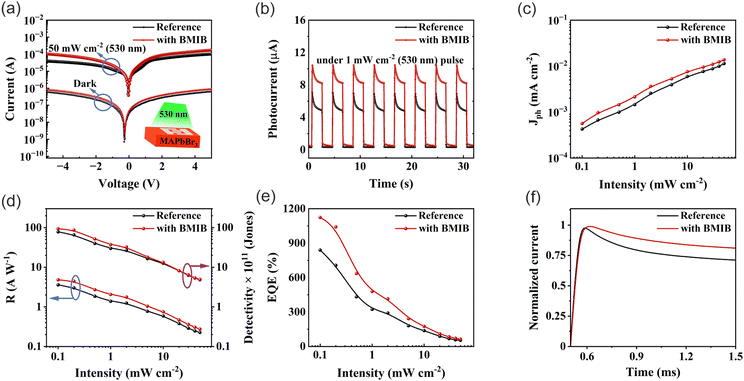 |
| Fig. 3 (a) Dark and light I–V characteristics. (b) Current–time (I–t) characteristics of the reference and BMIB-based PDs. (c) Photocurrent density (Jph), (d) R (left axis), D* (right axis), (e) EQE and (f) normalized transient photocurrent of the reference and BMIB-based PDs under green light at 2 V. | |
As the halide perovskites are known to exhibit hysteretic behaviour due to ion migration,12,60 we studied the effect of BMIB on hysteresis in PD. Fig. S10 (ESI†) shows the comparison of the I–V characteristics measured in forward and reverse scan directions at a 100 mV s−1 scan rate under a 50 mW cm−2 illumination. The lower difference in the current under forward and reverse bias (ΔI = Ibackward − Iforward) for the BMIB-based PD suggests reduced ion migration, which is beneficial for a stable performance. To shed more light on PD performance, we measured the transient photoresponses of both the PDs under a green LED (λ = 530 nm) light pulse with irradiance power densities ranging from 0.1 to 50 mW cm−2 at a fixed bias voltage of 2 V (Fig. S11, ESI†). The current–time (I–t) characteristics of each type of PD at a 1 mW cm−2 irradiance power are shown in Fig. 3b. The observed overshooting sharp transient peaks followed by photocurrent decay for a longer time scale in both I–t curves could be due to the dominant effect of the charge recombination and ion accumulation near the electrode with negligible effect of carrier trapping under the green light.61 The observed higher photocurrent on the BMIB-based PD indicates better photogenerated charge separation due to lower nonradiative recombination. The logarithmic photocurrent density (Jph) as a function of incident light intensity for each type of PD is shown in Fig. 3c. As can be seen, the values of Jph increase with increasing intensity of the incident light due to the increase in the charge generation rate. Notably, the BMIB-based PD generated almost 30% higher Jph than the reference MAPbBr3 SC-based PD, which suggests its improved performance. The responsivity (R), specific detectivity (D*), and external quantum efficiency (EQE) of the studied device were calculated using eqn (S3), (S4) and (S5), respectively (see Supplementary Note 2, ESI†). From Fig. 3d and e, we can observe that the BMIB-based PD exhibits higher R and EQE than the reference MAPbBr3 SC-based PD. The maximum R and EQE increases to 4.81 A W−1 and 1124% for the BMIB-based PD compared to the reference MAPbBr3 SC-based PD (3.5 A W−1 and 839%) under a green light of 0.1 mW cm−2. However, there was no significance difference in the values of D*, and the higher Id of the BMIB-based PD did not affect the overall performance of the PDs. The improved photodetection properties of the BMIB-based PD can also be attributed to longer LD, which plays a vital role in the extraction of photogenerated charge carriers and directly controls the responsivity of PDs.47
The decay behaviour of transient photoresponse of the MAPbBr3 SC-based PDs could give important information about charge recombination and ion accumulation under green light and bias.61 The normalized spectra of transient photocurrent decay of each type of PD at a 1 mW cm−2 irradiance power are shown in Fig. 3f. The observed slower photocurrent decay under green light on the BMIB-based PD indicates a lower level of charge recombination and ion accumulation than that of the reference MAPbBr3 SC-based PD. The response time depends on the capacity of PDs to follow the rapidly changing optical signals. The rise-time (τon, from 10% to 90% of the saturated values) and fall-time (τoff, from 90% to 10% of the saturated values) for each type of PD were extracted from the normalized photoresponse characteristics, as shown in Fig. S12. The BMIB-based PD exhibits faster response time (τon = 12.1 ms, τoff = 14.8 ms) compared to the reference MAPbBr3 SC-based PD with τon = 14.3 ms and τoff = 16.1 ms due to higher conductivity and mobility, which allows faster transport of the photogenerated charge carrier. The operational stability of PDs under continuous operation is an essential requirement from the commercialization perspective. The functional stability of the PDs is shown in Fig. S13 (ESI†). The output of both PDs is stable (∼92% performance remaining after 24 h of continuous operation under a 1 mW cm−2 optical signal), which is comparable with the previous studies.47,62 These results confirm that the BMIB-based MAPbBr3 SC can be an efficient semiconducting material for the fabrication of devices with excellent PD performance.
3. Conclusions
In summary, we have demonstrated a modified ITC approach to grow high-quality MAPbBr3 SCs through the addition of BMIB to the perovskite precursor solution, which allowed crystallization to occur at 60 °C. The addition of BMIB was found to facilitate the self-assembly and crystallization processes via the formation of PbBr2–ionic liquid complexes, which reduces the crystallization kinetics for the growth of large MAPbBr3 SCs. The structural, optical and electrical properties of the as-formed BMIB-based MAPbBr3 SC were studied and compared to those of the MAPbBr3 SC obtained by using conventional ITC method at 80 °C. The results revealed that BMIB-based MAPbBr3 SCs exhibited enhanced crystallinity with reduced lattice strain, nonradiative recombination and trap density, which make them attractive for photodetection applications. The planar PD with a structure of Pt/MAPbBr3 SC/Pt exhibited an R of 4.81 A W−1, EQE of 1124% and response time of 12.1 ms under 0.1 mW cm−2 green light (530 nm) and a 2 V bias, which are higher than those of the device based on the MAPbBr3 SC formed using the conventional ITC method at 80 °C and comparable to previously reported studies on MAPbBr3 SC-based PDs (Table S4, ESI†). The superior performance can be attributed to the observed high mobility, conductivity and diffusion length of the BMIB-based MAPbBr3 SC. We believe that the use of a low-temperature ITC method for the growth of high-quality MAPbBr3 SCs will lead to the development of a new generation of SC-based optoelectronic devices.
4. Experimental section
4.1. Materials
Lead(II) bromide (PbBr2, ≥98%) and dimethyl formamide (DMF, anhydrous 99.8%) were obtained from Sigma-Aldrich, methylammonium bromide (MABr, >99.99%) was obtained from Great Cell Solar Materials, and 1-butyl-3-methylimidazolium bromide IL (BMIB, >98%) was obtained from Sigma-Aldrich. All chemicals were used as received without any further purification.
4.2. Synthesis and characterization of SCs
Synthesis of MAPbBr3 SCs without and with BMIB ILs.
Equimolar amounts of MABr (1344 mg) and PbBr2 (4404 mg) were dissolved in 10 mL of DMF to make a 1.2 M solution. After complete dissolution, the mixture was filtered using a PTFE 0.2 μm filter, divided into 3 different vials, and slowly heated to 80 °C. After 8 h, SCs with sizes over 4 × 4 × 2 mm were crystallized, dried and used for further measurements. To synthesize MAPbBr3 with the BMIB IL, 1 (26 mg), 3 (79 mg), 5 (131 mg) and 10 (263 mg) mol% of BMIB IL were added to 10 mL 1.2 M precursor solution of MAPbBr3. After complete dissolution, the solution was filtered using a PTFE 0.2 μm filter, divided into three vials, and slowly heated to 60 °C. After 12 h, SCs with sizes over 4 × 4 × 2 mm were formed, dried and used for further measurements.
X-ray diffraction on SCs was performed using an Empyrean diffractometer (PANalytical) using a copper lamp (40 kV, 40 mA) with 2θ of over 10° to 50°, keeping the SC sample stationary. Steady-state photoluminescence spectra were collected using a Shimadzu RF-6000 spectrometer. Transiently resolved photoluminescence (TRPL) spectra were recorded using an Edinburgh Instrument (FLS1000). For SCLC measurements, vertical devices were made by sputtering Pt electrodes on the crystal's top face and opposite bottom face from 0 to 10 V with a step voltage of 25 mV. A short time voltage pulse (20 ms) was followed by a long rest time at 0 V (2 min).
4.3. Fabrication and characterization of the MAPbBr3 SC-based PDs
A 60 nm thick platinum (Pt) electrode was deposited over the (100) facets of the SCs using a spluttered magnetron (Leica EM MED020) with the help of a self-designed mask with a 150 μm channel. All dark J–V and PD response measurements were performed using a LASC probe station connected to a Bio-Logic SP-150e potentiostat at a scan rate of 100 mV s−1. Using Thorlabs GmbH, PM 100D, the illumination power of a green LED (Luxeon Star LEDs) was optimized.
Author contributions
A. M. – investigation, methodology, writing – original draft; V. A. – investigation, data curation; J. K. – formal analysis, visualization; N. M. – data curation, formal analysis; P. S. – data curation, formal analysis; P. Y. – formal analysis; D. P. – conceptualization, supervision, writing – review and editing.
Conflicts of interest
There are no conflicts to declare.
Acknowledgements
D. P., A. M. and V. A. acknowledge the National Science Centre (Grant OPUS-20, No. 2020/39/B/ST5/01497) for financial support. N. M. and P. S. acknowledge the financial support from the Slovak Research and Development Agency (APVV-21-0297 and SK-CZ-RD-21-0043) and the Slovak Academy of Sciences (V4-Japan/JRP/2021/96/PeDET).
References
- J. Miao and F. Zhang, J. Mater. Chem. C, 2019, 7, 1741–1791 RSC.
- M. Ahmadi, T. Wu and B. Hu, Adv. Mater., 2017, 29, 1605242 CrossRef PubMed.
- Z. Yi, N. H. Ladi, X. Shai, H. Li, Y. Shen and M. Wang, Nanoscale Adv., 2019, 1, 1276–1289 RSC.
- L. Chouhan, S. Ghimire, C. Subrahmanyam, T. Miyasaka and V. Biju, Chem. Soc. Rev., 2020, 49, 2869–2885 RSC.
- W. A. Dunlap-Shohl, Y. Zhou, N. P. Padture and D. B. Mitzi, Chem. Rev., 2019, 119, 3193–3295 CrossRef CAS PubMed.
- J. Sun, J. Wu, X. Tong, F. Lin, Y. Wang and Z. M. Wang, Adv. Sci., 2018, 5, 1700780 CrossRef PubMed.
- T. S. Sherkar, C. Momblona, L. Gil-Escrig, J. Ávila, M. Sessolo, H. J. Bolink and L. J. A. Koster, ACS Energy Lett., 2017, 2, 1214–1222 CrossRef CAS PubMed.
- W. Fu, A. G. Ricciardulli, Q. A. Akkerman, R. A. John, M. M. Tavakoli, S. Essig, M. V. Kovalenko and M. Saliba, Mater. Today, 2022, 58, 275–296 CrossRef CAS.
- S. D. Stranks, G. E. Eperon, G. Grancini, C. Menelaou, M. J. P. Alcocer, T. Leijtens, L. M. Herz, A. Petrozza and H. J. Snaith, Science, 2013, 342, 341–344 CrossRef CAS PubMed.
- Q. Dong, Y. Fang, Y. Shao, P. Mulligan, J. Qiu, L. Cao and J. Huang, Science, 2015, 347, 967–970 CrossRef CAS PubMed.
- X.-D. Wang, W.-G. Li, J.-F. Liao and D.-B. Kuang, Solar RRL, 2019, 3, 1800294 CrossRef.
- A. Mahapatra, R. Runjhun, J. Nawrocki, J. Lewiński, A. Kalam, P. Kumar, S. Trivedi, M. M. Tavakoli, D. Prochowicz and P. Yadav, Phys. Chem. Chem. Phys., 2020, 22, 11467–11473 RSC.
- S. Trivedi, D. Prochowicz, N. Parikh, A. Mahapatra, M. K. Pandey, A. Kalam, M. M. Tavakoli and P. Yadav, ACS Omega, 2021, 6, 1030–1042 CrossRef CAS PubMed.
- Y. Zhang, Y. Liu and S. (Frank) Liu, Adv. Funct. Mater., 2023, 33, 2210335 CrossRef CAS.
- J. Li, Y. Gu, Z. Han, J. Liu, Y. Zou and X. Xu, J. Phys. Chem. Lett., 2022, 13, 274–290 CrossRef CAS PubMed.
- J. Zhou and J. Huang, Adv. Sci., 2018, 5, 1700256 CrossRef PubMed.
- L. Lu, W. Weng, Y. Ma, Y. Liu, S. Han, X. Liu, H. Xu, W. Lin, Z. Sun and J. Luo, Angew. Chem., Int. Ed., 2022, 134, e202205030 CrossRef.
- Y. Ma, W. Guo, Q. Fan, H. Xu, L. Tang, Y. Liu, W. Li, X. Liu, J. Luo and Z. Sun, Adv. Funct. Mater., 2023, 33, 2210235 CrossRef CAS.
- Q. Wang, G. Zhang, H. Zhang, Y. Duan, Z. Yin and Y. Huang, Adv. Funct. Mater., 2021, 31, 2100857 CrossRef CAS.
- L. Li, S. Ye, J. Qu, F. Zhou, J. Song and G. Shen, Small, 2021, 17, 2005606 CrossRef CAS PubMed.
- X. Zhao, S. Wang, X. Shan, G. Meng and X. Fang, Cryst. Growth Des., 2021, 21, 5983–5997 CrossRef CAS.
- Y. Cho, H. R. Jung and W. Jo, Nanoscale, 2022, 14, 9248–9277 RSC.
- Z. Pan, L. Wu, J. Jiang, L. Shen and K. Yao, J. Phys. Chem. Lett., 2022, 13, 2851–2861 CrossRef CAS PubMed.
- K. Sakhatskyi, B. Turedi, G. J. Matt, E. Wu, A. Sakhatska, V. Bartosh, M. N. Lintangpradipto, R. Naphade, I. Shorubalko, O. F. Mohammed, S. Yakunin, O. M. Bakr and M. V. Kovalenko, Nat. Photonics, 2023, 17, 510–517 CrossRef CAS.
- Z. Zhang, H. Li, H. Di, D. Liu, W. Jiang, J. Ren, Z. Fan, F. Liao, L. Lei, G. Li, Y. Xiong and Y. Zhao, ACS Appl. Electron. Mater., 2023, 5, 388–396 CrossRef CAS.
- W. Yu, F. Li, L. Yu, M. R. Niazi, Y. Zou, D. Corzo, A. Basu, C. Ma, S. Dey, M. L. Tietze, U. Buttner, X. Wang, Z. Wang, M. N. Hedhili, C. Guo, T. Wu and A. Amassian, Nat. Commun., 2018, 9, 5354 CrossRef CAS PubMed.
- D. Shi, V. Adinolfi, R. Comin, M. Yuan, E. Alarousu, A. Buin, Y. Chen, S. Hoogland, A. Rothenberger, K. Katsiev, Y. Losovyj, X. Zhang, P. A. Dowben, O. F. Mohammed, E. H. Sargent and O. M. Bakr, Science, 2015, 347, 519–522 CrossRef CAS PubMed.
- A. A. Zhumekenov, V. M. Burlakov, M. I. Saidaminov, A. Alofi, M. A. Haque, B. Turedi, B. Davaasuren, I. Dursun, N. Cho, A. M. El-Zohry, M. De Bastiani, A. Giugni, B. Torre, E. Di Fabrizio, O. F. Mohammed, A. Rothenberger, T. Wu, A. Goriely and O. M. Bakr, ACS Energy Lett., 2017, 2, 1782–1788 CrossRef CAS.
- Y. Liu, Y. Zhang, Z. Yang, J. Feng, Z. Xu, Q. Li, M. Hu, H. Ye, X. Zhang, M. Liu, K. Zhao and S. (Frank) Liu, Mater. Today, 2019, 22, 67–75 CrossRef CAS.
- Y. Liu, X. Zheng, Y. Fang, Y. Zhou, Z. Ni, X. Xiao, S. Chen and J. Huang, Nat. Commun., 2021, 12, 1686 CrossRef CAS PubMed.
- A. Mahapatra, D. Prochowicz, J. Kruszyńska, S. Satapathi, S. Akin, H. Kumari, P. Kumar, Z. Fazel, M. Mahdi Tavakoli and P. Yadav, J. Mater. Chem. C, 2021, 9, 15189–15200 RSC.
- W. Wang, H. Meng, H. Qi, H. Xu, W. Du, Y. Yang, Y. Yi, S. Jing, S. Xu, F. Hong, J. Qin, J. Huang, Z. Xu, Y. Zhu, R. Xu, J. Lai, F. Xu, L. Wang and J. Zhu, Adv. Mater., 2020, 32, 2001540 CrossRef CAS PubMed.
- M. I. Saidaminov, A. L. Abdelhady, B. Murali, E. Alarousu, V. M. Burlakov, W. Peng, I. Dursun, L. Wang, Y. He, G. Maculan, A. Goriely, T. Wu, O. F. Mohammed and O. M. Bakr, Nat. Commun., 2015, 6, 7586 CrossRef PubMed.
- Z. Lian, Q. Yan, T. Gao, J. Ding, Q. Lv, C. Ning, Q. Li and J. Sun, J. Am. Chem. Soc., 2016, 138, 9409–9412 CrossRef CAS PubMed.
- Y. Liu, J. Sun, Z. Yang, D. Yang, X. Ren, H. Xu, Z. Yang and S. (Frank) Liu, Adv. Opt. Mater., 2016, 4, 1829–1837 CrossRef CAS.
- W.-G. Li, H.-S. Rao, B.-X. Chen, X.-D. Wang and D.-B. Kuang, J. Mater. Chem. A, 2017, 5, 19431–19438 RSC.
- Y. Zhao, H. Tan, H. Yuan, Z. Yang, J. Z. Fan, J. Kim, O. Voznyy, X. Gong, L. N. Quan, C. S. Tan, J. Hofkens, D. Yu, Q. Zhao and E. H. Sargent, Nat. Commun., 2018, 9, 1607 CrossRef PubMed.
- W. Zia, C. A. Aranda, J. Pospisil, A. Kovalenko, M. Rai, C. Momblona, S. Gorji, G. Muñoz-Matutano and M. Saliba, Chem. Mater., 2023, 35, 5458–5467 CrossRef CAS.
- P. K. Nayak, D. T. Moore, B. Wenger, S. Nayak, A. A. Haghighirad, A. Fineberg, N. K. Noel, O. G. Reid, G. Rumbles, P. Kukura, K. A. Vincent and H. J. Snaith, Nat. Commun., 2016, 7, 13303 CrossRef CAS PubMed.
- Q. Lv, Z. Lian, Q. Li, J.-L. Sun and Q. Yan, Chem. Commun., 2018, 54, 1049–1052 RSC.
- Y. Liu, Y. Zhang, X. Zhu, Z. Yang, W. Ke, J. Feng, X. Ren, K. Zhao, M. Liu, M. G. Kanatzidis and S. (Frank) Liu, Sci. Adv., 2021, 7, eabc8844 CrossRef CAS PubMed.
- Y. Liu, X. Zheng, Y. Fang, Y. Zhou, Z. Ni, X. Xiao, S. Chen and J. Huang, Nat. Commun., 2021, 12, 1686 CrossRef CAS PubMed.
- F. Wang, C. Ge, D. Duan, H. Lin, L. Li, P. Naumov and H. Hu, Small Struct., 2022, 3, 2200048 CrossRef CAS.
- Y. Wang, Y. Yang, N. Li, M. Hu, S. R. Raga, Y. Jiang, C. Wang, X.-L. Zhang, M. Lira-Cantu, F. Huang, Y.-B. Cheng and J. Lu, Adv. Funct. Mater., 2022, 32, 2204396 CrossRef CAS.
- S. Zhang, T. Xiao, F. Fadaei Tirani, R. Scopelliti, M. K. Nazeeruddin, D. Zhu, P. J. Dyson and Z. Fei, Inorg. Chem., 2022, 61, 5010–5016 CrossRef CAS PubMed.
- L. Chao, Y. Xia, X. Duan, Y. Wang, C. Ran, T. Niu, L. Gu, D. Li, J. Hu, X. Gao, J. Zhang and Y. Chen, Joule, 2022, 6, 2203–2217 CrossRef CAS.
- A. Mahapatra, V. Anilkumar, R. D. Chavan, P. Yadav and D. Prochowicz, ACS Photonics, 2023, 10, 1424–1433 CrossRef CAS.
- A. Mahapatra, N. Parikh, H. Kumari, M. K. Pandey, M. Kumar, D. Prochowicz, A. Kalam, M. M. Tavakoli and P. Yadav, J. Appl. Phys., 2020, 127, 185501 CrossRef CAS.
- Y. Liu, Y. Zhang, K. Zhao, Z. Yang, J. Feng, X. Zhang, K. Wang, L. Meng, H. Ye, M. Liu and S. (Frank) Liu, Adv. Mater., 2018, 30, 1707314 CrossRef PubMed.
- Y. Cho, H. R. Jung, Y. S. Kim, Y. Kim, J. Park, S. Yoon, Y. Lee, M. Cheon, S. Jeong and W. Jo, Nanoscale, 2021, 13, 8275–8282 RSC.
- S. Zhang, T. Xiao, F. Fadaei Tirani, R. Scopelliti, M. K. Nazeeruddin, D. Zhu, P. J. Dyson and Z. Fei, Inorg. Chem., 2022, 61, 5010–5016 CrossRef CAS PubMed.
- S. Amari, J.-M. Verilhac, E. Gros D’Aillon, A. Ibanez and J. Zaccaro, Cryst. Growth Des., 2020, 20, 1665–1672 CrossRef CAS.
- T. W. Jones, A. Osherov, M. Alsari, M. Sponseller, B. C. Duck, Y.-K. Jung, C. Settens, F. Niroui, R. Brenes, C. V. Stan, Y. Li, M. Abdi-Jalebi, N. Tamura, J. E. Macdonald, M. Burghammer, R. H. Friend, V. Bulović, A. Walsh, G. J. Wilson, S. Lilliu and S. D. Stranks, Energy Environ. Sci., 2019, 12, 596–606 RSC.
- A. Kalam, R. Runjhun, A. Mahapatra, M. M. Tavakoli, S. Trivedi, H. Tavakoli Dastjerdi, P. Kumar, J. Lewiński, M. Pandey, D. Prochowicz and P. Yadav, J. Phys. Chem. C, 2020, 124, 3496–3502 CrossRef CAS.
- V. M. Le Corre, E. A. Duijnstee, O. El Tambouli, J. M. Ball, H. J. Snaith, J. Lim and L. J. A. Koster, ACS Energy Lett., 2021, 6, 1087–1094 CrossRef CAS PubMed.
- B. Murali, E. Yengel, C. Yang, W. Peng, E. Alarousu, O. M. Bakr and O. F. Mohammed, ACS Energy Lett., 2017, 2, 846–856 CrossRef CAS.
- K. M. Boopathi, B. Martín-García, A. Ray, J. M. Pina, S. Marras, M. I. Saidaminov, F. Bonaccorso, F. Di Stasio, E. H. Sargent, L. Manna and A. L. Abdelhady, ACS Energy Lett., 2020, 5, 642–649 CrossRef CAS.
- J. Oh, W. Y. Jeong, S. Y. Lee, B. Lee and M.-Y. Ryu, Appl. Sci. Converg. Technol., 2020, 29, 19–22 CrossRef.
- H. Lu, H. Zhang, S. Yuan, J. Wang, Y. Zhan and L. Zheng, Phys. Chem. Chem. Phys., 2017, 19, 4516–4521 RSC.
- S. Meloni, T. Moehl, W. Tress, M. Franckevičius, M. Saliba, Y. H. Lee, P. Gao, M. K. Nazeeruddin, S. M. Zakeeruddin, U. Rothlisberger and M. Graetzel, Nat. Commun., 2016, 7, 10334 CrossRef CAS PubMed.
- A. Mahapatra, V. Anilkumar, J. Nawrocki, S. V. Pandey, R. D. Chavan, P. Yadav and D. Prochowicz, Adv. Elect. Mater., 2023, 9, 2300226 CrossRef CAS.
- S. Gavranovic, J. Pospisil, O. Zmeskal, V. Novak, P. Vanysek, K. Castkova, J. Cihlar and M. Weiter, ACS Appl. Mater. Interfaces, 2022, 14, 20159–20167 CrossRef CAS PubMed.
|
This journal is © The Royal Society of Chemistry 2024 |