DOI:
10.1039/D3QI02006A
(Review Article)
Inorg. Chem. Front., 2024,
11, 11-28
The multiple roles of rare earth elements in the field of photocatalysis
Received
1st October 2023
, Accepted 10th November 2023
First published on 20th November 2023
Abstract
When illuminated by light, different electron transitions occur within the energy levels due to the unique 4f energy level structure of rare earth elements. If rare earth elements are introduced into clean and sustainable photocatalysis technology, the electronic transitions in the energy level structure of the catalyst may change, forming a unique reaction mechanism and producing excellent catalytic performance. In recent years, there have been more and more studies and reports on rare earth photocatalysts due to the large number and different properties of rare earth elements. It is essential to collate and summarize these reports to gain a comprehensive understanding of their functions and applications. This article presents a review of the role and application scope of rare earth photocatalysts and provides a broad outlook on their potential future uses. We hope that this work can provide a new perspective for the development and design of novel and efficient rare earth photocatalysts.
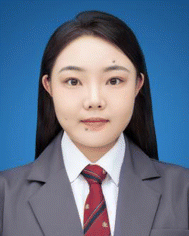 Jing An | Jing An received her B.S. degree in chemistry from Chifeng University in 2021. Now she is a postgraduate student at Heilongjiang University, under the supervision of Prof. Guofeng Wang and Prof. Ruihong Wang. Her current research interests focus on photocatalytic CO2 reduction. |
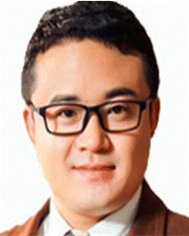 Yang Qu | Qu Yang is currently a full professor at Heilongjiang University. He received his Ph.D. degree in Physical Chemistry from the Institute of Theoretical Chemistry at Jilin University. Since July 2014, he has been working at the Key Laboratory of Functional Inorganic Materials Chemistry, Ministry of Education, at Heilongjiang University. His research mainly focuses on the development of nano-composite oxide-based photocatalysts for environmental applications and the study of their mechanisms in pollutant transformation and control processes. To date, he has published over 50 research papers in high-impact SCI journals such as Angewandte Chemie International Edition, Advanced Materials, Advanced Science, Applied Catalysis B: Environmental, Chemical Engineering Journal, Nano Research, Journal of Hazardous Materials, etc., with more than 3200 citations to his work. Currently, he serves as the editorial board member for Chinese Chemical Letters, Rare Metals, and Eco-Environment & Health (EEH). |
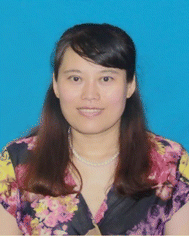 Guofeng Wang | Prof. Guofeng Wang received her M.S. degree in Physics from Northeast Normal University in 2005 and her Ph.D. degree in Electronic Science and Engineering from Jilin University in 2008. She joined the research group of Professor Yadong Li as a postdoctoral associate in 2009. She has been working at Heilongjiang University since 2010. She is the head of the Department of Materials Chemistry of the School of Chemistry and Materials Science at Heilongjiang University. She won the title of excellent postdoctoral student of Tsinghua University. She was awarded the title of “Outstanding Person of the New Century” by the Ministry of Education. |
1. Introduction
As is well known, with the continuous population growth, the demand for non-renewable resources such as coal, oil, and natural gas is rapidly increasing.1,2 However, since fossil energy reserves are limited, they cannot be exploited indefinitely.3,4 It is very important to find sustainable and clean energy to replace fossil energy to maintain normal lifestyles. In 1972, with the proposal of the Honda–Fujishima effect for the first time, it was considered as the beginning of a new era of multi-phase photocatalytic reactions.5 In the following decades, photocatalytic technology has developed rapidly.6–11 People have discovered that photocatalytic reactions play significant roles in many research fields, including photocatalytic CO2 reduction, pollutant degradation, multi-carbon-based material synthesis, water pollution control, and others.12–22 The mechanism of the photocatalytic reaction has also been confirmed by scientists: when a beam of light is irradiated on the semiconductor photocatalyst, the energy of the incident light (hυ) is higher than the forbidden bandwidth (Eg) of the semiconductor, the electrons (e−) in the valence band are excited to transition to the conduction band, and at the same time, the valence band produces holes (h+), and under the action of the electric field, the e− and h+ diffuse to the surface of the catalyst, and the e− and h+ are separated efficiently so that the conduction band and the valence band have the capacity to oxidize and reduce, respectively, which can have a redox reaction with the surface adsorption of the material to produce a product in line with the expectation of the people.23–26 Therefore, photocatalytic reactions are one of the most promising solutions to the environmental and energy crisis through the efficient and rational use of sunlight for redox reactions. However, despite the relatively mature development of photocatalytic technology currently, photocatalysts still commonly encounter many issues, such as insufficient active sites, high photogenerated electron–hole recombination rates, small light absorption ranges, poor product selectivity, etc., which seriously hinder large-scale practical application of photocatalysts.27–32
In 1794, the first rare earth element yttrium (Y) was extracted from an ore, and in the following 150 years, a total of 17 rare earth elements were extracted.33 With the deepening of research, people have discovered that rare earth elements have special electronic structures. The unfilled 4f electron sublayer (4f0–4f14) is shielded by the outermost 5s and 5p orbitals, allowing 4f level electrons to localize within the atom.34 As a result, rare earth elements are capable of producing very rich electronic energy levels and unique optical properties.35 The introduction of rare earth elements into photocatalysts may form unique electronic transitions between the energy levels of the catalyst, greatly improving the activity of the photocatalyst. Since different rare earth elements have different energy level structures, rare earth elements have different roles and effects in different systems and reaction conditions. Due to the varying electronic structures and response to light of each rare earth element, rare earth elements will exhibit unique mechanisms of action and catalytic effects in different catalytic systems. For example, Joanna Nadolna and his colleagues characterized and analyzed TiO2 doped with Er3+, Ho3+, Nd3+, and Tm ions. They analyzed the action mechanism of the catalysts by referring to the energy level structures and excitation paths of the different rare-earth elements, and then further verified it by theoretical calculations and summarized the mechanism of action of lanthanide metals in the catalysts.36 Usually, the doping of rare earth ions can enhance the activity of catalysts in many ways, such as narrowing the band gap of the catalyst, enhancing optical adsorption ability, expanding the light response range, improving the efficiency of electron–hole separation, and forming defect vacancies.37–39 If the energy level structure and mechanism of rare earth elements can be reasonably designed for photocatalytic reactions, the application range of rare earth elements in various fields will be expanded, and more new choices will be provided for the catalytic field. Currently, photocatalytic reactions involving rare earth ions are mainly carried out in four forms: rare earth ion-doped photocatalysts, heterogeneous composite photocatalysts, single atom photocatalysts, and other rare earth based new photocatalysts.40–43 Here, rare-earth ionic photocatalysts reported in recent years are summarized and analysed, and the role of rare-earth photocatalysts in the reaction process is outlined.44–46 The challenges and prospects of rare-earth photocatalysts in practical applications are also discussed, and the specific work is categorized as shown in Fig. 1. We hope that this article will provide new ideas and insights for the rational design of rare earth based photocatalysts.
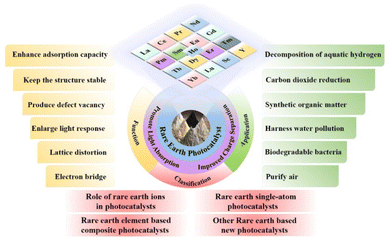 |
| Fig. 1 Role and application areas of rare earth photocatalysts. | |
2. Role of rare earth ions in photocatalysts
2.1 Role of rare earth ions in photocatalysts
Rare earth ion doping in photocatalysts mainly refers to introducing rare earth ions into the lattice of the catalyst, so that the rare earth ions replace other ions or occupy vacancies to form new active sites.47,48 It has been shown that the doping of rare earth ions in photocatalysts can significantly improve the activity of the catalysts.49–51 Among them, rare earth ions in the catalyst mainly play the roles of stabilizing the catalyst structure, adjusting the semiconductor band gap, etc. Here, we summarize several typical action mechanisms as follows.
Doping with rare earth elements can usually maintain the structural stability of the catalyst, while the newly formed chemical bonds can retain the original catalytic activity of the catalyst that was unstable during the preparation process and play a role in the overall bonding structure of the catalyst. For example, Gerardo Colón and his colleagues found that BiVO4 doped with Er3+ can maintain a stable tetragonal crystalline phase.52 Photocatalytic water decomposition was carried out in AgNO3 solution to produce oxygen. The experiment showed that the O2 generation rate of the Er3+ doped BiVO4 sample was 1014 mol h−1 g−1, which is 20 times higher than that of the undoped sample. Researchers analyzed the crystal structure of the catalyst and found that during the synthesis process of BiVO4, Er3+ partially replaced Bi to form ErVO4. Therefore, a mixture of tetragonal and monoclinic phases was formed in the catalyst, and the two heterogeneous structures significantly increased the activity of the catalyst (Fig. 2a and b).52Fig. 2c shows the catalytic mechanism of the reaction; T-BiVO4 serves as an internal sensitizer for m-BiVO4, providing photogenerated photons with sufficient energy. The mixed phase heterostructure formed by this doping is expected to promote the separation of photo-induced electron–hole pairs. Not coincidentally, Katherine Villa and colleagues doped WO3 with La for photocatalytic methane oxidation to produce methanol. WO3 is an ordered mesoporous metal oxide with a large surface area and good light capture performance, which usually leads to the excessive oxidation of the products of photocatalytic reactions to CO.53 However, with the introduction of La atoms, the methanol conversion rate of the catalyst is higher than that of other metal ion doping. Compared with pure WO3, the methanol yield of the catalyst added with the La atom increased by two times, and the CO yield significantly decreased (Fig. 2d).53 This may be due to La avoiding particle sintering during the WO3 calcination process, maintaining the well-ordered mesoporous structure of WO3, thereby improving the photocatalytic activity of the catalyst.
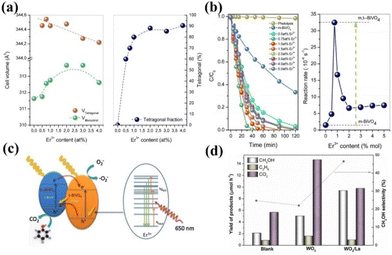 |
| Fig. 2 (a) Evolution of the cell volume and the tetragonal phase fraction with the Er3+ content. (b) Evolution of cell parameters for monoclinic and tetragonal crystalline phases for the Er-BiVO4 series. (c) Envisaged electronic scheme involved in the photocatalytic mechanism upon solar-like excitation.52 Copyright 2014, Elsevier. (d) Yield of products and methanol selectivity in the photocatalytic oxidation of CH4 on blank, WO3 and WO3/La at ∼55 °C under UVC–visible light irradiation. Catalyst dosage is 1 g L−1. Data corresponding to 2 h of irradiation at a continuous methane flow rate of 4.5 mL min−1.53 Copyright 2016, Elsevier. | |
In addition to this, the doping of rare earth ions can create vacancy defects on the surface of the catalyst. Under visible light irradiation, newly formed oxygen vacancies can capture photoelectrons, forming transition states and new energy levels between energy level structures, enhancing the light absorption range and effectively limiting the recombination of photo-generated electrons and holes.54 In addition, the formation of surface defects on the catalyst can also enhance its adsorption capacity for reactants and generate more free radicals, thereby improving photocatalytic activity.55 For example, Kuo's group reported Gd-doped TiO2 nanorods subsequently complexed with poly-o-phthalimide (PoPD) to form a heterojunction photocatalyst for simultaneous oxidation of benzyl alcohol (BA) and reduction of p-dinitrobenzene (p-DNB).56 The results showed that BA was selectively oxidized by photogenerated holes to produce benzaldehyde in 70.5% yield in water and 90.6% yield in acetonitrile solvent. In contrast, p-DNB underwent selective reduction in acetonitrile and water to produce p-nitroaniline (p-NA) in acetonitrile in 89.1% yield and p-phenylenediamine (p-PDA) in water in 85.0% yield (Fig. 3a–d).56 This can be attributed to the doping of Gd3+ induced by the generation of coordination unsaturated sites with unpaired electrons on the surface of the catalyst, forming oxygen vacancy defects. The presence of oxygen vacancies can cause defective energy levels in the band gap, adjust the structure of the energy bands, promote carrier separation, and improve the activity of the photocatalytic reaction (Fig. 3e).56 Zhao's team introduced two doping elements, Ti and Ce, into Nb2O5 photocatalysts and compared them by the performance of photocatalytic selective alcohol oxidation (Fig. 4a).57 The results showed that the performance of Ce-doped Nb2O5 was superior to that of Ti-doped Nb2O5. Researchers attributed this to the fact that Ce doping distorts the Nb2O5 structure less than Ti doping, resulting in the formation of more oxygen vacancies during the catalytic process, as shown in Fig. 4b and c,57 which is beneficial for the interface electron migration process. The presence of oxygen vacancies also increased the adsorption of alcohol molecules on the catalyst and enhanced the photocatalytic performance (Fig. 4d).57 Therefore, exploring rare earth ions compatible with photocatalysts and introducing them into the lattice of the catalysts can lead to a significant increase in the activity of the catalysts.
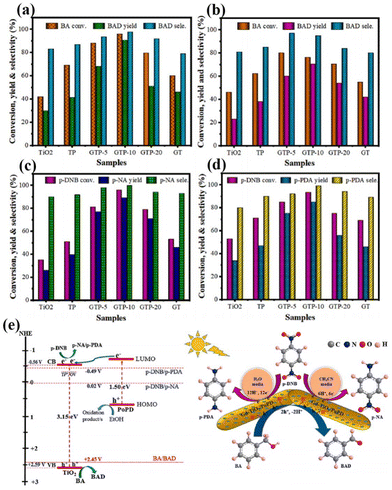 |
| Fig. 3 Selective oxidation of BA (a) in ACN and (b) in water. Selective reduction of p-DNB (c) in ACN and (d) in water. (e) Proposed mechanism for simultaneous oxidation of BA and reduction of p-DNB over GTP-10 under simulated solar light irradiation.56 Copyright 2023, Elsevier. | |
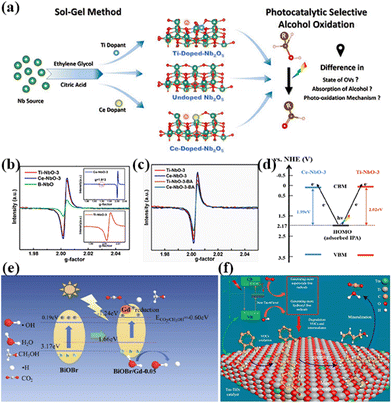 |
| Fig. 4 (a) Proposed mechanism of photocatalytic selective oxidation of alcohols to aldehydes over defective Nb2O5 with deposited Pt NPs. (b) Room temperature EPR spectra of Ti-NbO-3, Ce-NbO-3 and B-NbO (inset graph for 77 K EPR spectra of Ce-NbO-3 (above) and Ti-NbO-3 (below)). (c) Room temperature EPR spectra of Ti-NbO-3 and Ce-NbO-3 before and after adsorbing BA. (d) Schematic representation of the mechanism of the LMCT process in the case of adsorbed alcoholate species and doped Nb2O5.57 Copyright 2021, Elsevier. (e) The possible photocatalytic mechanism and transfer pathway of generated charges in the BiOBr/Gd composite.61 Copyright 2020, Elsevier. (f) Schematic diagram shows the mechanism of improving the photocatalytic activity by Tm-induced 4f levels promoting electron-to-electron transfer transition between Tm and TiO2.62 Copyright 2021, Elsevier. | |
Rare earth ions can not only adjust the structure of a single material but also regulate the light response range of the catalyst. As is well known, the most critical aspect of photocatalysis is that after the catalyst absorbs light energy, the oxidation–reduction ability of h+ and e− generated at the energy level causes the substances adsorbed on the surface to react. The redox ability of photocatalysts depends on the position of their conduction and valence bands; the more negative the conduction band, the stronger the reduction ability, and the more positive the valence band, the stronger the oxidation ability.58,59 In addition, whether a photocatalyst can be stimulated by light to produce h+ and e− pairs is also related to its energy gap (Eg); the narrower the Eg, the wider the light absorption range and the easier it is to be stimulated by light.60 Therefore, modulating the energy band structure of semiconductors is the most common method to broaden the spectral response range. For example, Hou and his colleagues doped Gd3+ onto BiOBr microspheres by in situ synthesis.61 The product catalysts were tested for photocatalytic CO2 reduction, with the most active BiOBr/Gd-0.05 methanol yielding up to 123.711 μmol g−1, which is a 5-fold increase in photocatalytic activity compared to pure BiOBr. It was shown that the doping of Gd changed the valence band of BiOBr from 3.17 to 1.66 eV and the conduction band from 0.19 eV to −1.24 eV, making the photocatalytic reduction of CO2 to methanol (−0.60 eV) possible. At the same time, the energy gap of the catalyst was narrowed, which broadened the visible light response range and improved the activity of the photocatalyst. The free radicals ˙CO2− can capture a photo-generated electron and convert it into HCOOH, which is then reduced to CH3OH, as shown in Fig. 4e.61 Sun's team synthesized Tm3+-modified TiO2 catalysts for organic pollutant degradation using a sol–gel method.62 The results showed that the catalyst significantly improved the gas-phase degradation of acetaldehyde, o-xylene, and their mixtures compared to pure TiO2. The photocatalytic activity was gradually enhanced with the increase of the Tm concentration. Among them, the photodegradation efficiency of the 0.5 mol% Tm-TiO2 sample for acetaldehyde and o-xylene could reach up to 93.1%. The researchers concluded that the improved photocatalytic performance was mainly attributed to the doping of the 4f energy level of Tm between the band gap of TiO2, which generated new redox sites for the effective separation of photogenerated electrons and holes, as well as increased adsorption capacity for acetaldehyde and o-xylene (Fig. 4f).62 In addition to changing the energy level structure of the catalyst, rare earth elements can also act as electron transfer bridges, enabling the directional transfer of photogenerated electrons to the active center of the catalyst, enhancing reactant activation, effectively inhibiting electron and hole recombination, and significantly improving the activity of the catalyst. Liu and his colleagues doped C and Y into the lattice of TiO2 for photocatalytic methyl blue degradation.63 It was shown that the degradation rate of methyl blue on (C, Y)-co-doped TiO2 samples was much higher than on other undoped samples. This can be attributed to the synergistic effect of C and Y doping, which narrows the energy gap of TiO2 from 3.2 eV to about 2.75 eV, and broadens the absorption band edge into the visible light region. When semiconductor nanomaterials are modified with different dielectric materials, a phenomenon occurs: their optical properties are strongly influenced by the surface environment, resulting in dielectric confinement effects. In addition, the doping of Y formed a doping energy level on TiO2, which made the electron transitions and transfers easier and could effectively inhibit the recombination of photogenerated electron/hole pairs. Meanwhile, the doping of Y can inhibit the growth of TiO2 particles, increase the specific surface area, and enhance the photocatalytic activity. Not coincidentally, Xu and his colleagues anchored elemental Sm and Ag quantum dots on CeO2 for photocatalytic water decomposition for hydrogen production.64 Compared with pure CeO2, Sm doping significantly increases the number of CeO2 oxygen vacancies, which can stimulate photogenerated electrons to form transition states closer to the CB under visible light irradiation, thereby reducing the bandgap of related products and effectively suppressing photogenerated electrons and holes. Meanwhile, the bandgap of CeO2 narrowed after rare earth ion doping, and the estimated bandgap values of CeO2, Sm@CeO2, Ag@CeO2, and Ag/Sm@CeO2 were 2.96, 2.91, 2.86, and 2.82 eV, respectively, which indicated that the doping of Sm was able to broaden the response range of light. The results showed that the H2 yield of the catalyst reached 342.12 μmol g−1 h−1, which was 6.83 times higher than the value of CeO2, and a CeO2 photocatalyst with high visible light catalytic performance was realized. In addition, the catalyst has better reproducibility, indicating that the doping of Sm3+ makes the structure of the catalyst more stable. In subsequent experiments, the researchers found that the catalyst liquid medium also exhibited high photocatalytic properties, such as the oxidation of bisphenol A (BPA) and the reduction of 4-nitrophenol (4-NP). Wang's group doped Er3+ and Eu3+ into CaTiO3 catalysts for comparison of their photocatalytic hydrogen production performances, respectively.65 The results showed that CaTiO3:Er3+ nanocrystals had the highest photocatalytic activity of up to 461.25 μmol h−1 under UV irradiation, which was higher than that of CaTiO3:Eu3+ nanocrystals and pure CaTiO3. The results of UV diffuse reflectance spectroscopy indicate that all sample absorption band edges are in the ultraviolet region (λ < 400 nm), which means that the doping of rare earth ions reduces the bandgap width of CaTiO3 from 3.5 eV to 3.3 eV, expanding the range of optical response. In addition, the doping of Er3+ resulted in enhanced light absorption at 400–700 nm in CaTiO3 compared to Eu3+-doped CaTiO3, which may be attributed to the special molecular orbital structure of Er3+ that gives rise to a unique mode of electron migration in the catalysts.
Wu's team tested the antimicrobial effects of TiO2 nanoparticles co-doped with Ce and Er against Staphylococcus aureus and Escherichia coli using the sol–gel method.66 The results showed that the photocatalytic activity of the catalysts first increased and then decreased with the increase of Er and Ce doping contents. When the Er and Ce doping doses were 0.5 mol% and 0.2 mol%, the 0.5Ce0.2Ti-O calcined at 800 °C had the best antimicrobial performance, with the antimicrobial efficiencies against Staphylococcus aureus and Escherichia coli of 91.23% and 92.8%, respectively. The UV-visible absorption spectra show that the absorption edge of the co-doped samples shows a significant redshift compared to the untreated TiO2, indicating that the doping of Er and Ce significantly reduces the bandgap of the catalyst, which is related to the incomplete occupation of the 4f and 5d orbitals of the rare earth ions. Special electronic orbitals not only reduce the band energy gap but also suppress the electron–hole recombination rate by providing new energy levels, which can effectively improve their photocatalytic performance. Therefore, the doping of Er3+ successfully converted the near-infrared radiation into the visible region, and the incorporation of Ce3+ also effectively expanded the spectral response range and improved the photocatalytic activity of co-doped TiO2. In summary, rare earth ion doping can change the valence band and conduction band of the catalyst, reduce the band gap width, and expand the light response range. In addition, due to the unique energy level structure of rare earth ions, they can also adjust the response of light of different wavelengths. Therefore, exploring suitable rare earth ion photocatalysts to improve the light energy utilization of sunlight will be a breakthrough in the field of photocatalysis.
2.2 Rare earth element based composite photocatalysts
Rare earth elements can also act as electronic additives to the catalyst. The combination of two semiconductor materials with different band structures will form a potential difference, and the electrons generated by light excitation will be transferred from one semiconductor to another semiconductor, effectively separating electrons and holes.67–72 For example, Zhang's team co-doped Yb3+ and Er3+ on BiOBr and complexed it with Bi4O5I2 to form a Z-type heterojunction catalyst for two-dimensional materials.73 The catalyst exhibited excellent performance in degrading BPA under full-spectrum and NIR light irradiation. Within 30 min, the degradation rate of BPA reached 100%, which was much higher than the performance of the catalyst before combining. This can be attributed to the fact that the doping of Yb3+ and Er3+ can capture near-infrared (NIR) light, which can then be converted to visible light by the upconversion function of rare earth ions, expanding the optical response range of the photocatalytic system. Meanwhile, the heterogeneous structure formed made it possible to generate active radicals ˙O2− and ˙OH by a redox reaction on the catalyst, which effectively inhibited the recombination of photogenerated electrons and holes, and significantly improved the photocatalytic activity. Wang and his colleagues doped Eu3+ on BiVO4 nanoplates, which were then coupled with CeOx nanoparticles to form -type-II heterojunctions for photocatalytic conversion of CO2 to CH4 and degradation of 2,4-dichlorophenol (2,4-DCP).74 The results showed that CeOx/BiVO4:Eu3+ nanoparticles possessed the most visible-light photocatalytic performance, while the photocatalytic degradation of 2,4-DCP by 2C/BVO-2 was 62.5%, which was higher than the degradation rate of 21.3% on BVO and 33.3% on BVO-2. This can be attributed to the fact that the doping of Eu3+ changes the electron-transition structure of the catalyst and promotes charge separation. When the catalyst is excited by a visible light source, the photoelectrons produced by BVO-2 can transition to the conduction band of CeOx. Meanwhile, the doping of Eu3+ increases the number of electrons excited by visible light (498 < λ ≤ 530 nm), so that high-energy electrons are transferred from BVO-2 to the conduction band of CeOx, giving the nanomaterials enough energy to promote oxidation and reduction reactions. In general, no matter which kind of heterojunction is formed, it will lead to effective separation of charges and holes. Therefore, the doping of rare earth ions into the catalyst and the formation of a new energy level structure as a channel connecting the electron transfer of the two semiconductor materials is an important breakthrough in the preparation of high-performance composite catalysts.
2.3 Rare earth single-atom photocatalysts
As is well known, the smaller the particle size of a photocatalyst, the shorter the time it takes for the electron–hole pair to reach the surface. The better the redox ability of semiconductors, the higher the catalytic activity or selectivity of catalysts. Therefore, people have always been committed to the synthesis of catalysts with small particle sizes and highly dispersed.75 In 2011, academician Tao Zhang's group at the Dalian Institute of Chemical Technology first proposed the concept of single-atom catalysis, bringing catalytic science to a smaller research scale, and the emergence of single-atom catalysts can maximize the catalytic efficiency of precious metals and reduce the cost of manufacturing for highly loaded metal catalysts.76–82 Therefore, the ideal state of loaded catalysts is to uniformly distribute the metal in the form of single atoms on the carrier. With the deepening of the research, it has been gradually discovered that single-atom catalysts can have excellent catalytic properties triggered by the reaction, such as sharply increasing the surface free energy, quantum size effect, unsaturated coordination environment, metal–carrier interactions, etc. These unique properties enable single-atom catalysts to have excellent catalytic performance.83 If rare earth elements are introduced into the structure of photocatalysts in the form of single atoms, and the unique 4f energy level of rare earth single atoms is reasonably utilized, the catalysts can take advantage of the dual advantages of the energy level of rare earth elements and the efficiency of single atoms, which will promote the entirely new discovery in the field of photocatalysis and further broaden the scope of application of rare earth photocatalysts.84
For example, Zhang and his colleagues doped La single atoms on MoO3−x for photocatalytic nitrogen fixation using the solvothermal method.85 The results showed that La single atoms underwent a strong bonding interaction with MoO3−x to form O2c–La–O2c bonds, and the La single atoms as active centers could enhance the adsorption of N2 on the surface and make the overall structure of the catalyst more stable. At the same time, the 5d orbital of La can spontaneously provide electrons to the orbitals of molecules with adsorbed N2, allowing the activation of the inert N
N bond to produce a continuous hydrogenation to synthesize NH3. In the absence of a sacrificial agent, the catalyst showed an NH3 production rate of 209.0 μmol g−1 h−1 under visible light. The researchers attributed it to the doping of La single atoms that optimized the electronic properties of the MoO3−x carrier and enhanced the surface adsorption activation of the catalysts, which facilitated the photocatalytic nitrogen fixation for NH3 production (Fig. 5a and b).85 Not coincidentally, Wang's team synthesized catalysts with controllable single-atom density dispersion properties (Er1/CN-NT) by loading rare-earth single-atom Er onto carbon nanotubes using a novel atomic confinement and coordination strategy (Fig. 5c).86 Meanwhile, the catalytic activity of the catalyst was excellent. The yields of CO and CH4 were 47.1 and 2.5 μmol g−1 h−1, respectively, under the conditions of pure water (Fig. 5d–f).86 The results of synchrotron radiation photoelectron spectroscopy and theoretical calculations showed that the presence of Er single atoms could improve the adsorption capacity on the catalyst surface. Meanwhile, the electronic structure of the Er single atom as the active center of the catalyst is closely related to the processes of adsorption, activation, and photocatalytic CO2 reduction, suggesting that rare earth ion-doped photocatalysts can significantly improve the activity of the catalytic reaction. Zhou's team used a single-atom engineered oxygen coordination strategy to successfully dope Pr single atoms with oxygen coordination on CN (Pr1-N4O2/CN), which was used to achieve the efficient photocatalytic reduction of CO2 to CH3OH (Fig. 6a).87 The experimental results show that the CH3OH yield of this catalyst is 511.1 μmol g−1 h−1, which is 28.9 and 1.9 times higher than that of CN and Pr1–N6/CN, respectively, and this performance outperforms most of the other metal-ion doped ones reported (Fig. 6b–e).87 The researchers concluded that Pr1-N4O2 induced the accumulation of photogenerated electrons as an active center and increased the photogenerated electron density in the active site. In addition, the Pr-loaded active center could promote the adsorption and activation of CO2 and provide high-density active sites for CO2 photoreduction (Fig. 6f).87
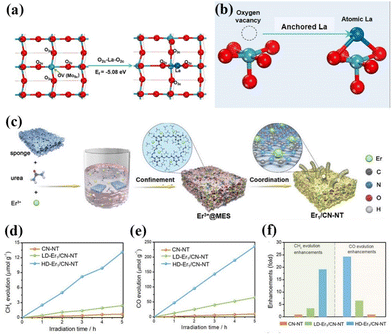 |
| Fig. 5 (a) Top view of the MoO3−x structure and the optimized configuration of the La atom at the terminal oxygen vacancy site. (b) Schematic illustration of a single La atom anchored on the oxygen vacancy site.85 Copyright 2022, Elsevier. (c) Schematic process of the synthesis of single-atom Er1/CN-NT catalysts. The evolution rates of CH4 (d) and CO (e) during the photocatalytic CO2 reduction with H2O over LD-Er1/CN-NT, HD-Er1/CN-NT, and CN-NT catalysts. (f) Enhancement of product evolution over the LD-Er1/CN-NT, HD-Er1/CN-NT, and CN-NT catalysts.86 Copyright 2020, Wiley-VCH. | |
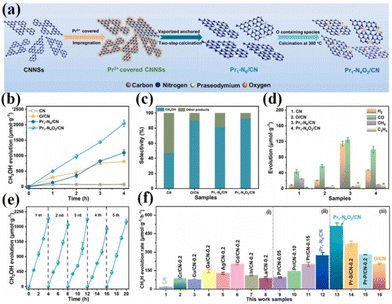 |
| Fig. 6 (a) Schematic of the synthesis process of Pr1–N6/CN and Pr1-N4O2/CN. (b) CH3OH production with time for CN, O/CN, Pr1–N6/CN, Pr1-N4O2/CN. (c) The selectivity of CH3OH and other products. (d) H2, CO, CH4, and C2H4 production in 4 h. (e) CH3OH production corresponding to cycle experiments (∼20 h) for the Pr1-N4O2/CN. CH3OH production of the reference photocatalysts synthesized in this work, such as (f) CN supported different kinds of metal elements, (ii) CN supported Pr single-atom with different densities, and (iii) CN supported Pr single-atom with different coordination configurations.87 Copyright 2023, Elsevier. | |
In addition to the above-mentioned rare-earth single-atom photocatalysts that can be used as active sites of the reaction to directly contact the reactants, rare-earth single atoms can also improve the activity of the reaction by indirectly coming into contact with the reactants, such as the formation of vacancy defects, free radicals, and so on. For example, Wang's group used a hydrothermal method to introduce La single-atoms on P/InVO4 for photocatalytic CO2 reduction.88 In the absence of any sacrificial agent, the CO yield was 11.96 μmol g−1 h−1 with a selectivity of about 100%. The test results showed that it was the doping of La single-atoms that led to a significant increase in the activity and selectivity of the catalysts, and then the results of theoretical calculations demonstrated that La single-atoms increased O defects in the catalysts, modulated the active sites on the surface of the catalysts, and promoted the adsorption of CO2 on the surface of the catalysts. As shown in Fig. 7a, two single component samples recombine to form a Z-type heterojunction, and CO2 is reduced to CO and CH4 under visible light irradiation.88 Wang and his colleagues loaded La single-atoms onto g-C3N4 by solvothermal and high-temperature reduction methods and tested the degradation performance of the SALa/CN photocatalytic system using the total organic carbon (TOC) characterization method.89 The results showed that SALa/CN exhibited a good removal rate (73.27%) of TC (tetracycline hydrochloride) within 30 min. The higher mineralization activity indicated that SALa/CN was capable of converting TC into non-polluting small molecules such as CO2 and H2O. The catalyst was then subjected to photocatalytic degradation experiments of oxytetracycline hydrochloride (OTC) and doxycycline hydrochloride (DOX). The photocatalytic degradation efficiencies of the SALa/CN photocatalyst for OTC and DOX reached 84.49% and 91.71%, respectively, within 30 min. It is shown that SALa/CN has good photocatalytic activity for the removal of antibiotics. The researchers attributed such high photodegradation activity to the effective separation of electrons and holes due to the doping of La single atoms, which improves the utilization of visible light. Meanwhile, the doping of La single-atoms provides a new active site and a special coordination structure, which enables the catalyst to reduce O2 to the active species O2− more often and remove pollutants effectively.
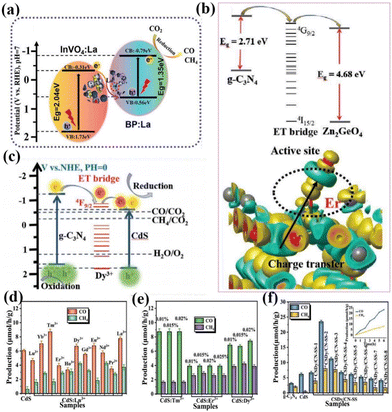 |
| Fig. 7 (a) Mechanism of photocatalytic CO2 reduction under visible light irradiation.88 Copyright 2022, Wiley-VCH. (b) The proposed mechanism diagram of photocatalysis.92 Copyright 2021, Wiley-VCH. (c) The proposed mechanism diagram of photocatalysis. Production of CO and CH4 from the CO2 photoreduction systems without a sacrificial agent for the photocatalytic reaction: (d) CdS:0.015%Ln3+, (e) CdS:Tm3+. CdS:Er3+, and CdS:Dy3+ with different doping concentrations, (f) g-CN and CdS:Dy3+@g-CN (CSDy/CN-SS) prepared by in situ synthesis methods (the inset shows the photocatalytic production rates).93 Copyright 2021, Wiley-VCH. | |
In addition, rare earth single atoms can form unique charge transfer channels within single and composite catalysts, and the formation of such electron transfer bridges largely improves the charge separation efficiency and enhances the reactivity of photocatalysts. For example, Dong and her colleagues immobilized La single atoms onto a g-C3N4 layer for photocatalytic CO reduction.90,91 The results showed that the electronic structure of the La–N bridge resulted in a CO yield of 92 μmol g−1 h−1 and a CO selectivity of 80.3%, which is superior to most CN photocatalysts. This can be attributed to the unique 4f and 5d orbital La single atoms that enable the electronic state of the catalyst to be changed. Meanwhile, the hybridization of the p–d orbitals of the La–N structure induces the formation of La–N electron-directed transfer bridges on the catalyst, which improves the charge separation efficiency. At the same time, the presence of La single atoms changed the energy level structure of the catalyst, which led to the expansion of the photoresponsive range of the catalyst. Subsequently, the researchers demonstrated that rare earth ions, as the key active centers of the whole catalyst, can significantly activate CO2 and promote the desorption of CO, thus improving photocatalytic efficiency. Wang's group composited Er single atom doped g-C3N4 with ZnGeO4 by in situ synthesis to obtain a photocatalytic heterojunction of type-II, which combines the dual advantages of rare-earth single atoms and heterostructures to make ZnGeO4 responsive in the visible light range (Fig. 7b).92 At the same time, the activity of the catalyst was made to be significantly enhanced relative to the semiconductors alone, and a series of experimental results showed that the 4f energy level of Er3+ could act as an electron transport bridge between the two semiconductors to enable the effective separation of photogenerated electrons. In subsequent work, Wang's team introduced several different rare earth ions into CdS for photocatalytic CO2 reduction (Fig. 7c).93 The results showed that Dy3+ doping could lead to optimal photocatalytic performance of the catalyst. Subsequently, the researchers carried out a composite trip heterojunction of Dy-doped CdS with CN. In the absence of a sacrificial agent, the CO yield was 23.44 μmol g−1 h−1 and the CH4 yield was 8.06 μmol g−1 h−1, which were superior to other metal ion doped photocatalysts (Fig. 7d–f).93 Through characterization and theoretical calculations, it is shown that the doping of Dy changes the density of the surrounding electron cloud, prompting Dy3+ to act as an electron-transport bridge in two bandgap-matched semiconductors, effectively suppressing the recombination of photogenerated electrons and holes. It can be seen that the chemical bond formed by rare earth doping can increase the density of photogenerated electrons in the active site, so that the photogenerated electrons can be transferred and enriched directionally to the active site of the catalyst, and the effective transfer of energy can be improved, so that the photogenerated electrons and holes can be effectively separated.
2.4 Other rare earth based new photocatalysts
Rare-earth oxides usually have the advantages of strong adsorption selectivity, good thermal stability, electron-type conductivity, and abundance of oxygen vacancies, and thus have received much attention. However, with continuous research, it has been found that a single rare earth oxide photocatalyst has many drawbacks, such as a wide band gap, a small light absorption range, and a high recombination rate of electrons and holes.94–96 Therefore, pure rare earth oxides are rarely used in practical applications. For example, Karunakaran and his coworkers compared the photocatalytic degradation of 1-naphthol by TiO2, ZnO, CeO2, CdO, WO3, Co3O4, Sb2O3, ZrO2, La2O3, Y2O3, Pr6O11, Sm2O3 and Al2O3.97 The results showed that the degradation of these oxides follows the Langmuir–Hinshelwood kinetic model (Fig. 8a).97 However, the performance of the catalysts was significantly different among them, TiO2 and ZnO were the most effective in degrading 1-naphthol, and the effects of the other oxide catalysts were not significant compared with those of TiO2 and ZnO. Although many efforts have been devoted to tuning the intrinsic surface properties of the catalysts, controlling the specific morphology with different surface exposures is essential for improving the catalytic performance of the catalysts. However, pure rare earth oxide photocatalysts still show limited catalytic activity and selectivity. As a result, rare earth oxide photocatalysts have been modified to overcome their drawbacks using methods such as metal ion doping and semiconductor combining. Janardhan Reddy Koduru's team composited graphene oxide (GO) with Gd2O3 for photocatalytic water pollution treatment for arsenic (As) removal.98 Characterization results showed that the composite of Gd2O3 significantly increased the catalyst surface area and increased the stability of the catalyst. Meanwhile, the adsorption capacity of the catalyst for As could reach up to 216.70 mg g−1. In addition, the GO-Gd2O3 nanocomposites showed a high bacterial photocatalytic deactivation rate. It shows that the composite of rare earth oxides can significantly improve the catalytic effect of the catalysts. Liu's team synthesized composites of ScO and CN for photocatalytic oxidation of organic pollutants by in situ calcination.99 It was shown that under visible light irradiation, the photogenerated electrons of CN migrated onto ScO through the Sc–C channel, which improved the charge separation and photogenerated electron transfer of the catalyst. Meanwhile, the surface-active sites provided by Sc atoms enhanced the adsorption and activation of PMS, which can further improve the rapid oxidation of organic pollutants and activation of PMS, and increase the catalytic activity of the catalysts.
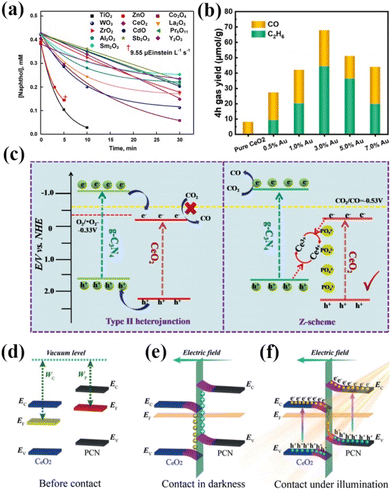 |
| Fig. 8 (a) Decay of 1-naphthol with the illumination time. 0.10 g of oxide loading, 7.8 mL s−1 airflow, 19.2 mg L−1 dissolved O2, 365 nm, 19.2 μEinstein L−1 s−1, 20 mL of naphthol solution, and CO2 photoreduction properties of samples.97 Copyright 2021, Elsevier. (b) CO and C2H6 formation yields as a function of the Au content of ACEO-x.102 Copyright 2023, Elsevier. (c) Schematic diagram of the photoinduced electron–hole separation process for the P-CeO2/g-C3N4 Z-scheme heterojunction.103 Copyright 2021, Elsevier. (d) The internal electric field with band-edge bending near the interface of CeO2/PCN. (e and f) The S-scheme transfer mechanism of photogenerated electrons under illumination.104 Copyright 2020, Wiley-VCH. | |
Tian and his colleagues synthesized a novel S-doped In2O3–CeO2 heterojunction for photocatalytic CO2 reduction.100 The results showed a CH4 yield of 60.6 μmol g−1 h−1 in the absence of a sacrificial agent with a selectivity of 92.4%, which is much higher than the activity of other common catalysts. The researchers concluded that the synergistic effect of heterojunction formation and catalyst surface defects resulted in effective transfer and separation of photogenerated carriers, enhanced visible light utilization and CO2 adsorption, and expanded catalytic active sites. Currently, there are relatively few areas of research on rare earth oxide photocatalysts. Among them, CeO2 has become the most widely researched and applied rare earth oxide photocatalyst due to its reversible Ce3+/Ce4+ redox pair, abundant oxygen vacancies, etc.101 Shen's team doped Au into CeO2 to form nanocomposites with Au–O–Ce sites for the highly selective conversion of photocatalytic CO2 to CH3CH3.102 The results showed that Au doping significantly improved the conversion of CO2 with an ethane production rate of 11.07 μmol g−1 h−1 and a product selectivity of 65.3%, which is 42.85 times higher than that of pristine CeO2 (Fig. 8b).102 This can be attributed to the better adsorption of CO2 at the Au–O–Ce active sites on the composites than at the oxygen vacancies on CeO2. Meanwhile, the doping of Au reduces the bandgap width of the catalyst, which enlarges the photoresponse range of the catalyst and improves the catalytic activity. Dong and his team utilized phosphate-modified octahedral CeO2{111} and complexed it with g-C3N4 for a photocatalytic CO2 reduction reaction.103 The results showed that the CO production rate of the catalyzed reaction was 4.184 μmol g−1. Subsequently, the researchers demonstrated that interfacial electrons were transferred from g-C3N4 to CeO2via a phosphate bridge, forming a Z-type heterojunction instead of a type-II heterojunction (Fig. 8c).103 In addition, the phosphate modification increased the active sites for CO2 adsorption. As a result, it led to a significant improvement in the performance of P-CeO2/g-C3N4. Zhang's team used in situ wet chemistry and subsequent heat treatment to composite polymer CN with CeO2 to design a 0D/2D S-type heterojunction for visible light photocatalytic inactivation of bacteria.104 The catalyst showed a photocatalytic bactericidal rate of 88.1% against Staphylococcus aureus under visible light irradiation, which was 2.7 and 8.2 times higher than that of CeO2 and PCN, respectively. Researchers believe that in the dark, CeO2 obtains electrons and the band edge bends downwards, resulting in negative charges in the nearby interface area, as shown in Fig. 8e. Therefore, an internal electric field is established at the interface of the heterojunction, which hinders the continuous flow of electrons. Under illumination, excited electrons transfer from CeO2 to PCN under an internal electric field and Coulomb interactions, forming an S-type heterojunction, as shown in Fig. 8f. The formation of this structure promotes the separation and transfer of photo-generated charge carriers, greatly improving the photocatalytic and antibacterial properties of CeO2/PCN heterojunction materials. Li and her colleagues constructed a Cuδ+/CeO2–TiO2 photocatalyst for photocatalytic CO2 conversion to C2H4.105 The results showed that the catalyst produced C2H4 at a rate of 4.51 μmol gcat−1 h−1 under simulated sunlight with a selectivity of 47.5% and an electron utilization selectivity of 73.9% for C2H4. The researchers concluded that the heterogeneous structure enhances charge separation and transfer, the Cuδ+ generated as a reflective active site stabilizes the intermediate, and the synergistic effect of multiple active sites improves the conversion and selectivity of the C2 product. In summary, rare-earth oxides can improve the catalyst activity by doping metal ions and composite semiconductors to form heterojunctions. However, so far, the real structure of rare earth oxide photocatalysts and their mechanisms in catalytic reactions is not clear. Therefore, future studies should pay more attention to the application mechanism of pure rare earth oxide photocatalysts.106
With the increasing research on rare earth oxides, many novel photocatalysts have been gradually discovered, such as Dy2Ce2O7, Nd2Sn2O7, La2Ti2O7, and so on.107–114 These new rare-earth oxide photocatalysts can usually be applied to the photocatalytic reaction alone. For example, Sahar Zinatloo-Ajabshir used the Dy2Ce2O7 photocatalyst for visible light decontamination of organic dyes (methyl orange, rhodamine B, and 2-naphthol pollutants) in water.115 The results showed that Dy2Ce2O7, calcined at 400 °C using grape juice as a catalyst, had good photocatalytic performance for the degradation of methyl orange, rhodamine B, and 2-naphthol pollutants under visible illumination. The degradation efficiency was 94.3% for rhodamine B and 92.4% for methyl orange, and the degradation efficiency of the 2-naphthol pollutant was 96.8% for sample 5 after 20 min of light exposure. This can be attributed to the fact that grape juice acts as a green surface covering agent and reducing agent in the catalytic process, and this green and non-polluting material brings new ideas for photocatalytic degradation. Subsequently, the group successively applied the synthesized Nd2Sn2O7 nanostructures to degrade eosin Y, infrared black T, and methyl violet pollutants in visible light and the erythrosine contaminant in ultraviolet light. Nd2Sn2O7 showed significant degradation properties.116,117 By coincidence, Wang and his colleagues successfully synthesized a series of cerium zirconium oxide (CexZryO2) nanocomposites using a hydrothermal method for photocatalytic sulfonamide performance testing.118 Among them, element Ce mainly exists in the form of Ce3+ and Ce4+, and element Zr exists in the form of Zr4+. The researchers found the optimal content ratio for the catalytic reaction by adjusting the content of Ce and Zr. The results showed that the Ce0.9Zr0.1O2 composite had the best photocatalytic performance under simulated sunlight, with an efficiency of 91.33% for the degradation of formamide within 30 min, which can be attributed to the large production of ˙O2− and ˙OH radicals. Despite the initial applications of novel rare earth oxides, most of the novel rare earth oxide photocatalysts reported so far have very wide band gaps (>3.5) and have no catalytic activity under visible light. Therefore, to efficiently develop and utilize rare earth oxide photocatalysts, they also need to be rationally photocatalytically modified.119–125 Generally speaking, the modification of rare earth oxide photocatalysts is similar to that of other catalysts, which mainly includes two aspects: broadening the light absorption range of photocatalysts and improving the separation efficiency of photogenerated carriers. Among them, broadening the light absorption range of photocatalysts is mainly realized by metal ion doping. For example, Zhang and her colleagues combined Au nanorods with La2Ti2O7 (Au-LTO NSP) to form a surface heterojunction-based plasma photocatalyst for photocatalytic hydrogen production in visible and near-infrared regions.126 The composites showed excellent hydrogen production performance when the light illumination wavelength was greater than 420 nm and 70 nm, respectively. The high apparent quantum efficiency (AEQ) of the catalyst was 1.4% under 920 nm light illumination, indicating that the catalyst could absorb infrared light efficiently. The researchers believe that this is due to the formation of a surface heterojunction between Au nanorods and La2Ti2O7, where a large number of photogenerated electrons are transferred from Au to the (010) surface, and then directionally migrate to the (012) surface of La2Ti2O7. The unique step structure of La2Ti2O7 retards the recombination of photogenerated electrons and holes in the Au nanorods, which proves that the semiconductor surface heterojunction facilitates interfacial electron transfer for efficient photocatalytic reactions (Fig. 9a).126 Tsunehiro Tanaka and his coworkers doped Ag in La2Ti2O7 with a layered chalcogenide structure for photocatalytic CO2 reduction in pure water.127 CO, H2, and O2 were produced at rates of 5.2, 4.9, and 5.3 mol h−1, respectively, with CO being the major product. With the increase of the calcination temperature and time, the grain size of La2Ti2O7 increases and the surface area decreases, and water acts as an electron donor for the photocatalytic conversion of CO2. The researchers maximized the photocatalytic activity of La2Ti2O7 by controlling the size and surface area of the grains. Yusuke Asakura and his colleagues obtained nitrogen-doped La2Ti2O7 for photocatalytic NOx oxidative decomposition by treating La2Ti2O7 nanocrystals in by treating them under an ammonia flow at 600–750 °C.128 The structures, optical properties, and photocatalytic activities of the catalysts with different nitrogen doping amounts were also compared (Fig. 9c).128 It is shown that the doping of N extends the light absorption range of the wide bandgap UV material La2Ti2O7 into the visible region, and the increase in the specific surface area leads to the enhancement of photocatalytic oxidation activity of NO gas (Fig. 9d).128 Not coincidentally, Zhang's team utilized Pt/La2Ti2O7 nanoplates for the photocatalytic reduction and defluorination of perfluorooctanoic acid (PFOA).129 The results showed that methanol acted as a sacrificial reagent and electron donor for the catalytic reaction, and the photocatalytic degradation rate of PFOA was significantly improved by reducing PFOA by 40% in the range of 180 min under UV254 irradiation (1 mW cm−2). This can be attributed to the enhanced separation of photogenerated electrons from holes by the doping of Pt, which broadens the photoresponsive range of the catalyst and improves photocatalytic activity. Huang's team used a sol–gel method to synthesize La2Zr2O7/rGO catalysts for the photocatalytic degradation of tetracycline under visible light.130 It was shown that the removal rate and reaction rate of LZO/rGO-3 were 82.1% and 0.3097 min−1, respectively, after 40 min, which were significantly higher than that of La2Zr2O7. The researchers attributed this to the doping of graphene which added more adsorption and reaction sites on the catalyst surface. Meanwhile, the doping of graphene oxide reduced the band gap of the catalyst and expanded the light absorption range (Fig. 10a and b).130 In addition, the doping of graphene formed vacancy defects, which enabled the effective separation of electrons and holes and further promoted the generation of active substances (Fig. 10c and d).130 Overall, doping metal ions in novel rare earth oxides can reduce the band gap width of the catalysts and expand the photoresponsive range while increasing the active sites of adsorbed reactants and improving the catalytic activity.
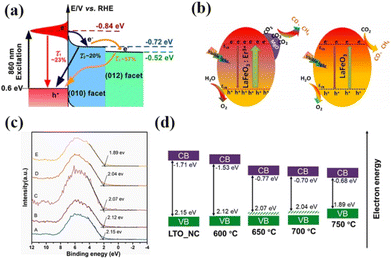 |
| Fig. 9 (a) Schematic illustration of the three different electron decay processes in the Au-LTO NSP system.126 Copyright 2018, ACS Catal. (b) The reaction mechanism for photoreduction of CO2 by H2O on MgO/LaFeO3:Er3+ and LaFeO3 under visible-light irradiation.140 Copyright 2020, Wiley-VCH. (c) Valence band spectra vs. Fermi level and (d) schematic illustration of the band structure diagram of LTO-NC before and after heat treatment in NH3 gas flow at different temperatures (600–750 °C) (the valence band energy and conduction band energy is vs. Fermi level).128 Copyright 2021, Elsevier. | |
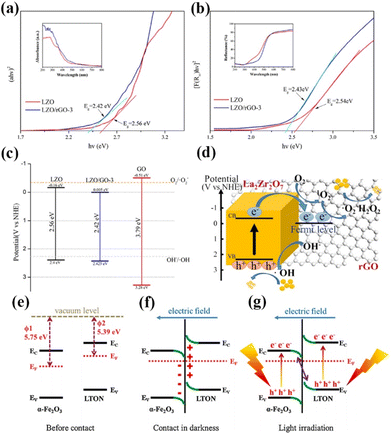 |
| Fig. 10 (a) Tauc plot (ahv)2 − hv curves of LZO and LZO/rGO-3; the inset shows UV–vis absorption spectra. (b) Kubelka–Munk plot (F(R∞)hυ)2 − hv curves of LZO and LZO/rGO-3; the inset shows UV–vis diffuse reflectance spectra. (c) Band gap, valence band and conductive band of LZO, GO and LZO/rGO-3; (d) photocatalytic mechanism of LZO/rGO.130 Copyright 2020, Elsevier. (e) The work functions of α-Fe2O3 and LTON before contact. (f) The internal electric field and band edge bending at the interface of α-Fe2O3/LTON after contact. (g) The Z-scheme charge transfer mechanism between α-Fe2O3 and LTON under light irradiation.141 Copyright 2021, Elsevier. | |
Since these new rare-earth oxides are usually compounded by reacting rare-earth oxides with other compounds, these catalysts usually have the multiple advantages of rare-earth elements and other compounds.131–134 Among them, the most widely studied is the combination of rare-earth oxides with chalcogenides, which gives the catalysts a variety of advantages, such as special electron-transitioning properties, high stability, and non-toxicity.135–139 However, owing to its disadvantage of high charge sufficiency, if the new rare earth oxide photocatalyst is compounded with other semiconductors, both efficient charge separations can be realized. For example, Wang's group doped Er into LaFeO3 and composited it with MgO to test its photocatalytic CO2 reduction ability under visible light.140 The experimental results showed that the Er-doped composites have better CO2 adsorption capacity and higher carrier separation efficiency, and the researchers attribute the enhancement of the catalyst photoactivity to the inhibition of the recombination of photogenerated electrons and holes by the presence of Er3+, which leads to a significant increase in the electron–hole separation ratio (Fig. 9b).140
Su's team complexed LaTiO2N with α-Fe2O3 for photocatalytic CO2 reduction.141 In the absence of a sacrificial agent, the CO and methane yields were 29.0 and 38.0 μmol g−1, respectively, with a total utilized photoelectron number (UPN) of 362.0 μmol g−1 after 3 h of light exposure, which was significantly better than that of the original LaTiO2N photocatalyst. The researchers believe that this is due to the formation of Z-type heterojunctions that enable efficient separation and transfer of photogenerated electrons and holes, resulting in an increase in the overall redox capacity of the catalyst and the realization of efficient overall gas-phase CO2 conversion (Fig. 10e–g).141 Yang's group combined La2Ti2O7 with a narrow bandgap semiconductor to construct a 2D/2D N-doped La2Ti2O7/ZnIn2S4 heterojunction for photocatalytic water decomposition for hydrogen production.142 The experimental results showed that the optimized catalyst has the best photo-driven H2 generation activity with a hydrogen production rate of 44.62 μmol h−1, which is 5.2 times higher than that of the pristine ZnIn2S4 sample. This is due to its unique 2D/2D heterostructure that generates high-speed charge transfer channels, which significantly improves the separation and migration efficiency of photocarriers. Meanwhile, N doping narrows the band gap of La2Ti2O7, which greatly inhibits the recombination of electron–hole pairs. Xu's team synthesized an ultrathin 2D type-II p–n heterojunction, La2Ti2O7/In2S3, for visible-light photocatalytic hydrogen production.143 It was shown that the activity of the catalyst was significantly better than that of La2Ti2O7 and In2S3, with an 18-fold increase in hydrogen production. This is attributed to the effective separation of photogenerated charges and holes due to the formation of the heterojunction, which prolongs the charge lifetime and enhances the photocatalytic activity. In summary, rare-earth oxide photocatalysts and new rare-earth oxide photocatalysts can be widely used in various fields of photocatalysis and show good catalytic activity, and if they can be reasonably modified and designed and applied with rare-earth element-containing oxides, they will show great potential in the future photocatalytic field.
3. Conclusions and future perspective
In summary, the role of rare earth ions in photocatalysts mainly includes the following aspects: (i) rare earth ion doping can inhibit the phase change of the catalyst and maintain structural stability. (ii) Rare earth ions can separate the lattice oxygen from the surface of the photocatalyst, creating oxygen vacancies. (iii) The introduction of rare earth elements will cause lattice distortion of the catalyst, making redox reactions more likely to occur. (iv) Rare earth ions can act as a bridge for electron transfer between two semiconductor materials to effectively separate photogenerated electrons. (v) The doping energy level can play a role in trapping photogenerated electrons or holes, effectively inhibiting the recombination of electrons and holes. (vi) The introduction of rare earth elements can change the energy level structure of the catalyst, allowing catalysts with a narrow band gap to obtain a larger photoresponse range. (vii) Rare earth elements can enhance the adsorption ability of the catalyst to reactants, etc. Based on these advantages of rare earth elements, the rational design of rare earth based photocatalysts is expected to occupy an important position in the field of catalysis in the future. However, it is worth noting that not all rare earth elements act positively on catalysts. Many studies have shown that the impact on catalyst performance depends on the type and doping concentration of rare earth ions. Therefore, selecting appropriate rare earth elements and doping concentrations to design ideal photocatalysts remains a huge challenge. In addition, it is necessary to overcome many difficulties to develop rare earth photocatalysts on a large scale, such as the high cost of raw materials and low yield of the reaction, which means that more energy is needed to promote the reaction. The current reaction conditions are relatively harsh, usually requiring conditions such as high temperature and high pressure. To some extent, it limits practical applications.144 Therefore, it is necessary to optimize the process preparation of catalysts.
In addition to the above applications, scientists also need to explore some little-known fields, for example, using rare earth elements in other industries such as construction, automotive, and textile to develop new materials, devices, and products with photocatalytic functions. It can also be combined with other technologies, such as optoelectronics and biotechnology, to develop more efficient, environmentally friendly, and promising composite technologies. In summary, rare earths, as a group of similar and different elements, have special electronic structures and spectral characteristics, which can play various important roles in catalysts and show great potential for application. Deeply studying the deep interaction mechanisms and specific advantages of different rare earth ions, and utilizing the energy level structure of each rare earth ion for catalyst design are expected to provide new ideas for the design and development of efficient or novel photocatalyst systems.
Conflicts of interest
There are no conflicts to declare.
Acknowledgements
This work was supported by the National Natural Science Foundation of China (No. 22271080).
References
- J. N. Ma, T. J. Miao and J. W. Tang, Charge carrier dynamics and reaction intermediates in heterogeneous photocatalysis by time-resolved spectroscopies, Chem. Soc. Rev., 2022, 51, 5777–5794 RSC.
- H. J. Lu, J. L. Tournet, K. Dastafkan, Y. Liu, Y. H. Ng, S. K. Karuturi, C. Zhao and Z. Y. Yin, Noble-metal-free multicomponent nanointegration for sustainable energy conversion, Chem. Rev., 2021, 121, 10271–10366 CrossRef CAS PubMed.
- E. Nikoloudakis, I. L. Duarte, G. Charalambidis, K. Ladomenou, M. Ince and A. G. Coutsolelos, Porphyrins and phthalocyanines as biomimetic tools for photocatalytic H2 production and CO2 reduction, Chem. Soc. Rev., 2022, 51, 6965–7045 RSC.
- R. Y. Zheng, Z. C. Liu, Y. D. Wang, Z. K. Xie and M. Y. He, The future of green energy and chemicals: Rational design of catalysis routes, Joule, 2022, 6, 1148–1159 CrossRef CAS.
- H. Hussain, G. Tocci, T. Woolcot, X. Torrelles, C. L. Pang, D. S. Humphrey, C. M. Yim, D. C. Grinter, G. Cabailh, O. Bikondoa, R. Lindsay, J. Zegenhagen, A. Michaelides and G. Thornton, Structure of a model TiO2 photocatalytic interface, Nat. Mater., 2017, 16, 461–466 CrossRef CAS PubMed.
- Z. H. Xue, D. Y. Luan, H. B. Zhang and X. W. (David) Lou, Single-atom catalysts for photocatalytic energy conversion, Joule, 2022, 6, 92–133 CrossRef CAS.
- H. L. Wang, L. S. Zhang, Z. G. Chen, J. Q. Hu, S. J. Li, Z. H. Wang, J. S. Liu and X. C. Wang, Semiconductor heterojunction photocatalysts: design, construction, and photocatalytic performances, Chem. Soc. Rev., 2014, 43, 5234–5244 RSC.
- J. C. Zhang and M. Rueping, Metallaphotoredox catalysis for sp3 C-H functionalizations through hydrogen atom transfer (HAT), Chem. Soc. Rev., 2023, 52, 4099–4120 RSC.
- S. Cao, Y. Chen, H. Wang, J. Chen, X. H. Shi, H. M. Li, P. Cheng, X. F. Liu, M. Liu and L. Piao, Ultrasmall CoP nanoparticles as efficient cocatalysts for photocatalytic formic acid dehydrogenation, Joule, 2018, 2, 549–557 CrossRef CAS.
- L. Yang, Y. T. Peng, X. D. Luo, Y. Dan, J. H. Ye, Y. Zhou and Z. G. Zou, Beyond C3N4 π-conjugated metal-free polymeric semiconductors for photocatalytic chemical transformations, Chem. Soc. Rev., 2021, 50, 2147–2172 RSC.
- T. T. Kong, Y. W. Jiang and Y. J. Xiong, Photocatalytic CO2 conversion: What can we learn from conventional COx hydrogenation?, Chem. Soc. Rev., 2020, 49, 6579–6591 RSC.
- W. Wang, M. O. Tade and Z. P. Shao, Research progress of perovskite materials in photocatalysis-and photovoltaics-related energy conversion and environmental treatment, Chem. Soc. Rev., 2015, 44, 5371–5408 RSC.
- X. J. Yuan, D. Floresyona, P.-H. Aubert, T.-T. Bui, S. Remita, S. Ghosh, F. Brisset, F. Goubard and H. Remita, Photocatalytic degradation of organic pollutant with polypyrrole nanostructures under UV and visible light, Appl. Catal., B, 2019, 242, 284–292 CrossRef CAS.
- F. He, W. Jeon and W. Choi, Photocatalytic air purification mimicking the self-cleaning process of the atmosphere, Nat. Commun., 2021, 12, 2528 CrossRef CAS PubMed.
- M. Zhou, H. H. Ou, S. R. Li, X. Qin, Y. X. Fang, S. C. Lee, X. C. Wang and W. K. Ho, Photocatalytic air purification using functional polymeric carbon nitrides, Adv.
Sci., 2021, 8, 2102376 CrossRef CAS PubMed.
- Q. N. Yang, X. Y. Li, L. Chen, X. Y. Han, F. R. Wang and J. W. Tang, Effective activation of strong C-Cl bonds for highly selective photosynthesis of bibenzyl via homo-coupling, Angew. Chem., Int. Ed., 2023, 7, 202307907 Search PubMed.
- H. Wang, C. Cao, D. F. Li, Y. X. Ge, R. T. Chen, R. Song, W. S. Gao, X. L. Wang, X. T. Deng, H. J. Zhang, B. J. Ye, Z. L. Li and C. Li, Achieving high selectivity in photocatalytic oxidation of toluene on amorphous BiOCl nanosheets coupled with TiO2, J. Am. Chem. Soc., 2023, 145, 16852–16861 CrossRef CAS PubMed.
- H. X. Li, R. J. Li, G. Liu, M. L. Zhai and J. G. Yu, Noble-metal-free single- and dual-atom catalysts for artificial photosynthesis, Adv. Mater., 2023, 2301307 CrossRef PubMed.
- A. Basak, S. Karak and R. Banerjee, Covalent organic frameworks as porous pigments for photocatalytic metal-free C-H borylation, J. Am. Chem. Soc., 2023, 145, 7592–7599 CrossRef CAS PubMed.
- M. Ratova, J. Redfern, J. Verran and P. J. Kelly, Highly efficient photocatalytic bismuth oxide coatings and their antimicrobial properties under visible light irradiation, Appl. Catal., B, 2018, 39, 223–232 CrossRef.
- A. H. Mamaghani, F. Haghighat and C.-S. Lee, Photocatalytic oxidation technology for indoor environment air purification: The state-of-the-art, Appl. Catal., B, 2017, 203, 247–269 CrossRef CAS.
- W. J. Wu, Y. L. Yang, Y. T. Wang, T. T. Lu, Q. S. Dong, J. W. Zhao, J. Y. Niu, Q. C. Liu, Z. M. Hao and S. Y. Song, Boosting electrosynthesis of urea from N2 and CO2 by defective Cu-Bi, Chem Catal., 2022, 2, 3225–3238 CrossRef CAS.
- G. F. Liao, C. X. Li, S.-Y. Liu, B. Z. Fang and H. M. Yang, Z-scheme systems: From fundamental principles to characterization, synthesis, and photocatalytic fuel-conversion applications, Phys. Rep., 2022, 983, 1–41 CrossRef CAS.
- B. Samanta, A. M. Garcıa, F. Illas, N. Goga, J. A. Anta, S. Calero, A. Hutter, F. Libisch, A. B. M. Garcı, M. Pavone and M. C. Toroker, Challenges of modeling nanostructured materials for photocatalytic water splitting, Chem. Soc. Rev., 2022, 51, 3794–3818 RSC.
- H. X. Mai, D. H. Chen, Y. Tachibana, H. Suzuki, R. Abe and R. A. Caruso, Developing sustainable, high-performance perovskites in photocatalysis: design strategies and applications, Chem. Soc. Rev., 2021, 50, 13692–13729 RSC.
- Y. Y. Zhao, J. P. Yuan, L. Zhu and Y. Fang, Photocatalytic synthesis of small-molecule drugs by porous framework materials, Chin. Chem. Lett., 2023, 9, 109065 CrossRef.
- X. Zhang, P. J. Ma, C. Wang, L. Y. Gan, X. J. Chen, P. Zhang, Y. Wang, H. Li, L. H. Wang, X. Y. Zhou and K. Zheng, Unraveling the dual defect sites in graphite carbon nitride for ultra-high photocatalytic H2O2 Evolution, Energy Environ. Sci., 2022, 15, 830–842 RSC.
- B. C. Moon, B. Bayarkhuu, K. A. I. Zhang, D. K. Lee and J. Byun, Solar-driven H2O2 production via cooperative auto- and photocatalytic oxidation in fine-tuned reaction media, Energy Environ. Sci., 2022, 15, 5082–5092 RSC.
- N. Waiskopf, Y. B. Shahar and U. Banin, Photocatalytic hybrid semiconductor-metal nanoparticles; from synergistic properties to emerging applications, Adv. Mater., 2018, 30, 1706697 CrossRef PubMed.
- R. D. Richter, T. Z. Ming, P. Davies, W. Liu and S. Caillol, Removal of non-CO2 greenhouse gases by large-scale atmospheric solar photocatalysis, Prog. Energy Combust. Sci., 2017, 60, 68–96 CrossRef.
- B. Y. Dai, J. J. Fang, Y. R. Yu, M. L. Sun, H. M. Huang, C. H. Lu, J. H. Kou, Y. J. Zhao and Z. Z. Xu, Construction of Infrared-Light-Responsive Photoinduced Carriers Driver for Enhanced Photocatalytic Hydrogen Evolution, Adv. Mater., 2020, 32, 1906361 CrossRef CAS PubMed.
- H. Guo, J. Li, X. R. Zou, H. S. Wang, A. Kang, H. Zhou, M. J. Li and X. Y. Zhao, Fabrication of GO-TiO2/(Ca,Y)F2:Tm,Yb composites with high-efficiency optical driving photocatalytic activity for degradation of organic dyes and bacteriostasis, Rare Met., 2022, 41, 650–662 CrossRef CAS.
- A. Dubey, C. H. Keat, V. V. Shvartsman, K. V. Yusenko, M. E. Castillo, A. G. Buzanich, U. Hagemann, S. A. Kovalenko, J. Stähler and D. C. Lupascu, Mono-, Di-, and Tri-Valent Cation Doped BiFe0.95Mn0.05O3 Nanoparticles: Ferroelectric Photocatalysts, Adv. Funct. Mater., 2022, 32, 2207105 CrossRef CAS.
- X. Gong, L. Tang, R. X. Wang, Z. H. Guo, P. L. Huang, L. Zhou, J. Zou, J. Lei, H. H. Liu, N. Li, X. R. Tang, W. K. Zhu and R. He, Achieving efficient photocatalytic uranium extraction within a record short period of 3 minutes by Up-conversion erbium doped ZnO nanosheets, Chem. Eng. J., 2022, 450, 138044 CrossRef CAS.
- H. Y. Wu, J. H. Peng, H. C. Sun, Q. S. Ruan, H. F. Dong, Y. H. Jin, Z. M. Sun and Y. H. Hu, Surface activation of calcium tungstate by europium doping for improving photocatalytic performance: Towards lanthanide site photocatalysis, Chem. Eng. J., 2022, 432, 134339 CrossRef CAS.
- P. Mazierski, A. Mikolajczyka, B. Bajorowicz, A. Malankowska, A. Zaleska-Medynska and J. Nadolna, The role of lanthanides in TiO2-based photocatalysis: A review, Appl. Catal., B, 2018, 233, 301–317 CrossRef CAS.
- S.-C. Jung, I.-S. Park, H.-J. Bang, Y.-K. Park, S.-J. Kim, K.-J. Jeon and K.-H. Chung, Photocatalytic hydrogen production with purification of wastewater from nuclear power plant under irradiation of liquid phase plasma, Chem. Eng. J., 2020, 386, 121552 CrossRef CAS.
- B. Xu, Q. T. Zhang, S. S. Yuan, M. Zhang and T. Ohno, Synthesis and photocatalytic performance of yttrium-doped CeO2 with a porous broom-like hierarchical structure, Appl. Catal., B, 2016, 183, 361–370 CrossRef CAS.
- J. Y. Zhang, S. H. Wu, X. M. Lu, P. Wu and J. W. Liu, Lanthanide-boosted singlet oxygen from diverse photosensitizers along with potent photocatalytic oxidation, ACS Nano, 2019, 13, 14152–14161 CrossRef CAS PubMed.
- Q. Y. Chen, G. Y. Gao, Y. Z. Zhang, Y. N. Li, H. Y. Zhu, P. F. Zhu, Y. Qu, G. F. Wang and W. P. Qin, Dual functions of CO2 molecular activation and 4f levels as electron transport bridges in erbium single atom composite photocatalysts therefore enhancing visible-light photoactivities, J. Mater. Chem. A, 2021, 9, 15820–15826 RSC.
- S. O.-B. Oppong, F. Opoku and P. P. Govender, Tuning the electronic and structural properties of Gd-TiO2-GO nanocomposites for enhancing photodegradation of IC dye: The role of Gd3+ ion, Appl. Catal., B, 2019, 243, 106–120 CrossRef CAS.
- Y. N. Li, Y. Qu and G. F. Wang, Europium single atom based heterojunction photocatalysts with enhanced visible-light catalytic Activity, J. Mater. Chem. A, 2022, 10, 5990–5997 RSC.
- S. Ida, S. Koga, T. Diao, H. Hagiwara and T. Ishihara, Direct imaging of light emission centers in two-dimensional crystals
and their luminescence and photocatalytic properties, Angew. Chem., Int. Ed., 2014, 53, 13078–13082 CrossRef CAS PubMed.
- Y. Xia, Z. H. Tian, T. Heil, A. Meng, B. Cheng, S. W. Cao, J. G. Yu and M. Antonietti, Highly selective CO2 capture and its direct photochemical conversion on ordered 2D/1D heterojunctions, Joule, 2019, 3, 2792–2805 CrossRef CAS.
- F. Sordello, I. Berruti, C. Gionco, M. C. Paganini, P. Calza and C. Minero, Photocatalytic performances of rare earth element-doped zinc oxide toward pollutant abatement in water and wastewater, Appl. Catal., B, 2019, 254, 159–166 CrossRef.
- Y. W. Yang, W. X. Que, X. Y. Zhang, X. T. Yin, Y. L. Xing, M. D. Que, H. Y. Zhao and Y. P. Du, High-quality Cu2ZnSnS4 and Cu2ZnSnSe4 nanocrystals hybrid with ZnO and NaYF4: Yb, Tm as efficient photocatalytic sensitizers, Appl. Catal., B, 2017, 200, 402–411 CrossRef CAS.
- G. Wang, R. Huang, J. W. Zhang, J. J. Mao, D. S. Wang and Y. D. Li, Synergistic modulation of the separation of photo-generated carriers via engineering of dual atomic sites for promoting photocatalytic performance, Adv. Mater., 2021, 33, 2105904 CrossRef CAS PubMed.
- Y. A. Zhu, Z. Y. Zhang, N. Lu, R. N. Hua and B. Dong, Prolonging charge-separation states by doping lanthanide-ions into {001}/{101} facets-coexposed TiO2 nanosheets for enhancing photocatalytic H2 evolution, Chin. J. Catal., 2019, 40, 413–423 CrossRef CAS.
- C. B. Bie, B. C. Zhu, F. Y. Xu, L. Y. Zhang and J. G. Yu, In situ grown monolayer N-doped graphene on CdS hollow spheres with seamless contact for photocatalytic CO2 reduction, Adv. Mater., 2019, 31, 1902868 CrossRef CAS PubMed.
- V. Vaiano, M. Matarangolo, O. Sacco and D. Sannino, Photocatalytic treatment of aqueous solutions at high dye concentration using praseodymium-doped ZnO catalysts, Appl. Catal., B, 2017, 209, 621–630 CrossRef CAS.
- H. H. Ren, F. H. Huang, J. M. Jiang, L. Wang and J. L. Zhang, Development of photocatalyst based on NaYF4: Yb, Tm@NaYF4: Yb, Ce/NH2-MIL-101 (Cr): doping Ce3+ ions to promote the efficient energy transfer between core and shell, Chem. Eng. J., 2022, 427, 132023 CrossRef CAS.
- S. Obregón and G. Colón, Heterostructured Er3+ doped BiVO4 with exceptional photocatalytic performance by cooperative electronic and luminescence sensitization mechanism, Appl. Catal., B, 2014, 158, 242–249 CrossRef.
- K. Villa, S. M. López, J. R. Morante and T. Andreu, An insight on the role of La in mesoporous WO3 for the photocatalytic conversion of methane into methanol, Appl. Catal., B, 2016, 187, 30–36 CrossRef CAS.
- W. Wang, M. O. Tadé and Z. P. Shao, Nitrogen-doped simple and complex oxides for photocatalysis: A review, Prog. Mater. Sci., 2018, 92, 33–63 CrossRef CAS.
- M. Miodynska, A. Mikolajczyk, B. Bajorowicz, J. Zwara, T. Klimczuk, W. Lisowski, G. Trykowski, H. P. Pinto and A. Z-M, Urchin-like TiO2 structures decorated with lanthanide-doped Bi2S3 quantum dots to boost hydrogen photogeneration performance, Appl. Catal., B, 2020, 272, 118962 CrossRef CAS.
- G. S. Wolde, D.-H. Kuo, M. H. Urgesa and T. N. Gemeda, Photocatalytic oxidation of benzyl alcohol coupled with p-dinitrobenzene reduction over poly (o-phenylenediamine) nanowires-decorated Gd-TiO2 nanorods, Chem. Eng. J., 2023, 469, 143916 CrossRef CAS.
- Y. T. Zou, Y. Z. Hu, A. Uhrich, Z. W. Shen, B. X. Peng, Z. Y. Ji, M. Muhler, G. X. Zhao, X. K. Wang and X. X. Xu, Steering accessible oxygen vacancies for alcohol oxidation over defective Nb2O5 under visible light illumination, Appl. Catal., B, 2021, 298, 120584 CrossRef CAS.
- C. P. Xu, P. R. Anusuyadevi, C. Aymonier, R. Luque and S. Marre, Nanostructured materials for photocatalysis, Chem. Soc. Rev., 2019, 48, 3868–3902 RSC.
- Q. Wang and K. Domen, Particulate photocatalysts for light-driven water splitting: mechanisms, challenges, and design strategies, Chem. Rev., 2020, 120, 919–985 CrossRef CAS PubMed.
- Z. Q. Zhao, R. V. Goncalves, S. K. Barman, E. J. Willard, E. Byle, R. Perry, Z. K. Wu, M. N. Huda, A. J. Moule and F. E. Osterloh, Electronic structure basis for enhanced overall water splitting photocatalysis with aluminum doped SrTiO3 in natural sunlight, Energy Environ. Sci., 2019, 12, 1385–1395 RSC.
- J. Wu, Y. Xie, Y. Ling, J. C. Si, X. Li, J. L. Wang, H. Ye, J. S. Zhao, S. Q. Li, Q. D. Zhao and Y. Hou, One-step synthesis and Gd3+ decoration of BiOBr microspheres consisting of nanosheets toward improving photocatalytic reduction of CO2 into hydrocarbon fuel, Chem. Eng. J., 2020, 400, 125944 CrossRef CAS.
- Z. P. Rao, G. S. Shi, Z. Wang, A. Mahmood, X. F. Xie and J. Sun, Photocatalytic degradation of gaseous VOCs over Tm3+-TiO2: Revealing the activity enhancement mechanism and different reaction paths, Chem. Eng. J., 2020, 395, 125078 CrossRef CAS.
- H. L. Gao, J. M. Liu, J. Zhang, Z. Q. Zhu, G. L. Zhang and Q. J. Liu, Influence of carbon and yttrium co-doping on the photocatalytic activity of mixed phase TiO2, Chin. J. Catal., 2017, 38, 1688–1696 CrossRef CAS.
- H. Yang, B. Xu, Q. T. Zhang, S. S. Yuan, Z. P. Zhang, Y. T. Liu, Z. D. Nan, M. Zhang and T. Ohna, Boosting visible-light-driven photocatalytic performance of waxberry-like CeO2 by samarium doping and silver QDs anchoring, Appl. Catal., B, 2021, 286, 119845 CrossRef CAS.
- L. Meng, K. F. Zhang, K. Pan, Y. Qu and G. F. Wang, Controlled synthesis of CaTiO3:Ln3+ nanocrystals for luminescence and photocatalytic hydrogen production, RSC Adv., 2016, 6, 5761–5766 RSC.
- Y. W. Ren, Y. J. Han, Z. Y. Li, X. M. Liu, S. L. Zhu, Y. Q. Liang, K. W. K. Yeung and S. L. Wu, Ce and Er Co-doped TiO2 for rapid bacteria-killing using visible light, Bioact. Mater., 2020, 5, 201–209 Search PubMed.
- W. C. Wang, L. L. Du, R. Q. Xia, R. H. Liang, T. Zhou, H. K. Lee, Z. P. Yan, H. Luo, C. X. Shang, D. L. Phillips and Z. X. Guo, In situ protonated-phosphorus interstitial doping induces long-lived shallow charge trapping in porous C3-xN4 photocatalysts for highly efficient H2 generation, Energy Environ. Sci., 2023, 16, 460–472 RSC.
- L. P. Xu, B. N. Tian, T. Y. Wang, Y. Yu, Y. C. Wu, J. W. Cui, Z. N. Cao, J. H. Wu, W. K. Zhang, Q. Zhang, J. Q. Liu, Z. F. Li and Y. Tian, Direct Z-scheme polymeric heterojunction boosts photocatalytic hydrogen production via a rebuilt extended π-delocalized network, Energy Environ. Sci., 2022, 15, 5059–5068 RSC.
- R. Das, K. Das, B. Ray, C. P. Vinod and S. C. Peter, Green transformation of CO2 to ethanol using water and sunlight by the combined effect of naturally abundant red phosphorus and Bi2MoO6, Energy Environ. Sci., 2022, 15, 1967–1976 RSC.
- P. Mialane, C. Mellot-Draznieks, P. Gairola, M. Duguet, Y. Benseghir, O. Oms and A. Dolbecq, Heterogenisation of polyoxometalates and other metal-based complexes in metal-organic frameworks: from synthesis to characterisation and applications in catalysis, Chem. Soc. Rev., 2021, 50, 6152–6220 RSC.
- A. Bavykina, N. Kolobov, Il S. Khan, J. A. Bau, A. Ramirez and J. Gascon, Metal-organic frameworks in heterogeneous catalysis: recent progress, new trends, and future perspectives, Chem. Rev., 2020, 120, 8468–8535 CrossRef CAS PubMed.
- C. Xu, Q. Zhou, W. Y. Huang, K. Yang, Y. C. Zhang, T. Xi. Liang and Z. Q. Liu, Constructing Z-scheme β-Bi2O3/ZrO2 heterojunctions with 3D mesoporous SiO2 nanospheres for efficient antibiotic remediation via synergistic adsorption and photocatalysis, Rare Met., 2022, 41, 2094–2107 CrossRef CAS.
- Y. J. Li, J. H. Ma, L. Xu, T. Liu, T. Z. Xiao, D. M. Chen, Z. G. Song, J. B. Qiu and Y. L. Zhang, Enhancement of charge separation and NIR light harvesting through construction of 2D–2D Bi4O5I2/BiOBr:Yb3+, Er3+ Z-scheme heterojunctions for improved full-spectrum photocatalytic performance, Adv. Sci., 2023, 10, 2207514 CrossRef CAS PubMed.
- B. Y. Xu, A. Zada, G. F. Wang and Y. Qu, Boosting the visible-light photoactivities of BiVO4 nanoplates by doping Eu and coupling CeOx nanoparticles for CO2 reduction and organic oxidation, Sustainable Energy Fuels, 2019, 1–3 Search PubMed.
- G. F. S. R. Rocha, M. A. R. da Silva, A. Rogolino, G. A. A. Diab, L. F. G. Noleto, M. Antonietti and I. F. Teixeira, Carbon nitride based materials: more than just a support for single-atom catalysis, Chem. Soc. Rev., 2023, 52, 4878–4932 RSC.
- S. W. Wang, L. G. Wang, D. S. Wang and Y. D. Li, Recent advances of single-atom catalysts in CO2 conversion, Energy Environ. Sci., 2023, 16, 2759–2803 RSC.
- P. F. Cai, J. Li, X. B. Wu, Z. Y. Li, J. Shen, J. J. Nie, Z. D. Cui, D. F. Chen, Y. Q. Liang, S. L. Zhu and S.-L. Wu, ALD-induced TiO2/Ag nanofilm for rapid surface photodynamic ion sterilization, Rare Met., 2022, 41, 4138–4148 CrossRef CAS PubMed.
- Y. P. Pang, C. Su, L. Q. Xu and Z. P. Shao, When nitrogen reduction meets single-atom catalysts, Prog. Mater. Sci., 2023, 132, 101044 CrossRef CAS.
- Y. Q. Wu, Q. Wu, Q. Q. Zhang, Z. Z. Lou, K. F. Liu, Y. D. Ma, Z. Y. Wang, Z. K. Zheng, H. F. Cheng, Y. Y. Liu, Y. Dai, B. B. Huang and P. Wang, An organometal halide perovskite supported Pt single-atom photocatalyst for H2 evolution, Energy Environ. Sci., 2022, 15, 1271–1281 RSC.
- F. F. Zhang, Y. L. Zhu, Q. Lin, L. Zhang, X. W. Zhang and H. T. Wang, Noble-metal single-atoms in thermocatalysis, electrocatalysis, and photocatalysis, Energy Environ. Sci., 2021, 14, 2954–3009 RSC.
- L. M. Wang, W. L. Chen, D. D. Zhang, Y. P. Du, R. Amal, S. Z. Qiao, J. B. Wu and Z. Y. Yin, Surface strategies for catalytic CO2 reduction: from two-dimensional materials to nanoclusters to single atoms, Chem. Soc. Rev., 2019, 48, 5310–5349 RSC.
- H. P. Peng, T. Yang, H. P. Lin, Y. Xu, Z. H. Wang, Q. H. Zhang, S. H. Liu, H. B. Geng, L. Gu, C. Wang, X. Fan, W. X. Chen and X. Huang, Ru/In dual-single atoms modulated charge separation for significantly accelerated photocatalytic H2 evolution in pure water, Adv. Energy Mater., 2022, 12, 2201688 CrossRef CAS.
- C. Gao, J. X. Low, R. Long, T. T. Kong, J. F. Zhu and Y. J. Xiong, Heterogeneous single-atom photocatalysts: fundamentals and applications, Chem. Rev., 2020, 120, 12175–12216 CrossRef CAS PubMed.
- A. Krukowska, M. J. Winiarski, J. S. Nowak, T. Klimczuk, W. Lisowski, A. Mikolajczyk, H. P. Pinto, T. Puzyn, T. Grzyb and A. Z. Medynsk, Rare earth ions doped K2Ta2O6 photocatalysts with enhanced UV-vis light activity, Appl. Catal., B, 2018, 224, 451–468 CrossRef CAS.
- X. F. Liu, Y. N. Luo, C. C. Ling, Y. B. Shi, G. M. Zhan, H. Li, H. Y. Gu, K. Wei, F. R. Guo, Z. H. Ai and L. Z. Zhang, Rare earth La single atoms supported MoO3-x for efficient photocatalytic nitrogen fixation, Appl. Catal., B, 2022, 301, 120766 CrossRef CAS.
- S. F. Ji, Y. Qu, T. Wang, Y. J. Chen, G. F. Wang, X. Li, J. C. Dong, Q. Y. Chen, W. Y. Zhang, Z. D. Zhang, S. Y. Liang, R. Yu, Y. Wang, D. S. Wang and Y. D. Li, Rare-earth single erbium atoms for enhanced photocatalytic CO2 reduction, Angew. Chem., Int. Ed., 2020, 59, 10651–10657 CrossRef CAS PubMed.
- M. Z. Ma, Z. A. Huang, L. N. Li, W. D. Zhang, R. Guo, R. R. Zhang, W. J. Fa, C. Q. Han, Y. H. Cao, S. Yu and Y. Zhou, Modulating photogenerated electron density of Pr single-atom sites by coordination environment engineering for boosting photoreduction of CO2 to CH3OH, Appl. Catal., B, 2023, 330, 122626 CrossRef CAS.
- Q. Y. Wang, L. Y. Dong, M. Z. Li, H. L. Lu, G. D. Wei, Y. Qu and G. F. Wang, Z-scheme heterojunction photocatalyst based on lanthanum single-atom anchored on black phosphorus for regulating surface active sites, therefore enhancing photocatalytic CO2 reduction with ≈ 100% CO selectivity, Adv. Funct. Mater., 2022, 32, 2207330 CrossRef CAS.
- L. L. Sun, X. S. Liu, Y. B. Feng, X. L. Ding, J. G. Wang, N. Jiang and S. J. Wang, High loads of rare earth single-atom lanthanum anchored carbon nitride with special double-layer coordination structure for efficient photocatalysis, Appl. Catal., B, 2023, 338, 122979 CrossRef CAS.
- P. Chen, B. Lei, X. A. Dong, H. Wang, J. P. Sheng, W. Cui, J. Y. Li, Y. J. Sun, Z. M. Wang and F. Dong, Rare-earth single-atom La-N charge-transfer bridge on carbon nitride for highly efficient and selective photocatalytic CO2 reduction, ACS Nano, 2020, 14, 15841–15852 CrossRef CAS PubMed.
- P. Chen, H. Wang, H. J. Liu, Z. L. Ni, J. Y. Li, Y. Zhou and F. Dong, Directional electron delivery and enhanced reactants activation enable efficient photocatalytic air purification on amorphous carbon nitride co-functionalized with O/La, Appl. Catal., B, 2019, 242, 19–30 CrossRef CAS.
- Z. D. Han, Y. Zhao, G. Y. Gao, W. Y. Zhang, Y. Qu, H. Y. Zhu, P. F. Zhu and G. F. Wang, Erbium single atom composite photocatalysts for reduction of CO2 under visible light: CO2 molecular activation and 4f levels as electron transport bridge, Small, 2021, 17, 2102089 CrossRef CAS PubMed.
- Y. Zhao, Z. D. Han, G. Y. Gao, W. Y. Zhang, Y. Qu, H. Y. Zhu, P. F. Zhu and G. F. Wang, Dual functions of CO2 molecular activation and 4f levels as electron transport bridge in dysprosium single atom composite photocatalysts with enhanced visible-light photoactivities, Adv. Funct. Mater., 2021, 31, 2104976 CrossRef CAS.
- J. E. Casillas, F. Tzompantzi, S. G. Castellanos, G. Mendoza-Damián, R. Pérez-Hernández, A. López-Gaona and A. Barrera, Promotion effect of ZnO on the photocatalytic activity of coupled Al2O3-Nd2O3-ZnO composites prepared by the sol-gel method in the degradation of phenol, Appl. Catal., B, 2017, 208, 161–170 CrossRef CAS.
- K. Ishisone, T. Isobe, S. Matsushita, M. Wakamura, M. Oshikiri and A. Nakajima, LaO1.5 surface modification of titanium-substituted hydroxyapatite photocatalyst and effects on 2-propanol photocatalytic decomposition mechanisms, Appl. Catal., B, 2021, 293, 119658 CrossRef.
- Z. Y. Zhong, H. Fu, S. Y. Wang, Y. N. Duan, Q. L. Wang, C. H. Yan and Y. P. Du, A Universal Synthesis Strategy for Lanthanide Sulfide Nanocrystals with Efficient Photocatalytic Hydrogen Production, Small, 2023, 19, 2301392 CrossRef CAS PubMed.
- C. Karunakaran, S. Narayanan and P. Gomathisankar, Photocatalytic degradation of 1-naphthol by oxide ceramics with added bacterial disinfection, J. Hazard. Mater., 2010, 181, 708–715 CrossRef CAS PubMed.
- L. P. Lingamdinne, S. Lee, J.-S. Choi, V. R. Lebaka, V. R. P. Durbaka and J. R. Koduru, Potential of the magnetic hollow sphere nanocomposite (graphene oxide-gadolinium oxide) for arsenic removal from real field water and antimicrobial applications, J. Hazard. Mater., 2021, 402, 123882 CrossRef CAS PubMed.
- M. L. Sun, P. Zhou, J. L. Peng, C. S. He, Y. Du, Z. C. Pan, S. J. Su, F. Dong, Y. Liu and B. Lai, Insights into peroxymonosulfate activation under visible Light: Sc2O3@C3N4 mediated photoexcited electron transfer, Chem. Eng. J., 2022, 435, 134836 CrossRef CAS.
- Q. Wang, Y. J. Chen, X. Liu, L. G. Li, L. Z. Du and G. H. Tian, Sulfur doped In2O3-CeO2 hollow hexagonal prisms with carbon coating for efficient photocatalytic CO2 reduction, Chem. Eng. J., 2021, 421, 129968 CrossRef CAS.
- X. B. Huang, K. Y. Zhang, B. X. Peng, G. Wang, M. Muhler and F. Wang, Ceria-based materials for thermocatalytic and photocatalytic organic synthesis, ACS Catal., 2021, 11, 9618–9678 CrossRef CAS.
- J. X. Ji, R. R. Li, H. Zhang, Y. N. Duan, Q. Liu, H. Z. Wang and Z. R. Shen, Highly selective photocatalytic reduction of CO2 to ethane over Au-O-Ce sites at micro-interface, Appl. Catal., B, 2023, 321, 122020 CrossRef CAS.
- W. Q. Li, L. Jin, F. Gao, H. Q. Wan, Y. Pu, X. Q. Wei, C. Chen, W. X. Zou, C. Z. Zhu and L. Dong, Advantageous roles of phosphate decorated octahedral CeO2 {111}/g-C3N4 in boosting photocatalytic CO2 reduction: Charge transfer bridge and Lewis basic site, Appl. Catal., B, 2021, 294, 120257 CrossRef CAS.
- P. F. Xia, S. W. Cao, B. C. Zhu, M. J. Liu, M. S. Shi, J. G. Yu and Y. F. Zhang, Designing a 0D/2D S-scheme heterojunction over polymeric carbon nitride for visible-light photocatalytic inactivation of bacteria, Angew. Chem., Int. Ed., 2020, 59, 5218–5225 CrossRef CAS PubMed.
- T. Wang, L. Chen, C. Chen, M. T. Huang, Y. J. Huang, S. J. Liu and B. X. Li, Engineering catalytic interfaces in Cuδ+/CeO2-TiO2 photocatalysts for synergistically boosting CO2 reduction to ethylene, ACS Nano, 2022, 16, 2306–2318 CrossRef CAS PubMed.
- Y. F. Bao, H. Zou, S. W. Du, X. S. Xin, S. W. Wang, G. S. Shao and F. X. Zhang, Metallic powder promotes nitridation kinetics for facile synthesis of (Oxy)nitride photocatalysts, Adv. Mater., 2023, 35, 2302276 CrossRef CAS PubMed.
- Y. Q. Liu, J. L. Zhou and Z. K. Sun, Direct synthesis of unnatural amino acids and modifications of peptides via LADA strategy, Chin. Chem. Lett., 2024, 35, 108553 CrossRef CAS.
- L. H. Lin, P. P. Kaewdee, V. Nandal, R. Shoji, H. Matsuzaki, K. Seki, M. Nakabayashi, N. Shibata, X. P. Tao, X. Z. Liang, Y. W. Ma, T. Hisatomi, T. Takata and K. Domen, Flux-assisted synthesis of Y2Ti2O5S2 for photocatalytic hydrogen and oxygen evolution reactions, Angew. Chem., Int. Ed., 2023, 202310607 Search PubMed.
- D. Kato, R. Abe and H. Kageyama, Extended layer-by-layer madelung potential analysis of layered oxyhalide photocatalysts and other layered systems, J. Mater. Chem. A, 2019, 7, 19846–19851 RSC.
- S. Zhong, L. H. Liu, G. G. Liu, L. F. Yan, W. Wang, L. S. Zhang and B. J. Liu, Piezoelectric polarization promoted separation of photogenerated carriers in Bi3.25La0.75Ti3O12 with different micro-morphologies for efficient elimination of 2,4-Dichlorophenol and tetracycline, J. Cleaner Prod., 2022, 373, 133644 CrossRef CAS.
- Y. J. Wei and B. Y. Ji, The health effects of artificial sweeteners: Towards personalized quantification and prediction through gut microbiome, Eco. Environ. Health, 2023, 2, 89–91 CrossRef.
- X. J. Liu, B. B. Liu, L. Li, Z. H. Zhuge, P. B. Chen, C. Li, Y. Y. Gong, L. Y. Niu, J. Y. Liu, L. Lei and C. Q. Sun, Cu2In2ZnS5/Gd2O2S:Tb for full solar spectrum photoreduction of Cr(VI) and CO2 from UV/vis to near-infrared light, Appl. Catal., B, 2019, 249, 82–90 CrossRef CAS.
- D. W. Zeng, Y. Qiu, L. Ma, M. Li, D. X. Cui, S. A. Zhang and R. Xiao, Tuning the support properties toward higher CO2 conversion during a chemical looping scheme, Environ. Sci. Technol., 2020, 54, 12467–12475 CrossRef CAS PubMed.
- P. G. Muñoz, F. Fresno, C. Lefevre, D. Robert and N. Keller, Highly robust La1-xTixFeO3 dual catalyst with combined photocatalytic and photo-CWPO activity under visible light for 4-chlorophenol removal in Water treatment, Appl. Catal., B, 2020, 262, 118310 CrossRef.
- S. Z. Ajabshir, Z. Salehi and M. S. Niasari, Green synthesis and characterization of Dy2Ce2O7 ceramic nanostructures with good photocatalytic properties under visible light for removal of organic dyes in water, J. Cleaner Prod., 2018, 192, 678–687 CrossRef.
- S. Z. Ajabshir, M. S. Morassaei and M. S. Niasari, Nd2Sn2O7 nanostructures as highly efficient visible light photocatalyst: green synthesis using pomegranate juice and characterization, J. Cleaner Prod., 2018, 198, 11–18 CrossRef.
- S. Z. Ajabshir, M. S. Morassaei and M. S. Niasari, Eco-friendly synthesis of Nd2Sn2O7-based nanostructure materials using grape juice as green fuel as photocatalyst for the degradation of erythrosine, Composites, Part B, 2019, 167, 643–653 CrossRef.
- P. S. Li, M. Guo, Q. Wang, Z. Li, C. Z. Wang, N. Chen, C. C. Wang, C. Q. Wan and S. W. Chen, Controllable synthesis of cerium zirconium oxide nanocomposites and their application for photocatalytic degradation of sulfonamides, Appl. Catal., B, 2019, 259, 118107 CrossRef CAS.
- K. Brlec, C. N. Savory and D. O. Scanlon, Understanding the electronic structure of Y2Ti2O5S2 for green hydrogen production: a hybrid DFT and GW study, J. Mater. Chem. A, 2023, 11, 16776–16787 RSC.
- H. Yoshida, Z. H. Pan, R. Shoji, V. Nandal, H. Matsuzaki, K. Seki, T. Hisatomi and K. Domen, Heterogeneous doping of visible-light-responsive Y2Ti2O5S2 for enhanced hydrogen evolution, J. Mater. Chem. A, 2022, 10, 24552–24560 RSC.
- R. H. Li, Z. D. Zha, Y. N. Zhang, M. J. Yang, L. H. Lin, Q. Wang, T. Hisatomi, M. Nakabayashi, N. Shibata, K. Domen and Y. Li, Band-tail states meditated visible-light-driven overall water splitting in Y2Ti2O5S2 photocatalyst, J. Mater. Chem. A, 2022, 10, 24247–24257 RSC.
- K. A. Brown, S. He, D. J. Eichelsdoerfer, M. C. Huang, I. Levy, H. Lee, S. Ryu, P. Irvin, J. M. Arroyo, C.-B. Eom, C. A. Mirkin and J. Levy, Giant conductivity switching of LaAlO3/SrTiO3 heterointerfaces governed by surface protonation, Nat. Commun., 2016, 7, 10681 CrossRef CAS PubMed.
- L. X. Zhang, K. Wang, Y. Q. Jia, L. P. Fang, C. Han, J. Q. Li, Z. P. Shao, X. Y. Li, J. S. Qiu and S. M. Liu, Self-assembled LaFeO3/ZnFe2O4/La2O3 ultracompact hybrids with enhanced piezo-phototronic effect for oxygen activation in ambient conditions, Adv. Funct. Mater., 2022, 32, 2205121 CrossRef CAS.
- K. L. Zhang, M. Zhou, K. Yang, C. L. Yu, P. Mu, Z. Z. Yu, K. Q. Lu, W. Y. Huang and W. X. Dai, Photocatalytic H2O2 production and removal of Cr(VI) via a novel Lu3NbO7: Yb, Ho/CQDs/AgInS2/In2S3 heterostructure with broad spectral response, J. Hazard. Mater., 2022, 423, 127172 CrossRef CAS PubMed.
- X. Y. Liu, Y. Y. Wang, H. R. Xiang, J. H. Wu, X. Yan, W. C. Zhang, Z. Lin and L. Y. Chai, Unveiling the crucial role of iron mineral phase transformation in antimony(V) elimination from natural water, Eco. Environ. Health, 2023, 2, 176–183 CrossRef.
- X. Y. Cai, M. S. Zhu, O. A. Elbanna, M. Fujitsuka, S. Kim, L. Mao, J. Y. Zhang and T. Majima, Au nanorod photosensitized La2Ti2O7 nanosteps: successive surface heterojunctions boosting visible to near-infrared photocatalytic H2 evolution, ACS Catal., 2018, 8, 122–131 CrossRef CAS.
- Z. Wang, K. Teramura, S. Hosokawa and T. Tanaka, Photocatalytic conversion of CO2 in water over Ag-modified La2Ti2O7, Appl. Catal., B, 2015, 163, 241–247 CrossRef CAS.
- J. W. Wang, Y. Asakura, T. Hasegawa and S. Yin, High-concentration N-doped La2Ti2O7 nanocrystals: Effects of nano-structuration and doping sites on enhancing the photocatalytic activity, Chem. Eng. J., 2021, 423, 130220 CrossRef CAS.
- C. Chen, Q. Q. Ma, F. Z. Liu, J. N. Gao, X. Y. Li, S. B. Sun, H. Yao, C. Q. Liu, J. Young and W. Zhang, Photocatalytically reductive defluorination of perfluorooctanoic acid (PFOA) using Pt/La2Ti2O7 nanoplates: experimental and DFT assessment, J. Hazard. Mater., 2021, 419, 126452 CrossRef CAS PubMed.
- Z. X. Wang, Y. X. Wang, L. H. Huang, X. W. Liu, Y. F. Han and L. S. Wang, La2Zr2O7/rGO synthesized by one-step sol-gel method for photocatalytic degradation of tetracycline under visible-light, Chem. Eng. J., 2020, 384, 123380 CrossRef CAS.
- L. Lu, S. M. Wang, C. G. Zhou, Z. Shi, H. Zhu, Z. Y. Xin, X. H. Wang, S. C. Yan and Z. G. Zou, Surface chemistry imposes selective reduction of CO2 to CO over Ta3N5/LaTiO2N photocatalyst, J. Mater. Chem. A, 2018, 6, 14838–14846 RSC.
- Y. L. Dong, G. Li, D. Y. Xu, Q. W. Wang, T. S. Yang, S. X. Pang, G. M. Zhang, L. Y. Lv, Y. G. Xia, Z. J. Ren and P. F. Wang, A novel hierarchical heterostructure of hollow La2Ti2O7/In2O3 with strong interface interaction for photocatalytic antibiotic degradation, Chem. Eng. J., 2022, 446, 136705 CrossRef CAS.
- H. Zhang, J. M. Ma, S. Y. Wang, J. X. Ji, Z. C. Zeng, Z. R. Shen, Y. P. Du and C. H. Yan, Novel Cerium-based sulfide nano-photocatalyst for highly efficient CO2 reduction, Small, 2023, 18, 2201332 CrossRef PubMed.
- Q. Q. Li, L. P. Song, Z. Liang, M. Z. Sun, T. Wu, B. L. Huang, F. Luo, Y. P. Du and C. H. Yan, A review on CeO2-based electrocatalyst and photocatalyst in energy conversion, Adv. Energy Sustainability Res., 2021, 2, 2000063 CrossRef CAS.
- R. Boppella, C. H. Choi, J. Moon and D. H. Kim, Spatial charge separation on strongly coupled 2D-hybrid of rGO/La2Ti2O7/NiFe-LDH heterostructures for highly efficient noble metal free photocatalytic hydrogen generation, Appl. Catal., B, 2018, 239, 178–186 CrossRef CAS.
- J. L. Li, Y. X. Zhao, M. Y. Xia, H. An, H. C. Bai, J. J. Wei, B. L. Yang and G. D. Yang, Highly efficient charge transfer at 2D/2D layered P-La2Ti2O7/Bi2WO6 contact heterojunctions for upgraded visible-light-driven photocatalysis, Appl. Catal., B, 2020, 261, 118244 CrossRef CAS.
- Y. H. Ao, K. D. Wang, P. F. Wang, C. Wang and J. Hou, Synthesis of novel 2D-2D p-n heterojunction BiOBr/La2Ti2O7 composite photocatalyst with enhanced photocatalytic performance under both UV and visible light irradiation, Appl. Catal., B, 2016, 194, 157–168 CrossRef CAS.
- S. Z. Ajabshir, Z. Z. Ajabshir, M. S. Niasari, S. Bagheri and S. B. A. Hamid, Facile preparation of Nd2Zr2O7-ZrO2 nanocomposites as an effective photocatalyst via a new route, J. Energy Chem., 2017, 26, 315–323 CrossRef.
- S. Z. Ajabshir and M. S. Niasari, Preparation of magnetically retrievable CoFe2O4@SiO2@Dy2Ce2O7 nanocomposites as novel photocatalyst for highly efficient degradation of organic contaminants, Composites, Part B, 2019, 174, 106930 CrossRef.
- S. J. Li, Y. Z. Li, Y. J. Chen, L. N. Xu, Q. Y. Chen, Y. Qu, G. F. Wang, P. F. Zhu, D. S. Wang and W. P. Qin, Enhanced Visible-Light Photoactivities of Perovskite-Type LaFeO3 Nanocrystals by Simultaneously Doping Er3+ and Coupling MgO for CO2 Reduction, ChemCatChem, 2020, 12, 623–630 CrossRef CAS.
- J. Song, Y. Lu, Y. Lin, Q. W. Liu, X. X. Wang and W. Y. Su, A direct Z-scheme α-Fe2O3/LaTiO2N visible-light photocatalyst for enhanced CO2 reduction activity, Appl. Catal., B, 2021, 292, 120185 CrossRef CAS.
- M. Y. Xia, X. Q. Yan, H. Li, N. Wells and G. D. Yang, Well-designed efficient charge separation in 2D/2D N doped La2Ti2O7/ZnIn2S4 heterojunction through band structure/morphology regulation synergistic effect, Nano Energy, 2020, 78, 105401 CrossRef CAS.
- E. B. Hua, S. Jin, X. R. Wang, S. A. Ni, G. Liu and X. X. Xu, Ultrathin 2D type-II p-n heterojunctions La2Ti2O7/In2S3 with efficient charge separations and photocatalytic hydrogen evolution under visible light illumination, Appl. Catal., B, 2019, 245, 733–742 CrossRef CAS.
- Y. Zhou, Y. L. He, M. Gao, N. K. Din, J. Y. Lei and Y. B. Zhou, Efficient photocatalytic NADH regeneration with Rh-loaded Z-scheme mediator-free system, Chin. Chem. Lett., 2023, 22, 108609 Search PubMed.
|
This journal is © the Partner Organisations 2024 |
Click here to see how this site uses Cookies. View our privacy policy here.