A novel route for microplastic mineralization: visible-light-driven heterogeneous photocatalysis and photothermal Fenton-like reaction†
Received
14th September 2023
, Accepted 17th November 2023
First published on 22nd November 2023
Abstract
Microplastic pollution has attracted considerable attention. Here, we develop a visible-light-driven photocatalysis and photothermal Fenton-like reaction method for their removal, utilizing an α-Fe2O3 nanoflower on TiO2 with a hierarchical structure of inverse opal-like layer/nanotube arrays (α-Fe2O3/TiO2HNTAs film). Without external H2O2 dosage, nearly 100% degradation of 310 nm polystyrene (PS) spheres is obtained after 4 h at 75 °C induced by visible-light irradiation. The light initiation and photothermal effect are essential for achieving high degradation efficiency of plastics on α-Fe2O3. A distinctive melting phenomenon of PS under mild conditions due to the synergistic effect is observed for the first time. The catalyst system also works well for PS spheres with large particle size (2.0–2.9 μm) and real PS plastic foam. We believe that this work provides a novel strategy and new insights for removing microplastics and bulk plastics.
Environmental significance
Microplastic pollution has attracted considerable attention due to its potential adverse effect on the environment and human health. Microplastic removal with solar energy is of significance in terms of the ecological environment and energy utilization. This study reports a synergic mode of heterogeneous photocatalysis and photothermal Fenton-like reaction for highly efficient microplastic degradation.
|
1. Introduction
Nearly 400 million tons of plastics were produced worldwide in 2020.1 Most of them are discarded as waste and could persist for hundreds or even thousands of years, causing severe environmental issues and a potential threat to public health.2,3 For example, microplastics either from industrial production or decomposition of large plastics distribute ubiquitously in water, land, air and oceans.3–5 These small plastics can be easily ingested by various organisms which eventually results in unexpected accumulative and adverse effects on the food chain.3–5 Therefore, removing microplastics from the natural environment is highly desirable.
Advanced oxidation processes (AOPs) are widely utilized for microplastic degradation. Photocatalysis is a branch of AOPs and various photocatalysts have been developed for plastic conversion,2,3,5,6 including TiO2 and ZnO based-semiconductors. The Fenton/Fenton-like reaction is another efficient route for mineralizing microplastics.5,7 However, peroxide dosage such as H2O2 is necessary for the thermal Fenton system and acidic conditions are required to inhibit the formation of iron hydroxides.8 Moreover, relatively high temperatures are always adopted for a high efficiency. For example, 140 °C should be applied to drive microplastic decomposition.9
Numerous studies have been conducted on heterogeneous photo-assisted Fenton/Fenton-like oxidation for removing organic pollutants.10,11 Besides the normal photo-activated process (eqn (1) and (2)), in such a system,
Fe3+ in Fe2O3 or FeOOH could be reduced by the photogenerated electrons, forming the active
Fe2+ centers for H2O2 activation (eqn (4)). Although sometimes external H2O2 is added to improve the efficiency,12–16 its consumption is lowered since in situ H2O2 is generated during the photocatalytic process (eqn (3)). There are still several challenges that need further endeavors: enhance the separation efficiency of h+/e− pairs and electron transfer rate; promote the redox–oxidation cycle of
Fe3+/Fe2+; and accelerate in situ H2O2 formation and activation. To date, the photo-assisted Fenton reaction on Fe2O3 for plastic degradation has not been reported yet. There are only a few reports utilizing iron(III) salts17 and composites of 1 wt% or 2 wt% iron(II) phthalocyanine on TiO2.18
In the organic synthesis field, the coupling of photocatalytic- and photothermal-effects exhibits superior properties to individual effects.19–22 It is promising to achieve such a synergic mode between photocatalysis and photothermal Fenton-like reaction for plastic degradation. Then, the synergistic effect could not only promote the photocatalytic generation of reactive oxidation species h+/˙OH/O2˙−/H2O2 (eqn (1)–(3)) but also accelerate the thermal Fenton reaction, namely, the limiting step of the redox cycle and activation of H2O2 (eqn (4)).
| α-Fe2O3 + hv → h+ + e− | (1) |
| h+ + H2O(air) → ˙OH + H+ | (2a) |
| e− + H2O2 → ˙OH + OH− | (2c) |
In situ H
2O
2 formation:
| 2h+ + H2O(air) → H2O2 + 2H+ | (3a) |
| 2H+ + O2(air) + 2e− → H2O2 | (3b) |
Fenton-like reaction:
| Fe3+ + e− → Fe2+ | (4a) |
| Fe2+ + H2O2 → Fe3+ + ˙OH + OH− | (4b) |
Thus, in this work, we fabricated an α-Fe2O3 nanoflower on TiO2 film with a hierarchical structure of inverse opal-like layer/nanotube arrays (denoted as α-Fe2O3/TiO2HNTAs). Through the synergic oxidation method (Table 1), the degradation of polystyrene (PS) spheres with diameters of 310 nm and 2.0–2.9 μm and real PS plastic foam is investigated and excellent performance is achieved under visible-light illumination. The study of light intensity and temperature demonstrates that light initiation and the photothermal effect are indispensable in our catalytic systems. The melting of PS at temperature much lower than its melting point due to the synergic mode is discovered for the first time. Experimental results also show that TiO2HNTAs is not the catalytic site but it could promote the PS degradation efficiency on α-Fe2O3.
Table 1 Heterogeneous catalytic degradation of microplastics or plastics through various routes reported
Plastic type |
Catalyst |
Methods |
Reaction conditions |
Degradation efficiency |
Ref. |
Small pieces (<0.6 mm, 5.0 g L−1) |
Mn@NCNTs |
Thermal catalysis |
PMS, 120 °C |
41% after 8 h |
23
|
PS small pieces (<1 mm, 1.0 g L−1) |
Fe3O4 |
Adsorption and thermal catalysis |
H2O2, ≈200–250 °C |
100% after 12 h |
24
|
PVC (100–200 μ m, 0.1 g L−1) |
TiO2/graphite cathode |
Electrocatalysis |
pH = 3, −0.7 V vs. Ag/AgCl, 100 °C |
56% after 6 h |
25
|
PS film |
Bacteria + homogeneous Fe(III) |
Microbial Fenton |
pH = 7, 25 °C |
6.1% after 14 d |
7
|
PS, PE, PP (<100 μm, 1.0 g L−1) |
Homogeneous Fe(II) |
Thermal Fenton |
pH ≤ 1, H2O2, 140 °C |
>90% after 16 h |
9
|
PE sphere (200–250 μm, 1.0 g L−1) |
BiOCl–X |
Photocatalysis |
Visible-light (250 W Xe lamp) |
5.4% after 5 h |
26
|
PVC film |
Dye-ZnO |
UV light, 356 nm |
20% after 2 h |
27
|
PE film |
TiO2 |
UV light, 254 nm |
86% after 300 h |
28
|
PE small pieces (<210 μm, 4.0 g L−1) |
C, N–TiO2 |
pH = 3, visible-light (50 W LED lamp) |
71.8% after 50 h |
29
|
PS sphere (400 nm) |
TiO2 film |
UV light, 365 nm |
98.4% after 12 h |
30
|
PS film |
FePc–TiO2 |
UV light, 254 nm |
82% after 20 d |
18
|
PE film |
Goethite |
UV light, 254 nm |
16% after 300 h |
31
|
PS film |
CuPc–TiO2 |
UV-visible-light, 310–750 nm |
16% after 250 h |
32
|
PS sphere (310 nm) |
α-Fe2O3/TiO2HNTAs film |
Photocatalysis and photothermal Fenton-like reaction
|
In air, visible-light (0.5 W cm−2, halogen lamp), 75 °C induced by light irradiation (catalyst surface temperature is 80–90 °C) |
Almost completely degrade after 4 h to 12 h |
This work |
PS sphere (2.0–2.9 μm) |
PS plastic foam |
|
|
2. Materials and methods
2.1. Chemicals and materials
A titanium sheet with a thickness of 0.2 mm was cut into slices (1 cm × 2.5 cm). All chemicals including ethylene glycol (EG, 99%), ammonium fluoride (NH4F, 98%), iron nitrate (Fe(NO3)3·9H2O, 98%), carboxylated polystyrene micro-spheres (310 nm, Aladdin Biochemical Technology Co.), carboxylated polystyrene (2.0–2.9 μm, Shanghai Macklin Biochemical Co.), and polystyrene plastic foam were used as received. All aqueous solutions were prepared using ultrapure water with a resistivity of 18.2 MΩ cm.
2.2. Catalyst preparation
Hierarchical TiO2 consisting of a top photonic crystal layer and a bottom tubular structure (TiO2HNTAs) was prepared by a two-step anodic oxidation process:33 the Ti slices were firstly sonicated in acetone, deionized water, and ethanol for 10 min respectively. After drying with N2 flow, anodic oxidation was carried out on a DC power supply utilizing the Ti slice (1 cm × 1 cm working area) as an anode and a platinum foil (1 cm × 1 cm) as a cathode. 0.3 wt% ammonium fluoride, 2% v/v water in a solution of ethylene glycol, was used as the electrolyte. In the first step of the anodic oxidation process, a potential of 50 V was applied for 3 h. After anodization, the obtained films were immediately immersed in ethanol for 5 min and then sonicated in ultrapure water for 10 min to remove the formed nanotubes, leaving hexagonal nanoimprints on the Ti surface. In the second anodization step, a potential of 50 V was applied for 1 h. The prepared TiO2HNTAs samples were then immersed in ethanol immediately for 5 min and rinsed with ethanol. Finally, the samples were annealed at 450 °C for 3 h to obtain anatase titanium dioxide with high crystallinity.
Preparation of α-Fe2O3/TiO2HNTAs: 1.01 g of iron nitrate nonahydrate was ultrasonically dissolved in 50 mL water and then sealed with a heat-resistant sealing film. The TiO2HNTAs was vertically immersed into the solution through the slit in the middle of the sealed film. Then the solution was stirred at 90 °C for a certain time (1 h, 2 h or 3 h). The obtained sample slice was taken out and rinsed with ethanol and finally calcined at 550 °C for 1 h to obtain α-Fe2O3/TiO2HNTAs. α-Fe2O3/TiO2HNTAs-1, α-Fe2O3/TiO2HNTAs-2 and α-Fe2O3/TiO2HNTAs-3 refer to the hydrothermal time of 1 h, 2 h and 3 h, respectively.
2.3. Characterization
The X-ray diffraction (XRD) patterns of the samples were recorded on a Rigaku Ultima IV diffractometer using Cu Kα radiation (λ = 1.5418 Å). The light absorption spectra of the samples were determined using a UV-2600 spectrophotometer (Shimadzu). Fourier transform infrared (FTIR) spectroscopy was recorded using an iS50 infrared spectrometer (Thermo) with wavenumber of 4000–400 cm−1. To investigate the valence state of Fe and the local environment of C and O, X-ray photoelectron spectroscopy (XPS) measurements were performed (Thermo Scientific Escalab 250Xi). The morphology of the samples was investigated by FE-SEM (Merlin Compact).
2.4. Activity test
PS sphere degradation: 1.0 mL of polystyrene microspheres (2.5 wt% in DI water, 310 nm) was diluted with 9.0 mL ultrapure water. After ultrasonication, 100 μL of the mixture was dropped and coated on the prepared α-Fe2O3/TiO2HNTAs via a spin-coating method with a rotational speed of 1660 rpm for 40 s. After spin-coating, it was heated at 90 °C on an electric heating plate for 10 min to prevent the PS spheres from falling off the catalyst surface. The photocatalytic process was conducted utilizing a halogen lamp as the visible-light source with a light intensity of 0.5 W cm−2 from 2 h, 3 h to 4 h. The environmental temperature was 75 °C, induced by light irradiation, and the surface temperature of the catalyst monitored using a thermometer (JK804, Changzhou Jinailian Electronic Technology Co.) was 80–90 °C, if not specified. The degradation of monodispersed PS spheres was characterized with SEM. The mineralization product CO2 was characterized by gas chromatography. The reaction in the dark was conducted in an oven at 75 °C for 4 h. A Hg lamp (365 nm, 0.5 W cm−2) was utilized as the light source for the experiments under UV light irradiation.
The experimental procedure for PS spheres with large particle size is the same as above.
Real PS plastic foam: 0.03 g PS plastic foam was first dissolved in 1 mL dichloromethane. Then 100 μL of the solution was dropped uniformly on the prepared α-Fe2O3/TiO2HNTAs and kept still, forming a transparent film after 5 h. The film on α-Fe2O3/TiO2HNTAs was irradiated with visible-light for 12 h under the same experimental conditions as above.
Liquid product test with 1H NMR: during the photocatalytic reaction for a certain time, the sample was immersed in dichloromethane and then the solution was taken for an NMR test. The solvent for the NMR test was CDCl3.
Radical scavenger experiments: the experimental process is the same as the PS sphere degradation experiment except that 0.05 g DMPO was dissolved in 1.0 mL of the diluted PS sphere solution.
3. Results and discussion
3.1. Characterization of catalysts and degradation of PS spheres
The catalyst preparation process is given in Fig. 1a and the crystal planes of the α-Fe2O3 phase and TiO2 anatase phase could be confirmed by the XRD pattern in Fig. 1b.12,34 As shown in Fig. 1c, both the TiO2HNTAs film and its scratched powder exhibit visible-light absorption, which is further enhanced for the former. This is caused by F doping formed during the anodization process and by the hierarchical structure of inverse opal-like layer/nanotube arrays (Fig. 1d), which has been reported in the literature and our former works.33 The absorption edge extends to 700 nm for the deposited α-Fe2O3 samples (Fig. 1c).
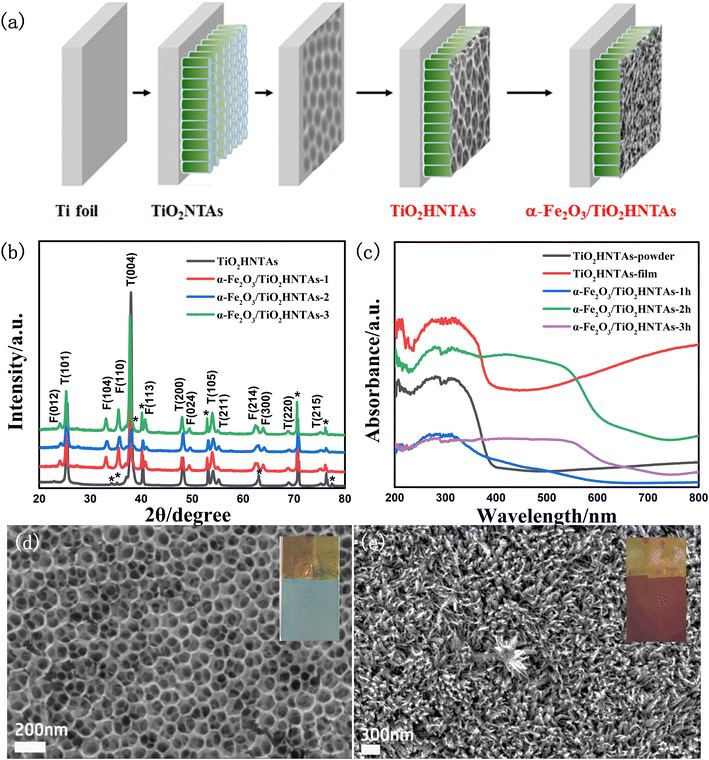 |
| Fig. 1 (a) Process for the catalyst preparation. (b) XRD pattern (*: Ti foil, T: TiO2, F: α-Fe2O3) and (c) UV-vis spectra of α-Fe2O3/TiO2HNTAs (deposited for 1 h, 2 h, 3 h) and TiO2HNTAs. The samples were scratched off from the Ti foil for the UV-vis test. The UV-vis adsorption spectrum of TiO2HNTAs film is also provided. Corresponding SEM images and digital photos (inset) for TiO2HNTAs (d) and α-Fe2O3/TiO2HNTAs (e). | |
For the TiO2HNTAs film, the average pore size for the inverse opal-like layer is 120 nm according to the pore size distribution analysis (Fig. S1a†), while the average length of the bottom nanotube array is 4.3–4.4 μm according to the cross-section image and length distribution analysis (Fig. S1b†). After deposition, the α-Fe2O3 nanoflower is formed on TiO2HNTAs (Fig. 1e) and its average thickness increases from 260 nm, 310 nm to 330 nm for deposition time of 1 h, 2 h, and 3 h, respectively (Fig. S2†). Compared with other catalysts, the sample with a deposition time of 2 h achieved nearly 100% PS degradation (Fig. S3†) and was utilized as a model photocatalyst for the following investigation, marked as α-Fe2O3/TiO2HNTAs.
We first compare the PS (310 nm) degradation on TiO2HNTAs and α-Fe2O3/TiO2HNTAs. The visible-light intensity is 0.5 W cm−2 and the reaction temperature is 75 °C, induced by light irradiation. As shown in Fig. 2a, although TiO2HNTAs could be excited by visible-light, there is no degradation of PS on TiO2HNTAs after irradiating for 4 h even up to 12 h. For α-Fe2O3/TiO2HNTAs, the PS melted after 2 h (Fig. 2b), which is probably caused by the stretching and opening of carbon chains as well as the decomposition of the polymer forming reaction intermediates. The corresponding SEM-EDS mapping is given in Fig. S5.† This is a totally different phenomenon compared with TiO2HNTAs under UV-light irradiation, where only the size of the PS sphere becomes smaller and smaller from 6 h to 12 h until it disappears after 24 h (Fig. S6†). The melting of PS could also result in a large surface area of interfacial contact between PS and α-Fe2O3 and in turn accelerate the degradation rate. Complete degradation is achieved on α-Fe2O3/TiO2HNTAs after 4 h (Fig. 2d). 1H NMR results also confirm that the intermediate species disappeared after complete degradation (Fig. S8†). During the photocatalytic reaction, the real temperature of the catalyst surface remained at 80–90 °C due to light irradiation (Fig. 2e).
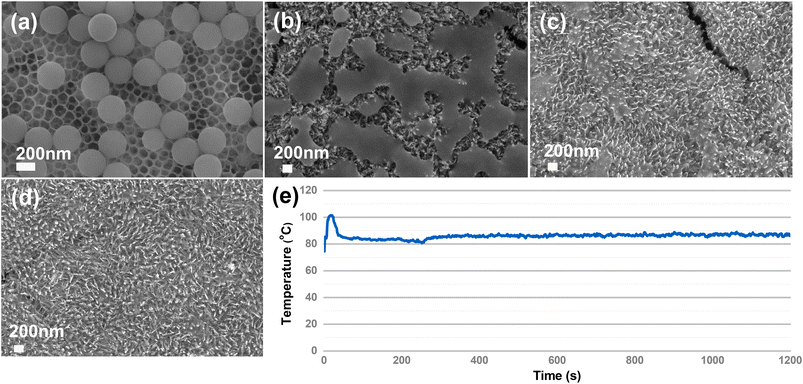 |
| Fig. 2 The degradation of PS on TiO2HNTAs after irradiating for (a) 4 h to 12 h; on α-Fe2O3/TiO2HNTAs after irradiating for 2 h (b), 3 h (c), and 4 h (d). The SEM images at 1 μm scale for (a–d) are provided in Fig. S4.† (e) The real-time temperature monitoring of the catalyst surface during reaction with a thermometer. The environmental temperature was 75 °C induced by visible-light irradiation, 0.5 W cm−2. | |
An XPS test was also conducted for α-Fe2O3/TiO2HNTAs and the sample coated with PS spheres before and after photodegradation for 2 h. The analysis of C1s and O1s is given in Fig. 3, while Fe2p and full spectra are provided in Fig. S7.† For C1s, the peak at 284.8 eV ascribed to the C–C bond increased a lot after coating of PS spheres when compared with pure α-Fe2O3/TiO2HNTAs. The new small peak at 288.9 eV and the extended peak at 291.5 eV are ascribed to C
O and –COO groups of carboxylated PS spheres, respectively. After light illumination for 2 h, the content of the C–C bond decreased dramatically, indicating the degradation of the carbon chain in PS. For O1s, the binding energy at 530.0 eV is assigned to the lattice oxygen, while that at 531.7 eV is assigned to the typical oxygen groups such as carbonyl and OH.28 After coating of carboxylated PS spheres, a new peak at 533.3 eV, which comes from the –COOH group, is observed and the peak area increases after illuminating for 2 h. The increase of this peak is probably induced by the formation of carboxylic acids or carboxylates as intermediate products during the degradation process.
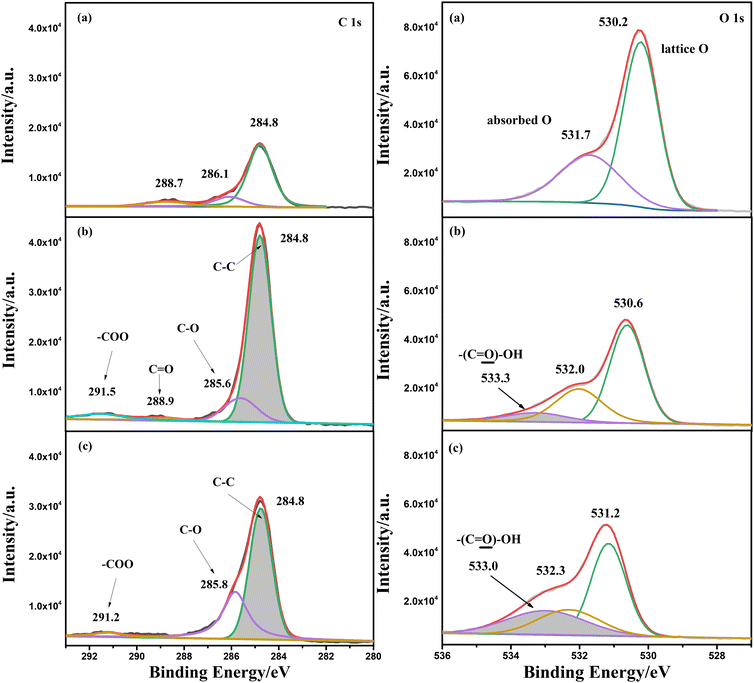 |
| Fig. 3 XPS analysis of C1s and O1s for (a) α-Fe2O3/TiO2HNTAs and (b and c) PS sphere coated α-Fe2O3/TiO2HNTAs before and after photodegradation. The degradation time is 2 h. The Fe2p and full spectra are given in Fig. S7.† | |
3.2. Influence of light and temperature
Utilizing α-Fe2O3/TiO2HNTAs as a catalyst, we investigate the light effect on the PS photodegradation efficiency. The degradation rate increases with the increase of light intensity and the results are listed in Fig. 4. In the absence of light (0 W cm−2), no degradation was observed. The size and shape of PS spheres remained the same when the reaction system was heated to 90 °C in the dark. Even up to 130 °C, the PS on α-Fe2O3/TiO2HNTAs are still capable of keeping the spherical structure with only a slight deformation (Fig. S9†). These results demonstrate that light initiation is essential for the reaction. With light irradiation at 0.33 W cm−2, a melting phenomenon and obvious degradation of PS could be observed (Fig. S10†). Nearly 100% mineralization is obtained when the light intensity increased to 0.5 W cm−2. The influence of light wavelength on the activity was then studied while keeping the light intensity and reaction temperature unchanged. The PS spheres melt and degrade even with the light wavelength over 660 nm (Fig. S11†) and the performance is in the order of 400–800 nm > 550–800 nm > 660–800 nm.
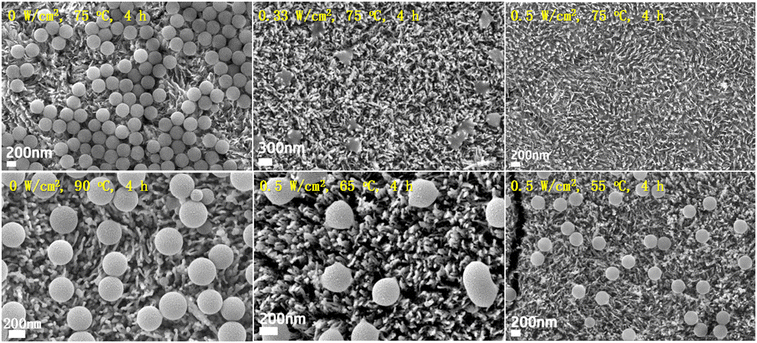 |
| Fig. 4 The influence of light intensity and temperature on PS degradation over α-Fe2O3/TiO2HNTAs. Under light irradiation, the temperature was controlled by the heating or cooling system. In the absence of light, the experiment was conducted in an oven at certain temperature. | |
Moreover, the temperature under visible-light irradiation (0.5 W cm−2) is varied by cooling systems. When the temperature is down to 65 °C, the degradation efficiency decreases but the melting process still occurs. With a continuous decrease of temperature to 55 °C, only a slight reduction of PS size from 310 nm to ∼296 nm is observed and the size distribution is shown in Fig. S12.† It can be concluded that the high temperature induced by light irradiation is also required for the high degradation rate. The above bare light system at low temperature and bare heating experiments in the dark indicate that there is a synergistic effect between light initiation and Fenton-like reaction (thermal catalysis) to overcome the activation energy and drive the reaction.
3.3. Large size of PS spheres
Large size PS spheres were studied. We purchased PS spheres with a diameter of 2.0–2.9 μm as a model. The experimental procedure was the same as the PS with a diameter of 300 nm. After 4 h of irradiation, the PS spheres are almost completely degraded (Fig. 5a and b), which demonstrates that the catalytic system works well for nano-microplastics with large size. The EDS mapping in Fig. 5b and c confirms that the particle is the remaining part of the PS sphere rather than the surface Fe2O3 particle. Compared with the complete degradation of PS with a diameter of 300 nm, the results are consistent with the fact that larger size plastics require a longer degradation time than smaller ones.
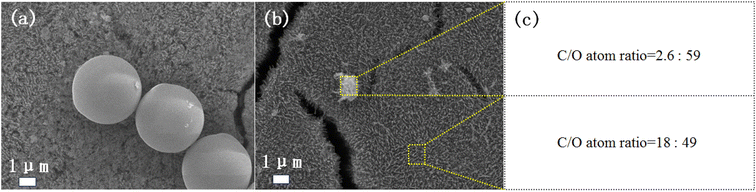 |
| Fig. 5 SEM images for PS spheres with a diameter of 2.0–2.9 μm before (a) and after photocatalysis for 4 h (b), and the corresponding EDS mapping results (c). The environmental temperature was 75 °C induced by visible-light irradiation, 0.5 W cm−2. | |
3.4. Mineralization of real PS plastic foam
Real plastic foam was also studied with our catalyst system. The polystyrene plastic foam was first dissolved in dichloromethane and then coated onto the surface of α-Fe2O3/TiO2HNTAs to form a transparent thin film. The SEM image of the film is shown in Fig. 6a. After visible-light irradiation for 12 h, more than 90% of the film disappeared and the exposed surface of α-Fe2O3/TiO2HNTAs was left (Fig. 6b). Such a performance is among the highest active systems that have been reported (Table 1). The corresponding FT-IR characterization is conducted and given in Fig. 6c. The major bands at 2917 cm−1 and 2849 cm−1 are the typical hydrocarbon stretching,35 or can be ascribed to asymmetric and symmetric stretching vibrations of the CH2 group.9,25 The peak at 1595 cm−1 is from the C
C group and the major peak at 1445 cm−1 is from C–H.35 These peaks for hydrocarbons including C–H, CH2, and C
C dramatically decrease after photodegradation. Compared with the undegraded film, new peaks at 1732 cm−1 and 1630 cm−1 assigned to C
O of carboxylic acid or ketone groups appeared, which are reaction intermediates of PS degradation in air.9,25,35,36 These are consistent with the observed results from SEM images. The possible final liquid product and gas product were detected. A liquid product is not observed according to the result of the NMR test (Fig. 6d and e), while the mineralization product CO2 is confirmed by gas chromatography in a sealed system and only CO2 was detected as the gas product (Fig. 6f). These further confirm the complete degradation of the plastic to CO2.
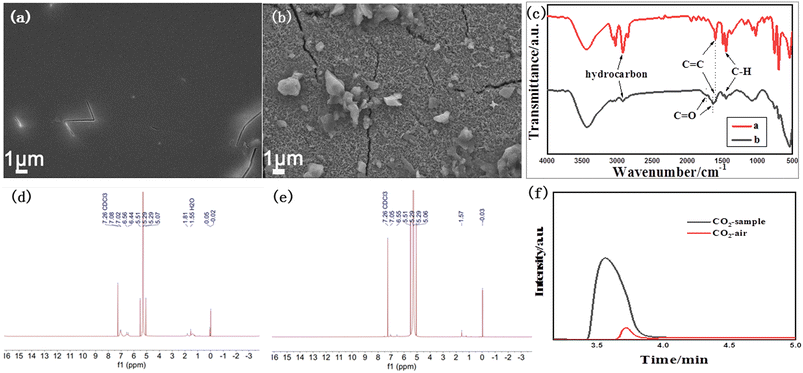 |
| Fig. 6 The degradation of real plastic waste on α-Fe2O3/TiO2HNTAs. (a) The plastic film was prepared from PS plastic foam via the solvent dissolution and dropping method. (b) Photodegradation after 12 h under visible-light irradiation, 75 °C, 0.5 W cm−2. (c) Corresponding FT-IR spectra for (a) and (b). (d–f) The NMR and GC spectra for the liquid product and gas product. (d and e) 1H NMR spectra for the PS plastic film before and after photodegradation on the catalyst. (f) GC test for the mineralization product CO2 in a sealed system for the sample and no other gas product was observed (CO2, CH4, C2H4, C2H6 standard gas were used as reference, high concentration of CO2 will lead to the left shift of retention time). | |
3.5. Mechanism investigation
Amylopectin and KI were used to confirm the formation of H2O2 for the light-excited α-Fe2O3 powder system in PS sphere solution (75 °C, 0.5 W cm−2). The reaction solution was filtered after the reaction. After adding amylopectin and KI, the color of the filtrate obviously changed (Fig. 7a, ii). Without light irradiation, no color change was observed at 75 °C (Fig. 7b). As also shown in Fig. 7b, for the experiment at 55 °C with a light intensity of 0.5 W cm−2, there was much less color change than that at 75 °C with the same light intensity (Fig. 7a-ii). This demonstrates that light irradiation and the thermal effect by light illumination are essential to produce a high concentration of H2O2. Under the same experimental conditions as in Fig. 2d, the presence of radical scavenger DMPO dramatically decreases the mineralization rate of PS (Fig. 7c and d). All these experiments confirm that the synergistic effect of photocatalysis and the photothermal effect contributes to H2O2 production and the high degradation rate of PS.
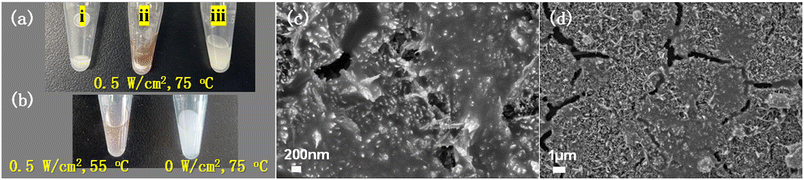 |
| Fig. 7 (a and b) Control experiments for H2O2 formation in the filtrate after light irradiation with α-Fe2O3 as a catalyst: (a) i-KI, ii-amylopectin and KI, iii-amylopectin, visible-light irradiation (75 °C); (b) amylopectin and KI, visible-light irradiation (55 °C) and in the dark (75 °C). (c and d) SEM image after reaction with radical scavenger DMPO on α-Fe2O3/TiO2HNTAs. Reaction conditions: visible-light irradiation for 4 h, 75 °C, 0.5 W cm−2. | |
The role of α-Fe2O3 was further investigated. We deposited Cu2O on TiO2HNTAs and applied it in PS degradation. The results are given in Fig. S13.† Although both Cu2O and Fe2O3 possess strong visible-light absorption bands, the activity of Cu2O is much lower than that of α-Fe2O3, probably caused by the Fenton-like reaction process of
Fe3+/Fe2+ in eqn (4) and (4b). Based on the investigation above, we proposed the possible mechanism for PS degradation via photocatalysis and photothermal Fenton-like reaction on α-Fe2O3. As shown in Scheme 1, the α-Fe2O3 semiconductor is excited by visible-light irradiation and generates electron/hole pairs. The electrons could not only activate O2 producing in situ H2O2, but also reduce
Fe3+ in Fe2O3 forming the active
Fe2+ centers. Then,
Fe2+ reacts with H2O2 and ·OH forms. At the same time,
Fe2+ returns back to its original
Fe3+ state. This photo-assisted Fenton-like reaction process is promoted by the photothermal effect (red color). The holes generated in the valence band of α-Fe2O3 are also able to oxidize the moisture in air and generate ·OH. The ·OH with high oxidizing power attacks the PS on the α-Fe2O3 surface, oxidizing PS to intermediate species and then to the final product CO2. This oxidation process is dramatically accelerated by the melting phenomenon due to the close and large contact area at the PS/catalyst solid–solid interface. To further investigate the possible radical species, an EPR test was conducted for the methanol solution with the addition of DMPO after immersing the α-Fe2O3/TiO2HNTAs film under light irradiation. According to ref. 37 and 38 and the calculated splitting constant (Fig. S14†), the formation of O2˙− and ·OH was confirmed.
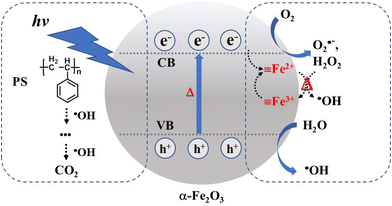 |
| Scheme 1 Proposed mechanism for the degradation of PS via photocatalysis and photothermal Fenton-like reaction on α-Fe2O3. CB – conduction band, VB – valence band. | |
When utilizing α-Fe2O3 deposited on Ti foil as a catalyst, the melting of PS and a high degradation rate are observed (Fig. S15†) which indicates lower activity than α-Fe2O3/TiO2HNTAs. Thus, we believe that α-Fe2O3 is the catalytic site and plays a major role in PS mineralization, while TiO2HNTAs makes a minor contribution and could promote the degradation efficiency of PS on α-Fe2O3. The enhanced efficiency is either because visible-light-excited TiO2HNTAs could populate electrons to the conduction band of α-Fe2O3 or the light adsorption energy of the TiO2HNTAs film matches with the energy level of α-Fe2O3 generating h+/e− pairs in α-Fe2O3.
3.6. Recyclability
To illustrate the stability of the photocatalyst, we repeat the photodegradation process three times. It can be seen from Fig. 8a and b that excellent degradation efficiency could still be obtained and there is no morphology change for the α-Fe2O3/TiO2HNTAs catalyst. There is also no crystal phase change of α-Fe2O3/TiO2HNTAs after recycling (Fig. 8c).
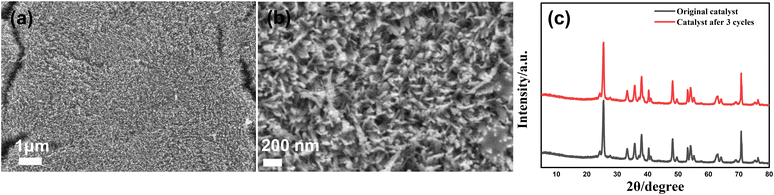 |
| Fig. 8 Repeating PS degradation on α-Fe2O3/TiO2HNTAs three times. (a and b) The SEM images and (c) XRD of the catalyst after the 3rd cycle. | |
4. Conclusion
In summary, we demonstrate that the synergistic effect between heterogeneous photocatalysis and photothermal Fenton-like reaction could dramatically accelerate the PS degradation rate on the α-Fe2O3/TiO2HNTAs surface. The light initiation and photothermal effect are essential for the melting phenomenon of PS driven at a much lower temperature than its melting point. PS spheres with nanosize and microsize diameters as well as real PS plastic foam almost completely degrade after 4 h to 12 h, under visible-light (0.5 W cm−2, halogen lamp) irradiation and at 75 °C induced by light irradiation (catalyst surface temperature is 80–90 °C).
Author contributions
Z. H. Xue: investigation, methodology, validation, data curation. X. Yu: investigation, validation. X. B. Ke: writing – reviewing & editing. Jian Zhao: supervision, conceptualization, writing – original draft and reviewing & editing.
Conflicts of interest
There is no conflict of interests.
Acknowledgements
The National Natural Science Foundation of China (NSFC, 21703154) and the Natural Science Foundation of Tianjin (18JCYBJC43100) are gratefully acknowledged.
References
- R. Cao, M. Q. Zhang, C. Hu, D. Xiao, M. Wang and D. Ma, Catalytic oxidation of polystyrene to aromatic oxygenates over a graphitic carbon nitride catalyst, Nat. Commun., 2022, 13, 4809 CrossRef CAS PubMed.
- X. Jiao, K. Zheng, Z. Hu, S. Zhu, Y. Sun and Y. Xie, Catalytic oxidation of polystyrene to aromatic oxygenates over a graphitic carbon nitride catalyst, Adv. Mater., 2021, 33, 2002192 Search PubMed.
- H. Du, Y. Xie and J. Wang, Microplastic degradation methods and corresponding degradation mechanism: research status and future perspectives, J. Hazard. Mater., 2021, 418, 126377 CrossRef CAS PubMed.
- K. Hu, Y. Yang, J. Zuo, W. Tian, Y. Wang, X. Duan and S. Wang, Emerging microplastics in the environment: properties, distributions, and impacts, Chemosphere, 2022, 297, 134118 CrossRef CAS PubMed.
- K. Hu, W. Tian, Y. Yang, G. Nie, P. Zhou, Y. Wang, X. Duan and S. Wang, Microplastics remediation in aqueous systems: strategies and technologies, Water Res., 2021, 198, 117144 CrossRef CAS PubMed.
- S. Chu, B. Zhang, X. Zhao, H. S. Soo, F. Wang, R. Xiao and H. Zhang, Microplastics remediation in aqueous systems: strategies and technologies, Adv. Energy Mater., 2022, 12, 2200435 CrossRef CAS.
- Y. Yang, J. Chen, Z. Chen, Z. Yu, J. Xue, T. Luan, S. Chen and S. Zhou, Microplastics remediation in aqueous systems: strategies and technologies, Water Res., 2022, 223, 118979 CrossRef CAS PubMed.
- P. Liu, L. Qian, H. Wang, X. Zhan, K. Lu, C. Gu and S. Gao, New insights into the aging behavior of microplastics accelerated by advanced oxidation processes, Environ. Sci. Technol., 2019, 53, 3579–3588 CrossRef CAS PubMed.
- K. Hu, P. Zhou, Y. Yang, T. Hall, G. Nie, Y. Yao, X. Duan and S. Wang, Degradation of microplastics by a thermal Fenton reaction, ACS ES&T Engg, 2021, 2, 110–120 Search PubMed.
- Y. Liu, Y. Zhao and J. Wang, Fenton/Fenton-like processes with in situ production of hydrogen peroxide/hydroxyl radical for degradation of emerging contaminants: advances and prospects, J. Hazard. Mater., 2021, 404, 124191 CrossRef CAS PubMed.
- X. Wang, X. Zhang, Y. Zhang, Y. Wang, S.-P. Sun, W. D. Wu and Z. Wu, Fenton/Fenton-like processes with in situ production of hydrogen peroxide/hydroxyl radical for degradation of emerging contaminants: advances and prospects, J. Mater. Chem. A, 2020, 8, 15513–15546 RSC.
- S. Han, L. Hu, Z. Liang, S. Wageh, A. A. Al-Ghamdi, Y. Chen and X. Fang, One-step hydrothermal synthesis of 2D hexagonal nanoplates of α-Fe2O3/graphene composites with enhanced photocatalytic activity, Adv. Funct. Mater., 2014, 24, 5719–5727 CrossRef CAS.
- X. Qian, M. Ren, Y. Zhu, D. Yue, Y. Han, J. Jia and Y. Zhao, Visible light assisted heterogeneous Fenton-like degradation of organic pollutant via α-FeOOH/mesoporous carbon composites, Environ. Sci. Technol., 2017, 51, 3993–4000 CrossRef CAS PubMed.
- Q. Li, G. Wei, Y. Yang, Z. Li, L. Zhang, L. Shao and S. Lai, Insight into the enhanced catalytic activity of a red mud based Fe2O3/Zn–Al layered double hydroxide in the photo-Fenton reaction, Catal. Sci. Technol., 2020, 10, 7365–7377 RSC.
- Y. Zhang, L. Wu, Y. Wang, Y. Zhang, H. Wang, X. Wang, X. D. Chen and Z. Wu, Highly dispersed titania-supported iron oxide catalysts for efficient heterogeneous photo-Fenton oxidation: influencing factors, synergistic effects and mechanism insight, J. Colloid Interface Sci., 2021, 587, 467–478 CrossRef CAS PubMed.
- L. Wu, G. Jiang, X. Wang, Y. Wang, Y. Zhou and Z. Wu, Highly dispersed titania-supported iron oxide catalysts for efficient heterogeneous photo-Fenton oxidation: influencing factors, synergistic effects and mechanism insight, J. Colloid Interface Sci., 2022, 622, 62–74 CrossRef CAS PubMed.
- H. M. Feng, J. C. Zheng, N. Y. Lei, L. Yu, K. H. K. Kong, H. Q. Yu, T. C. Lau and M. H. W. Lam, Photoassisted Fenton degradation of polystyrene, Environ. Sci. Technol., 2011, 45, 744–750 CrossRef CAS PubMed.
- W. Fa, L. Zan, C. Gong, J. Zhong and K. Deng, Solid-phase photocatalytic degradation of polystyrene with TiO2 modified by iron (II) phthalocyanine, Appl. Catal., B, 2008, 79, 216–223 CrossRef CAS.
- J. Zhao, J. Wang, A. J. Brock and H. Zhu, Plasmonic heterogeneous catalysis for organic transformations, J. Photochem. Photobiol., C, 2022, 52, 100539 CrossRef CAS.
- R. Ma, J. Sun, D. H. Li and J. J. Wei, Review of synergistic photo-thermo-catalysis: mechanisms, materials and applications, Int. J. Hydrogen Energy, 2020, 45, 30288–30324 CrossRef CAS.
- J. Zhao, S. Xue, R. Ji, B. Li and J. Li, Localized surface plasmon resonance for enhanced electrocatalysis, Chem. Soc. Rev., 2021, 50, 12070–12097 RSC.
- Y. Zhou and J. Zhao, Glaser coupling-into Sonogashira coupling-control over CuxO nanoparticles/carbon nanotube by switching visible-light off and on, Appl. Catal., B, 2022, 300, 120721 CrossRef CAS.
- J. Kang, L. Zhou, X. Duan, H. Sun, Z. Ao and S. Wang, Glaser coupling-into Sonogashira coupling-control over CuxO nanoparticles/carbon nanotube by switching visible-light off and on, Matter, 2019, 1, 745–758 CrossRef CAS.
- M. Zandieh and J. Liu, Removal and degradation of microplastics using the magnetic and nanozyme activities of bare iron oxide nanoaggregates, Angew. Chem., Int. Ed., 2022, 61, e202212013 CrossRef CAS PubMed.
- F. Miao, Y. Liu, M. Gao, X. Yu, P. Xiao, M. Wang, S. Wang and X. Wang, Degradation of polyvinyl chloride microplastics via an electro-Fenton-like system with a TiO2/graphite cathode, J. Hazard. Mater., 2020, 399, 123023 CrossRef CAS PubMed.
- R. Jiang, G. Lu, Z. Yan, J. Liu, D. Wu and Y. Wang, Microplastic degradation by hydroxy-rich bismuth oxychloride, J. Hazard. Mater., 2021, 405, 124247 CrossRef CAS PubMed.
- S. Chakrabarti, B. Chaudhuri, S. Bhattacharjee, P. Das and B. K. Dutta, Degradation mechanism and kinetic model for photocatalytic oxidation of PVC-ZnO composite film in presence of a sensitizing dye and UV radiation, J. Hazard. Mater., 2008, 154, 230–236 CrossRef CAS PubMed.
- X. Zhao, Z. Li, Y. Chen, L. Shi and Y. Zhu, Solid-phase photocatalytic degradation of polyethylene plastic under UV and solar light irradiation, J. Mol. Catal. A: Chem., 2007, 268, 101–106 CrossRef CAS.
- M. C. Ariza-Tarazona, J. F. Villarreal-Chiu, J. M. Hernandez-Lopez, J. Rivera De la Rosa, V. Barbieri, C. Siligardi and E. I. Cedillo-Gonzalez, Microplastic pollution reduction by a carbon and nitrogen-doped TiO2: effect of pH and temperature in the photocatalytic degradation process, J. Hazard. Mater., 2020, 395, 122632 CrossRef CAS PubMed.
- I. Nabi, A. U. Bacha, K. Li, H. Cheng, T. Wang, Y. Liu, S. Ajmal, Y. Yang, Y. Feng and L. Zhang, Complete photocatalytic mineralization of microplastic on TiO2 nanoparticle film, iScience, 2020, 23, 101326 CrossRef CAS PubMed.
- G. Liu, D. Zhu, W. Zhou, S. Liao, J. Cui, K. Wu and D. Hamilton, Solid-phase photocatalytic degradation of polystyrene plastic with goethite modified by boron under UV-vis light irradiation, Appl. Surf. Sci., 2010, 256, 2546–2551 CrossRef CAS.
- J. Shang, M. Chai and Y. F. Zhu, Photocatalytic degradation of polystyrene plastic under fluorescent light, Environ. Sci. Technol., 2003, 37, 4494–4499 CrossRef CAS PubMed.
- J. Meng, Y. Xiao, Y. Huang, X. Liu and J. Zhao, Solvent-free hydroamination of phenylacetylene by plasmonic gold nanoparticles coupled with a TiO2 2D photonic layer on nanotube arrays, New J. Chem., 2020, 44, 17289–17295 RSC.
- R. Zhang, M. Sun, G. Zhao, G. Yin and B. Liu, Hierarchical Fe2O3 nanorods/TiO2 nanosheets heterostructure: growth mechanism, enhanced visible-light photocatalytic and photoelectrochemical performances, Appl. Surf. Sci., 2019, 475, 380–388 CrossRef CAS.
- K. Zhu, H. Jia, S. Zhao, T. Xia, X. Guo, T. Wang and L. Zhu, Formation of environmentally persistent free radicals on microplastics under light irradiation, Environ. Sci. Technol., 2019, 53, 8177–8186 CrossRef CAS PubMed.
- Y. Lou, P. Ekaterina, S. S. Yang, B. Lu, B. Liu, N. Ren, P. F. Corvini and D. Xing, Biodegradation of polyethylene and polystyrene by greater wax moth larvae (galleria mellonella L.) and the effect of co-diet supplementation on the core gut microbiome, Environ. Sci. Technol., 2020, 54, 2821–2831 CrossRef CAS PubMed.
- Y. Chen, S. H. Guan, H. Ge, X. Chen, Z. M. Xu, Y. H. Yue, H. Yamashita, H. Yu, H. X. Li and Z. F. Bian, Photocatalytic dissolution of precious metals by TiO2 through photogenerated free radicals, Angew. Chem., Int. Ed., 2022, 61, e202213640 CrossRef CAS PubMed.
- X. Chen, S. H. Guan, J. J. Zhou, H. J. Shang, J. Y. Zhang, F. J. Lv, H. Yu, H. X. Li and Z. F. Bian, Photocatalytic free radical-controlled synthesis of high-performance single-atom catalysts, Angew. Chem., Int. Ed., 2023, e202312734 CAS.
|
This journal is © The Royal Society of Chemistry 2024 |