DOI:
10.1039/D3SU00440F
(Critical Review)
RSC Sustain., 2024,
2, 1269-1288
Phytoremediation as a green and sustainable prospective method for heavy metal contamination: a review
Received
26th November 2023
, Accepted 15th April 2024
First published on 20th April 2024
Abstract
The presence of heavy metals in soil has significantly increased due to both natural processes and human activities, notably those associated with industrial activities. The nonbiodegradable nature of heavy metals gives rise to concerns. This tenacity can result in their integration into the food chain via agricultural plants and, ultimately, their buildup in the human body via biomagnification, resulting in detrimental impacts on both human well-being and the environment. Phytoremediation is a promising and environmentally conscious solution for reducing heavy metal pollution in soil. It possesses the capacity to serve as a cost-effective remedy for regenerating vegetation in polluted regions. In order to enhance the efficacy of phytoremediation, it is imperative to have a comprehensive understanding of the mechanisms governing the accumulation and persistence of heavy metals in plants. The mechanisms behind the absorption, translocation, and elimination of heavy metals in plants are highly significant. The current analysis will concentrate on the recent advancements in various processes, such as the use of microbes, genetic engineering, natural organic chemicals, and chelation. In addition, we emphasise the benefits, possible limitations, present condition, and future outlook of these procedures.
Sustainability spotlight
The problem of heavy metal (HM) contamination is a worldwide concern and poses serious health risks to humans. The buildup of HMs pollution in food chains via biomagnification can result in dangerous effects on plants, animals, and people (damage to the endocrine system, impact on immunity, neurological illnesses, and cancer). The pathways associated with the absorption, transportation, and elimination of HMs through plants are detailed in this extensive review. Cleaning up heavy metal-polluted soil through phytoremediation is an economical, aesthetically beautiful, and environmentally responsible alternative to chemical or physicochemical approaches for heavy metal decontamination. Several methods have been extensively analyzed to enhance the effectiveness of phytoremediation. These include microbe-assisted techniques, genetic engineering, the utilization of natural organic compounds, and chelation. The review highlights the benefits, potential limitations, present state, and future prospects.
|
1. Introduction
Heavy metals (HMs), a class of chemical substances with large average atomic weights, atomic numbers, and densities, pose significant threats to the natural world due to population growth and rapid industrialization. These heavy metals, including arsenic, cadmium, zinc, mercury, lead, chromium, copper, and nickel, are essential to living organism's physiological and biochemical functions throughout their life cycle. However, too much of these heavy metals can be harmful, polluting the environment, hindering biological and physiological functions, and decreasing crop yields.1–8
We must implement remediation procedures to reduce polluted soil and prevent heavy metals (HMs) from entering terrestrial, atmospheric, and marine habitats.9,10 Several methods have been explored for cleaning up heavy metals in soil, including precipitation, excavation, electro-remediation, chemical leaching, heat treatment, excavation and landfill, soil washing, and solidification.11–14 However, these methods have drawbacks such as their high cost, ineffectiveness when small amounts of pollutants are present, the development of subsequent pollutants, and permanent modifications in the physicochemical and biological characteristics of soils. Ekeoma et al.15 have recently provided a thorough examination of enzyme-based bioremediation methods that are both cost-efficient and successful in addressing emerging contaminants. The comprehensive review conducted by Nguyen et al.16 involved an examination of the utilisation of fly ash in the context of environmental remediation and the production of value-added commodities derived from fly ash. To address the pressing need to reclaim soil polluted with HMs, it is essential to create treatment solutions that are both affordable and kind to the environment. By addressing the underlying causes of HM pollution, we can work towards a more sustainable future for all living organisms.11–14,17 Berti and Cunningham's research on phytoremediation focuses on the use of plants to remove and eliminate elemental contaminants from soil.18 They argue that plants' roots can absorb ionic chemicals in small amounts, allowing them to maintain soil fertility. Plants can take in heavy metals and change how bioavailable they are by spreading their roots deeper into the soil and creating rhizosphere ecosystems.14,17,19 The utilisation of phytoremediation can yield numerous advantages, encompassing the following aspects:
(i) Financially viable: phytoremediation is an autotrophic technology driven by solar energy, making it easy to run with cheap costs for both initial setup and ongoing upkeep.
(ii) Ecologically sound: it lessens contaminant release into the natural world.
(iii) Applicability: it is possible to use it throughout a broad area, and it may be discarded in an uncomplicated manner.
(iv) By stabilizing heavy metals, it reduces erosion and metal leaching, lowering the possibility of toxins being transmitted and preserving the environment.
(v) As a result of the biological material that it releases into the soil, it helps enhance productivity.13,19,20
Various toxins and habitats undergo treatment using phytoremediation techniques such as phytodegradation, phytofiltration, phytoextraction, phytostabilization, and phytovolatilization.21,22 The characteristics of contaminants, soil limitations, and the types of plants used influence these techniques, which include in situ stabilization or degradation and the elimination of pollutants through volatilization or extraction (Table 1).23 Over the past few decades, research has focused on understanding the molecular processes responsible for heavy metal tolerance and developing strategies to enhance the phytoremediation process.
Table 1 Phytoremediation methods compared and contrasted with other technologies23
Technology name |
Action on pollutants |
Main type of pollutants |
Vegetation |
Phytostabilization |
Retained in situ |
Organics and metals |
Cover maintained |
Phytodegradation |
Attenuated in situ |
Organics |
Cover maintained |
Phytovolatilization |
Removed |
Organics and metals |
Cover maintained |
Phytoextraction |
Removed |
Metals |
Harvested repeatedly |
This comprehensive review provides a detailed description of the mechanisms underlying the absorption, transportation, and elimination of heavy metals in plants. The present review will centre on the latest advancements achieved in several processes, encompassing the active participation of microorganisms, genetic manipulation, utilisation of natural organic compounds, and chelation techniques. Furthermore, we highlight the advantages, potential constraints, current state, and future prospects of these techniques.
2. Heavy metals
In the most recent decade, heavy metals (HMs) have garnered a significant amount of concern as possible contaminants for the environment. The mining and processing of minerals from the earth, the burning of fossil fuels, and the discharge of waste from factories are the primary contributors to the presence of heavy metals in the environment. Arsenic (As), lead (Pb), mercury (Hg), cadmium (Cd), nickel (Ni), chromium (Cr), and aluminium (Al) are the most common heavy metals that contribute to the poisoning of soil ecological systems, affecting both vegetation and wildlife. Plants readily store the majority of these metals, which then enter the food chain and eventually reach people, causing serious health problems.2,24 Even though some heavy metals, including iron (Fe), copper (Cu), selenium (Se), and zinc (Zn), are essential in low amounts, the accumulation of these metals at greater levels can cause them to become extremely harmful to the environment.25,26Fig. 1 (ref. 27) depicts the diverse origins of major HMs in the environment, whereas Fig. 2 illustrates the adverse effects resulting from the presence of heavy metals in the natural surroundings.
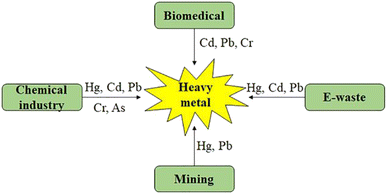 |
| Fig. 1 An illustrative graphic depicting the sources of heavy metal contamination.27 | |
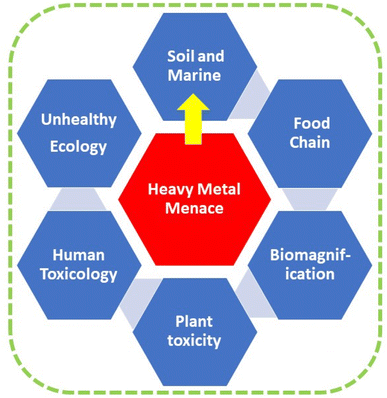 |
| Fig. 2 The recurring pattern of detrimental impacts caused by heavy metals in the environment. | |
The chemical composition of the metal, soil properties (including pH, clay, and organic matter concentration), and exchange reactions (precipitation and adsorption–desorption procedures) are what govern the quantity of HMs in the soil's solution.28,29 The building up of large concentrations of HMs can cause oxidative damage to chlorophyll and/or enzymes through the process of antagonism, which results in the exchange of vital ions. The most prominent indications of HMs poisoning are a slowing of plant development and a decrease in the amount of photosynthetic activity30 (see Fig. 3).
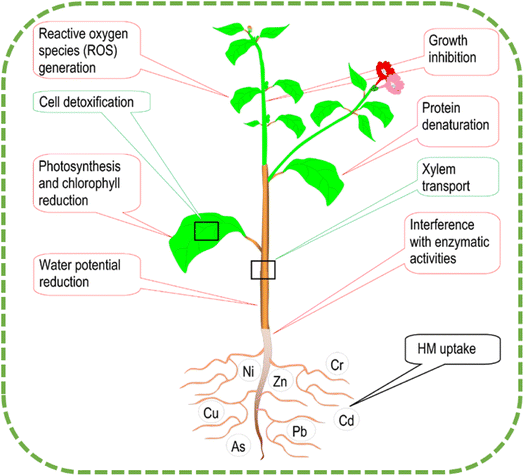 |
| Fig. 3 Plant's mechanisms for dealing with heavy metal toxicity (including absorption, translocation, and detoxification).21 | |
3. Absorption and transfer mechanisms of heavy metals in plants
The buildup of heavy metal in plants is the result of a range of operations, some of which are heavy metal mobilization, root absorption, xylem loading, root-to-shoot transfer, cellular compartmentation, and sequestration. Heavy metal mobilization is one of these mechanisms. The majority of the heavy metal in the soil is in an adherent state, which makes it unavailable for plant growth. Dalvi and Bhalerao31 say that plants can make nutrients more bioavailable by releasing different exudates from their roots. These exudates tend to change the pH of the rhizosphere and make it easier for heavy metals to dissolve. The outermost layers of the root absorb the bioavailable metal, which then passes through the cellular membrane and into the root cells. Both apoplastic and symplastic routes are primarily responsible for the absorption of heavy metals into the roots of plants. Passive diffusion is part of apoplastic routes, while active transfer over electrochemical potential differences and content across the plasma membrane is part of symplastic routes. According to Peer et al.'s research from 2005,32 the widespread absorption of heavy metals occurs through the symplastic route, and it is dependent on an energy mechanism that is facilitated by metal ion transporters or complexing mediators.
Heavy metal ions, once they have entered the root cells, have the potential to form complexes with a variety of chelators, including organic acids.33 These newly generated complexes, which include carbonate, sulphate, and phosphate precipitate, are subsequently immobilized in either the outside of the cell area (apoplastic cellular walls) or the intracellular space (symplastic sections, like vacuoles). The metal ions that are stored within the vacuoles might potentially move towards the stele, where they are absorbed by the xylem stream in the root symplasm.34 The xylem vessels then transport the metal ions to the shoots. The apoplast or symplast, which stores the ions in extracellular sections (cell walls) or the plant vacuole, allows them to pass through and disseminate in the leaves, preventing the accumulation of free metal ions in the cytosol.35
In summary, this section explores the subject of heavy metal absorption and transfer mechanisms in plants, emphasising the distinct roles played by various plant components.
4. Hyper-accumulator species
“Hyper-accumulator species” are plants that can absorb and store high levels of certain metals and minerals in their tissues without experiencing negative effects. According to research, some plant species have the ability to accumulate hazardous heavy metals in amounts that surpass the levels found in the soil.36 According to research, plants that develop in contaminated soils have developed various methods to deal with the harmful effects of heavy metals. These strategies include avoiding the accumulation of HMs, the removal of the metals, and the discharge of the metals from the plant tissues.37 The development of hyperaccumulator plants under excessive amounts of HMs is attributed to various biochemical processes. These processes help to keep the amount of metal in the cytoplasm below that in the soil, which prevents the harmful impact of heavy metals on cytoplasmic organelles. This is achieved through vacuolar categorization. Plant species that lack a removal method are able to absorb and transport significant amounts of HMs and store them in their shoots without exhibiting any signs of toxic effects.36
Sequestration or compartmentalization of metals in cell divisions (particularly vacuoles) away from the cytosol achieves heavy metal tolerance, protecting areas of vulnerability from HMs and preventing the inhibition of metabolic processes in the cytoplasm. In contaminated areas, organic solutes and amino acids like proline help plants flourish. Metal–solute complexation reduces HM transport to vulnerable plant tissues.38 In order to maintain normal development and metabolic processes despite exposure to HMs, plants have evolved different detoxification systems. Plants use two main tactics:21 limiting the intake of HMs and aggregation with tolerance process activities to shield their organs from poisonous HMs. The first approach restricts metal precipitation to lower HM concentrations. However, the second approach isolates the poisonous HMs from the rest of the cell, particularly in the vacuoles39 (Fig. 4). Rhizospheric microbes, including arbuscular mycorrhizal fungi (AMF) and bacteria, are linked to HMs uptake limitation. By producing metal–chelating substances like citric acid, oxalic acid, and phenolic compounds, these microbes can reduce HM absorption by plant cells.40 Vacuolar compartmentalization serves as another technique to control HM distribution and prevent its detrimental effects on cells.41 Numerous plants create complexes with phytochelatins (PCs) to deal with HMs, and then carry the resulting complex metal peptides into the vacuole.42
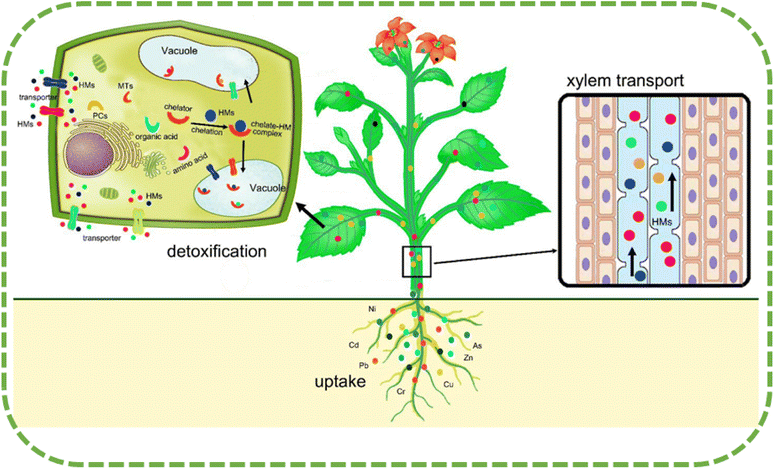 |
| Fig. 4 A schematic illustrating the absorption, transfer, and subsequent sequestration of heavy metals in plants.22 | |
Based on the metal concentrations in their tissues,21 we can classify different kinds of plants into three categories: (i) HM aggregator species, (ii) HM species that indicate, and (iii) HM excluder species. The accumulating species are plants that can take in HM concentrations (in their shoots or roots) that far surpass those found in the soil. The indicator species accumulates the HMs to a greater extent than the soil concentration. The HMs can't easily enter the roots or move from the roots to the shoots in the excluder species.37 Tolerant species are those that can reproduce in soil contaminated with heavy metals at concentrations that would kill non-tolerant species. Species that act as indicators or hyper-accumulators are tolerant; however, tolerant species are not required to act in any of these roles.
According to scientific literature, plants that are appropriate for phytoremediation possess four key characteristics. These include:21 (i) a fast growth rate and significant biomass; (ii) a deep and extensive root system; (iii) ease of harvesting; and (iv) the ability to accumulate significant quantities of heavy metals in their shoots. The prevalence of hyperaccumulator plants in polluted soil suggests that hyper-accumulation is a significant eco-physiological behavior for tolerance to heavy metals and serves as a measure of toxin adaptation. These types of plants are typically uncommon in nature. Certain plants known as metal hyperaccumulators have the ability to accumulate extremely high levels of heavy metals, exceeding 1000 parts per million. Non-accumulator or sensitive plants do not accumulate these metals at a rate higher than 10 ppm, which is in contrast to accumulator plants. According to a source,38 hyperaccumulator plants have the ability to absorb significant quantities of HMs in their above-ground structures throughout their regular growth and reproductive processes. In their study, Baker and Brooks43 established the threshold amounts for metals that are hyper-accumulated in plants. The amounts were found to be Cd = 100 ppm dry weight (DW), Ni, Cu, Co, Pb = 1000 ppm DW, and Zn, Mn = 10
000 ppm DW, as presented in Table 2.
Table 2 The limit for classifying certain plant species as hyper-accumulators43
S. No. |
Trace elements |
Threshold for hyper-accumulator plants (ppm DW) |
1 |
Mercury (Hg) |
1000 |
2 |
Selenium (Se) |
1000 |
3 |
Cadmium (Cd) |
100 |
4 |
Copper (Cu) |
1000 |
5 |
Cobalt (Co) |
1000 |
6 |
Nickel (Ni) |
1000 |
7 |
Chromium (Cr) |
1000 |
8 |
Lead (Pb) |
1000 |
9 |
Zinc (Zn) |
10 000 |
10 |
Manganese (Mg) |
10 000 |
The amounts of accumulation observed were significantly greater than those detected in non-accumulator species. Over 500 species have been identified as hyperaccumulators, with the majority of them belonging to families such as Brassicaceae, Asteraceae, Amaranthaceae, Cyperaceae, Fabaceae, Lamiaceae, Poaceae, and Euphorbiaceae.21 This information suggests that there is a diverse range of plant species that have the ability to hyperaccumulate. The genus Brassicaceae is known to have a significant number of hyperaccumulator plants.
This section summarises the role of hyperaccumulator plants in detoxifying HMs. Hyperaccumulator plants can handle HMs by storing them in cell divisions, keeping weak spots safe from HMs, and stopping metabolism processes in the cytoplasm. The section categorizes plants into HM aggregator species, indicator species, and excluder species, highlighting their specific functions in detoxifying HMs and their potential impact on plant cells.
5. Methods of detoxification
According to Thakur et al.,34 one of the most important prerequisites for the application of phytoremediation is the completion of HM detoxification. Overall, there are essentially two types of protective mechanisms that plants utilize to deal with the toxicity of HMs. These mechanisms are known as avoidance and tolerance. Plants are able to keep the cellular levels of HMs below the limits that indicate hazards because of two distinct processes.
5.1. Avoidance
The concept of avoidance tactics for plants pertains to their capacity to regulate the absorption of HMs and confine their translocation into plant tissues via root cells.31 This mechanism is effective because it can act as the main defence against threats from the outside by using different methods, such as root sorption, metal ion precipitation, and metal exclusion.31 Plants initially try to immobilize HMs through either root sorption or modification of metal ions when exposed to them. Root exudates, including organic acids and amino acids, have the ability to bind with HMs and create complexes with stability in the rhizosphere. Dalvi and Bhalerao31 reported this information. According to them, certain root exudates have a tendency to alter the pH of the rhizosphere. This alteration can result in the precipitation of HMs, ultimately reducing their bioavailability and toxic effects. The plant has a metal exclusion process that creates constraints between the root and shoot systems. These hurdles inhibit the movement of HMs from the soil to only the roots. The absorption and transportation of HMs from the roots to the shoots are limited to safeguarding the uppermost layers of the plant from adverse consequences.
Arbuscular mycorrhizas can stop HMs from getting into the root in different ways, such as by absorbing, adsorbing, or chelating HMs in the rhizosphere. This makes them effective in preventing HM intake and acting as an exclusion obstacle. One procedure for HM avoidance is the integration of HMs in plant cell walls, as noted by Memon and Schröder.44 Polygalacturonic acids, which contain carboxylic groups, compose the cell wall pectins. These groups have a negative charge and can effectively bind HMs. The cell wall functions as a cation exchanger by limiting the access of unbound HM ions to the cells.
5.2. Tolerance
Plants employ a tolerance method to manage the harmful effects of accumulated HM ions once they enter the cytosol. According to Dalvi and Bhalerao,31 there are several strategies involved in the subsequent phase of defence at the intracellular level against HM ions. These pathways include inactivation, chelation, and compartmentalization.
According to Manara,45 plants undergo a detoxification process to minimize the harmful consequences of excessive HM ions that develop in the cytosol. Chelation, which involves the use of ligands to create complexes with these ions, is the main method for removing HM ions. The process of chelation effectively reduces the quantity of metal ions unbound to other molecules. The cytoplasm contains both organic and inorganic ligands that facilitate HM chelation. The process of HM ion chelation involves various organic compounds such as organic acids, amino acids, phytochelatins (PCs), metallothioneins (MTs), cell wall proteins, pectins, and polyphenols.46,47 The presence of organic acids in cells has a tendency to prevent HMs from remaining as free ions in the cytoplasm. The process of complexing and reducing their bioavailability to plants achieves this. Sun et al.48 did research that showed that citrate binds Ni in the leaves of T. goesingense and that acetic and citric acids bind Cd in the leaves of Solanum nigrum. The malate contributes to the chelation of Zn in A. halleri. The accumulation of specific types of amino acids is a result of heavy metal stress. Cd can induce the generation of cysteine in Arabidopsis thaliana, as observed in a study by Domínguez-Solís et al.49 Ni hyperaccumulation can also induce the accumulation of histidine. Exposure to Cd, Pb, Zn, and Cu stress can result in a significant increase in the accumulation of proline. Amino acids have the ability to chelate heavy metal ions present in cells and xylem sap, thereby facilitating the detoxification of heavy metals. High levels of heavy metals can activate PCs and MTs. The text discusses the chelation of Cd by PCs in tobacco leaves and the role of MTs in mediating the response to Cu stress in Silene vulgaris. The PCs of tobaco leaves have the ability to chelate Cd. Conversely, a link exists between higher levels of the MT gene and improved Cu acceptability in Silene vulgaris, suggesting the role of MTs in mediating the response to Cu stress.
According to Tong et al.,35 chelation transports ligand–HM complexes from the cytosol to passive compartments, including vacuoles. This process effectively stores the complexes without triggering any harmful effects. According to Sheoran et al.,50 the procedures of sequestration and vacuolar compartmentalization can effectively safeguard against the harmful impacts of HMs. Eliminating dangerous HM ions from the cell's vulnerable regions, where cell division and respiration occur, achieves this. This reduces the relationships between HM ions and cell metabolism processes, thereby minimizing the risk of harm to cell functions. Fig. 4 depicts the process of HM absorption, transfer, and detoxification in plants.
Robinson et al.51 and Eapen and D'souza52 report that plants can store and separate HM ions into various parts such as leaf petioles, leaf sheathes, trichomes, and vacuoles. This procedure helps to lessen the damage that HMs cause to the plant. According to Thakur et al.,34 natural leaf shedding can facilitate the removal of heavy metals from the plant body by translocating them to older leaves. Plantago lanceolata only transports Zn to its leaves in the final week before leaf shedding. As a result, the plant sheds its leaves and eliminates the Zn.
If there are too many HMs in the environment and the above methods aren't enough to protect cells from their harmful effects, an excessive buildup of metal ions in the cytoplasm can cause reactive oxygen species (ROS) to be produced. The excessive generation of ROS can lead to oxidative stress, which can disturb cell homeostasis, prevent cellular operations, trigger DNA destruction, and lead to protein oxidation. Researchers such as Huang et al.53 and DalCorso et al.14 have noted this. When exposed to heavy metals, plant cells respond by activating their ROS-scavenging tools. This is done by making antioxidant enzymes like superoxide dismutase (SOD), catalase (CAT), peroxidase (POD), and glutathione reductase (GR) work, as well as antioxidant substances that aren't enzymes, like ascorbate, tocopherols, flavonoids, and carotenoids.14,54,55 The role of the anti-oxidative defence mechanism in plants is significant when it comes to responding to heavy metal stress.
In brief, the completion of HM detoxification is a crucial prerequisite for the implementation of phytoremediation. Plants employ two primary defensive mechanisms to mitigate the toxicity of HMs. The terms used to describe these systems are avoidance and tolerance. We thoroughly examine the operational mechanics of these two defensive systems.
6. Working mechanism of phytoremediation
Certain plants possess the ability to accumulate HMs such as Ag, Cd, Hg, and Pb, which do not serve any known biological purpose, in addition to essential elements like Cu, Fe, Mg, Mo, Mn, Se, and Zn that are crucial for plant growth.21 The excessive buildup of HMs has the potential to be harmful to the majority of plant life due to their toxic nature. We utilize five categories of phytoremediation methods to clean up polluted soils.21,22 These methods include phytoextraction, which involves plants absorbing pollutants through their roots and storing them in their tissues; phytodegradation/phytotransformation, which involves plants breaking down pollutants into less harmful substances; phytovolatilization, which involves plants releasing pollutants into the air through their leaves; phytofiltration, which involves different parts of plants such as roots, shoots, or seedlings to eliminate contaminants from polluted surface waters or waste waters; and phytostabilization, which involves plants immobilizing pollutants in the soil to prevent them from spreading.
6.1. Phytoextraction
Phytoextraction is a technique that involves the cultivation of plant species that have the ability to accumulate high levels of contaminants, specifically exceeding 0.1% of dry weight (DW), in their shoots. One possible approach for selecting these plants involves considering the extent of pollution transport from the roots to the shoot. We can categorize this strategy into two distinct methods: continuous phytoextraction and induced phytoextraction. The process of continuous phytoextraction involves the utilization of native plants that possess inherent qualities to gather significant amounts of HMs, also known as hyper-accumulators. The process of induced phytoextraction involves the use of chemical substances, such as chelates, to enhance the accumulation of metals in plants. Researchers have found that this method effectively increases the extraction of metal from the soil. Plants that possess a greater amount of biomass and deeper root systems are known to improve phytoextraction. According to the findings of Fourati et al.,56Sesuvium portulacastrum showed a higher accumulation of Ni in its aboveground part, with a concentration of 1050 μg g−1 DW.
Jacobs et al.57 found that the amount of Zn in the leaves of Noccaea caerulescensis was over 300 g Cd ha−1 after 2 months of growing in the field before the plants were moved. In their study, Ghazaryan et al.58 compared the effectiveness of Melilotus officinalis and Amaranthus retroflexus at cleaning up Cu and Mo-polluted soils. According to the results, A. retroflexus tends to accumulate Cu and Mo in its shoot, whereas M. officinalis tends to store Zn in its roots. In a recent study by Yang et al.,59 the authors investigated the absorption of Cd and Zn in three different kinds of Napier grass (Pennisetum purpureum) under field conditions. The results showed that P. purpureum cv. Guiminyin had the highest accumulation of Cd (197.5 g ha−1) and Zn (5023.9 g ha−1) in their shoots. Khalid et al.60 evaluated Alternanthera bettzickiana's phytoextraction ability towards Ni and Cu through pot experiments. The results showed that after 8 weeks of treatment, this plant species had accumulated twice as much Cu in its shoots in comparison with the control group of plants.
6.2. Phytodegradation or phytotransformation
Phytodegradation, also referred to as phytotransformation, is the breakdown of pollutants that plants absorb through the process of metabolism. It can also mean that enzymes made by the roots, like dehalogenases, nitroreductases, and peroxidases, break down contaminants outside of the plant. A study reveals that genetically modified yellow poplar (Liriodendron tulipifera) can thrive in tissue culture, despite exposure to elevated mercury levels. Additionally, this altered plant has the ability to convert the extremely harmful mercury Hg2+ into a much less harmful form, Hg0. The procedure described enables plants to convert harmful substances into harmless ones. According to Das et al.,61Vetiveria zizanioides plants have the ability to remove up to 97% of trinitrotoluene (TNT) from the soil. Hannink et al.62 reported that Nicotiana tabacum facilitated the breakdown of TNT by producing the NfsI nitroreductase enzyme through its roots. According to Just and Schnoor's research,63 it was found that Populus deltoids plants have the ability to convert hexahydro-1,3,5-trinitro-1,3,5-triazine (RDX) into metabolic elements.
Certain plants have the ability to create optimal conditions for the growth and interaction of bacteria and mycorrhizae, which can aid in the breakdown of harmful toxins. The deterioration process entails either the volatilization of its constituents or their incorporation into the soil matrix.64 The generation of sugars and organic acids by plants can lead to a rise in the populations of fungi and bacteria.65 Favourable soil features like soil aeration and moisture level can improve the process of rhizodegradation.66 Papadopoulos and Zalidis67 conducted a study to address the issue of wetland contamination caused by terbuthylazine (TER). Their findings revealed that the rhizomes of Typha latifolia showed potential for TER phytodegradation. Sampaio et al.68 observed the degradation of polycyclic aromatic hydrocarbons (PAHs) in polluted sediments when they linked Rhizophora mangle mangrove with rhizobacteria that promote plant growth (Pseudomonas aeruginosa and Bacillus sp).
6.3. Phytovolatilization
Phytovolatilization is a technique in phytoremediation that involves using plants to absorb contaminants from the soil. The plants then convert these harmful substances into less hazardous volatile forms, releasing them into the surrounding environment through the leaf or foliage mechanism of transpiration. According to Mahar et al.,69 this method has the potential to facilitate the removal of organic contaminants and certain HMs such as Se, Hg, and As through detoxification. The Brassicaceae family, specifically Brassica juncea, effectively volatilizes Se. Inorganic Se is first absorbed into the organic amino acids selenocysteine (SeCys) and selenomethionine (SeMet). SeMet is changed into dimethylselenide (DMSe) through biomethylation. DMSe is less dangerous than inorganic Se because it is volatile.70,71 Mercury (Hg) exists in a liquid state at room temperature in its elemental form and has a high tendency to vaporise readily. Marques et al.72 state that the highly reactive nature of mercury leads to its primary existence as a divalent cation, Hg2+, upon release into the environment. Either the roots or leaves absorb methylmercury and transform it into ionic mercury. Bizily et al.73 report that the substance transforms into a less hazardous elemental form and then releases as a gas into the atmosphere.
The phytovolatilization method of phytoremediation has a clear advantage over other methods because it gets rid of HMs (metalloid) pollutants from the affected area and spreads them as gases. This process does not require plant harvesting and removal. Phytovolatilization, while a remedial approach, does not result in the complete elimination of contaminants from the surroundings. The contaminants remain present in the environment. The process merely moves harmful substances from the soil to the atmosphere, resulting in the release of hazardous, volatile substances that can pollute the surrounding air. Precipitation may also re-deposit them into the soil.74 It is necessary to conduct a hazard evaluation prior to implementing it in the field.
6.4. Phytofiltration
Phytofiltration is a method that involves the use of various plant components, including roots, shoots, and seedlings, to remove contaminants from polluted surface waters or waste waters.75 Rhizofiltration involves the use of roots, caulofiltration involves the use of shoots, and blastofiltration involves the use of seedlings. Rhizofiltration removes heavy metals from contaminated water through two mechanisms: adsorption onto the root surface or absorption by the roots. According to Javed et al.,76 root exudates can change the pH of the rhizosphere, which can cause the deposition of heavy metals on plant roots. This process can help reduce the migration of heavy metals into underground water.
Rhizofiltration involves the hydroponic cultivation of plants in clean water in order to promote the growth of a robust root network. After developing a substantial root structure, we expose the plants to contaminated water to facilitate their acclimation. The process involves transferring the plants to the polluted location subsequent to acclimation, with the aim of eliminating HMs. Wuana and Okieimen77 state that they harvest and dispose of the roots once they become saturated. Rhizofiltration requires plants with a large root system, high biomass production, and the ability to handle heavy metals (HMs). Both terrestrial and aquatic plants can achieve rhizofiltration. We frequently utilize aquatic plants like hyacinth, azolla, duckweed, cattail, and poplar for the treatment of wetland water. This is because of their substantial accumulation of HMs, excessive tolerance, rapid growth, and substantial biomass creation.78 Terrestrial plants like Indian mustard (B. juncea) and sunflower (H. annuus) have deeper and hairier root structures in contrast with aquatic plants. Researchers have observed that certain plants can effectively accumulate heavy metals during rhizofiltration.79–81
6.5. Phytostabilization
This strategy involves the storage of contaminants by plant roots or their precipitation through root exudates. The described technique reduces transportation contaminants and prevents their migration to ground Nedjimi and Daoud38 conducted a study that suggests Atriplex halimus, a halophyte native to North Africa and a member of the Amaranthaceae family, could be a viable option for phytostabilization of Cd. According to the authors, the species in question exhibited a notable disparity in Cd accumulation between its roots and shoots. Specifically, the roots collected significantly higher amounts of Cd (606.51 ppm DW) in comparison to the shoots (217.52 ppm DW). The key traits that make plants appropriate for phytostabilization are their morphology and the depth of their roots. Multiple fine root network extensions effectively utilized the soil matrix, several factors, including the type of plant, the amount of moisture present, the soil texture, the presence of calcareous encrustations, dry conditions, and any soil modifications, can influence the depth of a plant's roots.
Certain plant species have the ability to alter the solubility and/or mobility of metals by releasing root exudates. Observations show that certain species can accumulate significant quantities of heavy metals in their root systems. Al Chami et al.82 conducted a study through pot experiments to investigate the efficacy of Sorghum bicolor and Carthamus tinctorius in remedying HM contamination of Ni, Pb, and Zn. The results showed that both species were capable of absorbing these HMs. Researchers found a higher concentration of metals in the roots compared to the leaves. Researchers have found that Erica australis can absorb copper, cadmium, and lead through its roots without experiencing any harm.83 This suggests that it could be a suitable species for phytostabilization purposes. Bacchetta et al.84 say that Helichrysum microphyllum is a good plant for phytostabilization because it mostly stops Zn, Cd, and Pb from entering its root tissue.
Fig. 5 depicts the summary of HM phytoremediation, which involves numerous stages. Table 3 showcases a selection of plants recognized for their proficiency in utilizing phytoremediation mechanisms. The selection of these plants is based on their capacity to remediate contaminated environments. Scientists have conducted extensive research on these particular species.
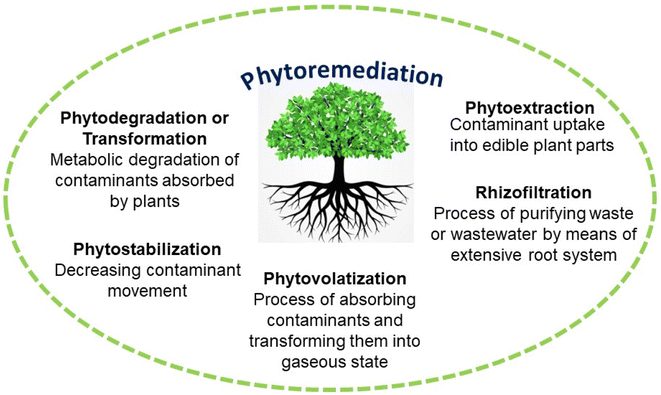 |
| Fig. 5 Heavy metal phytoremediation involves multiple processes. | |
Table 3 The following is a list of some plants that are recognized for their ability to employ phytoremediation mechanisms85
Phytoremediation technique |
Species scientific name |
Contaminants |
Remarks |
Ref. |
Phytoextraction |
Sesbania drummondii
|
Pb |
EDTA enhanced uptake of Pb and accumulation |
86
|
Phytoextraction |
Pteris vittate
|
As |
126-fold arsenic in the shoots vs. soil, without the need of chelating agents or other soil amendments |
87
|
Phytoextraction |
Brassica juncea
|
Pb |
Chelating agents like EDTA is applied to soil to mobilize Pb and facilitate extraction |
87
|
Phytoextraction |
Lactuca sativa
|
Ni, Co, and Fe |
Higher absorption capacity and lower intrinsic velocity |
88
|
Phytoextraction |
Nicotiana tabacum
|
Cd |
Cd higher in stems and leaves |
89
|
Phytoextraction |
Mesembryanthemum criniforum
|
Pb |
Bacteria tolerant to Pb used |
90
|
Phytoextraction |
Zea mays
|
Pb, Ti |
Chelators induced the phytoextraction of Pb and Ti |
91
|
Phytoextraction |
Xanthium strumarium
|
Cd, Pb, Ni, Zn |
Has highest BCF for Ni-1.651, Cd-1.574 and Pb-1.048 |
92
|
Phytodegradation |
Spirodela polyrhiza
|
Ofloxacin (OFX) |
(93.73–98.36%) reduction in OFX |
93
|
Phytodegradation |
Pontederia crassipes
|
Sodium dodecyl sulfate (SDS) |
Removal efficiency as well as degradation of SDS were enhanced by ascorbate peroxidase (APX) activity in the presence of Chromolaenaodorata L. extract |
94
|
Phytodegradation |
Phragmites australis
|
Ibuprofen |
P. australis could be a good plant in construction on wetland |
95
|
Phytodegradation |
Ipomoea carnea
|
Textile azo dyes |
Induction of hairy roots in explants of in vitro grown seedlings of Ipomoea carnea J. with Agrobacterium rhizogenesfavoured phytodegradation |
96
|
Phytodegradation |
Chlorella
|
Pentachlorophenol (PCP) |
Algae activity may have been reduced by the cycling of light exposure |
97
|
Phytodegradation |
Blumea malcolmii
|
Malachite green |
Phytodegradation of industrial waste |
98
|
Phytovolatilization |
Polypogonmon speliensis
|
As |
Dimethylchloroarsine (AsCl(CH3)2) and pentamethylarsine (As(CH3)5) is volatilized. More toxic form of As |
99
|
Phytovolatilization |
Juncus effuses
|
Ammonium |
Methane is also released |
100
|
Phytovolatilization |
Phragmites australis
|
Organochlorines (OCs) |
γ-hexachlorocyclohexane (γHCH) is volatilized. Solubility and volatility of the OCs affects translocation from roots to shoots |
101
|
Phytovolatilization |
Scirpus robustus
|
Se |
Wetlands plants |
102
|
Rhizofiltration |
Eichhornia crassipes
|
Fe, Cr, Cu, Cd, Zn, Ni, As |
Translocation factor: Fe: 0.69, Cr: 0.56, Cu: 0.79, Cd: 0.78, Zn: 0.67, Ni: 0.55, As: 0.45; BCF: 0.002346 |
103
|
Rhizofiltration |
Azolla caroliniana
|
As |
BCF: 0.000397 |
104
|
Rhizofiltration |
Callitriche lusitanica
|
As |
BCF: 0.002346 |
104
|
Rhizofiltration |
Callitriche stagnalis
|
U |
BCF: 0.00194841 |
105
|
Rhizofiltration |
Typha angustifolia
|
Cu, Pb, Ni, Fe, Mn, and Zn |
Industrial waste containing phenol (100–800 mg L−1) and melanoidin (2500–8500 Co–Pt) |
106
|
Rhizofiltration |
Fontinalis antipyretica
|
U |
BCF: 0.00023479 |
105
|
Rhizofiltration |
Lemna minor
|
U |
BCF: 0.0000529 |
105
|
Rhizofiltration |
Ranunculus trichophyllus
|
As |
BCF: 0.000354 |
104
|
Phytostabilization |
Eupatorium cannabinum
|
As |
Citric acid (CA) addition 20 mg L−1 addition favored phytostabilization |
107
|
Phytostabilization |
Kosteletzkya pentacarpos
|
Cd, Zn |
Salinity favors plants against metal toxicity. Cytokinin promotes Zn resistance |
108
|
Phytostabilization |
Salix sps
|
Cd |
Non-flooding Salix has higher BCF than flooding species |
109
|
Phytostabilization |
Helianthus annuus
|
Cu, Zn, Pb, Hg, As, Cd |
Vermicompost as a supplement, usually for low level of metal contaminants |
110
|
Phytostabilization |
Solanum nigrum
|
Cu, Zn, Cd |
10% biochar/attapulgite addition recommended |
111
|
In summary, phytoremediation working mechanisms involve phytoextraction, phytodegradation/phytotransformation, phytovolatilization, phytofiltration, and phytostabilization. These methods work by absorbing pollutants through the roots, breaking them down into less harmful substances, and immobilizing pollutants in the soil to prevent their spread.
7. Maximization of phytoremediation mechanism
The ability of plants to eliminate organic and inorganic pollutants from soil and water is dependent on various factors, such as the type of plant species and its surroundings. Studies by Anton and Mathe-Gaspar112 and Antoniadis et al.113 have highlighted this. Dary et al.114 assert that plant's interactions with their environment, which includes soil, water, air, and microorganisms, significantly influence the removal of pollutants. Various factors, including the type of pollutant present, the specific plant species utilized, and the characteristics of the treated soil, determine the success of treatment techniques. Various factors, including soil pH, electric conductivity, organic matter contents, microbial activities, and other nutrients in the soil, influence the efficacy of remediation.85 Plant biomass and metabolism are crucial in the course of this procedure. Anton and Mathe-Gaspar112 and Nissim et al.115 have highlighted the significance of these factors.
The effectiveness of plants in removing pollutants from the environment is often assessed through the calculation of two factors: bioconcentration factor (BCF) and translocation factor (TF). BCF is determined by comparing the amount of pollutants in the plant parts to that in the surrounding environment. TF, on the other hand, measures the accumulation of elements in the plant's shoot relative to its root. This method of evaluation was presented by Wu et al.116
8. Enhancing the efficiency of plants: phytoremediation perspective
To reduce the impact of the constraints, a number of techniques have been established. These approaches involve changing and increasing specific characteristics of the plants in order to increase their capacity for phytoremediation. The phytoremediation potential does have a few limits, such as sluggish growth, which prevents swift and substantial utilization of the plants;117 and adaptability to an array of environmental circumstances, such as soils lacking in nutrients.118 However, these constraints do not prevent phytoremediation from being a viable option for cleaning up polluted environments. The employment of certain bacteria, genetic engineering, the application of natural organic chemicals, and chelating agents are some of the tactics that are being implemented in order to circumvent these constraints. Table 4 provides an overview of various phytoremediation support processes and their corresponding boosting impacts on the process of phytoremediation.
Table 4 Helpful phytoremediation tools, involved processes, and their boosting impact85
Phytoremediation support processes |
Boosting effects on phytoremediation |
1. Microbial augmentation (a) plant growth promoting rhizobacteria (PGPR) |
Raises metal bioavailability and absorption in addition to organic acid and enzyme synthesis, boost plant growth, root hair formation, and metal tolerance, lower generation of ethylene and increased bacterial auxin generation |
(b) Plant growth promoting endophytic bacteria (PGPE) |
(c) Arbuscular mycorrhizal fungi (AMF) |
Boosts nutrient, water, and metal absorption by increasing root absorptive surface area and facilitating rhizosphere oxidative enzymes and early ring cleavage of aromatic hydrocarbons |
2. Genetic engineering (gene transfer, manipulation and cloning) |
Increases plant biomass and tolerance to metals, as well as the oxidative stress pathway, overexpressing genes involved in metal transport and absorption |
3. Natural organic support (a) sugar beet residue (SBR) |
Improves metal bioavailability, phytoextraction, and microbial biomass and performance |
(b) Composted sewage sludge |
Phytoextraction, metal bioavailability enhancement |
(c) Paper waste |
Boosts plant growth and fixes soil concerns |
(d) Biochar |
Boosts plant biomass, promotes improved nutrient cycling, boosts water and nutrient retention, and decreases metal solubility |
4. Chemical support through chelation (a) ethylenediaminetetraacetic acid (EDTA) |
Complexation of metals with chemicals (acting as ligands), which increases metal absorption |
(b) Ethylene glycol tetra acetic acid (EGTA) |
(c) Sodium dodecyl sulfate (SDS) |
8.1. Boosting plant efficiency using microorganisms
Microorganisms are able to exert their impact on the phytoremediation operation in a variety of ways. Through biostimulation and bioaugmentation, it is possible to get microbes to break down organic contaminants and help microbes that depend on HMs take them in. According to Goswami et al.,119 biostimulation is the act of encouraging indigenous microorganisms in contaminated soil to digest hazardous contaminants. We accomplish this by adding nutrients like nitrogen, phosphorus, and electron donors to the soil. According to Goswami et al.,119 bioaugmentation is the technique of adding naturally occurring or genetically engineered microbes to damaged soil in order to facilitate the removal of hazardous contaminants. According to Lacalle et al.'s research,120 the most successful method for transforming hexavalent chromium into trivalent chromium and lindane into a less harmful form was determined to be the inclusion of organic substances, which was then followed by bioaugmentation with an actinobacteria consortium mixed with Brassica napus. Monti et al.121 say that bioaugmentation with Pseudomonas fluorescens could break down 2,4-dinitrotoluene. This meant that it had less of an effect on the growth of Arabidopsis thaliana. According to the findings of another study,122 biostimulation with nitrogen and phosphorus had a significant impact on the breakdown of isoproturon.
Researchers discovered that using PGPR (Plant Growth Promoting Rhizobacteria) and PGPE (Plant Growth Promoting Endophytic Bacteria) could potentially increase metal solubility by secreting organic anions and protons.123,124 PGPR can improve the effectiveness of phytoremediation by increasing plant growth and biomass, making plants more resistant to pathogens and metals, improving their ability to absorb nutrients, and moving heavy metals by creating organic acids, enzymes, antibiotics, phytohormones, and siderophores.125 The increased creation of organic acids, enzymes, antibiotics, phytohormones, and siderophores is responsible for these benefits. Braud et al.126 reported an improvement in the bioavailability and absorption of lead and cadmium in maize plants infected with microbes that produce siderophores. Chang et al.127 say that PGPR's ability to make ACC deaminase may lead to lower levels of ethylene synthesis, which in turn helps the plant grow. Bacillus,128Pseudomonas,129 and Serratia130 are responsible for enhanced plant biomass and enhanced phytoremediation through the release of ACC deaminase. Another thing that PGPR can do is release bacterial auxin, which helps plants grow and, in turn, improves phytoremediation.14,131 This makes root hairs grow and lateral roots sprout. We do this to enhance phytoremediation. According to Rane et al.,132 interactions between plants and microbes in the rhizosphere can promote phytoremediation of both organic and inorganic contaminants in a synergistic manner.
Fungi are useful in phytoremediation because they can interact with pollutants in many ways. Mycoremediation provides a benefit over bacterial remediation because fungi may grow at the interface between air and water.133 Mycorrhizal fungi change the properties of the soil and the chemical make-up of plant root exudates, which changes how metals are taken up by plants.117,134 To be more specific, arbuscular mycorrhizal fungi (AMF) boost the capacity of plants to absorb nutrients and water, as well as enhance the bioavailability of metals.135 Additionally, AMF's ability to generate phytohormones can stimulate plant development and ease phytoremediation.136 Red clover (Trifolium pratense L.) yields increased when inoculated with AMF in Zn-contaminated soil, according to research by Chen et al.137 They also found that AMF took Zn from the soil and carried it to the roots, leading to a greater buildup of Zn in plant roots. Mycorrhizal fungi play a function in the breakdown of organic pollutants in soil, in addition to their role in HM remediation. Some studies have shown that ectomycorrhizal fungi (ECM) can break down organic pollutants like 2,4-dichlorophenol,138 2,4,6-trinitrotoluene,139 atrazine,140 polychlorinated biphenyls,141 and polycyclic aromatic hydrocarbons.142 Mycorrhizal fungi have been shown to influence oxidative enzyme activity in the root and rhizosphere, which may explain the reported effects.143 Criquet et al.144 and oxidative enzymes help polycyclic aromatic hydrocarbons break up their rings early on.
8.2. Genetic engineering
Researchers have demonstrated the potential strategy of using genetic engineering to enhance plants' phytoremediation capacities in response to heavy metal contamination. By inserting a gene from another creature, such as another plant, microbes, or animals, into the plant's genome, we can genetically modify plants. Plants acquire the foreign gene and its characteristics as a result of DNA exchange. Genetic engineering has the potential to speed up the modification of plants with favourable features for phytoremediation in comparison to conventional breeding methods. Conventional breeding strategies, including crossing, are unable to transmit beneficial genes from hyperaccumulators to sexually unsuitable plant species, but genetic engineering enables this.145,146 So, the topic of phytoremediation is looking promising thanks to the use of genetic engineering to create transgenic plants with the needed properties. Rather than making hyperaccumulators for large-biomass generation, it would be easier and more practical to change fast-growing, high-biomass species so they can become more tolerant and better at storing heavy metals. Therefore, scientists typically modify hyperaccumulators, known for their rapid growth and high biomass content, to enhance their resistance to or absorption of heavy metals. Therefore, understanding the mechanisms of heavy metal endurance and deposition in plants should inform the choice of genes for use in genetic engineering.
The robustness of the defence system against oxidative stress, which heavy metals can cause, typically reflects tolerance to heavy metals. Therefore, overexpressing genes that regulate antioxidant mechanisms is the most popular technique to promote heavy metal resistance.147 Introducing and overexpressing genes associated with the absorption, translocation, and sequestration of heavy metals148,149 is a typical technique for boosting the buildup of heavy metals through genetic engineering. Therefore, we can enhance heavy metal accumulation by transferring and overexpressing genes encoding heavy metal and metalloid transporters in targeted plants. The aforementioned genes encode metal ion transporters, such as ZIP, MTP, MATE, and HMA family members. Increasing heavy metal buildup by encouraging the production of metal chelators through genetic engineering is a good idea. This is because metal chelators work as metal binding ligands to improve heavy metal bioavailability, promote heavy metal absorption and root-to-shoot translocation, and help heavy metal ions get stored inside cells in organelles. We can overexpress natural chelator genes to enhance heavy metal absorption and translocation.150
The use of genetic engineering to boost the efficiency of plants in heavy metal phytoremediation has produced promising results, but it is not without its drawbacks. The genetic modification of several genes to enhance desirable features is time-consuming and typically unsuccessful because the mechanisms of detoxification and buildup of heavy metals are so complex and require so many genes. Because of concerns about food and environmental safety, it can be challenging to get permission to conduct field tests with genetically modified plants in some parts of the world. Therefore, if genetic engineering proves to be impractical, we must develop alternative methods to improve plant efficiency in phytoextraction.
8.3. Natural and organic modifications
The addition of natural organic modifications, or chemicals derived from natural sources, improves phytoremediation. Aspergillus niger treatment of sugar beet residue (SBR) improved plant growth and phytoextraction.151,152 This is because SBR has a lot of polysaccharides and phosphatases. Adding SBR to the soil where plants like Trifolium repens L.,151,152Tetraclinisarticulata sps (Vahl) Mast, and Crithmummaritimum sps L.153 grow made it easier for the plants to extract more HMs. It is believed that the application of SBR enhances microbial biomass in the rhizosphere region, thereby enhancing the effectiveness of phytoextraction. Microbial metal reduction also improved metal bioavailability.151,152
Composting sludge from sewage systems yields an industrial byproduct with agricultural applications. Liphadzi and Kirkham154 conducted a study on Cd and Ni phytoextraction by sunflower and found that natural chelating compounds found in sewage sludge resulted in enhanced metal bioavailability. Doichinova and Velizarova155 noted the positive impact of waste paper on phytoremediation. After fertilizing with paper trash, red oak (Quercus rubra L.) and Austrian pine (Pinus nigra Arn.) seedlings were less likely to absorb Pb and Cd. Enhanced plant growth and soil physical qualities like density and water holding capacity were responsible for this outcome.
By pyrolyzing plant and animal waste. Surface area, porosity, carbon content, pH, and cation exchange characteristics are only a few of the unusual characteristics of this material.156,157 Numerous investigations have demonstrated that biochar reduces metal bioavailability and phytotoxicity. The ability of biochar to retain water and nutrients, promote microbial growth, and recycle nutrients further enhances its positive impacts on soil. Wood biochar treatment reduced Pb levels in soil and As, Cd, and Cu levels in Zea mays shoots.158 Researchers found that the inclusion of bamboo and rice straw biochar increased the biomass of Sedum plubizincicola and decreased the concentrations of Cu and Pb in plant shoots by 46 and 71%, respectively.159 The incorporation of biochar and hydrochar into industrial soils enhanced the phytoremediation performance of Sinapis alba.160 Chuaphasuk and Prapagdee161 found that cadmium phytoextraction by Chlorophytum laxum sps R. Br. was enhanced when the bacteria were immobilized in biochar. Biochar has many applications, but there are also some drawbacks to using it. Researchers have linked the pyrolysis method of creating biochar to air pollution and other environmental problems.162 Biochar may also play a role in the release of HMs, or polycarbonate hydrocarbons, in the soil, according to several studies.163,164
To increase phytoextraction of HMs from the soil, synthetic root exudates may decrease the pH in the rhizosphere.165 In soil polluted with the organic contaminants pyrene, Lu et al.166 administered synthetic root exudates consisting of glucose, organic acid, and serine. According to the findings, glucose was more effective than organic acids and serine in degrading pyrene. Changes in the function of dehydrogenase and the composition of the microbiome caused by root exudates are likely responsible for the improvement. The use of humic acid as a phytoremediation aid in metal-contaminated soil has been documented,167,168 providing a substitute for the use of chemical chelators. By creating stable complexes with the metal ions, the organic matter rich in humic compounds can effectively immobilize the ions.169 Metal binding to humic acids has been hypothesized to reduce the bioavailability of Cu and Pb ions170 because of their high affinity for organic materials. It was found by Bandiera et al.171 that the metals were easily transferred to the shoots of fodder radish because humic compounds naturally chelate metals.
8.4. Chemical chelation
According to Jiang et al.172 and Yan & Lo,173 chemically enhanced technology is an efficient way to promote HMs absorption and their translocation in plants. Often, researchers apply multiple chemical supplements, also known as chelating agents, to enhance the results of the phytoextraction process. In the course of soil remediation, some of the most common chelating agents are chemical additives such as ethylenediamine tetraacetic acid (EDTA), ethylene glycol tetraacetic acid (EGTA), and sodium dodecyl sulphate (SDS). Among these, EDTA has garnered the most attention and research, widely acknowledged as the chemical that significantly contributes to the phytoextraction process.
Because of its ability to mobilize heavy metals, EDTA finds widespread application in the agricultural sector. Multiple reports have shown that EDTA facilitates plant uptake of HMs. Typically, hyperaccumulator plant species absorb metal–EDTA complexes.174 The quantity of EDTA, plant species, quantity of HMs, chemical characteristics of metals, and type of soil all play a role in how well EDTA works to remove polluted soil.175 In a study by Zhao et al.,176 EDTA increased Pb absorption in hydroponically grown Zea mays by a factor of 6–7 when compared to the control. When EDTA was added to the roots of Sedum alfredii,177Vicia faba seedlings,178Vetiveria zizanioides roots,179 and Canavalia ensiformis L. shoots,180 they all took in more Pb.
Researchers have discovered that EGTA, another frequently used chelating substance like EDTA, also encourages metal absorption and buildup. Asclepias rosea, Mirabilis jalapa, and Calendula officinalis all had higher Cd concentrations than expected.181,182 Additionally, Cicer arietinum plants treated with EGTA showed elevated Pb buildup in their aerial tissues.183
Researchers have documented the use of SDS, a widely available surfactant, in the field of soil contamination remediation.184 When herbaceous plants are used in an SDS-strengthening phytoremediation procedure in conjunction with soil flooding,185 HMs including Cu, Zn, Pb, and Cd become more soluble. After receiving SDS treatment, Althaea rosea186 and Calendula officinalis182 both accumulated more Cd in their roots and shoots. Pierattini et al.184 found increased Zn translocation in Populus alba.
For example, using chemicals for phytoremediation could be bad for the environment because chelating agents can be harmful to soil microorganisms, enzyme activity, and plant species. This has been highlighted in studies conducted by Mühlbachová187 and Neugschwandtner et al.188 Chelating agents have low biodegradability, which can result in their persistence in soil for extended periods. This persistence can result in metal contamination and negative environmental impacts, as noted by Lee and Sung189 and Smolínska and Kŕol.190 The observation suggests that we should apply optimal doses of chemical chelators as phytoremediation enhancers to mitigate adverse environmental effects. Fig. 6 illustrates the various methods attempted to enhance heavy metal phytoremediation, including genetic engineering, microbe-assisted, and chelate-assisted phytoremediation.22
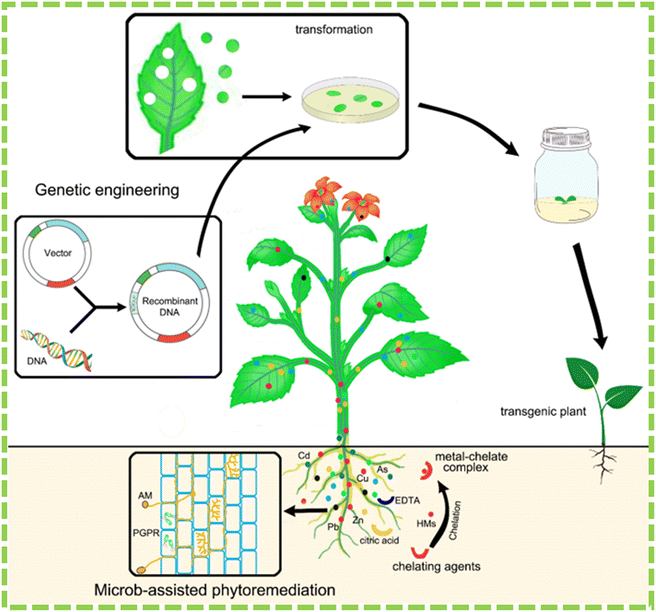 |
| Fig. 6 Methodologies that are utilized to enhance phytoremediation are depicted in the schematic diagram.22 | |
In a nutshell, phytoremediation presents a feasible approach for remediating contaminated areas. Various strategies are being employed to overcome the limitations faced by plant species, including the utilisation of certain microbes, genetic engineering techniques, the application of natural organic compounds, and the use of chelating agents.
9. Major advantages of phytoremediation
9.1. Ecologically sound and sustainable
Plants provide an environmentally friendly treatment option that eliminates contaminants with less energy and expense than traditional decontamination techniques for environmental remediation.191 This is because it utilizes solar energy and the natural processes of plants. Plants have the ability to reduce the levels of contaminants in a variety of environments, like the soil, the air, and the water. Phytoremediation will increase the capture of carbon, but only in a roundabout way. This is due to the fact that growing more plants to eliminate hazardous contaminants from the environment will lead to a reduction in the amount of carbon dioxide in the atmosphere.
The combination of phytoremediation and effective site management, also referred to as “phytomanagement,” can result in various benefits, such as economic, ecological, and social advantages.192 Several researchers have proposed the idea of integrating phytoremediation with environmental amenities,85,193 which include nutrient recycling, growth, carbon capture, transportation of water, and the treatment of water. Phytoremediation focuses solely on hazard reduction, whereas phytomanagement incorporates site management and other advantages.192 Restoration of natural resource quantity and effectiveness should be secondary objectives of phytoemediation85 rather than primary ones. We may monitor characteristics such as pH, texture, cation exchange capacity, and the variety and abundance of microbial life that represent the performance and efficiency of regenerated soil.192 Continuous evaluation programmes were recommended by Epelde et al.194 as a means of foreseeing the effectiveness of phytomanagement.
9.2. Commercially viable
Large-scale studies have demonstrated the economic viability of phytoremediation. Numerous studies have demonstrated that the expense of employing phytoremediation is far lower than that of more traditional treatment approaches. In comparison to the expense of chemical treatment, which ranges from $100 to $500 per tonne of waste eliminated, the projected price for treatment employing phytoremediation is only $20 to $40 per tonne of waste eliminated.195 According to Mulbry et al.'s196 report, phytoremediation for dairy effluent treatment outperformed more conventional methods economically. Cunningham et al.197 conducted a similar analysis, finding that phytoremediation cost $2500 to $15,000 per hectare, while microorganism treatment cost $20,000 to $60,000 per hectare, and in situ microorganism treatment cost $7500 to $20,000 per hectare to remove petroleum hydrocarbons. Additionally, the study revealed that phytoremediation significantly outperformed excavation ($40,500 to $48,600) in removing Pb from polluted locations. The overall expense of irrigation and treatment equipment (traditional approach) over a 5 year period was substantially more than the average price of $250,000 for phytoremediation, which Gatliff198 characterized as being more economically efficient. Therefore, in contrast to more traditional methods of environmental removal, phytoremediation offers a viable, cost-effective alternative.
9.3. Remediates various contaminants
The phytoremediation process has the capacity to remove the toxic effects of HMs from a wide variety of media, including soil, water, and air. This section will provide a brief analysis of the adaptable nature of the phytoremediation method, which can effectively clean up a wide range of pollutants.
Phytoremediation techniques can remediate soil that contains heavy metals (HMs), organic pollutants (OPs), or radionuclides (Rn). There are more than 400 known species of hyperaccumulators, each of which may take up to 500 times as much heavy metal from the soil as regular plants. The dry mass of 1 kg of shoots from the following plant families can accumulate: >1 mg Au and Ag; >100 mg Cd, Se, and Ta; >1000 mg Cu, Co, Cr, Ni, Pb, U, and As; and >10
000 mg Mn and Zn.199,200 You can extract zinc and cadmium from Thlaspi caerulescens.201 You can collect these plants, burn them, and use the ashes as fertilizer in soils that lack micronutrients.202
Another potential application of phytoremediation techniques is the treatment of wastewater. Historically, the marine environment has been home to a wide variety of marine plant species, including Pistia stratiotes, Salvinia molesta, Lemna spp., Azolla pinnata, Spirodela polyrhiza, Marsilea mutica, Eichhornia crassipes, Riccia fluitans, and floaters. Industries associated with agriculture, commercial, and residential domains produce effluent that contains a wide variety of hazardous materials and organic contaminants, which these plants can effectively remove due to their superior absorption capacity.203 According to the findings of a study that was conducted by Goala et al.,204 employing Azolla pinnata for the purpose of removing dairy wastewater, the effluent was removed most successfully when it was diluted by 75%.
Additionally, phytoremediation may play a significant role in the elimination of air contaminants, primarily through the process of phytovolatilization. The willow tree and hybrid poplar are capable of phytovolatilizing richoloroethylene (TCE) and perchloroethylene (PCE). Similarly, researchers found that Nephrolepsis obliterate and Chamaedorea elegans were 90% to 100% and 65% to 100% effective in eliminating formaldehyde within 2 days, respectively.205 According to De Kempeneer et al.,206Azalea indica was effective in removing toluene with 95% efficacy within 7 to 76 hours. Fig. 7(a) presents a comprehensive overview of the notable benefits linked to the phytoremediation technique.
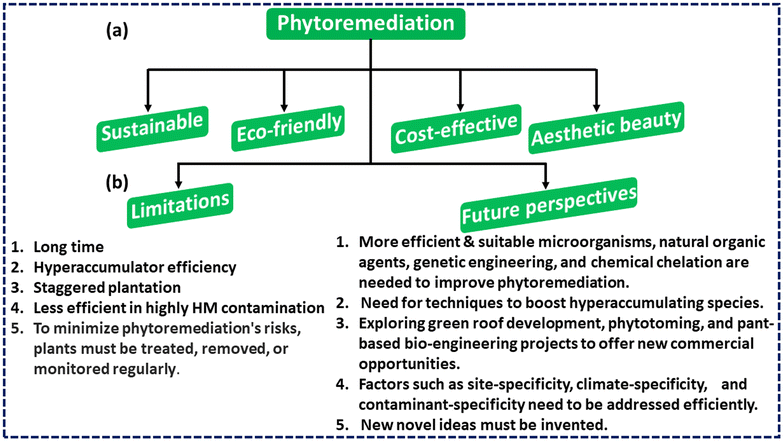 |
| Fig. 7 (a) Significant advantages associated with phytoremediation. (b) Main phytoremediation constraints and potential prospects. | |
In summary, this section provides a comprehensive analysis of the primary benefits associated with phytoremediation. Phytomanagement has the potential to yield a range of advantages, encompassing economic, ecological, and social dimensions. The visualisation and analysis of the eco-friendliness and sustainability, cost effectiveness, visually pleasant character, and remediation capabilities of the subject matter are undertaken.
10. Limitations of phytoremediation
Phytoremediation is a method that shows great promise for the removal of heavy metal contamination, but it is not without its share of drawbacks, such as:17,85
(i) It will take a very long time for remediation.
(ii) Most research is done in a controlled environment over a short period of time. This may not produce accurate results as compared to long-term field testing. Thus, several long-term field studies are needed to determine phytoremediation's full capability.
(iii) Most metal hyperaccumulators don't work well for phytoremediation because they grow slowly and don't have enough biomass.
(iv) Extensive roots and large root biomass will improve cleanup efficacy. Hence, plants must be staggered to maximize phytoremediation capability.
(v) Since plant life cannot be maintained in soils with high levels of metal pollution, this method is only useful in less contaminated places.
(vi) Animals and other living beings can be put in danger if they consume plants that contain a substantial concentration of translocated pollutants because doing so might cause toxicity. Because of this, proper management of these plants, including their treatment, removal, or monitoring, is necessary in order to reduce the potentially harmful effects of phytoremediation.
11. Current state and future prospectives of phytoremediation
Phytoremediation is widely considered the most cost-effective and environmentally friendly method for cleaning up environmental pollution.26 Although the concept of employing plants to remove environmental pollution is not new, the field is just beginning to develop. Site-specificity, climate-specificity, and contaminant-specificity are only a few of the factors that will likely slow its progress. Findings from the study, however, have substantially improved the area and are making strides towards addressing the restrictions. Researchers use methods such as suitable microbes, natural organic agents, genetic engineering, and chemical chelation to enhance the efficiency of phytoremediation. As environmental researchers and technologists look to the natural world for answers, the field's popularity and recognition have grown gradually. Positive advances include the large number of findings testing its practicality and capacity, which has drawn the interest of many people in this technology.207 As more and more successful projects come to completion, the general public's and lawmaker's perceptions of phytoremediation continue to rise.
Although recent discoveries and advancements in the field of studies have resulted in the development of a novel technique that is on the rise, holds great promise, and is efficient in terms of expense, there are still further fields in which additional study is required. For instance, additional research is necessary to ascertain the potential harmful effects of waste products or toxins released into the environment or accumulated over time on the ecosystem. In addition, there is a necessity for strategies that might promote the growth of hyperaccumulating species in extremely polluted environments, such as abandoned mining fields that are not conducive to the development of plant life. Additionally, there exists a new potential to uncover business prospects through specific markets such as green roof development, phytotoming, and certain plant-based bio-engineering projects. Fig. 7(b) summarises the main limits and future prospects that must be addressed immediately.
12. Conclusions
The issue of heavy metal pollution is a matter of global concern and poses a significant health risk to populations around the world. The utilization of phytoremediation as a method for decontaminating heavy metals (HMs) is considered to be a cost-effective, aesthetically pleasing, and environmentally sound alternative to chemical or physicochemical methods. We highlight the mechanisms through which plants assimilate various HMs and their internal transfer processes. We analyse the role of hyperaccumulator plants in detoxifying HMs. The cytoplasm of hyperaccumulator plants can store HMs by dividing cells. This protects weak spots from HMs and stops metabolic processes throughout the plant. This study investigates the classification of plants into three categories: HM aggregator species, indicator species, and excluder species. The focus is on understanding their distinct roles in detoxifying HMs and the potential effects they may have on plant cells. In order to minimise the toxicity of HMs, plants utilise two main defence mechanisms: avoidance and tolerance mechanisms. A comprehensive investigation of the operational mechanisms of phytoremediation, which include phytoextraction, phytodegradation/transformation, phytovolatilization, phytofiltration, and phytostabilization, is analysed. These techniques function by assimilating pollutants via the roots, decomposing them into less detrimental compounds, and immobilising pollutants in the soil to hinder their dissemination. Phytoremediation offers a viable strategy for the remediation of polluted regions. Different approaches are being utilised to address the constraints encountered by plant species, such as the incorporation of certain microorganisms, genetic manipulation methods, the utilisation of natural organic compounds, and the application of chelating agents. Phytomanagement possesses the capacity to generate a multitude of benefits, spanning across economic, ecological, and social aspects. Ultimately, the constraints, present conditions, and future prospects of phytoremediation have been emphasised.
Abbreviations
AMF | Arbuscular mycorrhizal fungi |
BCF | Bioconcentration Factor |
CAT | Catalase |
DMSe | Dimethylselenide |
EDTA | Ethylenediaminetetraacetic acid |
EGTA | Ethylene glycol tetra acetic acid |
GR | Glutathione reductase |
MTs | metallothioneins |
PCs | Phytochelatins |
PCE | Perchloroethylene |
PGPR | Plant growth promoting rhizobacteria |
PGPE | Plant growth promoting endophytic |
POD | Peroxidase |
RDX | Hexahydro-1,3,5-trinitro-1,3,5-triazine |
ROS | Reactive oxygen species |
SBR | Sugar beet residue |
SDS | Sodium dodecyl sulphate |
SeCys | Selenocysteine |
SeMet | Selenomethionine |
SOD | Superoxide dismutase |
TER | Terbuthylazine |
TF | Translocation factor |
TNT | Trinitrotoluene |
Conflicts of interest
Authors declare no conflict of interest.
Acknowledgements
Authors greatly acknowledge R&D section of IIMT University Meerut, SoBST, Uttar Pradesh, India. No government, private, or nonprofit organization provided particular funding for this study.
References
- V. Shah and A. Daverey, Environ. Technol. Innovation, 2020, 18, 100774 CrossRef.
- B. Nedjimi, Acta Bot. Gallica, 2009, 156(3), 391–397 CrossRef CAS.
- N. Sarwar, S. S. Malhi, M. H. Zia, A. Naeem, S. Bibi and G. Farid, J. Sci. Food Agric., 2010, 90, 925–937 CrossRef CAS PubMed.
- M. Z. U. Rehman, M. Rizwan, S. Ali, Y. S. Ok, W. Ishaque and Saifullah,
et al.
, Ecotoxicol. Environ. Saf., 2017, 143, 236–248 CrossRef CAS PubMed.
- J. Suman, O. Uhlik, J. Viktorova and T. Macek, Front. Plant Sci., 2018, 9, 1476 CrossRef PubMed.
- M. Cempel and G. Nikel, Pol. J. Environ. Stud., 2006, 15, 375–382 CAS.
- S. Clemens, Biochimie, 2006, 88(11), 1707–1719 CrossRef CAS PubMed.
- E. Fasani, A. Manara, F. Martini, A. Furini and G. DalCorso, Plant, Cell Environ., 2018, 41(5), 1201–1232 CrossRef CAS PubMed.
- K. E. Gerhardt, P. D. Gerwing and B. M. Greenberg, Plant Sci., 2017, 256, 170–185 CrossRef CAS PubMed.
- M. M. Hasan, M. N. Uddin, I. Ara-Sharmeen, H. F. Alharby, Y. Alzahrani, K. R. Hakeem and L. Zhang, Plants, 2019, 8(9), 295 CrossRef CAS PubMed.
- B. Nedjimi and Y. Daoud, Desalination, 2009, 249(1), 163–166 CrossRef CAS.
- V. Sheoran, A. S. Sheoran and andP. Poonia, Crit. Rev. Environ. Sci. Technol., 2010, 41(2), 168–214 CrossRef.
- R. A. Wuana and F. E. Okieimen, Int. Scholarly Res. Not., 2011, 2011, 402647 Search PubMed.
- G. DalCorso, E. Fasani, A. Manara, G. Visioli and A. Furini, Int. J. Mol. Sci., 2019, 20(14), 3412 CrossRef CAS PubMed.
- B. C. Ekeoma, L. N. Ekeoma, M. Yusuf, A. Haruna, C. K. Ikeogu and Z. M. A. Merican,
et al.
, J. Biotechnol., 2023, 369, 14–34 CrossRef CAS PubMed.
- H. H. T. Nguyen, H. T. Nguyen, S. F. Ahmed, N. Rajamohan, M. Yusuf and A. Sharma,
et al.
, Environ. Res., 2023, 227, 115800 CrossRef CAS PubMed.
- H. Ali, E. Khan and andM. A. Sajad, Chemosphere, 2013, 91(7), 869–881 CrossRef CAS PubMed.
-
W. R. Berti, and S. D. Cunningham, Phytostabilization of metals. Phytoremediation of Toxic Metals: Using Plants to Clean up the Environment. Wiley, New York, 2000, pp. 71–88 Search PubMed.
- J. M. Jacob, C. Karthik, R. G. Saratale, S. S. Kumar, D. Prabakar, K. Kadirvelu and A. Pugazhendhi, J. Environ. Manage., 2018, 217, 56–70 CrossRef CAS PubMed.
- B. V. Aken, P. A. Correa and J. L. Schnoor, Environ. Sci. Technol., 2010, 44(8), 2767–2776 CrossRef PubMed.
- B. Nedjimi, SN Appl. Sci., 2021, 3(3), 286 CrossRef CAS.
- A. Yan, Y. Wang, S. N. Tan, M. L. Mohd Yusof, S. Ghosh and Z. Chen, Front. Plant Sci., 2020, 11, 359 CrossRef PubMed.
- S. Greipsson, Nat. Edu. Knowl., 2011, 3(10), 7 Search PubMed.
- S. H. Awa and T. Hadibarata, Water, Air, Soil Pollut., 2020, 231(2), 47 CrossRef CAS.
- P. Masson, T. Dalix and S. Bussiere, Commun. Soil Sci. Plant Anal., 2010, 41(3), 231–243 CrossRef CAS.
- S. Ashraf, Q. Ali, Z. A. Zahir, S. Ashraf and H. N. Asghar, Ecotoxicol. Environ. Saf., 2019, 174, 714–727 CrossRef CAS PubMed.
- S. Das, K. W. Sultana, A. R. Ndhlala, M. Mondal and andI. Chandra, Environ. Health Insights, 2023, 17, 11786302231201259 CrossRef PubMed.
-
R. Naidu, D. Oliver and S. McConnell, Heavy metal phytotoxicity in soils. in Proceedings of the Fifth National Workshop on the Assessment of Site Contamination, National Environment Protection Council Service Corporation, 2003, pp. 235–241 Search PubMed.
- V. Shah and A. Daverey, Environ. Technol. Innovation, 2020, 18, 100774 CrossRef.
- A. Sharma, V. Kumar, B. Shahzad, M. Ramakrishnan, G. P. Singh Sidhu and A. S. Bali,
et al.
, J. Plant Growth Regul., 2020, 39, 509–531 CrossRef CAS.
- A. A. Dalvi and S. A. Bhalerao, Ann. Plant Sci., 2013, 2(9), 362–368 Search PubMed.
-
W. A. Peer, I. R. Baxter, E. L. Richards, J. L. Freeman, and A. S. Murphy, Phytoremediation and hyperaccumulator plants, in Molecular Biology of Metal Homeostasis and Detoxification, ed. M. J. Tamas and E. Martinoia, Springer, Berlin, 2005, pp. 299–340 Search PubMed.
- H. Ali, E. Khan and M. A. Sajad, Chemosphere, 2013, 91(7), 869–881 CrossRef CAS PubMed.
- S. Thakur, L. Singh, Z. A. Wahid, M. F. Siddiqui, S. M. Atnaw and M. F. M. Din, Environ. Monit. Assess., 2016, 188, 1–11 CrossRef PubMed.
- Y. P. Tong, R. Kneer and Y. G. Zhu, Trends Plant Sci., 2004, 9(1), 7–9 CrossRef CAS PubMed.
- A. R. Memon, D. Aktoprakligil, A. Özdemir and A. Vertii, Turk. J. Bot., 2001, 25(3), 111–121 Search PubMed.
-
J. Kadukova, and J. Kavuličova,Phytoremediation of heavy metal contaminated soils—plant stress assessment, in Handbook of Phytoremediation, ed. I. A. Golubev, Nova Science Publishers, Inc., New York, 2010 Search PubMed.
- B. Nedjimi and Y. Daoud, Flora: Morphol. Distrib. Funct. Ecol. Plants., 2009, 204(4), 316–324 CrossRef.
- S. Clemens, Biochimie, 2006, 88(11), 1707–1719 CrossRef CAS PubMed.
- M. Gómez-Garrido, J. Mora Navarro, F. J. Murcia Navarro and Á. Faz Cano, Int. J. Phytorem., 2018, 20(10), 1033–1042 CrossRef PubMed.
- X. Zhang, H. Rui, F. Zhang, Z. Hu, Y. Xia and Z. Shen, Front. Plant Sci., 2018, 9, 107 CrossRef PubMed.
- X. Yang, Y. Feng, Z. He and P. J. Stoffella, J. Trace Elem. Med. Biol., 2005, 18(4), 339–353 CrossRef CAS PubMed.
- A. J. Baker and R. Brooks, Biorecovery, 1989, 1(2), 81–126 CAS.
- A. R. Memon and P. Schröder, Environ. Sci. Pollut. Res., 2009, 16, 162–175 CrossRef CAS PubMed.
-
A. Manara, Plant responses to heavy metal toxicity, in Plants and HeavyMetals, ed. A. Furini, Springer, Dordrecht, 2012, pp. 27–53 Search PubMed.
- S. S. Sharma and K. J. Dietz, J. Exp. Bot., 2006, 57(4), 711–726 CrossRef CAS PubMed.
-
D. K. Gupta, H. Vandenhove, and M. Inouhe, Role of phytochelatins in heavy metal stress and detoxification mechanisms in plants, in Heavy MetalStress
in Plants, ed. D. K. Gupta, F. J. Corpas, and J. M. Palma, Springer, Berlin, 2013, pp. 73–94 Search PubMed.
- R. L. Sun, Q. X. Zhou and C. X. Jin, Plant Soil, 2006, 285, 125–134 CrossRef CAS.
- J. R. Domínguez-Solís, M. C. López-Martín, F. J. Ager, M. D. Ynsa, L. C. Romero and C. Gotor, Plant Biotechnol. J., 2004, 2(6), 469–476 CrossRef PubMed.
- V. Sheoran, A. S. Sheoran and P. Poonia, Crit. Rev. Environ. Sci. Technol., 2010, 41(2), 168–214 CrossRef.
- B. H. Robinson, E. Lombi, F. J. Zhao and S. P. McGrath, New Phytol., 2003, 158(2), 279–285 CrossRef CAS.
- S. Eapen and S. F. D'souza, Biotechnol. Adv., 2005, 23(2), 97–114 CrossRef CAS PubMed.
- H. Huang, D. K. Gupta, S. Tian, X. E. Yang and T. Li, Environ. Sci. Pollut. Res., 2012, 19, 1640–1651 CrossRef CAS PubMed.
- D. K. Gupta, F. T. Nicoloso, M. R. C. Schetinger, L. V. Rossato, L. B. Pereira, G. Y. Castro and R. D. Tripathi, J. Hazard. Mater., 2009, 172(1), 479–484 CrossRef CAS PubMed.
- M. Jozefczak, T. Remans, J. Vangronsveld and A. Cuypers, Int. J. Mol. Sci., 2012, 13(3), 3145–3175 CrossRef CAS PubMed.
- E. Fourati, M. Wali, K. Vogel-Mikuš, C. Abdelly and T. Ghnaya, Plant Physiol. Biochem., 2016, 108, 295–303 CrossRef CAS PubMed.
- A. Jacobs, T. Drouet and N. Noret, Plant Soil, 2018, 430, 381–394 CrossRef CAS.
- K. A. Ghazaryan, H. S. Movsesyan, T. M. Minkina, S. N. Sushkova and V. D. Rajput, Environ. Geochem. Health, 2021, 43, 1327–1335 CrossRef CAS PubMed.
- W. J. Yang, J. F. Gu, H. Zhou, F. Huang, T. Y. Yuan, J. Y. Zhang and B. H. Liao, Sci. Pollut. Res., 2020, 27, 16134–16144 CrossRef CAS PubMed.
- A. Khalid, M. Farid, M. Zubair, M. Rizwan, U. Iftikhar, H. K. Ishaq and S. Ali, Int. J. Environ. Res., 2020, 14, 243–255 CrossRef CAS.
- P. Das, R. Datta, K. C. Makris and D. Sarkar, Environ. Pollut., 2010, 158(5), 1980–1983 CrossRef CAS PubMed.
- N. K. Hannink, M. Subramanian, S. J. Rosser, A. Basran, J. A. Murray, J. V. Shanks and N. C. Bruce, Int. J. Phytorem., 2007, 9(5), 385–401 CrossRef CAS PubMed.
- C. L. Just and J. L. Schnoor, Environ. Sci. Technol., 2004, 38(1), 290–295 CrossRef CAS PubMed.
- V. de Farias, L. T. Maranho, E. C. de Vasconcelos, M. A. da Silva Carvalho Filho, L. G. Lacerda, J. A. M. Azevedo and C. R. Soccol, Appl. Biochem. Biotechnol., 2009, 157, 10–22 CrossRef CAS PubMed.
- T. Shahzad, C. Chenu, P. Genet, S. Barot, N. Perveen, C. Mougin and S. Fontaine, Soil Biol. Biochem., 2015, 80, 146–155 CrossRef CAS.
- J. L. Kirk, J. N. Klironomos, H. Lee and J. T. Trevors, Environ. Pollut., 2005, 133(3), 455–465 CrossRef CAS PubMed.
- N. Papadopoulos and G. Zalidis, Environ. Processes, 2019, 6(4), 985–1003 CrossRef CAS.
- C. J. Sampaio, J. R. de Souza, A. O. Damião, T. C. Bahiense and M. R. Roque, Biotechnology, 2019, 9, 1–10 Search PubMed.
- A. Mahar, P. Wang, A. Ali, M. K. Awasthi, A. H. Lahori, Q. Wang and Z. Zhang, Ecotoxicol. Environ. Saf., 2016, 126, 111–121 CrossRef CAS PubMed.
- M. P. de Souza, C. M. Lytle, M. M. Mulholland, M. L. Otte and N. Terry, Plant Physiol., 2000, 122(4), 1281–1288 CrossRef CAS PubMed.
- N. Terry, A. M. Zayed, M. P. De Souza and A. S. Tarun, Annu. Rev. Plant Biol., 2000, 51(1), 401–432 CrossRef CAS PubMed.
- A. P. Marques, A. O. Rangel and P. M. Castro, Crit. Rev. Environ. Sci. Technol., 2009, 39(8), 622–654 CrossRef CAS.
- S. P. Bizily, C. L. Rugh and R. B. Meagher, Int. Biotechnol., 2000, 18(2), 213–217 CAS.
- J. Vangronsveld, R. Herzig, N. Weyens, J. Boulet, K. Adriaensen, A. Ruttens and M. Mench, Environ. Sci. Pollut. Res., 2009, 16, 765–794 CrossRef CAS PubMed.
- J. Mesjasz-Przybyłowicz, M. Nakonieczny, P. Migula, M. Augustyniak, M. Tarnawska, W. Reimold and E. Głowacka, Environ. Sci. Pollut. Res., 2004, 46, 75–85 Search PubMed.
-
M. T. Javed, K. Tanwir, M. S. Akram, M. Shahid, N. K. Niazi, S. Lindberg, Chapter 20 – Phytoremediation of cadmium-polluted water/sediment by aquatic macrophytes: role of plant-induced pH changes, in Cadmium Toxicity and Tolerance in Plants, ed. M. Hasanuzzaman, M. N. V. Prasad and M. Fujita, Academic Press, London, 2019, pp. 495–529 Search PubMed.
- R. A. Wuana and F. E. Okieimen, Ecology, 2011, 2011, 402647 Search PubMed.
- V. Hooda, J. Environ. Biol., 2007, 28(2), 367–376 CAS.
- F. V. Tomé, P. B. Rodríguez and J. C. Lozano, Sci. Total Environ., 2008, 393(2–3), 351–357 CrossRef PubMed.
- S. Rezania, S. M. Taib, M. F. M. Din, F. A. Dahalan and H. Kamyab, J. Hazard. Mater., 2016, 318, 587–599 CrossRef CAS PubMed.
-
P. Dhanwal, A. Kumar, S. Dudeja, V. Chhokar and V. Beniwal, Advanced Environmental Biotechnology, Springer, Singapore, 2017, pp. 227–241 Search PubMed.
- Z. Al Chami, N. Amer, L. Al Bitar and I. Cavoski, Int. J. Environ. Sci. Technol., 2015, 12, 3957–3970 CrossRef CAS.
- F. Monaci, D. Trigueros, M. D. Mingorance and S. Rossini-Oliva, Environ. Geochem. Health, 2020, 42, 2345–2360 CrossRef CAS PubMed.
- G. Bacchetta, M. E. Boi, G. Cappai, G. De Giudici, M. Piredda and M. Porceddu, Environ. Contam. Toxicol., 2018, 101(6), 758–765 CrossRef CAS PubMed.
- A. Kafle, A. Timilsina, A. Gautam, K. Adhikari, A. Bhattarai and N. Aryal, Environ. Adv., 2022, 8, 100203 CrossRef CAS.
-
R. Barlow, N. Bryant, J. Andersland and S. Sahi, Proceedings of the 2000 Conference on Hazardous Waste Research, pp. , pp. 112–114 Search PubMed.
- A. L. Salido, K. L. Hasty, J. M. Lim and D. J. Butcher, Int. J. Phytorem., 2003, 5(2), 89–103 CrossRef CAS PubMed.
-
A. Hernández, N. Loera, M. Contreras, L. Fischer and D. Sánchez, Energy Technology 2019: Carbon Dioxide Management and Other Technologies, Springer International Publishing, 2019, pp. 137–147 Search PubMed.
- Y. Yang, Y. Ge, P. Tu, H. Zeng, X. Zhou, D. Zou and Q. Zeng, J. Hazard. Mater., 2019, 363, 385–393 CrossRef CAS PubMed.
- M. Manzoor, I. Gul, I. Ahmed, M. Zeeshan, I. Hashmi, B. A. Z. Amin and M. Arshad, Chemosphere, 2019, 227, 561–569 CrossRef CAS PubMed.
- X. Huang, D. Luo, X. Chen, L. Wei, Y. Liu, Q. Wu and L. Liu, Int. J. Environ. Res. Public Health, 2019, 16(8), 1328 CrossRef CAS PubMed.
- N. Khalid, A. Noman, M. Aqeel, A. Masood and A. Tufail, Int. J. Environ. Sci. Technol., 2019, 16, 2091–2100 CrossRef CAS.
- V. Singh, B. Pandey and S. Suthar, Ecotoxicol. Environ. Saf., 2019, 179, 88–95 CrossRef CAS PubMed.
- Y. Gong, J. Chen and R. Pu, Int. J. Phytorem., 2019, 21(11), 1080–1089 CrossRef CAS PubMed.
- Y. He, A. A. Langenhoff, N. B. Sutton, H. H. Rijnaarts, M. H. Blokland, F. Chen and P. Schröder, Environ. Sci. Technol., 2017, 51(8), 4576–4584 CrossRef CAS PubMed.
- P. Jha, R. Jobby and N. S. Desai, J. Hazard. Mater., 2016, 311, 158–167 CrossRef CAS PubMed.
- J. V. Headley, K. M. Peru, J. L. Du, N. Gurprasad and D. W. Mcmartin, J. Environ. Sci. Health, Part A, 2008, 43(4), 361–364 CrossRef CAS PubMed.
- A. N. Kagalkar, M. U. Jadhav, V. A. Bapat and S. P. Govindwar, Bioresour. Technol., 2011, 102(22), 10312–10318 CrossRef CAS PubMed.
- L. Ruppert, Z. Q. Lin, R. P. Dixon and K. A. Johnson, J. Hazard. Mater., 2013, 262, 1230–1236 CrossRef CAS PubMed.
- A. Wiessner, U. Kappelmeyer, M. Kaestner, L. Schultze-Nobre and P. Kuschk, Water Res., 2013, 47(13), 4265–4273 CrossRef CAS PubMed.
- A. San Miguel, P. Ravanel and M. Raveton, J. Hazard. Mater., 2013, 244, 60–69 CrossRef PubMed.
- E. L. Arthur, P. J. Rice, P. J. Rice, T. A. Anderson, S. M. Baladi, K. L. Henderson and J. R. Coats, Crit. Rev. Plant Sci., 2005, 24(2), 109–122 CrossRef CAS.
- P. K. Rai, Environ. Technol. Innovation, 2019, 15, 100393 CrossRef.
- P. J. Favas, J. Pratas and M. N. V. Prasad, Sci. Total Environ., 2012, 433, 390–397 CrossRef CAS PubMed.
- J. Pratas, P. J. Favas, C. Paulo, N. Rodrigues and M. N. V. Prasad, Int. J. Phytorem., 2012, 14(3), 221–234 CrossRef CAS PubMed.
- R. Chandra and S. Yadav, Ecol. Eng., 2010, 36(10), 1277–1284 CrossRef.
- H. González, D. Fernández-Fuego, A. Bertrand and A. González, Environ. Sci. Pollut. Res., 2019, 26, 26242–26253 CrossRef PubMed.
- M. Zhou, T. Ghnaya, H. Dailly, G. Cui, B. Vanpee, R. Han and S. Lutts, Chemosphere, 2019, 233, 954–965 CrossRef CAS PubMed.
- W. Yang, Y. Yang, Z. Ding, X. Yang, F. Zhao and Z. Zhu, Ecol. Eng., 2019, 136, 79–88 CrossRef.
- C. D. Jadia and M. H. Fulekar, Int. J. Environ. Eng. Manag., 2008, 7(5), 547–558 CrossRef CAS.
- X. Li, X. Zhang, X. Wang and Z. Cui, Ecotoxicol. Environ. Saf., 2019, 180, 517–525 CrossRef CAS PubMed.
- A. Anton and G. Mathe-Gaspar, Z. Naturforsch., C: J. Biosci., 2005, 60, 244–246 CAS.
- V. Antoniadis, E. Levizou, S. M. Shaheen, Y. S. Ok, A. Sebastian, C. Baum and J. Rinklebe, Earth Sci. Rev., 2017, 171, 621–645 CrossRef CAS.
- M. Dary, M. A. Chamber-Pérez, A. J. Palomares and E. Pajuelo, J. Hazard. Mater., 2010, 177(1–3), 323–330 CrossRef CAS PubMed.
- W. Guidi Nissim, E. Palm, S. Mancuso and E. Azzarello, Environ. Sci. Pollut. Res., 2018, 25, 9114–9131 CrossRef CAS PubMed.
- Q. Wu, S. Wang, P. Thangavel, Q. Li, H. Zheng, J. Bai and R. Qiu, Int. J. Phytorem., 2011, 13(8), 788–804 CrossRef PubMed.
- N. Sarwar, M. Imran, M. R. Shaheen, W. Ishaque, M. A. Kamran, A. Matloob and S. Hussain, Chemosphere, 2017, 171, 710–721 CrossRef CAS PubMed.
- K. E. Gerhardt, P. D. Gerwing and B. M. Greenberg, Plant Sci., 2017, 256, 170–185 CrossRef CAS PubMed.
- M. Goswami, P. Chakraborty, K. Mukherjee, G. Mitra, P. Bhattacharyya, S. Dey and P. Tribedi, J. Exp. Microbiol., 2018, 6(5), 223–231 Search PubMed.
- R. G. Lacalle, J. D. Aparicio, U. Artetxe, E. Urionabarrenetxea, M. A. Polti, M. Soto and J. M. Becerril, Heliyon, 2020, 6(8), e04550 CrossRef CAS PubMed.
- M. R. Monti, A. M. Smania, G. Fabro, M. E. Alvarez and C. E. Argarana, Appl. Environ. Microbiol., 2005, 71(12), 8864–8872 CrossRef CAS PubMed.
- G. Perrin-Ganier, F. Schiavon, J. L. Morel and M. Schiavon, Chemosphere, 2001, 44(4), 887–892 CrossRef PubMed.
- C. Becerra-Castro, A. Prieto-Fernández, V. Álvarez-Lopez, C. Monterroso, M. I. Cabello-Conejo, M. J. Acea and P. S. Kidd, Int. J. Phytorem., 2011, 13(1), 229–244 CrossRef CAS PubMed.
- R. A. Gill, S. Ahmar, B. Ali, M. H. Saleem, M. U. Khan, W. Zhou and S. Liu, Int. J. Mol. Sci., 2021, 22(23), 12792 CrossRef CAS PubMed.
- Y. Ma, M. N. V. Prasad, M. Rajkumar and H. J. B. A. Freitas, Biotechnol. Adv., 2011, 29(2), 248–258 CrossRef CAS PubMed.
- A. Braud, K. Jézéquel, S. Bazot and T. Lebeau, Chemosphere, 2009, 74(2), 280–286 CrossRef PubMed.
- P. Chang, K. E. Gerhardt, X. D. Huang, X. M. Yu, B. R. Glick, P. D. Gerwing and B. M. Greenberg, Int. J. Phytorem., 2014, 16(11), 1133–1147 CrossRef CAS PubMed.
- A. G. Babu, J. D. Kim and B. T. Oh, J. Hazard. Mater., 2013, 250, 477–483 CrossRef PubMed.
- A. C. Agnello, M. Bagard, E. D. Van Hullebusch, G. Esposito and D. Huguenot, Sci. Total Environ., 2016, 563, 693–703 CrossRef PubMed.
- R. Dong, L. Gu, C. Guo, F. Xun and J. Liu, Ecotoxicology, 2014, 23, 674–680 CrossRef CAS PubMed.
- B. R. Glick, Microbiol. Res., 2014, 169(1), 30–39 CrossRef CAS PubMed.
- N. R. Rane, S. Tapase, A. Kanojia, A. Watharkar, E. S. Salama, M. Jang and B. H. Jeon, Bioresour. Technol., 2022, 344, 126246 CrossRef CAS PubMed.
-
A. Kumar, A. K. Chaturvedi, K. Yadav, K. P. Arunkumar, S. K. Malyan, P. Raja and A. N. Yadav, Recent Advancement in White Biotechnology Through Fungi Volume 3: Perspective for Sustainable Environments 2019, 437–461 Search PubMed.
- P. Bhantana, M. S. Rana, X. C. Sun, M. G. Moussa, M. H. Saleem, M. Syaifudin and C. X. Hu, Symbiosis, 2021, 84, 19–37 CrossRef CAS.
- V. Göhre and U. Paszkowski, Planta, 2006, 223, 1115–1122 CrossRef PubMed.
- T. Vamerali, M. Bandiera and G. Mosca, Environ. Chem. Lett., 2010, 8, 1–17 CrossRef CAS.
- B. D. Chen, X. L. Li, H. Q. Tao, P. Christie and M. H. Wong, Chemosphere, 2003, 50(6), 839–846 CrossRef CAS PubMed.
- A. A. Meharg, J. W. Cairney and N. Maguire, Chemosphere, 1997, 34(12), 2495–2504 CrossRef CAS.
- K. Scheibner, M. Hofrichter, A. Herre, J. Michels and W. Fritsche, Appl. Microbiol. Biotechnol., 1997, 47, 452–457 CrossRef CAS PubMed.
- P. K. Donnelly, J. A. Entry and D. L. Crawford, Appl. Environ. Microbiol., 1993, 59(8), 2642–2647 CrossRef CAS PubMed.
- P. K. Donnelly and J. S. Fletcher, Environ. Contam. Toxicol., 1995, 54(4), 507–513 CAS.
- A. Braun-Lüllemann, A. Hüttermann and A. Majcherczyk, Appl. Microbiol. Biotechnol., 1999, 53, 127–132 CrossRef.
- E. J. Joner and C. Leyval, Sustain. Agric., 2009, 885–894 Search PubMed.
- S. Criquet, E. Joner, P. Leglize and C. Leyval, Biotechnol. Lett., 2000, 22(21), 1733–1737 CrossRef CAS.
- A. Berken, M. M. Mulholland, D. L. LeDuc and N. Terry, Crit. Rev. Plant Sci., 2002, 21, 567–582 CrossRef CAS.
- A. P. Marques, A. O. Rangel and P. M. Castro, Crit. Rev. Environ. Sci. Technol., 2009, 39, 622–654 CrossRef CAS.
- A. Koźmińska, A. Wiszniewska, E. Hanus-Fajerska and E. Muszyńska, Plant Biotechnol. Rep., 2018, 12, 1–14 CrossRef PubMed.
- D. Mani and C. Kumar, Int. J. Environ. Sci. Technol., 2014, 11, 843–872 CrossRef CAS.
- N. Das, S. Bhattacharya and M. K. Maiti, Plant Physiol. Biochem., 2016, 105, 297–309 CrossRef CAS PubMed.
- G. Wu, H. Kang, X. Zhang, H. Shao, L. Chu and C. Ruan, J. Hazard. Mater., 2010, 174(1–3), 1–8 CrossRef CAS PubMed.
- R. Azcón, A. Medina, A. Roldán, B. Biró and A. Vivas, Chemosphere, 2009, 75(3), 327–334 CrossRef PubMed.
- A. Medina, M. Vassileva, J. M. Barea and R. Azcón, Appl. Soil Ecol., 2006, 33(1), 87–98 CrossRef.
- D. A. Fernández, A. Roldán, R. Azcón, F. Caravaca and E. Bååth, Microb. Ecol., 2012, 63, 794–803 CrossRef PubMed.
- M. S. Liphadzi and M. B. Kirkham, J. Crop. Improv., 2006, 16(1–2), 153–172 CrossRef CAS.
- V. Doichinova and E. Velizarova, Procedia Environ. Sci., 2013, 18, 731–736 CrossRef CAS.
- J. Jiang, R. K. Xu, T. Y. Jiang and Z. Li, J. Hazard. Mater., 2012, 229, 145–150 CrossRef PubMed.
- J. Lehmann, M. C. Rillig, J. Thies, C. A. Masiello, W. C. Hockaday and D. Crowley, Soil Biol. Biochem., 2011, 43(9), 1812–1836 CrossRef CAS.
- T. Namgay, B. Singh and B. P. Singh, Soil Res., 2010, 48(7), 638–647 CrossRef CAS.
- H. Lu, Z. Li, S. Fu, A. Mendez, G. Gasco and J. Paz-Ferreiro, PLoS One, 2014, 9(4), e95218 CrossRef PubMed.
- E. Cárdenas-Aguiar, B. Ruiz, E. Fuente, G. Gascó and A. Méndez, Waste Biomass Valorization, 2020, 11, 5197–5210 CrossRef.
- C. Chuaphasuk and B. Prapagdee, Environ. Sci. Pollut. Res., 2019, 26, 23679–23688 CrossRef CAS PubMed.
- J. M. dos Santos Barbosa, N. Ré-Poppi and M. Santiago-Silva, Environ. Res., 2006, 101(3), 304–311 CrossRef PubMed.
- D. L. Jones, G. Edwards-Jones and D. Y. Murphy, Soil Biol. Biochem., 2011, 43(4), 804–813 CrossRef CAS.
- A. Mendez, A. Gomez, J. Paz-Ferreiro and G. Gasco, Chemosphere, 2012, 89(11), 1354–1359 CrossRef CAS PubMed.
- P. Thangavel and C. V. Subbhuraam, Proc. Natl. Acad. Sci. India B, 2004, 70(1), 109–130 CAS.
- H. Lu, J. Sun and L. Zhu, Sci. Rep., 2017, 7(1), 7130 CrossRef PubMed.
- M. W. Evangelou, H. Daghan and A. Schaeffer, Chemosphere, 2004, 57(3), 207–213 CrossRef CAS PubMed.
- J. A. L. Figueroa, K. Wrobel, S. Afton, J. A. Caruso, J. F. G. Corona and K. Wrobel, Chemosphere, 2008, 70(11), 2084–2091 CrossRef CAS PubMed.
- N. Hattab, M. Soubrand, R. Guégan, M. Motelica-Heino, X. Bourrat, O. Faure and J. L. Bouchardon, Environ. Sci. Pollut. Res., 2014, 21, 10470–10480 CrossRef CAS PubMed.
- A. Wiszniewska, E. Hanus-Fajerska, E. Muszyńska and K. Ciarkowska, Pedosphere, 2016, 26(1), 1–12 CrossRef.
- M. Bandiera, G. Mosca and T. Vamerali, Desalination, 2009, 246(1–3), 78–91 CrossRef CAS.
- M. Jiang, S. Liu, Y. Li, X. Li, Z. Luo, H. Song and Q. Chen, Ecotoxicol. Environ. Saf., 2019, 170, 502–512 CrossRef CAS PubMed.
- D. Y. Yan and I. M. Lo, J. Hazard. Mater., 2012, 199, 51–57 CrossRef PubMed.
- E. Awokunmi, S. Asaolu, O. Ajayi and O. Adebayo, Br. J. Environ. Clim. Change, 2012, 2, 153 CrossRef PubMed.
- M. W. Evangelou, M. Ebel and A. Schaeffer, Chemosphere, 2007, 68, 989–1003 CrossRef CAS PubMed.
- Z. Zhao, M. Xi, G. Jiang, X. Liu, Z. Bai and Y. Huang, J. Hazard. Mater., 2010, 181, 455–459 CrossRef CAS PubMed.
- Y. Sun, Q. Zhou, L. Wang and W. Liu, Bull. Environ. Contam. Toxicol., 2009, 82, 348 CrossRef CAS PubMed.
- M. Shahid, A. Austruy, G. Echevarria, M. Arshad, M. Sanaullah, M. Aslam, M. Nadeem, W. Nasim and C. Dumat, Soil Sediment Contam., 2014, 23, 389–416 CrossRef CAS.
- S. S. Andra, R. Datta, D. Sarkar, S. K. Saminathan, C. P. Mullens and S. B. Bach, Environ. Pollut., 2009, 157, 2173–2183 CrossRef CAS PubMed.
- M. B. Gabos, C. A. D. Abreu and A. R. Coscione, Sci. Agric., 2009, 66, 506–514 CrossRef CAS.
- J. N. Liu, Q. X. Zhou, T. Sun, L. Q. Ma and S. Wang, Bull. Environ. Contam. Toxicol., 2008, 80, 260–265 CrossRef CAS PubMed.
- J. Liu, Q. Zhou and S. Wang, Int. J. Phytorem., 2010, 12, 503–515 CrossRef CAS PubMed.
- L. Sakouhi, S. Rahoui, M. B. Massoud, S. Munemasa, E. E. Ferjani, Y. Murata and A. Chaoui, J. Plant Growth Regul., 2016, 35, 1064–1073 CrossRef CAS.
- E. C. Pierattini, A. Francini, A. Raffaelli and L. Sebastiani, Tree Physiol., 2018, 38, 109–118 CrossRef CAS PubMed.
- A. Ramamurthy and H. Schalchian, Environ. Prot. Eng., 2013, 39, 87–99 CAS.
- J. N. Liu, Q. X. Zhou, S. Wang and T. Sun, Environ. Monit. Assess., 2009, 149, 419–427 CrossRef CAS PubMed.
- G. Mühlbachová, Ecol. Eng., 2011, 37, 1064–1071 CrossRef.
- R. W. Neugschwandtner, P. Tlustoš, M. Komárek, J. Száková and L. Jakoubková, Int. J. Phytorem., 2012, 14, 754–771 CrossRef CAS PubMed.
- J. Lee and K. Sung, Ecol. Eng., 2014, 73, 386–394 CrossRef.
- B. Smolínska, K. Kŕol and J. Chem, Technol. Biotechnol., 2012, 87, 1360–1365 CrossRef.
- S. Susarla, V. F. Medina and S. C. McCutcheon, Ecol. Eng., 2002, 18, 647–658 CrossRef.
- A. Burges, I. Alkorta, L. Epelde and C. Garbisu, Int. J. Phytorem., 2018, 20(4), 384–397 CrossRef CAS PubMed.
- M. Rutgers, H. J. Van Wijnen, A. J. Schouten, C. Mulder, A. M. P. Kuiten, L. Brussaard and A. M. Breure, Sci. Total Environ., 2012, 415, 39–48 CrossRef CAS PubMed.
- L. Epelde, J. M. Becerril, I. Alkorta and C. Garbisu, Int. J. Phytorem., 2014, 16(10), 971–981 CrossRef PubMed.
- I. Raskin and B. D. Ensley, Curr. Opin. Biotechnol., 2000, 8, 221–226 CrossRef PubMed.
- W. Mulbry, S. Kondrad, C. Pizarro and E. Kebede-Westhead, Bioresour. Technol., 2008, 99, 8137–8142 CrossRef CAS PubMed.
- S. D. Cunningham, T. A. Anderson, A. P. Schwab and F. Hsu, Adv. Agron., 1996, 56, 55–114 CrossRef CAS.
- E. G. Gatliff, Remed. J., 1994, 4, 343–352 CrossRef.
- A. J. Baker, S. P. McGrath, R. D. Reeves and J. A. C. Smith, Phytorem. Contam. Soil Water, 2020, 85–107 Search PubMed.
- M. N. Vara Prasad and H. M. De Oliveira Freitas, Electron, J. Biotechnol., 2003, 6, 285–321 Search PubMed.
- L. Epelde, J. M. Becerril, J. Hernández-Allica, O. Barrutia and C. Garbisu, Appl. Soil Ecol., 2008, 39, 299–310 CrossRef.
- G. Koptsik, Eurasian Soil Sci., 2014, 47, 923–939 CrossRef CAS.
- H. M. Mustafa and G. Hayder, Ain Shams Eng. J., 2021, 12, 355–365 CrossRef.
- M. Goala, K. K. Yadav, J. Alam, B. Adelodun, K. S. Choi, M. M. Cabral-Pinto, A. A. Hamid, M. Alhoshan, F. A. A. Ali and A. K. Shukla, J. Water Process Eng., 2021, 42, 102152 CrossRef.
- H. Teiri, H. Pourzamani and Y. Hajizadeh, Chemosphere, 2018, 197, 375–381 CrossRef CAS PubMed.
- L. De Kempeneer, B. Sercu, W. Vanbrabant, H. Van Langenhove and W. Verstraete, Appl. Microbiol. Biotechnol., 2004, 64, 284–288 CrossRef CAS PubMed.
- I. Alkorta and C. Garbisu, Bioresour. Technol., 2001, 79, 273–276 CrossRef CAS PubMed.
|
This journal is © The Royal Society of Chemistry 2024 |