Synergistic dispersion and biodegradation of oil in seawater based on Janus nanosheets and oil-degrading bacteria†
Received
26th August 2023
, Accepted 6th December 2023
First published on 7th December 2023
Abstract
Marine oil spills cause significant pollution to the global environment. Dispersants are frequently used in such spills to break down the oil slick into smaller droplets, facilitating the biodegradation of the oil. Herein, Janus nanosheets (JNs) were used as a new particulate dispersant, which could act synergistically with bacteria in seawater to remediate marine oil spill pollution efficiently. The JNs not only possessed amphiphilic properties and low toxicity but also demonstrated exceptional emulsification capabilities for oil, synergistically enhancing the remediation process alongside oil-degrading bacteria. Due to the inherent constraints on interfacial rotation and multilayer steric hindrance at the diesel–water interface, the emulsion created by JNs remained highly stable, maintaining a small oil droplet size even after a month. Significantly, the JNs enabled the binding of negatively charged oil-degrading bacteria through electrostatic interactions. Following their combination, the JNs effectively transported bacteria directly to the surface of the oil droplets through the influence of sea waves. This cooperative treatment system not only enhanced the oil's dispersibility but also markedly augmented the biodegradation of the oil when compared to the use of bacteria alone. At a diesel concentration of 6 mL L−1 and a JN concentration of 0.3 g L−1, the bacterial biodegradation of diesel was reduced from 5 days to 3 days, and the diesel biodegradation rate increased from 70% to 85%. These findings underscore the remarkable capacity of JNs to effectively emulsify oil and enhance bacterial oil degradation in seawater.
Environmental significance
With the escalation of offshore oil exploitation and the incidence of maritime oil spills, the ecological devastation caused by marine oil spills is significant. Moreover, due to the sluggish processing cycle of oil by hydrocarbon-degrading bacteria, this hazard persists for extended periods. In this study, a Janus nanosheet material was synthesized using a sol–gel method. The Janus nanosheets possess the capability to emulsify oil–water mixtures due to their amphiphilic nature, while exhibiting low toxicity to oil-degrading bacteria. This synergistic system can expedite the remediation of marine oil spills, presenting a promising approach for addressing this ongoing challenge.
|
1. Introduction
Due to the escalating global demand for oil and the cost-effectiveness of marine transportation, oil spill incidents have become increasingly common. Unfortunately, these occurrences unavoidably result in significant oil pollution in the global environment.1,2 The addition of dispersants can help decrease the surface tension between oil and water, resulting in the dispersion of oil into smaller droplets, and this process contributes to enhancing the biodegradation efficiency of oil droplets.3 Nevertheless, the introduction of dispersants could potentially result in further pollution to marine ecosystems.4,5 Therefore, researchers have developed materials such as bio-surfactants and particle dispersants to emulsify oil spills in the ocean.3 Particulate dispersants mainly include clay, silica, and carbon black, which are predominantly homogeneous particles in nature.6–8 These materials have low toxicity, are readily amenable to surface modification, and the Pickering emulsion formed by them is less affected by environmental factors, such as pH, salinity, and temperature.9
In order to improve the emulsification of solid particles, Casagrande et al. prepared semi-hydrophilic and semi-hydrophobic microbeads to make the particles amphiphilic in 1989, which are also called Janus particles.10 Since then, a range of Janus particles have been engineered, such as claw, snowman, dumbbell, cylinder, and nanosheets.11–14 Janus nanosheets (JNs) are a compelling category of Janus materials due to their substantial aspect ratio, elevated adsorption energy, notable interfacial activity, and pronounced restriction in rotational movement at interfaces.15 To produce JNs, several synthetic strategies have been suggested, encompassing regioselective surface modification, emulsion interface self-assembly sol–gel, and template-assisted sol–gel methods.16–18 The most effective method for the large-scale preparation of JNs is the emulsion interface self-assembly sol–gel method.19 Using this method, particles containing materials like paraffin, zinc spheres, and comparable substances are synthesized at the emulsion interface, with silane acting as the Janus spherical shell originating from the silane precursor. Afterward, the central spherical core is eliminated, and the spherical shell is broken down, resulting in the production of JNs.17,20 To meet various requirements, various types of JNs have been prepared and applied in various fields, such as drug delivery,21 catalysis,22 and pollutant adsorption.23 In the field of emulsion stabilization, JNs with different response types have received some attention; for example, the wettability on both sides of the nanosheets can be turned by exchanging the anion group or adjusting the pH or temperature.24–26 Nevertheless, there are scarce reports available regarding the utilization of JNs as dispersants for addressing oil spills within the marine ecosystem.
As is widely recognized, dispersants are capable of breaking down oil spills into smaller droplets; nonetheless, biodegradation remains the ultimate mechanism for eliminating oil pollutants from the marine environment.27 During an oil spill event, petroleum actively triggers the proliferation of indigenous oil-degrading bacteria by providing petroleum-derived hydrocarbons as carbon and energy sources. However, the process is prolonged due to the challenge of accumulating oil-degrading bacteria on the surface of oil droplets. To achieve both efficient petroleum dispersion and accelerated oil biodegradation, dispersants can be synergistically employed alongside bacteria, utilizing electrical attributes, extracellular polymers, and chemical interactions.9,28,29 Archakunakorn et al. associated chitosan with Escherichia coli (E. coli) to improve the bacterial hydrophobicity and the emulsification index, and droplet size analysis revealed that the chitosan-E. coli system was an excellent emulsion stabilizer.30 Within our research group, it was demonstrated that bacteria modified with poly(allylamine hydrochloride)-attapulgite (PAH-ATP) displayed a remarkable capability for both efficient emulsification and the simultaneous biodegradation of oil pollution.31 These results indicate the significant potential of employing bacterial cells in conjunction with particulate dispersants for oil spill treatment. The robust attachment of bacterial cells to droplet surfaces facilitated by particulate dispersants greatly enhances the rate of oil biodegradation.31,32
In this work, a novel silicon dioxide Janus nanosheet was developed as a dispersant, forming a synergistic partnership with oil-degrading bacteria. Here, the synergistic emulsification and oil degradation of this system was initially explored by simulating oil-contaminated seawater with artificial seawater containing diesel. One side of the prepared JNs was modified with n-octadecyltrimethoxysilane (ODMS) to impart hydrophobic properties, while the other side was treated with γ-aminopropyltriethoxysilane (APTES) to make it hydrophilic and positively charged. Subsequently, oil-degrading bacteria isolated from the sediment of the Loushan River were attracted to the JNs through electrostatic interactions. This synergistic system facilitated bacteria adhering to oil droplet surfaces, thus boosting the biodegradation of oil and providing a novel preliminary approach for addressing marine oil spills.
2. Experimental section
2.1 Materials
Toluene, tetraethyl orthosilicate (TEOS), Luria–Bertani (LB) medium, cyclohexane, glutaraldehyde solution (purity >25%), and artificial sea salt were purchased from Sinopharm Chemical Reagent Co., Ltd. (P.R. China). Styrene (purity >99%) was obtained from Sigma-Aldrich Trading Co. Ltd. (Netherlands). N-Octadecyltrimethoxysilane (ODMS, purity >90%) was obtained from Shanghai Yien Chemical Technology Co., Ltd (P.R. China). Resazurin sodium salt was purchased from Meilunbio (US). 2,2-Azobis(2-methylpropionitrile) and maleic anhydride (purity >99%) were purchased from Aladdin (P.R. China). γ-Aminopropyltriethoxysilane (APTES, purity >98%) was purchased from Aladdin (P.R. China). All the above reagents were analytical grade. N-Hexane (HPLC) was purchased from Merck KgaA (Germany). Section paraffin (melting point: 50–52 °C) was purchased from Shanghai Hualing Healing Equipment Factory (P.R. China). Diesel oil (0#) was purchased from Sinopec gas station (P.R. China). The characteristics of diesel were as follows: kinematic viscosity, 5 mm2 s−1; freezing point, 0 °C; density, 0.85 g cm−3.
2.2 Synthesis of the JNs
First, 15 mL of hydrolyzed styrovine–maleic anhydride (HSMA, aliquot of 10 wt%; the detailed preparation steps are given in the ESI† (Text S1)) was mixed with 75 mL of water, subsequently, 2 mol L−1 aqueous hydrochloric acid was slowly added to adjust the pH to 2.5, and the solution was heated to 70 °C. Concurrently, an oil phase consisting of 25 g of paraffin slices, 5.579 mL of TEOS, 2.118 mL of ODMS, and 1.201 mL of APTES was uniformly mixed at 70 °C. Then, the oil phase was dispersed into HSMA aqueous solution for 5 min at a speed of 10
000 r min−1 with a homogenizer (Ultra-Turrax T10 basic, IKA, Germany), resulting in an oil-in-water emulsion. This emulsion was maintained at 70 °C for 12 h to undergo a sol–gel process.
After the reaction, 100 ml of cyclohexane was added to dissolve the internal paraffin, forming Janus hollow spheres. JNs were obtained by breaking the Janus hollow spheres utilizing an ultrasonic disperser (LC-JY98-IIIDN, Ningbo Scientz Biotechnology Co., Ltd., China). Finally, the JNs were washed with ethanol and dried at 60 °C for 24 h. Fig. S1(a)† shows the JNs production process. After drying, 10 mL tubes were first stripped using an electronic analytical balance, and then 5 mL of the compacted JNs particles was weighed out, resulting in a weight of 0.982 g.
2.3 Culture of the bacteria
The oil-degrading bacteria (Acinetobacter junii AP-1, GenBank: MZ675665.1) used in this experiment were obtained from the laboratory's bacterial bank. A colony of AP-1 was cultivated in LB medium at 30 °C with shaking at 120 rpm for 36 h. After centrifugation at 8000 rpm for 5 min, the oil-degrading bacteria were collected and washed twice with 0.85% NaCl solution. The bacterial suspension was obtained by adding 100 mL 0.1 mol L−1 phosphate buffered brine (pH 6.8).
2.4 Combination of the JNs and AP-1 bacteria
To create artificial seawater, 3.0 g of artificial sea salt was added to 100 mL of water and sterilized at 121 °C for 30 min. Then, 2 mL of the bacterial suspension and JNs were added to the sterilized seawater. Subsequently, the mixtures were dispersed at 8000 rpm for 2 min in conical flasks to make the JNs combine with the bacterial cells by electrostatic attractions. The process for this step is illustrated in Fig. S1(b).†
2.5 Dispersion of diesel
The emulsions were prepared under different JNs concentrations, diesel–water volume ratios, and bacterial concentrations. The mixture of diesel, water (or bacterial suspension), and JNs was dispersed with a homogenizer at 8000 rpm for 2 min, forming water-in-oil emulsions. After standing for 24 h, the emulsions were observed and analyzed.
2.6 Degradation of diesel
Preliminary experiment.
100 mL sterilized artificial seawater and diesel of different volumes were added into conical flasks. After adding 2 mL bacterial suspension, the conical flasks were shaken at 30 °C on a rotary shaker at 150 rpm for 5 days.
Experimental group.
2 mL bacterial suspension and different qualities of JNs were added to the sterilized artificial seawater with the same diesel–water ratio. These mixtures were dispersed at 8000 rpm for 2 min in conical flasks and then placed at 30 °C into a rotary shaker at a rotation speed of 150 rpm. Samples were taken at the time points of 1, 2, 3, 4, 5, 6, and 7 days, respectively.
The biodegraded oily seawater was repeatedly extracted with 50 mL of hexane. Gas chromatography (GC-FID, 7890B-7000C, Agilent Technologies, USA) was used for detection, and the extent of oil depletion was calculated as a percentage of the normalized concentration in the uninoculated oily seawater from that specific day in comparison to the normalized concentration in the inoculated oily seawater.
2.7 Characterization
Structure and morphology analyses of the JNs and bacteria.
The JNs for SEM observation were prepared by vacuum sputtering with Au after being dried at 60 °C. In order to confirm the amphiphilic structure of the prepared JNs, silica particles were loaded as markers on the hydrophilic side; the detailed experimental procedures are reported in the ESI† (Text S2). The marked JNs were visualized by field-emission scanning electron microscopy (FESEM; S-4800, Hitachi, Japan). In order to observe the system of the JNs and bacteria, the bacterial suspension was combined with JNs and shaken for 2 h and chemically fixed with glutaraldehyde; the detailed experimental procedures for the chemical fixation are given in the ESI† (Text S3). The thickness of the JNs was measured by AFM (SPM-9700, Shimadzu, Japan). The surface elements of the JNs were measured by X-ray photoelectron spectrometry (XPS; Thermo Escalab 250Xi, USA).
Measurements of the zeta potentials, interfacial tension, and contact angle.
The zeta potentials of the silica nanosheets, bacteria, and their combination were determined using a zeta nanosizer instrument (ZS90, Malvern Instruments, UK) at a fixed scattering angle of 90°. Interfacial tension was assessed using an automated surface tensiometer (BZY-2, Hengping Instruments, China), which included studying the interfacial tension of the diesel–water and diesel–bacterial suspension systems both in the absence and presence of JNs. A contact angle measurement instrument (OCA 20, Data Physics ES, Germany) was used to analyze the wettability of both sides of the JNs. Here, a few JNs were deposited onto a water surface, allowing the hydrophilic side to interact with water and the hydrophobic side to face the air. After drying, the contact angle of the air-exposed side was measured. Then the dried JNs were stuck with tape so that the hydrophilic side was exposed to air and the hydrophobic side was exposed to the tape. The contact angle of the side exposed to air was also measured.
Emulsification analysis.
The emulsion droplets were imaged by optical microscopy (OM; Leica DM1000 LED, Leica, Germany) and the average droplet size was estimated using nano-measurement software. The emulsification index (EI) was measured by a ruler. The stability of the emulsion was obtained by LUMiFuge (LF110, LUM Instruments, Germany), operating at 1500 rpm for 45 min at room temperature. To evaluate the emulsion stability, transmission profiles were assessed in the middle region between the positions 109.5 and 129 mm within the detection region of 106–130 mm.
Assessing the effect of the JNs on bacterial activity.
The bacteria activity was measured to exclude the inhibition of bacterial growth by the material. First, bacterial growth curve was measured. A colony of AP-1 was added into 100 mL LB medium and bacterial culture suspension was obtained after culturing. The optical density (OD) value of the bacteria was measured every 8 h by UV-visible spectrophotometry (Alpha-1860s, Puyuan Instruments, China), with the detection wavelength set to 600 nm. The OD value represented the bacterial concentration. Second, toxicity tests were conducted. Different qualities of pasteurized JNs and 2 mL of bacterial suspension were added to 100 mL LB medium. Following a 36 h cultivation, the bacterial suspension was diluted and coated on to the plates. The plates were then put in an incubator at 30 °C for 48 h and then the bacterial colony number was counted by the dilution spread plate method. Third, resazurin tests were conducted. Here, suspensions including AP-1 and different concentrations of JNs were prepared and incubated for 15 min. Then, 0.005 mg mL−1 of resazurin dye was added in to the above solution and cultivated for 3 h.
3. Results and discussion
3.1 Characterization of the JNs
As can be seen from Fig. 1(a), the JNs displayed smooth surfaces on both sides and a length of 3–5 μm. This outcome was attributed to the hydrolysis rate of the precursor surpassing its condensation rate under acidic conditions.33 After the negatively charged silica particles were added as a marker, they combined with the amino groups on the hydrophilic side of JNs to form a rough surface through electrostatic interactions, thus proving the successful preparation of the Janus materials, as shown in Fig. 1(b and c). Fig. 1(d) shows the SEM image of AP-1, which manifested a peanut-like shape with an average length of 1 μm. Upon the formation of the admixture of JNs with AP-1, as visually depicted in Fig. 1(e), it became evident that the electronegative characteristics of AP-1 facilitated its electrostatic interaction with the positively charged facet of the JNs. The thickness of the nanosheet, as shown in Fig. 1(f), was 3.23 nm, indicating it was negligible relative to the length of the JNs.
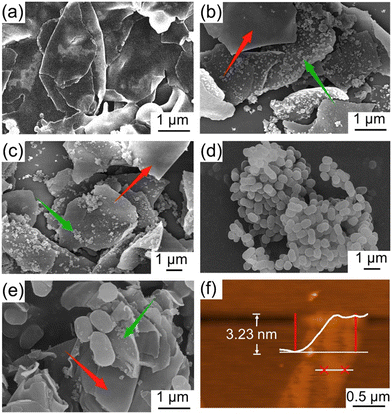 |
| Fig. 1 SEM images of the (a) JNs, (b and c) JNs marked by silica particles, (d) AP-1 bacteria, (e) JNs marked by silica particles combined with AP-1 (hydrophobic side indicated by the red arrow, and hydrophilic side by the green arrow). (f) AFM images of JNs. | |
To validate the presence of grafted functional groups on both sides of the JNs, XPS analysis was carried out and the results are indicated in Fig. 2(a). The full XPS spectrum of the JNs revealed both N and C elements existed, which indicated that ODMS and APTES were successfully grafted on to the surface of SiO2. Further peak-fitting analysis of the JNs showed the high-resolution XPS spectrum of Si2p (Fig. S2†). Notably, the peak at 103.2 eV originated from the R-SiO3 bond and constituted a significant portion, while the presence of SiO2 particles formed during the reaction process was reflected by the peak at 104.3 eV.34 As shown in Fig. 2(b), the C1s peak of the sample was composed of four main components, corresponding to C–C (284.8 eV), C–O/C–N (286.4 eV), C–Si (282.9 eV), and C
O (288.6 eV). The C–C and C–O peaks could be attributed to ODMS, thereby confirming the successful grafting of ODMS onto the surface.35 The C
O peak was attributed to the physical adsorption of hydrocarbon impurities on the surface.36 Combined with the O1s spectrum shown in Fig. 2(c), the three peaks of Si–O (532.6 eV), –OH (531.0 eV), and C–O (534.4 eV) further confirmed our results above. Analysis of the N1s high-resolution spectrum in Fig. 2(d) revealed the emergence of –NH3+ (401.6 eV) and –NH2 (399.6 eV), both attributed to APTES.37 Meanwhile, the appearance of NSiO2 (402.6 eV) indicated that the amino group of APTES combined with the negatively charged SiO2 particles generated during the preparation process.
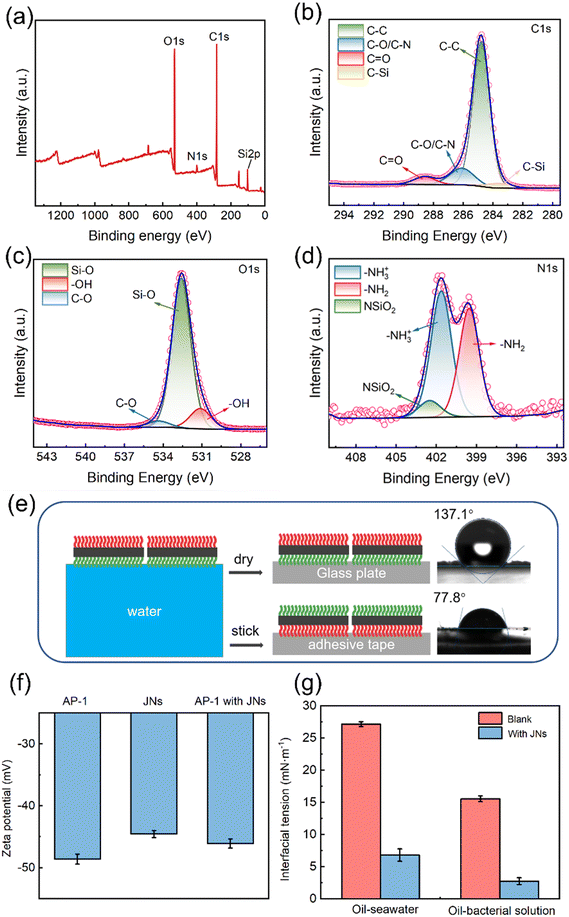 |
| Fig. 2 XPS spectra of the JNs: (a) full spectrum, (b) C1s, (c) O1s, (d) N1s. (e) Water contact angle of the hydrophilic side and hydrophobic side of JNs (green and red depict the hydrophilic and hydrophobic sides of JNs, respectively). (f) Zeta potential distribution of bacteria, JNs aqueous dispersion, and JNs bacteria suspension dispersion. (g) Effect of JNs on the interfacial tension of diesel–water or diesel–bacterial suspension (the concentration of JNs is 8 g L−1). | |
The wettability of the material was analyzed by contact angle measurements. As can be seen from Fig. 2(e), the contact angle of water droplets on the hydrophilic side of the JNs (θN) was about 77.8°, while on the hydrophobic side, it was 137.1° (θC), affirming the amphiphilic nature of the JNs on both sides. The zeta potentials of the JNs and AP-1 were about −44.6 and −48.6 mV, as shown in Fig. 2(f). Upon combining the JNs with the bacterial cells, the zeta potential of the composited material was changed to −46.1 mV, i.e., lying between the values of the JNs and bacterial cells in isolation. This change suggested the occurrence of electrostatic interactions between them. The influence of the JNs and bacterial cells on the interfacial tension between oil and water is shown in Fig. 2(g). The interfacial tension between diesel and seawater was 27.15 mN m−1, whereas it decreased to 15.53 mN m−1 between diesel and bacterial suspension, indicating that the bacteria may produce emulsifiers in the process of metabolism. The introduction of the JNs into the mixture notably diminished the interfacial tension between diesel and seawater to 6.78 mN m−1, as well as between diesel and the bacterial suspension to 2.73 mN m−1, effectively demonstrating the remarkable interfacial activity of the JNs.
3.2 Synergistic emulsification of the JNs and bacteria
JNs can function as an effective Pickering emulsifier in seawater. The size of oil droplets and the emulsification index (EI) can be used to evaluate the stability of an emulsion.38 The EI index refers to the proportion of the emulsion layer volume to the total volume, and EI24 refers to the value of the EI after the emulsion has been left for 24 h. Typically, smaller oil droplet sizes and higher EI values are indicative of effective emulsification. Fig. 3(a and b) shows the changes in the oil droplet size and EI concerning different JNs concentrations and diesel–seawater volume ratios. When maintaining the same diesel–water volume ratio, higher concentrations of JNs were correlated with smaller oil droplet sizes. However, once the JNs concentration surpassed 8 g L−1, the droplet size alterations became slighter. Similarly, at the same concentration of JNs, lower diesel–water volume ratios yielded smaller oil droplet sizes. When the diesel–water ratio was below 1
:
20, the oil droplet size approached about 30 μm. We also compared the dispersion effect of the novel particulate dispersant prepared in this study with some already reported works. As shown in Table S1,† the dispersion of JNs prepared in this work was similar to those of some other dispersants reported in previous studies. The oil droplet size and EI under different bacterial concentrations were also measured, as shown in Fig. 3(c). The concentration of bacteria can be expressed by the OD600 value. It was found that the average size of oil droplets decreased slightly with the increase in bacterial concentration, but the EI value increased slightly. Fig. S3† shows the size and shape of oil droplets in the diesel–seawater and diesel–bacterial suspension systems. It was noteworthy that, when solely utilizing JNs as emulsifiers, the oil droplets took on an oval shape, measuring around 60 μm. However, in the presence of AP-1 cells, the oil droplets became more rounded, with an average droplet size of about 53 μm. This result indicates that the synergistic emulsification of JNs and AP-1 bacteria can generate a more effective Pickering emulsion.
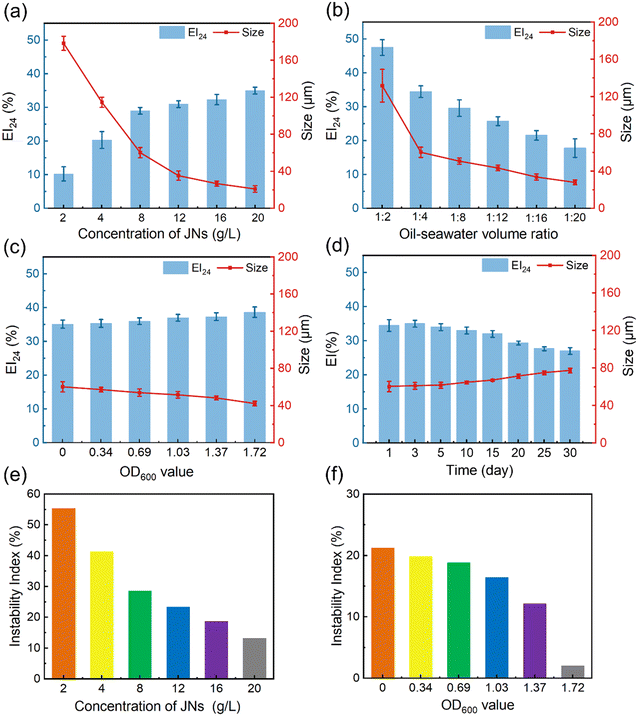 |
| Fig. 3 Dependence of the EI value and oil average droplet size on (a) the JNs concentration (the volume ratio of diesel–seawater was 1 : 4), (b) the volume ratio of diesel–seawater (the concentration of JNs was 8 g L−1), (c) the concentration of bacteria (the concentration of JNs was 8 g L−1, the volume ratio of diesel–seawater was 1 : 4), (d) time (the concentration of JNs was 8 g L−1, the volume ratio of diesel–seawater was 1 : 4). Instability index in different (e) concentrations of JNs (the volume ratio of diesel–seawater was 1 : 4), (f) concentration of bacteria (the concentration of JNs was 8 g L−1, the volume ratio of diesel–seawater was 1 : 4). | |
The diesel–seawater emulsion dispersed by JNs alone was observed for one month and its stability was evaluated by LUMiFuge under centrifugal force. Fig. 3(d) shows that the emulsion maintained a robust emulsifying state for at least one month. Across the initial 15 days, the oil droplet size and EI exhibited minimal fluctuations, while starting from the 15th day, the oil droplet size gradually increased to 77 μm. LUMifuge recorded the transmission profiles based on the position and time of all the emulsion samples (Fig. S4†). Using SEPView 6.3 software, the instability index was derived by dividing the clarification rate at a specific separation time by the maximum clarification rate.39 A smaller instability index means a more stable emulsion. Fig. 3(e) presents the trend of the instability index of emulsions with the incorporation of different concentrations of JNs. It could be concluded that JNs play an important role in stabilizing the emulsion. With the increase in the JNs concentration, the instability index of the emulsion decreased rapidly at first and then slowed down, which means that excessive JNs had little effect on the emulsion stability. At a concentration of 8 g L−1 JNs, the instability index was 28.5%. Fig. 3(f) shows the stability of the emulsions formed by the JNs and different concentrations of bacteria. Initially, the instability index diminished gradually, followed by a sharper decline in response to increasing the bacterial concentration. When OD600 = 1.72, the emulsion stability increased significantly. This also implied the great synergistic stabilization between the JNs and bacterial cells. The mechanism for this is analyzed in the next section.
3.3 Stabilization mechanism of the emulsions
Based on the preceding emulsification findings, the stabilization mechanism underlying the dispersion of emulsions by JNs could be primarily attributed to two fundamental factors. First, the distinctive amphiphilic Janus configuration plays a crucial role. Second, the nanosheets form a closely packed arrangement on the oil droplet surfaces, establishing an effective spatial barrier that greatly impedes the coalescence of oil droplets. The free energy of particle desorption from the diesel–water interface to the aqueous or oil phase is defined as adsorption energy (ΔG). In order to evaluate the ΔG roughly, two assumptions were used: 1) the JNs are parallel to the oil–water interface, 2) a JNs monolayer is formed on the surface of the emulsion droplets. The corresponding ΔG values of the JNs desorbing into the diesel and aqueous phase can be calculated by eqn (1) and (2):14 | ΔG1 = Eoil − Einterface = A(γo-N − γw-N + γo–w) | (1) |
| ΔG2 = Ewater − Einterface = A(γw-C − γo-C + γo–w) | (2) |
where, A is the area of the JNs at the oil–water interface, γo–w, γo-N, γw-N, γw-C, and γo-C are the interfacial tension between oil and water, oil and the hydrophilic face of JNs, water and the hydrophilic face of JNs, water and the hydrophobic face of JNs, oil and the hydrophobic face of JNs, respectively. The interfacial tensions are related to the contact angle by Young's eqn (3):40 | γo-JNs − γw-JNs = γo–w cos θ | (3) |
where, θ represents the three-phase contact angle of the JNs at the oil–water interface. Fig. 4(a) shows the measurement method for the contact angle θ. The red dotted line represents the outer contour of the oil drop, while θ denotes the angle between the red dotted line and the JNs normal line. Since it is difficult to be directly measure the contact angle of JNs forming an emulsion, the adsorption energy ΔG1 of the JNs from the oil–water interface to the oil phase was calculated using the contact angle θC (137.1°) of the hydrophobic side, and the adsorption energy ΔG2 of the JNs from the oil–water interface to the water phase was calculated using the contact angle θN (77.8°) of the hydrophilic side. Therefore, eqn (1) and (2) can be recast in terms of θ to obtain eqn (4) and (5): | ΔG1 = Aγo–w[1 + cos θN] | (4) |
| ΔG2 = Aγo–w[1 − cos θC] | (5) |
where, θN and θC represent the three-phase contact angle on the hydrophilic and hydrophobic side of the JNs, respectively. Since the length of the prepared JNs was 3–5 μm and the thickness was about 3.23 nm, considering JNs as a square sheet with a side length (l) of 4 μm and negligible thickness, the area of the JNs (A) is equal to the area of the square, which is A = l2. Based on these assumptions and data, the ΔG1 of the JNs was calculated to be 5.26 × 10−13 J and the ΔG2 of the JNs was 7.51 × 10−13 J. Our research group has prepared spherical Janus silica particles (JSP) with a diameter of 350 nm, and measured their contact angle as 113.3° and the oil–water interfacial tension as 18.41 mN m−1.14 Based on some assumptions and calculations, the ΔG1 value of a JSP was calculated to be between 1.76 × 10−15 J and 1.86 × 10−14 J, while the ΔG2 value was between 9.4 × 10−15 J and 7.51 × 10−13 J, indicating that the ΔG value of a Janus nanosheet is at least one order of magnitude higher than that of a Janus sphere. These results show that the JNs have better emulsion stability because of their peculiar lamellar structure.
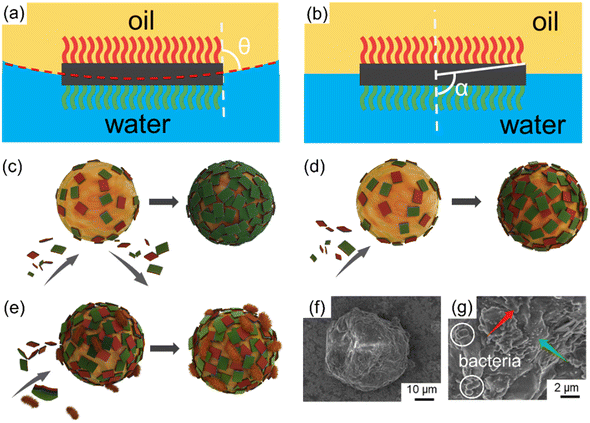 |
| Fig. 4 (a) Three-phase contact angle (θ) of the JNs at the oil–water interface during emulsion formation. (b) Angle α parametrization of the relative areas ratio of the hydrophilic and hydrophobic surfaces. (c) First hypothesis of the emulsification process, (d) second hypothesis of the emulsification process, (e) hypothesis of the synergistic interactions between JNs and AP-1 bacteria. (f) SEM of paraffin oil droplets, (g) SEM of multilayer JNs on the surface of paraffin oil droplets (green and red represent the hydrophilic and hydrophobic sides of JNs, respectively). | |
Referring to the hydrophilic–lipophilic balance (HLB) of classical surfactants, the Janus equilibrium (J) is defined as the ratio of the work required to transfer Janus particles from the oil–water interface to the oil phase or the water phase,41 and is calculated using eqn (6) as follows:
| 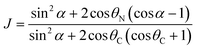 | (6) |
where,
θN and
θC are the contact angle of the hydrophilic side and the hydrophobic side of the JNs, respectively, and the relative area ratio of the hydrophilic and hydrophobic surfaces is represented by the angle
α (0–180°), referring to
Fig. 4(b). Since the thickness of the JNs was very small (length ≫ thickness),
α can be considered equal to 90°. It has been widely acknowledged that when
J = 1, the Pickering emulsion stabilized by Janus particle shows the highest stability.
41,42 According to
eqn (6), the
J value of the JNs prepared in this study was calculated to be 0.949, indicating a high stabilization of the JNs for oil droplets.
In fact, the JNs may form multilayers rather than monolayers on the surface of emulsion droplets. From the optical microscope, the oil droplets exhibited an irregular elliptic shape, which is consistent with the molecular simulation results.42 Based on the limited coalescence theory,43 Meng and coworkers derived the relationship relating the interfacial coverage, the average oil droplet size, the amount and size of nanosheets, and the volume of the oil phase, as shown in eqn (7):44
|  | (7) |
where
D is the average droplet size of the oil,
h is the thickness of the nanosheets,
ρp is the density of nanosheets,
Voil is the oil phase volume,
C is the interfacial coverage, and
mtotp is the total mass of nanosheets. According to our experimental results, the density of JNs was measured to be 0.196 g cm
−3 and the thickness of the JNs was about 3.16 nm. When 8 g L
−1 of JNs and a 1
![[thin space (1/6-em)]](https://www.rsc.org/images/entities/char_2009.gif)
:
![[thin space (1/6-em)]](https://www.rsc.org/images/entities/char_2009.gif)
4 diesel–water volume ratio were used (
mtotp of JNs was 0.08 g, diesel phase volume was 2 ml), the oil droplet size was measured to be 60.3 μm. According to
eqn (7),
C was calculated to be 649%. Too much JNs dispersed in the continuous phase would result in an interfacial coverage over 100%.
44 Therefore, the JNs may form multilayer steric hindrance on the droplet surface, thus leading to the irregular shape of the oil droplets because of the high interface rotation limitation of the JNs and multilayer steric hindrance caused by the JNs. In order to explore how the multilayer steric hindrance is formed by JNs in the process of emulsion formation, two conjectures are proposed.
45 One hypothesis is that in the early stage of emulsification, JNs randomly adhere to the surface of oil droplets under the control of diffusion. Nevertheless, under the influence of substantial energy supplied by the disperser, collisions among the JNs occur during their motion, resulting in the formation of ridges and trenches. This ultimately leads to the reorientation of the hydrophilic side of the JNs toward the aqueous phase. This specific process is shown in
Fig. 4(c). Another hypothesis is that when JNs are randomly attached to the surface of oil droplets in the early stage, the hydrophobic sides of JNs exposed to the water phase continue to be covered by additional JNs until the hydrophilic side is exposed to the water phase, as shown in
Fig. 4(d). From the SEM images of emulsified paraffin oil droplets shown in
Fig. 4(f and g), multilayer JNs are formed on the surface of oil droplets and most of the JNs expose their hydrophilic side to the water phase (rough side), but a small number of the JNs also expose their hydrophobic side on the droplet surface (smooth side). Thus, the emulsification process of oil by JN particles may occur in the second way proposed above. The synergistic interactions between the JNs and bacterial cells contribute greatly to oil emulsification. The adsorbed bacterial cells can also increase the steric hindrance, thus improving the stability of the emulsion further, as shown in
Fig. 4(e and g).
3.4 Toxicity of dispersants on bacteria
In actual offshore oil pollution treatment, biodegradation is the ultimate fate to remove petroleum hydrocarbon pollutants, so the toxicity of dispersants on oil-degrading bacteria is important to evaluate the practicability of dispersants.46,47 Studies have shown that conventional dispersants tend to inhibit bacterial growth.48,49 The toxicity of the JNs on AP-1 was evaluated here. The results in Fig. 5(a) show that the bacterial colonies grew exponentially in the logarithmic phase and plateaued after 36 h. At this time, the value of OD600 reached 1.72, which means the bacterial concentration was about 4.15 × 108 CFU mL−1 according to the curve of the OD value versus the bacterial concentration (Fig. S5†). Fig. 5(b) shows that under different concentrations of JNs, the number of bacterial colonies was basically maintained at 4.15 × 108 CFU mL−1. It was thus proved that the presence of JNs had no effect on the growth and reproduction of bacteria. The growth of bacteria in an agar plate is shown in Fig. S6.†Fig. 5(c) shows that the metabolic activity of AP-1 was only slightly reduced at high concentrations of JNs. To sum up, it could be concluded that JNs, compared with traditional chemical dispersants, showed small negative effects on bacterial growth and metabolic activity.
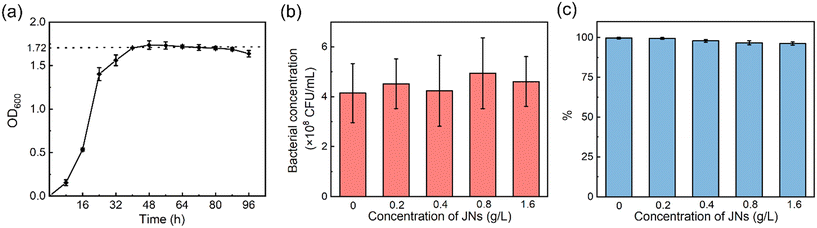 |
| Fig. 5 (a) Bacterial growth curve. (b) Toxicity test results in the presence of JNs. (c) Resazurin test results in the presence of JNs. | |
3.5 Biodegradation of oil
Dispersants can stimulate oil biodegradation in marine environment because of the increased interfacial area between oil and water.50 AP-1 are a strain of oil-degrading bacteria screened from Loushan River sediment, and are suitable for growth at 30 °C. It is important to note that in the following biodegradation experiments, the concentrations of the targeted compounds/compound groups were normalized against hopane for determination of biotransformation.51
A preliminary test was conducted to measure the biodegradation ratio of oil, as can be seen from Fig. 6(a). Then, the amount of biodegraded diesel was calculated, by using the initial amount of diesel multiplied by the biodegradation ratio, and the values are shown in Table 1. With the increase in diesel concentration, the amount of diesel biodegradation increased first and then decreased. When the diesel concentration was 6 mL L−1, the amount of diesel biodegradation reached the maximum. So, in the subsequent experiments, this diesel concentration was used.
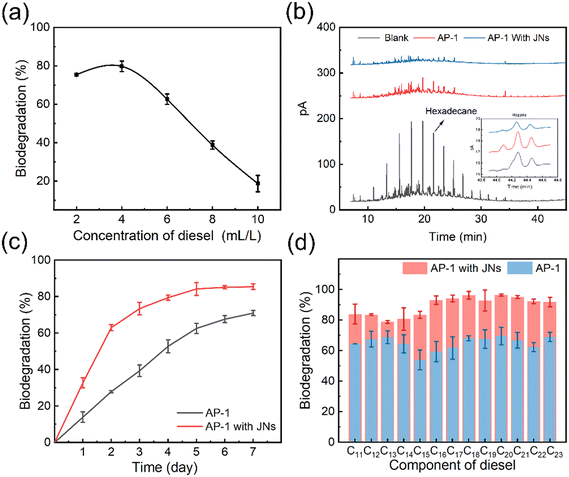 |
| Fig. 6 (a) Biodegradation effect at various diesel concentrations (time for 5 days), (b) biodegradation effects of AP-1 bacteria on diesel oil alone and synergistically with JNs (the concentration of JNs was 0.3 g L−1 and diesel was 6 mL L−1, time for 5 days), inset: enlargement of 43.8–44.8 min, (c) biodegradation effect over time (the concentration of JNs was 0.3 g L−1 and diesel was 6 mL L−1), (d) biodegradation effects of different diesel components (the concentration of JNs was 0.3 g L−1 and diesel was 6 mL L−1, time for 5 days). In the above experiments, the inoculation quantity of the bacterial suspension was 0.2 mL L−1. | |
Table 1 Biodegradation of diesel
Initial concentration of diesel (mL L−1) |
2 |
4 |
6 |
8 |
10 |
Biodegradation (%) |
75.4 |
79.7 |
62.6 |
38.7 |
18.7 |
Amount of biodegraded diesel (mL) |
0.15 |
0.32 |
0.38 |
0.31 |
0.19 |
Fig. 6(b) shows the biodegradation effects of AP-1 bacteria on diesel oil alone and when acting synergistically with JNs. The black line corresponds to the diesel gas chromatogram at 6 ml L−1, displaying a total peak area of 3619.806 pA s. In contrast, the red line represents the gas chromatogram after 5 days of bacterial degradation for 6 ml L−1 diesel oil, resulting in a total peak area of 922.016 pA s. Similarly, the blue line signifies the gas chromatogram after 5 days of bacterial degradation of 6 ml L−1 diesel oil in the presence of JNs, showcasing a total peak area of 370.064 pA s. Indeed, the blue line's reduced peak area compared to the red line signified the enhanced biodegradability of diesel oil in the presence of JNs. This improvement could be attributed to the smaller oil droplet size facilitated by the JNs dispersant, which in turn increased the contact area between AP-1 bacteria and diesel oil. As a result, the biodegradation effect was positively impacted.
The influence of JNs on the biodegradation of diesel was analyzed. As can be seen from Fig. 6(c), the JNs could improve the biodegradation of diesel remarkably. After 5 days, the biodegradation of bacteria for diesel reached about 85% and it became stable in the presence of the JNs. The kinetic fitting of the bacterial degradation for diesel was carried out and it was found that the biodegradation of diesel without JNs was more consistent with the first-order degradation kinetics, and the fitting equation was ln
C = 0.1907t + 4.1126, R2 = 0.9936, half-life t = 3.63 days. After adding JNs, the biodegradation of diesel was more consistent with the second-order degradation kinetics, and the fitting equation was 1/C = 0.01566 t + 0.01684, R2 = 0.9928, and half-life t = 1.08 days (The detailed data are given in the ESI† Table S2 and Fig. S7).52 It was speculated that the reason for this change was that in the early stage of diesel biodegradation, the JNs make the size of the oil droplets smaller, which greatly increases the contact area between the bacteria and diesel. In the late stage of diesel biodegradation addition, more bacteria bind to the surface of JNs, due to the influence of gravity, and the diesel–JNs–bacteria system settles into seawater, which reduces the diesel concentration and makes the contact area larger, thus improving the efficiency of diesel biodegradation.53
Finally, the biodegradation of different components in diesel was compared by bacteria using a gas chromatography technique. It can be found from Fig. 6(d) that the presence of JNs improved the biodegradation of all components in diesel, among which C16 was the most easily biodegraded. It was interesting to note that the biodegradation of long-chain n-alkanes (C16–C23) was improved more in the presence of JNs. This was because JNs not only enhanced the stability of short-chain n-alkanes in the seawater, but also reduced the volatilization of these alkanes, which is called ‘n-alkane fixation’ function.29 In conclusion, JNs play an important role in the bacterial degradation of oil, and the synergistic system of JNs and bacteria can greatly increase the biodegradation of oil, which provides a theoretical basis for the treatment of offshore oil spill accidents.
4. Conclusion
In conclusion, Janus nanosheets were successfully synthesized through the template-assisted sol–gel method, and then these nanosheets were combined with negatively charged oil-degrading bacteria, forming a synergistic approach for efficient oil treatment in seawater. As an amphiphilic solid material, JNs can reduce the interfacial tension between the oil and water interface and form steric hindrance on the surface of oil droplets. Furthermore, the restricted rotation of the flake structure contributes to an enhanced emulsification and emulsion stability in oil–water mixtures. Mechanistic analysis revealed that the adsorption energy of the JNs at the oil–water interface surpassed that of spherical Janus particles by at least an order of magnitude, resulting in the formation of a more stable emulsion. Moreover, the fact that the JNs exhibited a negligible impact on the reproduction and activity of the oil-degrading bacterium AP-1 suggests the potential of this synergistic approach involving JNs and bacteria as a promising and environmentally friendly solution for comprehensive oil pollutant remediation. In comparison to conventional dispersants, environmentally friendly JNs can not only effectively disperse oil spills but also enhance the oil biodegradation efficiency. These findings provide a new perspective for the practical application of JNs, indicating their potential in the synergistic treatment of offshore oil spills with oil-degrading bacteria. Further work is underway to modify JNs to enhance their synergistic oil degradation with bacteria.
Author contributions
Haosen Zhao: conceptualization, data curation, formal analysis, investigation, methodology, writing – original draft. Xin Hu: methodology, investigation. Hu Kang: methodology, writing – review & editing. Dongdong Yang: software, visualization. Mutai Bao: conceptualization, resources, validation. Yiming Li: resources, funding acquisition, data curation, project administration, validation, supervision.
Conflicts of interest
The authors declare that they have no known competing financial interests or personal relationships that could have appeared to influence the work reported in this paper.
Acknowledgements
This research is supported by National Natural Science Foundation of China (22172149) and Taishan Scholars Foundation of Shandong Province (tsqn202211053).
References
- J. Chen, W. Zhang, Z. Wan, S. Li, T. Huang and Y. Fei, Oil spills from global tankers: Status review and future governance, J. Cleaner Prod., 2019, 227, 20–32 CrossRef.
- E. Cakir, C. Sevgili and R. Fiskin, An analysis of severity of oil spill caused by vessel accidents, Transp. Res. D: Transp. Environ., 2021, 90, 102662 CrossRef.
- Z. Zhu, X. Song, Y. Cao, B. Chen, K. Lee and B. Zhang, Recent advancement in the development of new dispersants as oil spill treating agents, Curr. Opin. Chem. Eng., 2022, 36, 100770 CrossRef.
- S. W. Jung, O. Y. Kwon, C. K. Joo, J. H. Kang, M. Kim, W. J. Shim and Y. O. Kim, Stronger impact of dispersant plus crude oil on natural plankton assemblages in short-term marine mesocosms, J. Hazard. Mater., 2012, 217–218, 338–349 CrossRef CAS PubMed.
- G. Claireaux, M. Theron, M. Prineau, M. Dussauze, F. X. Merlin and S. Le Floch, Effects of oil exposure and dispersant use upon environmental adaptation performance and fitness in the European sea bass, Dicentrarchus labrax, Aquat. Toxicol., 2013, 130–131, 160–170 CrossRef CAS PubMed.
- A. Saha, A. Nikova, P. Venkataraman, V. T. John and A. Bose, Oil emulsification using surface-tunable carbon black particles, ACS Appl. Mater. Interfaces, 2013, 5, 3094–3100 CrossRef CAS PubMed.
- R. Aveyard, B. P. Binks and J. H. Clint, Emulsions stabilised solely by colloidal particles, Adv. Colloid Interface Sci., 2003, 100–102, 503–546 CrossRef CAS.
- R. F. A. Teixeira, H. S. McKenzie, A. A. Boyd and S. A. F. Bon, Pickering Emulsion Polymerization Using Laponite Clay as Stabilizer To Prepare Armored “Soft” Polymer Latexes, Macromolecules, 2011, 44, 7415–7422 CrossRef CAS.
- M. Omarova, L. T. Swientoniewski, I. K. M. Tsengam, A. Panchal, T. Yu, D. A. Blake, Y. M. Lvov, D. Zhang and V. John, Engineered Clays as Sustainable Oil Dispersants in the Presence of Model Hydrocarbon Degrading Bacteria: The Role of Bacterial Sequestration and Biofilm Formation, ACS Sustainable Chem. Eng., 2018, 6, 14143–14153 CrossRef CAS.
- C. Casagrande, P. Fabre, E. Raphaël and M. Veyssié, “Janus Beads”: Realization and Behaviour at Water/Oil Interfaces, Europhys. Lett., 1989, 9, 251–255 CrossRef CAS.
- J. Zhang, B. A. Grzybowski and S. Granick, Janus Particle Synthesis, Assembly, and Application, Langmuir, 2017, 33, 6964–6977 CrossRef CAS PubMed.
- Y. Duan, X. Zhao, M. Sun and H. Hao, Research Advances in the Synthesis, Application, Assembly, and Calculation of Janus Materials, Ind. Eng. Chem. Res., 2021, 60, 1071–1095 CrossRef CAS.
- A. Kirillova, C. Marschelke and A. Synytska, Hybrid Janus Particles: Challenges and Opportunities for the Design of Active Functional Interfaces and Surfaces, ACS Appl. Mater. Interfaces, 2019, 11, 9643–9671 CrossRef CAS PubMed.
- Y. Hou, Y. Li, L. Wang, D. Chen, M. Bao and Z. Wang, Amphiphilic Janus particles for efficient dispersion of oil contaminants in seawater, J. Colloid Interface Sci., 2019, 556, 54–64 CrossRef CAS PubMed.
- A. C. de Leon, B. J. Rodier, Q. Luo, C. M. Hemmingsen, P. Wei, K. Abbasi, R. Advincula and E. B. Pentzer, Distinct Chemical and Physical Properties of Janus Nanosheets, ACS Nano, 2017, 11, 7485–7493 CrossRef CAS PubMed.
- T. Yin, Z. Yang, M. Lin, J. Zhang and Z. Dong, Preparation of Janus nanosheets via reusable cross-linked polymer microspheres template, Chem. Eng. J., 2019, 371, 507–515 CrossRef CAS.
- F. Liang, J. Liu, C. Zhang, X. Qu, J. Li and Z. Yang, Janus hollow spheres by emulsion interfacial self-assembled sol-gel process, Chem. Commun., 2011, 47, 1231–1233 RSC.
- Y. Chen, F. Liang, H. Yang, C. Zhang, Q. Wang, X. Qu, J. Li, Y. Cai, D. Qiu and Z. Yang, Janus Nanosheets of Polymer–Inorganic Layered Composites, Macromolecules, 2012, 45, 1460–1467 CrossRef CAS.
- R. Suzuki, M. Sudo, M. Hirano, N. Idota, M. Kunitake, T. Nishimi and Y. Sugahara, Inorganic Janus nanosheets bearing two types of covalently bound organophosphonate groups via regioselective surface modification of K4Nb6O17·3H2O, Chem. Commun., 2018, 54, 5756–5759 RSC.
- T. Yin, Z. Yang, Z. Dong, M. Lin and J. Zhang, Physicochemical properties and potential applications of silica-based amphiphilic Janus nanosheets for enhanced oil recovery, Fuel, 2019, 237, 344–351 CrossRef CAS.
- B. Shaghaghi, S. Khoee and S. Bonakdar, Preparation of multifunctional Janus nanoparticles on the basis of SPIONs as targeted drug delivery system, Int. J. Pharm., 2019, 559, 1–12 CrossRef CAS PubMed.
- D. Xue, Q. B. Meng and X. M. Song, Magnetic-Responsive Janus Nanosheets with Catalytic Properties, ACS Appl. Mater. Interfaces, 2019, 11, 10967–10974 CrossRef CAS PubMed.
- J. Liu, P. Wang, M. Zhou, Y. Ma, X. Niu, G. Pan and J. Pan, Tailored Janus silica nanosheets integrating bispecific artificial receptors for simultaneous adsorption of 2,6-dichlorophenol and Pb(ii), J. Mater. Chem. A, 2019, 7, 16161–16175 RSC.
- X. Ji, Q. Zhang, F. Liang, Q. Chen, X. Qu, C. Zhang, Q. Wang, J. Li, X. Song and Z. Yang, Ionic liquid functionalized Janus nanosheets, Chem. Commun., 2014, 50, 5706–5709 RSC.
- Z. Zhao, F. Liang, G. Zhang, X. Ji, Q. Wang, X. Qu, X. Song and Z. Yang, Dually Responsive Janus Composite Nanosheets, Macromolecules, 2015, 48, 3598–3603 CrossRef CAS.
- H. Qi, T. Zhou, S. Mei, X. Chen and C. Y. Li, Responsive Shape Change of Sub-5 nm Thin, Janus Polymer Nanoplates, ACS Macro Lett., 2016, 5, 651–655 CrossRef CAS PubMed.
- S. Fujii, Y. Yokoyama, Y. Miyanari, T. Shiono, M. Ito, S. Yusa and Y. Nakamura, Micrometer-sized gold-silica Janus particles as particulate emulsifiers, Langmuir, 2013, 29, 5457–5465 CrossRef CAS PubMed.
- H. Cheng, Z. Li, Y. Li, Z. Shi, M. Bao, C. Han and Z. Wang, Multi-functional magnetic bacteria as efficient and economical Pickering emulsifiers for encapsulation and removal of oil from water, J. Colloid Interface Sci., 2020, 560, 349–358 CrossRef CAS PubMed.
- Q. Chen, M. Bao, X. Fan, S. Liang and P. Sun, Rhamnolipids enhance marine oil spill bioremediation in laboratory system, Mar. Pollut. Bull., 2013, 71, 269–275 CrossRef CAS PubMed.
- S. Archakunakorn, N. Charoenrat, S. Khamsakhon, T. Pongtharangkul, P. Wongkongkatep, M. Suphantharika and J. Wongkongkatep, Emulsification efficiency of adsorbed chitosan for bacterial cells accumulation at the oil-water interface, Bioprocess Biosyst. Eng., 2015, 38, 701–709 CrossRef CAS PubMed.
- X. Chen, Y. Hou, H. Cheng, M. Bao and Y. Li, Rapid capturing of oil-degrading bacteria by engineered attapulgite and their synergistic remediation for oil spill, J. Colloid Interface Sci., 2021, 604, 272–280 CrossRef CAS PubMed.
- Z. Zhu, F. Merlin, M. Yang, K. Lee, B. Chen, B. Liu, Y. Cao, X. Song, X. Ye, Q. K. Li, C. W. Greer, M. C. Boufadel, L. Isaacman and B. Zhang, Recent advances in chemical and biological degradation of spilled oil: A review of dispersants application in the marine environment, J. Hazard. Mater., 2022, 436, 129260 CrossRef CAS PubMed.
- F. Liang, K. Shen, X. Qu, C. Zhang, Q. Wang, J. Li, J. Liu and Z. Yang, Inorganic Janus nanosheets, Angew. Chem., Int. Ed., 2011, 50, 2379–2382 CrossRef CAS PubMed.
- T. P. Chen, Y. Liu, C. Q. Sun, M. S. Tse, J. H. Hsieh, Y. Q. Fu, Y. C. Liu and S. Fung, Core-Level Shift of Si Nanocrystals Embedded in a SiO2 Matrix, J. Phys. Chem. B, 2004, 108, 16609–16612 CrossRef CAS.
- Y. Paska and H. Haick, Controlling Surface Energetics of Silicon by Intermolecular Interactions between Parallel Self-Assembled Molecular Dipoles, J. Phys. Chem. C, 2009, 113, 1993–1997 CrossRef CAS.
- L. Chen, J. Xu, Q. Hasi, Y. Zhang, X. Jiang, L. Zhang, K. Xu, L. Li and A. Li, Oleophilic pitch derived porous carbon loading with microbials for selective absorption and efficient degradation of petroleum pollutions, Sep. Purif. Technol., 2022, 286, 120453 CrossRef CAS.
- Y. Zheng, C. Xiong, X. Li and L. Zhang, Covalent attachment of cell-adhesive peptide Gly-Arg-Gly-Asp (GRGD) to poly(etheretherketone) surface by tailored silanization layers technique, Appl. Surf. Sci., 2014, 320, 93–101 CrossRef CAS.
- H. Amani and H. Kariminezhad, Study on emulsification of crude oil in water using emulsan biosurfactant for pipeline transportation, Pet. Sci. Technol., 2016, 34, 216–222 CrossRef CAS.
- W. Hoffmann, Potential and restrictions of photocentrifugation for determining the emulsion stability of melted spreadable processed cheese, Dairy Sci. Technol., 2015, 96, 251–259 CrossRef.
- M. A. Creighton, Y. Ohata, J. Miyawaki, A. Bose and R. H. Hurt, Two-dimensional materials as emulsion stabilizers: interfacial thermodynamics and molecular barrier properties, Langmuir, 2014, 30, 3687–3696 CrossRef CAS PubMed.
- S. Jiang and S. Granick, Janus balance of amphiphilic colloidal particles, J. Chem. Phys., 2007, 127, 161102 CrossRef PubMed.
- W. Xiang, S. Zhao, X. Song, S. Fang, F. Wang, C. Zhong and Z. Luo, Amphiphilic nanosheet self-assembly at the water/oil interface: computer simulations, Phys. Chem. Chem. Phys., 2017, 19, 7576–7586 RSC.
- S. Arditty, C. P. Whitby, B. P. Binks, V. Schmitt and F. Leal-Calderon, Some general features of limited coalescence in solid-stabilized emulsions, Eur. Phys. J. E: Soft Matter Biol. Phys., 2003, 11, 273–281 CrossRef CAS PubMed.
- Q. B. Meng, P. Yang, T. Feng, X. Ji, Q. Zhang, D. Liu, S. Wu, F. Liang, Z. Zheng and X. M. Song, Phosphomolybdic acid-responsive Pickering emulsions stabilized by ionic liquid functionalized Janus nanosheets, J. Colloid Interface Sci., 2017, 507, 74–82 CrossRef CAS PubMed.
- T. M. Ruhland, A. H. Groschel, N. Ballard, T. S. Skelhon, A. Walther, A. H. Muller and S. A. Bon, Influence of Janus particle shape on their interfacial behavior at liquid-liquid interfaces, Langmuir, 2013, 29, 1388–1394 CrossRef CAS PubMed.
- T. C. Hazen, R. C. Prince and N. Mahmoudi, Marine Oil Biodegradation, Environ. Sci. Technol., 2016, 50, 2121–2129 CrossRef CAS PubMed.
- I. M. Head, D. M. Jones and W. F. Roling, Marine microorganisms make a meal of oil, Nat. Rev. Microbiol., 2006, 4, 173–182 CrossRef CAS PubMed.
- S. Kleindienst, M. Seidel, K. Ziervogel, S. Grim, K. Loftis, S. Harrison, S. Y. Malkin, M. J. Perkins, J. Field, M. L. Sogin, T. Dittmar, U. Passow, P. M. Medeiros and S. B. Joye, Chemical dispersants can suppress the activity of natural oil-degrading microorganisms, Proc. Natl. Acad. Sci. U. S. A., 2015, 112, 14900–14905 CrossRef CAS PubMed.
- S. Mishra and S. N. Singh, Microbial degradation of n-hexadecane in mineral salt medium as mediated by degradative enzymes, Bioresour. Technol., 2012, 111, 148–154 CrossRef CAS PubMed.
- J. Tremblay, E. Yergeau, N. Fortin, S. Cobanli, M. Elias, T. L. King, K. Lee and C. W. Greer, Chemical dispersants enhance the activity of oil- and gas condensate-degrading marine bacteria, ISME J., 2017, 11, 2793–2808 CrossRef CAS PubMed.
- S. Lofthus, I. K. Almas, P. Evans, O. Pelz and O. G. Brakstad, Biodegradation in seawater of PAH and alkylphenols from produced water of a North Sea platform, Chemosphere, 2018, 206, 465–473 CrossRef CAS PubMed.
- A. Dadrasnia and P. Agamuthu, Dynamics of diesel fuel degradation in contaminated soil using organic wastes, Int. J. Environ. Sci. Technol., 2013, 10, 769–778 CrossRef CAS.
- B. Pequin, Q. Cai, K. Lee and C. W. Greer, Natural attenuation of oil in marine environments: A review, Mar. Pollut. Bull., 2022, 176, 113464 CrossRef CAS PubMed.
|
This journal is © The Royal Society of Chemistry 2024 |