Source-specific ecological and health risks of polycyclic aromatic hydrocarbons in the adjacent coastal area of the Yellow River Estuary, China†
Received
26th September 2023
, Accepted 9th November 2023
First published on 27th November 2023
Abstract
Industrialization and urbanization have led to increasing levels of PAH pollution in highly urbanized estuaries and their adjacent coastal areas globally. This study focused on the adjacent coastal area of the Yellow River Estuary (YRE) and collected surface seawater, surface sediment, and clams Ruditapes philippinarum and Mactra veneriformis at four sites (S1 to S4) in May, August, and October 2021 to analyze the source-specific ecological and health risks and bioeffects. The findings revealed that the main sources of PAHs were traffic emission (25.2% to 28.5%), petroleum sources (23.3% to 29.5%), coal combustion (24.7% to 27.5%), and biomass combustion (19.8% to 20.7%). Further, the PMF-RQ and PMF-ILCR analyses indicated that traffic emission was the primary contributor to ecological risks in seawater and health risks in both clam species, while coal combustion was the major contributor in sediment. Taken together, it is recommended to implement control strategies for PAH pollution following the priority order: traffic > coal > petroleum > biomass, to reduce the content and risk of PAHs in the YRE.
Environmental significance
The Yellow River is one of the world's most sediment-laden rivers and has witnessed an increase in PAH pollution in the Yellow River Estuary (YRE) in recent years. This study investigated the pollution risk and the specific source of PAHs in the adjacent coastal area of the YRE, and the results revealed that traffic emissions were the highest contributor to ecological risks in seawater and to health risks in two clam species, while coal combustion was the major contributor in sediment. Additionally, the petroleum source was likely to have a significant impact on the risk of bioeffects.
|
1. Introduction
The estuarine ecosystem is situated at the interface of freshwater and seawater, and it is subject to influences from both the river and the ocean, while 80% of major cities worldwide are located in estuary areas.1 Due to the proximity of estuaries to urban environments, a significant amount of persistent organic pollutants, such as polycyclic aromatic hydrocarbons (PAHs), are emitted into the estuarine ecosystem and ultimately accumulate in the adjacent coastal areas.2,3 PAHs have garnered global attention due to their carcinogenic, teratogenic, and mutagenic effects.4,5 Previous studies have reported high levels of PAH pollution in water bodies in major estuaries in South America,6 sediments in major estuaries worldwide,7 and aquatic products from major estuaries in North and South America.8 Therefore, it is imperative to establish an analytical technique from the source of pollution to the final sink for effective control of PAH pollution in estuary areas globally.
Environmental risk assessment involves evaluating the ecological damage and health risks resulting from environmental pollution, which mainly includes ecological risk assessment and health risk assessment.9,10 However, environmental risk assessment cannot fully reflect the bioeffects of pollutants and thus cannot meet the needs of risk assessment of marine pollution.11 Biomarkers, as a type of biological effects indicator with an ‘early warning’ function for toxic substances, have been commonly used in marine environmental assessment.12 However, problems often occur due to the limitations in sensitivity and accuracy, as well as the lack of biomarker screening research, resulting in biomarkers not matching the pollution.13 Integrated biomarker indexes based on the biomarkers can merge the response of multiple biomarkers to pollution status. In recent years, it has been widely applied in marine environmental assessment and has become an important technology in the field of risk assessment.14,15 Nevertheless, there are no reports on the comprehensive study of ecological and health risks, as well as bioeffects of PAH pollution in estuary areas.
Source apportionment has become an effective approach for pollution governance and is regarded as a crucial link in the process of formulating pollution control strategies.16 Among various methods of pollution source analysis, receptor modeling has been extensively utilized for source apportionment of PAHs in estuarine areas worldwide, including atmosphere,17 water,15 sediment,18 and marine organisms.19 However, the current source apportionment which is based solely on receptor models can only provide preliminary estimates of pollution sources and lacks integration with risk assessment, making it difficult to provide source profiles that cause major ecological and health risks. In recent years, scholars have developed a series of source-specific risk assessment approaches based on receptor models and risk assessment methods to integrate source apportionment and risk assessment, which can quantitatively evaluate the sources of ecological and health risks and provide scientific support for pollution control.20 For example, the Nemerow integrated risk index (NIRI) was employed in conjunction with the positive matrix factorization (PMF) method to identify key pollution sources and assess the ecological risks posed by heavy metals in the road dust of Beijing, which revealed that traffic emission, pesticides, and fertilizers were the primary contributors to significant ecological risks.21 In another study, the toxicity equivalent factor (TEQ) and PMF-based PMF-TEQ approach were utilized to evaluate the source-specific health risks of PAHs in the seawater of the Persian Gulf, and the results indicated that the emissions from gasoline/diesel vehicles were the primary source of sediment PAHs and had the highest contribution to the carcinogenic risk of PAHs.22 Consequently, source-specific risk assessment methods have been widely employed in various environmental media. Nonetheless, research on source-specific risks of PAHs in biological media and comprehensive evaluations of pollution source-specific risks and bioeffects are yet to be reported.
The Yellow River is one of the world's most sediment-laden rivers and has witnessed an increase in PAH pollution in the Yellow River Estuary (YRE) in recent years.23 Moreover, Shandong Province, the location of the YRE, is recognized as one of the regions with the highest emissions of PAH pollution in China.24 This study focused on the adjacent coastal area of the YRE and collected surface seawater, surface sediment, and clams (Ruditapes philippinarum and Mactra veneriformis) at four sites in May, August, and October 2021. The research encompassed biomonitoring, risk assessment, source apportionment, and source-specific risk of PAH pollution to evaluate the contributions of different PAH sources to ecological and health risks. Hence, the objective of this research is to provide robust technical support for skills transferable to other areas of environmental science, i.e. the source-specific risk assessment and its combination with bioeffect assessment, to further shed light on the pollution control based on the source of ecological, biological, and health risks.
2. Materials and methods
2.1. Study area and sampling in the adjacent coastal area of the YRE
The Shengli Oilfield, China's second-largest oilfield, is located in the YRE25 (Fig. 1). Shandong Province contributed the most thermal power generation in 2021 (ref. 26) and there are currently seven large groups of coal power plants settled near the YRE (Fig. 1). Hence, the adjacent coastal area of the YRE was selected as the study area based on relevant studies that mainly focused on the coastal area of Dongying City, Shandong,27,28 including four sites, i.e., S1 – Dongying Port (38°5′18′′ N, 118°57′40′′ E), S2 – Yellow River Estuary (37°49′54′′ N, 119°16′35′′ E), S3 – Huanghekou Town (37°39′48′′ N, 119°0′59′′ E), and S4 – Guangli Port (37°23′14′′ N, 118°55′56′′ E) (Fig. 1). In May, August, and October 2021, seawater and sediment were sampled in triplicate from the low tidal, mid-tidal, and high tidal regions of the intertidal zone of each site. The Chinese National Standard Method GB 17378.3-2007 was followed for sample collection, transportation, and storage.29 Surface seawater (0–0.5 m depth) was collected and stored in brown glass bottles at 4 °C, while the surface sediment (0–5 cm depth) was collected and stored in glass flasks at −20 °C.
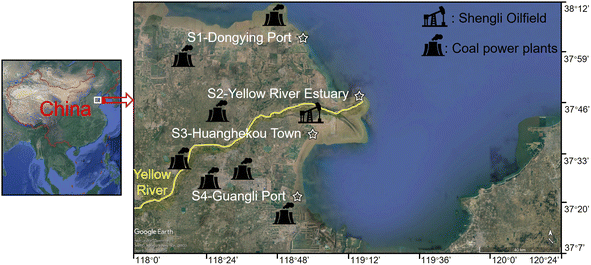 |
| Fig. 1 Sampling sites (S1–S4) in the adjacent coastal area of the Yellow River Estuary. | |
90 specimens of R. philippinarum and 90 specimens of M. veneriformis (both male and female) were collected and transported alive from the low, mid, and high tidal regions of each site to the laboratory under cold conditions (4 °C) within a span of 3 hours. The weight and size of the clams did not differ significantly among sites. For R. philippinarum, the average weight was 9.57 ± 2.12 g and the shell length was 37.72 ± 2.85 mm. For M. veneriformis, the average weight was 12.01 ± 3.18 g and the shell length was 35.17 ± 2.57 mm. The clams from different tidal regions were divided into 3 replicates (sub-sample) for the determination of PAH contents and biomarkers, with each replicate consisting of 30 clams. In each replicate, 5 clams were randomly selected to measure various aspects of their body condition, such as wet body weight, shell weight, shell length, shell height, weight of soft gonad, weight of the digestive gland, and weight of soft tissue. These clams were then put back to the 30 clams and among them, 10 clams were dissected to obtain their entire soft tissue for the determination of PAH contents. The gills and digestive glands of the remaining 20 clams were dissected and pooled together as one sample.
Afterwards, these samples were then instantly frozen at −80 °C and treated as one sample for the subsequent analysis of biomarkers (ethoxyresorufin-O-deethylase (EROD), glutathione S-transferase (GST), total antioxidant capacity (T-AOC), superoxide dismutase (SOD), catalase (CAT), glutathione peroxidase (GPx), glutathione (GSH), lipid peroxidation (LPO), DNA damage, protein carbonylation (PC) content, and acetylcholinesterase (AChE)) and relevant gene expression (heat shock proteins (HSP22-2, 40A, 60, 70, and 90), P-glycoprotein (P-gp), aryl hydrocarbon receptor (AhR), cytochrome P450 family 1 subfamily A member 1 (CYP1A1), glutathione S-transferase (GST-pi and mu), ATP binding cassette subfamily C member 1 (ABCC1), Mn superoxide dismutase (MnSOD), CAT, and GPx). Additionally, the hemolymph of the remaining 20 clams was collected from the anterior adductor muscle using sterilized syringes. After centrifugation (700 g, 10 min, 4 °C), both the supernatant (plasma) and the pellet (hemocytes) were collected and labeled with numbers. The hemocytes were immediately used to measure the total hemocyte count (THC), phagocytic activity, and lysosomal membrane stability (LMS). The plasma, on the other hand, was frozen using liquid nitrogen and later used to analyze antibacterial activity and bacteriolytic activity after storage at −80 °C.
2.2. PAH concentration and environmental characterization analysis
We analyzed the concentrations of 16 EPA priority PAH compounds in seawater, sediment, and clam soft tissue using a Shimadzu gas chromatography-mass spectrometer (GCMS-QP2020 NX, Shimadzu, Japan) equipped with an SH-Rxi-5Sil MS capillary column (30 m length × 0.25 mm i.d. × 0.25 μm film thickness), following the Chinese National Standard Methods GB/T 26411-2010,30 HJ 805-2016,31 and GB 5009.265-2016,32 respectively. We provided detailed information on the extraction, analysis, quality assurance, and quality control procedures in the ESI.† Environmental characterization of the seawater, including water temperature (WT, °C), salinity (S, ‰), and pH, was in situ performed by using a thermometer, salinometer, and pH meter, respectively. Additionally, we analyzed the contents of NH4+, NO2−, NO3−, total nitrogen (TN), and total phosphorus (TP) in seawater using a spectrophotometer (Multiskan GO 1500, Thermofisher, USA) according to the Chinese National Standard Method GB/T 17378.4-2007.33 We also determined the contents of total organic carbon (TOC) in sediments following the Chinese National Standard Method GB 17378.5-2007.34 The particle-size distribution of the sediment, including clay, silt, and sand, was analyzed using a laser diffraction particle-size analyzer (Mastersizer 2000, Malvern Instruments, USA).
2.3. Biomarker analysis
We measured shell length, shell height, and the weight of the gonad, digestive gland, soft tissue, and shell immediately during sampling to calculate the indices of five conditions that reflect the physiological condition of mussels (condition index (CI), condition index based on the shell length (CIL), shell thickness (ST), gonad index (GI), and hepato-somatic index (HSI)). After dissection, we collected gills and digestive glands of clams to measure relevant biomarkers, such as EROD, GST, T-AOC, SOD, CAT, GPx, GSH, LPO, DNA damage, PC content, and AChE, using a spectrophotometer (Multiskan GO 1500, Thermofisher, USA), spectrofluorometer (Molecular Spectroscopy LS 55, P.E., MA, USA), and UV spectrophotometer (Lambda 25, P.E., MA, USA). We also separated the hemocytes and plasma of clams to measure the biomarkers, including THC, phagocytic activity, LMS, antibacterial activity, and bacteriolytic activity, using an inverted phase contrast microscope (Olympus, Japan) and a spectrophotometer (Multiskan GO 1500, Thermofisher, USA). Moreover, we only isolated total RNA from the gills and digestive glands of R. philippinarum, as there is currently no gene sequence for M. veneriformis in the NCBI database. We analyzed mRNA expression levels of relevant biomarkers, such as HSP22-2, HSP40A, HSP60, HSP70, HSP90, P-gp, AhR, CYP1A1, GST-pi and GST-mu, ABCC1, MnSOD, CAT, GPx, and two reference housekeeping genes (EF1A and RPS23) using the comparative Ct method (2−ΔΔCt method),35 for further details see the ESI (pages S6–S7).†
2.4. Risk assessment
We assessed the ecological risk and health risk of PAHs in the seawater, sediment, and organisms using the risk quotient (RQ) and incremental lifetime cancer risk (ILCR) approaches based on previous studies.9,36,37 Additionally, we utilized previously published methods of integrated biomarker response (IBR) and multi-biomarker pollution index (MPI) to evaluate the responses of multiple biomarkers (ST, EROD, GST, SOD, CAT, GPx, LPO, AChE, and HSP22-2) in two species of clams, R. philippinarum and M. veneriformis.38,39 Further details of the assessment are provided in the ESI (pages S7–S9).†
2.5. Source apportionment
In this study, diagnostic ratios were used to analyze the source profile according to a recent study,40 including the proportion of anthracene to anthracene adding phenanthrene (Ant/(Ant+Phe)), fluoranthene to fluoranthene adding pyrene (Fla/(Fla+Pyr)), benzo[a]anthracene to benzo[a]anthracene adding chrysene (BaA/(BaA+Chr)), and indeno[1,2,3-cd]pyrene to indeno[1,2,3-cd]pyrene adding benzo[g,h,i]perylene (Ind/(Ind+BghiP)). Additionally, the positive matrix factorization (PMF) model was adopted to identify the sources of PAHs in seawater and sediment based on our previously published research41 (see details in ESI page S9†).
2.6. Source-specific risk assessment
In this study, the PMF-RQ method was utilized to quantitatively assess the ecological risks posed by various sources of PAHs, represented by eqn (1) and (2): | RQiPMF = RQi × SFij (0 ≤ i ≤ 16) | (1) |
| 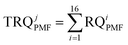 | (2) |
In eqn (1), the values of RQi denote the contribution rate of the RQ for the ith PAH to the total RQ value in this study, as presented in Table S1.† SFij represents the contribution rate of the ith PAH to the jth factor (corresponding to the jth source) of PMF, derived from the PMF model and displayed in Table S2.† RQiPMF indicates the calculated ecological risk value of individual PAHs in relation to the contribution of the jth source. Total RQiPMF (TRQjPMF) signifies the sum of RQiPMF for the 16 PAHs, representing the contribution rate of the jth source to RQ. The values of RQiPMF and TRQjPMF in this study are provided in Tables S3 and S4.†
Similarly, the PMF-ILCR was employed to quantitatively evaluate the health risks associated with different sources of PAHs, represented by eqn (3) and (4):
| ILCRiPMF = ILCRi × SFij (0 ≤ i ≤ 16) | (3) |
| 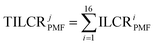 | (4) |
In eqn (3), the values of ILCRi represent the contribution rate of the ILCR for the ith PAH to the total ILCR value, as shown in Table S5.† SFij denotes the contribution rate of the ith PAH to the jth factor (corresponding to the jth source) of PMF and is displayed in Table S6.† ILCRiPMF indicates the calculated health risk value of individual PAHs from the contribution of the jth source. Total ILCRiPMF (TILCRjPMF represents the sum of ILCRiPMF for the 16 PAHs, which signifies the contribution rate of the jth source to ILCR. The values of ILCRiPMF and TILCRjPMF in this study can be found in Tables S7 and S8.†
2.7. Data analysis
Before statistical analysis, the Kolmogorov–Smirnov test was used to ensure the normal distribution and heterogeneity of variances. For the data exhibited non-normal distribution, a logarithmic transformation of the data was performed to provide a statistically normalized distribution. Differences among groups were analyzed using one-way ANOVA coupled with multiple comparisons of Tukey's test statistics, with a significance level of P < 0.05 and P < 0.01. Separate Tukey's tests and the Bonferroni correction were then conducted to adjust the observed significance level for each group. The data analysis was performed using SPSS 27.0, EPA PMF 5.0, and R Studio. The results were visualized using Google Earth, Origin 2021, Adobe Illustrator 2021, and R Studio.
3. Results and discussion
3.1. Distribution patterns of PAHs in the adjacent coastal area of the YRE
Fig. 2 shows that the concentration of total PAHs in the seawater adjacent to the YRE ranged from 32.77 to 84.38 ng L−1, which was lower than that of other coastal areas in China like the Bohai Bay,42 Pearl River Estuary,43 and Hainan Island.44 The concentrations were slightly higher than or comparable to those observed globally such as in the Arctic,45 Rea Sea,46 and Oman Sea47 (Table S9†). The concentration of total PAHs in the sediment adjacent to the YRE ranged from 38.76 to 218.17 ng g−1 dry weight (d.w.), which was lower than in different estuaries such as the Yangtze River Estuary, China,48 the Mahanadi River Estuary, India,49 and the Estuary of St. Lawrence, Canada.50 It was comparable to or slightly higher than those in the YRE detected before by other researchers48 and other estuaries including estuaries in Santa Monica Bay of southern California,51 the Goiana Estuary of Brazil,6 and the Paranagua Estuarine System of South Atlantic,52 respectively (Table S9†). The concentrations of total PAHs in the clam soft tissue of R. philippinarum and M. veneriformis in this study ranged from 118.35 to 321.92 ng g−1 d.w. and 146.01 to 317.42 ng g−1 d.w., respectively. The concentrations were much lower than in other shellfishes from France, China, and Indonesia, such as mussel Mytilus edulis,53 pearl oyster Pinctada martensii,54 and green mussel Perna viridis55 (Table S9†). However, these concentrations were slightly higher than or comparable to those in other shellfishes from Chile, and the North and South Atlantic, such as mussels Perumytilus purpuratus,56Mytilus galloprovincialis,57 and Perna perna58 (Table S9†). Noteworthily, the seasonal variation of total PAH concentration was obvious that samples collected in August showed significantly higher content than those in May and October (P < 0.05), except for the seawater collected at S1 and S2, which was the most polluted in October. A similar temporal trend was also revealed in seawater, sediment, and clam soft tissue of our former studies11,15 and in the water column of Pearl River Estuary.43 Specifically, the reason why sediment samples collected in August had a significantly much higher content of PAHs than other two months was possibly attributed to the geographic location. The adjacent coastal area of the YRE is highly influenced by the riverine input of the Yellow River, one of the world's most sediment-laden rivers,23 which may renew the surface sediment periodically to form obviously different contents of pollutants.
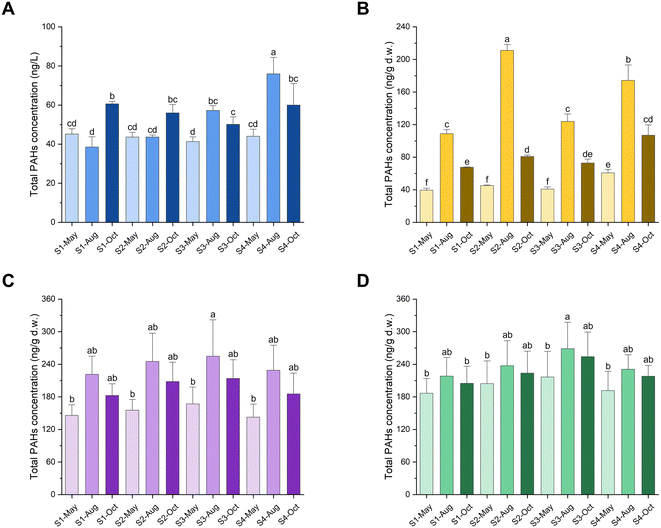 |
| Fig. 2 Total PAH concentration in seawater (A), sediment (B), and soft tissue of Ruditapes philippinarum (C) and Mactra veneriformis (D) at 4 sampling sites in 3 seasons. Different letters (a–f) represent that differences are significant at P < 0.05. | |
The characterization of seawater and sediment are presented in Table 1. Among these characteristics, TN, NO2−, and TP in seawater as well as TOC, clay, and sand in sediment varied significantly among sites (P < 0.05), which may influence the distribution of PAHs in seawater and sediment. Hence, the Spearman correlation analysis was performed to reveal their association. The increasing contents of NH4+, NO3−, and TP showed a significant correlation with the increasing total concentration of PAHs in seawater (P < 0.05) as indicated by Table 2, and it could be explained that the optimal growth rates of indigenous microorganisms that contribute to the biodegradation of PAHs may be limited by nitrogen (NH4+, NO3−) and phosphorus (TP).59 In sediment, only the relevance between TOC and PAHs was (extremely) significant (P < 0.01), which might be related to the absorbability of sediments to PAHs affected by the content of TOC.60 Meanwhile, the relationship between TOC and PAHs in sediment may be more complicated and likely dependent on the depth at which samples were collected.51 Overall, the PAH concentrations in seawater, sediment, and clams R. philippinarum and M. veneriformis were comparable to or slightly lower/higher than those in other coastal areas globally, and environmental characterization significantly affected PAH pollution.
Table 1 Environmental characterization in seawater and sediment at the 4 sites of the Yellow River Estuary in 2021
|
S1 |
S2 |
S3 |
S4 |
Seawater |
WT (°C) |
17.96 ± 7.02 |
18.87 ± 5.88 |
17.04 ± 5.60 |
16.76 ± 5.19 |
S (‰) |
29.78 ± 0.22 |
29.91 ± 0.27 |
29.95 ± 0.34 |
29.94 ± 0.31 |
pH |
7.78 ± 0.07 |
7.82 ± 0.07 |
7.81 ± 0.04 |
7.78 ± 0.04 |
TN (mg L−1) |
0.64 ± 0.13 |
0.90 ± 0.20 |
1.03 ± 0.31 |
0.65 ± 0.19 |
NH4+ (mg L−1) |
0.31 ± 0.05 |
0.30 ± 0.04 |
0.32 ± 0.06 |
0.23 ± 0.05 |
NO2− (mg L−1) |
0.036 ± 0.007 |
0.048 ± 0.018 |
0.073 ± 0.014 |
0.098 ± 0.017 |
NO3− (mg L−1) |
0.24 ± 0.05 |
0.25 ± 0.04 |
0.29 ± 0.08 |
0.24 ± 0.04 |
TP (mg L−1) |
0.030 ± 0.005 |
0.045 ± 0.024 |
0.029 ± 0.015 |
0.068 ± 0.016 |
Sediment |
TOC (%) |
0.29 ± 0.03 |
0.40 ± 0.06 |
0.49 ± 0.15 |
0.29 ± 0.12 |
Clay (%) |
15.55 ± 2.48 |
16.04 ± 5.88 |
23.97 ± 9.55 |
7.40 ± 1.09 |
Silt (%) |
53.87 ± 7.00 |
61.72 ± 7.18 |
54.98 ± 9.09 |
55.20 ± 15.98 |
Sand (%) |
30.58 ± 6.28 |
22.24 ± 8.98 |
21.05 ± 18.07 |
37.40 ± 16.28 |
Table 2 Spearman correlation analysis between total PAH concentrations and environmental characterization in seawater and sedimenta
|
Total PAH concentrations in seawater |
Total PAH concentrations in sediment |
R
|
Sig. |
R
|
Sig. |
Notes: asterisk denotes significant differences between the total PAH concentrations and the environmental characterization using Spearman correlation analysis (*Sig. < 0.05, **Sig. < 0.01).
|
Seawater |
WT (°C) |
−0.009 |
0.957 |
— |
— |
S (‰) |
−0.113 |
0.512 |
— |
— |
pH |
−0.141 |
0.414 |
— |
— |
TN (mg L−1) |
0.302 |
0.074 |
— |
— |
NH4+ (mg L−1) |
0.489 |
0.003** |
— |
— |
NO2− (mg L−1) |
0.248 |
0.145 |
— |
— |
NO3− (mg L−1) |
0.343 |
0.041* |
— |
— |
TP (mg L−1) |
0.379 |
0.023* |
— |
— |
Sediment |
TOC (%) |
— |
— |
0.454 |
0.005** |
Clay (%) |
— |
— |
0.098 |
0.571 |
Silt (%) |
— |
— |
−0.157 |
0.36 |
Sand (%) |
— |
— |
−0.06 |
0.726 |
3.2. Risk assessment of PAHs in seawater, sediment, and organisms
PAHs found in the marine environment can potentially endanger aquatic organisms by posing ecological risks, health risks, and toxic bioeffects.13,61,62 Here, an assessment of PAH ecological risks in seawater and sediment was conducted using the RQ method (Fig. 3A and B). The results of the RQ method revealed moderate risk 2 for seawater at most sites months, as indicated by RQΣPAHs(NCs) < 800 and RQΣPAHs(MPCs) > 1 (Table S10†). Additionally, low-risk levels were observed for sediment at all sites months, as indicated by RQΣPAHs(NCs) < 800 and RQΣPAHs(MPCs) < 1 (Table S10†). These results were consistent with a previous study of PAH pollution in the coastal area of Shandong Province.41 The consumption of seafood is a crucial pathway for human exposure to PAHs,63 which can cause diseases like reproductive disorders and cancer.64 The proportion of seafood in our diet was expected to increase, with 12–25% of the projected global meat demand of 9.8 billion people by 2050 being filled by seafood.65 Therefore, the health risks posed by PAHs through the consumption of seafood, specifically R. philippinarum and M. veneriformis, popular seafood in North China, were assessed using the ILCR approach (Fig. 3C and D). The ILCR results indicated an acceptably low risk (10−6 < ILCR < 10−4) for all sites-months for both clams, which was consistent with other studies of health risk performed on these species.13,66 According to a previous study, future research should focus on prioritizing the connection between environmental biomonitoring and human health.67 Moreover, the potential of biomonitoring in assessing the exposure of the human body to organic pollutants and the subsequent bioeffects was emphasized.68 However, there is currently a lack in the interdisciplinary field of bioeffect and health risk assessment. Thus, it is crucial to investigate the health risks of PAHs in humans and develop more efficient biomonitoring techniques.
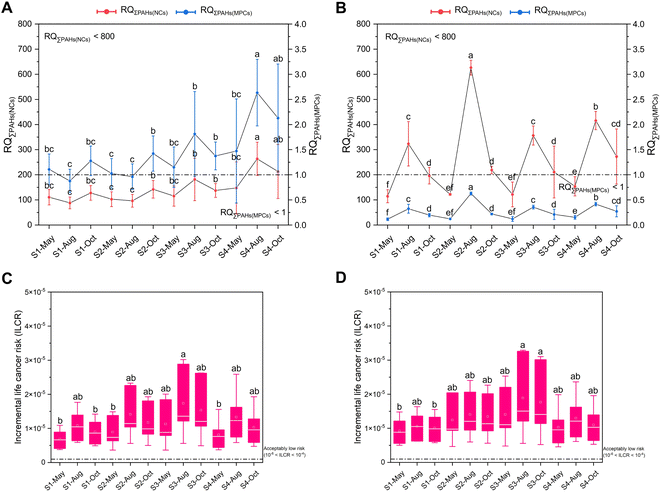 |
| Fig. 3 Results of ecological risk assessment for seawater (A) and sediment (B), as well as health risk assessment for clams Ruditapes philippinarum (C) and Mactra veneriformis (D). Different letters (a, b, c, d, e, and f) represent that differences are significant at P < 0.05. | |
Hence, in this study, a total of 35 biomarkers in R. philippinarum and 21 biomarkers in M. veneriformis were determined to clarify the bioeffect of PAHs in marine organisms (Fig. S1–S7†). The Spearman correlation analysis was performed between these biomarkers and total PAH concentrations in sediment and clam soft tissue according to our previously published study,13 to identify the sensitive biomarkers. Thereafter, 8 and 5 sensitive biomarkers were identified for R. philippinarum and M. veneriformis, respectively (Fig. 4A and B), including biometric indices (ST), detoxification-related enzyme activities (EROD, GST), antioxidant-related enzyme activities (SOD, CAT, GPx), oxidative damage-related indice (LPO), neurotoxicity-related enzyme activity (AChE), and mRNA expression of heat shock protein (HSP22-2). These biomarkers strongly correlated with the PAH concentration both in sediment and clam soft tissue (Tables S11 and S12†). Thereinto, some biomarkers were also found to be sensitive in our previous study13 and a recent study of antioxidant systems in three species of oysters exposed to Chr (a kind of 16 PAHs),69 including SOD, CAT, and GPx, suggesting their valuable appliance in field monitoring. Noteworthily, for those biomarkers determined both in the gills and digestive gland, only those with greater correlation in the single organ were selected, which is the digestive gland for R. philippinarum and the gills for M. veneriformis, and these biomarkers along with those detected for the biometric indices and in hemocyte and plasma were therefore used as indicators for evaluating the toxic bioeffect of PAH pollution for R. philippinarum and M. veneriformis, respectively. Our study emphasizes the importance of considering tissue specificity in obtaining accurate information on biomarker responses, similar to the findings of a recent study that observed variations in the residues of heavy metals among different tissues of bivalves, including M. veneriformis.70 This enhances the risk assessment of the PAH bioeffect in marine organisms. Moreover, in this study, we utilized IBR/n and MPI to conduct a comprehensive assessment of bioeffects, as depicted in Fig. 4C–F. The findings revealed that S3 and August exhibited high IBR/n and MPI values, while S1 and May had the lowest values for both clams. However, it is important to note that IBR and MPI, despite providing a mathematical correlation among bioeffect data, often fail to elucidate the biological significance, which can potentially lead to misinterpretation.71 Therefore, in environmental monitoring, it is recommended to integrate biomarker responses with chemical data to avoid such misinterpretation.62 Here, the relatively high concentrations of PAHs in both clams were found at S3 while they were lower at S1 (Fig. 2), as demonstrated by the trend of IBR and MPI. This validated the effectiveness of these two approaches, showing more severe biological stress for marine organisms at S3 than at S1. Meanwhile, the results of health risk (ILCR) and bioeffects (IBR and MPI) also exhibited a similar pattern (Fig. 3C and D), with the highest value observed at S3 and the lowest value at S1. This study provides preliminary evidence of a connection between environmental biomonitoring and human health, shedding light on the further ecotoxicological effects of PAHs on biota. However, further investigation is needed to validate these findings on a larger scale and across different research areas and species.
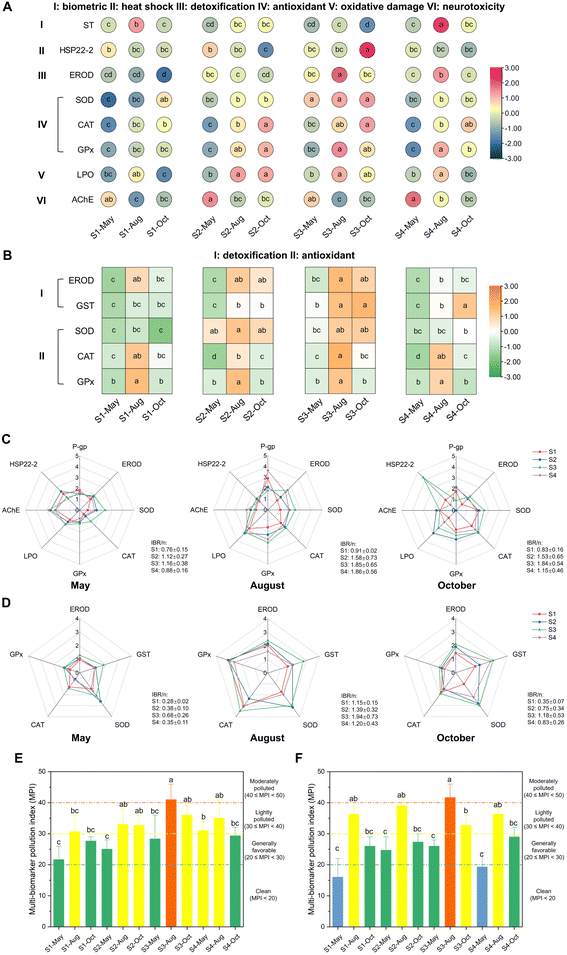 |
| Fig. 4 Normalized sensitive biomarker responses in clams Ruditapes philippinarum (A) and Mactra veneriformis (B) and integrated biomarker response (IBR/n) and multi-biomarker pollution index (MPI) of sensitive biomarkers in clam Ruditapes philippinarum (C and E) and Mactra veneriformis (D and F). ST: Shell thickness, EROD: ethoxyresorufin-O-deethylase, SOD: superoxide dismutase, CAT: catalase, GPx: glutathione peroxidase, LPO: lipid peroxidation, AChE: acetylcholinesterase, HSP22-2: heat shock protein 22–2, GST: glutathione S-transferase. Different letters (a and b) represent that differences are significant at P < 0.05. | |
3.3. Source apportionment of PAHs in seawater, sediment, and organisms
Source apportionment can provide scientific foundations for the development of strategies and policies for controlling pollution,16 and the receptor model is a commonly used method in source apportionment.72 Here, receptor models including diagnosis ratios and the PMF model were adopted in the PAH source analysis of seawater and sediment, and marine organisms. As shown in Fig. 5A–D, the results of diagnosis ratios showed that PAHs in both environmental media at most sites were petrogenic or derived from petroleum combustion, i.e., refined petroleum release and crude oil leakage source or the pyrolysis of petroleum fuel like those used in gasoline and diesel engines of vehicles, which were consistent with studies performed in water40,73 and marine sediment74,75 globally. However, the sources of PAHs in clams R. philippinarum and M. veneriformis were more diverse, which varied from petrogenic and petroleum combustion to biomass and coal combustion (Fig. 5E–H). It was similar to a study performed in Portugal that also analyzed the diagnosis ratio of PAHs in marine mollusks, and their results showed that bioaccumulated PAHs were derived from crude oil refining and combustion of grasses, coal, wood and petroleum.76 Therefore, the diagnosis ratios provide initial insight into the distinction between pollution sources in environmental media (seawater and sediment) and biological media (clams R. philippinarum and M. veneriformis).
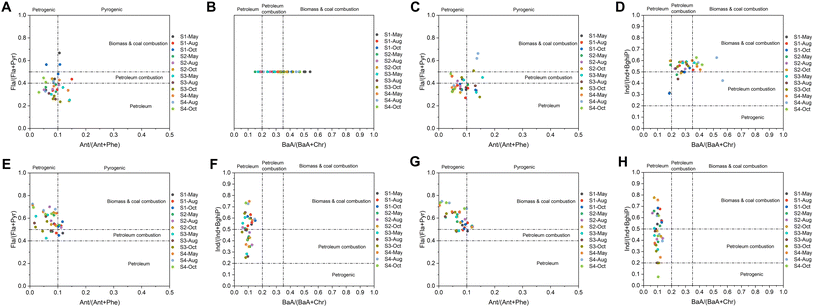 |
| Fig. 5 Results of source apportionment performed using diagnosis ratios for seawater (A and B), sediment (C and D), and clams Ruditapes philippinarum (E and F) and Mactra veneriformis (G and H). Phe: Phenanthrene, Ant: anthracene, Fla: fluoranthene, Pyr: pyrene, BaA: benzo[a]anthracene, Chr: chrysene, Ind: indeno[1,2,3-cd]pyrene, BghiP: benzo[g,h,i]perylene. | |
Further, the contribution of each PAH congener to each source was quantitatively analyzed by using the PMF model. The PMF model was run in EPA PMF 5.0 software with 3 to 6 factors initialized with different starting points.77 The four-factor solution exhibited the closest value between Q (robust) and Q (true) in seawater, sediment, and clams (Table S13†) and was therefore used to identify sources. As shown in Fig. 6, PAHs were mainly derived from traffic emission sources (traffic-red, 28.4%, 28.5%, 25.2%, and 26.2% for seawater, sediment, clams R. philippinarum and M. veneriformis, respectively), refined petroleum release or crude oil leakage source (petroleum-purple, 25.9%, 23.3%, 29.5%, and 28.3% for seawater, sediment, clams R. philippinarum and M. veneriformis, respectively), coal combustion source (coal-yellow, 25.9%, 27.5%, 24.7%, and 25.3% for seawater, sediment, clams R. philippinarum and M. veneriformis, respectively), and biomass combustion source (biomass-green, 19.8%, 20.7%, 20.6%, and 20.2% for seawater, sediment, clams R. philippinarum and M. veneriformis, respectively), respectively. Among the four factors, traffic emission source was identified due to the high load of HMW PAHs such as BkF, BaP, DahA, Ind and BghiP, which were regarded as good indicators of diesel or gasoline engine emissions.78,79 The coal combustion source was dominated by middle 4-ring PAHs including Fla, Pyr, and BaA, which were recorded as typical indicators of coal combustion and specifically, BaA is named as the coal combustion component.80 The petroleum source was largely loaded by LMW PAHs including Ace, Flu and Phe, especially Phe. This was similar to the factor profile in a relevant study, where Phe was also dominating and assigned to the evaporation of unburned petroleum-based fuels.81 The biomass combustion source was highly loaded by LMW PAHs like Acy and Ace, moderately contributed by the HMW PAHs like BbF and BkF, which could be attributed to combustion of straw and firewood, i.e., traditional biomass energy, that are widely used in most rural areas.82,83 In some results, Ant was dominating in a certain source, which is also considered as biomass combustion as ant is a marker of wood burning.84 Noteworthily, in environmental media (seawater and sediment), PAHs were mainly derived from traffic emissions and in organisms (R. philippinarum and M. veneriformis), they were highly petrogenic. It was consistent with a study of source quantification of PAHs in both marine sediment and organisms (including fish, shrimp, and crab) in Haizhou Bay, China that PAHs in sediment were mostly from transportation sources (48.12%) and in organisms, the oil source took twice the proportion (25.82%) compared to that in sediment (11.33%).19 This may be attributed to factors that influence the bioaccumulation of PAHs. Generally, PAHs with higher octanol–water partition coefficients (Kow) have a greater capacity for bioaccumulation, which aligns with their enrichment patterns in the natural environment.85,86 However, numerous studies have demonstrated that there are other significant factors affecting the process of PAH bioaccumulation, including environmental characterization, the number of PAH rings, and the biometric characteristics and detoxification metabolism of organisms.87–89 The bioaccumulation manifested a different PAH composition to the environment, and due to the specific uptake patterns, some sources may have higher importance to the biota.
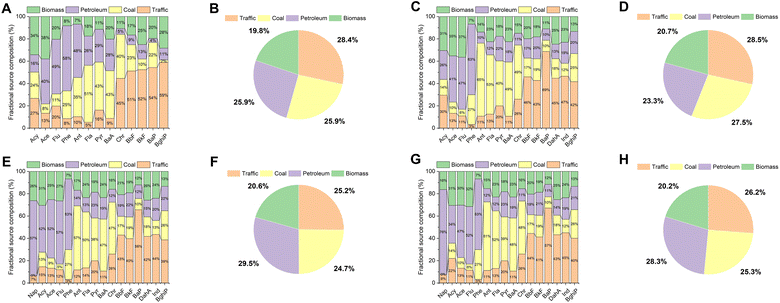 |
| Fig. 6 Results of source apportionment performed by positive matrix factorization for seawater (A and B), sediment (C and D), and clams Ruditapes philippinarum (E and F) and Mactra veneriformis (G and H). Nap: Naphthalene, Acy: acenaphthylene, Ace: acenaphthene, Flu: fluorene, Phe: phenanthrene, Ant: anthracene, Fla: fluoranthene, Pyr: pyrene, BaA: benzo[a]anthracene, Chr: chrysene, BbF: benzo[b]fluoranthene, BkF: benzo[k]fluoranthene, BaP: benzo[a]pyrene, Ind: indeno[1,2,3-cd]pyrene, DahA: dibenzo[a,h]anthracene, BghiP: benzo[g,h,i]perylene, traffic: traffic emission source, petroleum: refined petroleum release or crude oil leakage source, coal: coal combustion source, biomass: biomass combustion source. | |
3.4. Source-specific risk assessment and its implications for pollution control
Previous research has predominantly assessed pollution risks based on the concentration of pollutants, overlooking their sources that also contributed to the overall risk.90 However, recent studies have revealed that the contribution of each pollution source to the overall pollution risk is different from its contribution to the concentration of pollutants.21 Therefore, it is essential to gain a comprehensive assessment of the source-specific risk in order to develop control strategies for environmental pollution. Here, we employed the PMF-RQ and PMF-ILCR methods, which integrate sources with ecological and health risks, to identify the contribution of various sources to different risks in the adjacent coastal area of the YRE. As shown in Fig. 7A and B, the traffic emissions were found to be the highest contributor to RQ for PAHs in seawater, while in sediment, the combustion of coal was the highest contributor. This was similar to the results of PMF that PAHs were mainly derived from traffic and coal sources in seawater and sediment. It could be explained that the Shandong Province where the YRE is located is a very important industrial region in China, which had the second largest amount of road vehicles and contributed the most coal power generation in China.26,91 More specific data can be found in Tables S3 and S4.† For the PMF-ILCR results of clams R. philippinarum and M. veneriformis, traffic emissions were also the major source contributing to the health risk in both species (Fig. 7C and D). These results differ from the results of PMF, which showed that PAHs in clam soft tissue were mainly derived from refined petroleum release or crude oil leakage sources. These differences can be explained by the different values of TEFs used in the ILCR method.92 PAH congeners of traffic emission sources (BbF, BkF, BaP, DahA, Ind, and BghiP) had much higher TEFs (0.01 to 1) than those of other sources (0.001 to 0.01) (Table S14†), leading to an increase in the contribution of traffic emissions to the health risk. More specific data can be found in Tables S7 and S8.†
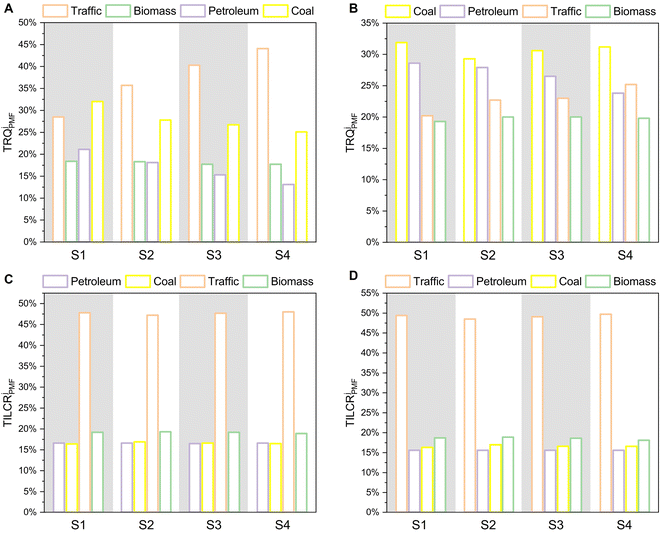 |
| Fig. 7 Results of source-specific risk assessment apportionment performed by PMF-RQ for seawater (A) and sediment (B) and PMF-ILCR for clams Ruditapes philippinarum (C) and Mactra veneriformis (D). Traffic: traffic emission source, petroleum: refined petroleum release or crude oil leakage source, coal: coal combustion source, biomass: biomass combustion source. | |
Taken together, our findings demonstrated that the source-specific risk varied due to differences in concentration and composite components among each source. This observation aligns with previous studies conducted in the urban river–lake system sediment and the seawater of the Persian Gulf.22,93 Notably, traffic emission emerged as the primary contributor to the overall risk in the adjacent coastal area of the YRE, and coal combustion was second to it. In order to mitigate pollution risks, it is crucial to undergo a large-scale transition from fossil fuels to clean energy rapidly in transportation and power generation.94 For instance, the popularization of electric vehicles presents an effective strategy towards achieving this goal, which should be put forward as a policy of pollution governance.15 Additionally, due to the limit of source-specific risk assessment, there is currently no way to find out the source-specific risk of bioeffects, i.e., biomarkers that correlated with the pollution level. However, the source apportionment of PAHs in the soft tissue of both clam species showed that the primary source of bioaccumulated PAHs was petrogenic (Fig. 5 and 6). Therefore, the petroleum source was likely to contribute significantly to the risk of bioeffects and should be taken into consideration for pollution control. Hence, the priority for PAH pollution control in the adjacent coastal area of the Yellow River Estuary, taking into account both source-specific risk assessment and bioeffects, should be as follows: traffic > coal > petroleum > biomass.
4. Conclusions
In this study, we aim to investigate the source-specific ecological and health risks and bioeffects of PAH pollution in the adjacent coastal area of the YRE, to further contribute to the pollution control based on the source of ecological, biological, and health risks. To achieve this, we analyzed the contents of PAHs in the seawater, sediment, and soft tissue of two clam species, R. philippinarum and M. veneriformis, near the YRE, which turned out to be comparable to those in other coastal areas globally, contributing to the understanding of coastal environments worldwide. Furthermore, we assessed the ecological and health risks associated with PAHs in seawater, sediment, and clam soft tissue using the RQ and ILCR methods as well as their bioeffects in clams, providing preliminary evidence of a connection between environmental biomonitoring and human health.
To analyze the source of PAHs and their risks in seawater, sediment, and marine organisms, we employed receptor models such as diagnosis ratios and the PMF model as well as PMF-RQ and PMF-ILCR. The results of diagnosis ratios and PMF revealed that PAHs in seawater and sediment were mainly derived from traffic emission, while PAHs in clams were mostly petrogenic. However, the results of PMF-RQ and PMF-ILCR indicated that traffic emission was the highest contributor to ecological risks in seawater, while coal combustion was the major contributor in sediment. Additionally, traffic emission was also the primary source contributing to health risks in both clam species. Meanwhile, petroleum sources may highly contribute to the risk of bioeffects. Based on these findings, it is recommended to implement control strategies for PAH pollution following the priority order: traffic > coal > petroleum > biomass, to reduce the content and risk of PAHs in the adjacent coastal area of the YRE.
Conflicts of interest
There are no conflicts of interest to declare.
Acknowledgements
We thank the staff at the Laboratory of Environmental Physiology of Aquatic Animal for assisting in sampling the seawater, sediment, and clams R. philippinarum and M. veneriformis. This work was financially supported by the Key Technology Research and Development Program of Shandong Province (no. 2018GHY115007) and the National Natural Science Foundation of China (General Program) (no. 32273106).
References
- H. W. Paerl, N. S. Hall, B. L. Peierls and K. L. Rossignol, Evolving Paradigms and Challenges in Estuarine and Coastal Eutrophication Dynamics in a Culturally and Climatically Stressed World, Estuaries Coasts, 2014, 37, 243–258 CrossRef.
- S. J. Yoon, S. Hong, B. O. Kwon, J. Ryu, C. H. Lee, J. Nam and J. S. Khim, Distributions of persistent organic contaminants in sediments and their potential impact on macrobenthic faunal community of the Geum River Estuary and Saemangeum Coast, Korea, Chemosphere, 2017, 173, 216–226 CrossRef CAS.
- P. A. Neves, P. G. Costa, L. C. Portz, M. R. Garcia and G. Fillmann, Levels and sources of hydrocarbons in the Patos Lagoon estuary and Cassino Beach mud bank (South Atlantic, Brazil): evidence of transference between environments, Environ. Monit. Assess., 2023, 195, 484 CrossRef CAS.
- B. González-Gaya, A. Martinez-Varela, M. Vila-Costa, P. Casal, E. Cerro-Galvez, N. Berrojalbiz, D. Lundin, M. Vidal, C. Mompean, A. Bode, B. Jimenez and J. Dachs, Biodegradation as an important sink of aromatic hydrocarbons in the oceans, Nat. Geosci., 2019, 12, 119–125 CrossRef.
- M. Kumar, N. S. Bolan, S. A. Hoang, A. D. Sawarkar, T. Jasemizad, B. W. Gao, S. Keerthanan, L. P. Padhye, L. Singh, S. Kumar, M. Vithanage, Y. Li, M. Zhang, M. B. Kirkham, A. Vinu and J. Rinklebe, Remediation of soils and sediments polluted with polycyclic aromatic hydrocarbons: To immobilize, mobilize, or degrade?, J. Hazard. Mater., 2021, 420, 126534 CrossRef CAS PubMed.
- M. Barletta, A. R. A. Lima and M. F. Costa, Distribution, sources and consequences of nutrients, persistent organic pollutants, metals and microplastics in South American estuaries, Sci. Total Environ., 2019, 651, 1199–1218 CrossRef CAS PubMed.
- J. Guo, J. Chen and J. Wang, Sedimentary records of polycyclic aromatic hydrocarbons in China: A comparison to the worldwide, Crit. Rev. Environ. Sci. Technol., 2017, 47, 1612–1667 CrossRef CAS.
- J. V. de Pinho, A. P. Lopes, P. D. Rodrigues, R. G. Ferrari, R. A. Hauser-Davis and C. A. Conte, Food safety concerns on polycyclic aromatic hydrocarbon contamination in fish products from estuarine bays throughout the American continent, Sci. Total Environ., 2023, 858, 159930 CrossRef CAS PubMed.
- Z. G. Cao, J. L. Liu, Y. Luan, Y. L. Li, M. Y. Ma, J. Xu and S. L. Han, Distribution and ecosystem risk assessment of polycyclic aromatic hydrocarbons in the Luan River, China, Ecotoxicology, 2010, 19, 827–837 CrossRef CAS PubMed.
- M. S. Rahman, M. D. H. Khan, Y. N. Jolly, J. Kabir, S. Akter and A. Salam, Assessing risk to human health for heavy metal contamination through street dust in the Southeast Asian Megacity: Dhaka, Bangladesh, Sci. Total Environ., 2019, 660, 1610–1622 CrossRef PubMed.
- Z. Y. Li, Y. H. Cao, H. W. Qin, Y. Q. Ma, L. Q. Pan and J. W. Sun, Integration of chemical and biological methods: A case study of polycyclic aromatic hydrocarbons pollution monitoring in Shandong Peninsula, China, J. Environ. Sci., 2022, 111, 24–37 CrossRef CAS.
- K. Broeg and K. K. Lehtonen, Indices for the assessment of environmental pollution of the Baltic Sea coasts: Integrated assessment of a multi-biomarker approach, Mar. Pollut. Bull., 2006, 53, 508–522 CrossRef CAS PubMed.
- J. W. Sun, L. Q. Pan, Y. H. Cao and Z. Y. Li, Biomonitoring of polycyclic aromatic hydrocarbons (PAHs) from Manila clam Ruditapes philippinarum in Laizhou, Rushan and Jiaozhou, bays of China, and investigation of its relationship with human carcinogenic risk, Mar. Pollut. Bull., 2020, 160, 111556 CrossRef CAS PubMed.
- L. Q. Pan, M. Y. Zhang, Q. Jin and R. W. Ji, Multi-biomarker approach in the scallop Chlamys farreri to assess PAHs pollution in Qingdao coastal areas of China, Environ. Sci.: Processes Impacts, 2017, 19, 1387–1403 RSC.
- Z. Y. Li, R. C. Qi, Y. F. Li, J. J. Miao, Y. B. Li, M. Y. Zhang, Z. H. He, N. Zhang and L. Q. Pan, The ban on the sale of new petrol and diesel cars: Can it help control prospective marine pollution of polycyclic aromatic hydrocarbons (PAHs) in Shandong Province, China?, J. Hazard. Mater., 2023, 460, 132451 CrossRef CAS.
- X. Li, Q. Zhang, Y. Zhang, L. Zhang, Y. X. Wang, Q. Q. Zhang, M. Li, Y. X. Zheng, G. N. Geng, T. J. Wallington, W. J. Han, W. Shen and K. B. He, Attribution of PM2.5 exposure in Beijing-Tianjin-Hebei region to emissions: implication to control strategies, Sci. Bull., 2017, 62, 957–964 CrossRef CAS.
- J. H. Lee, C. L. Gigliotti, J. H. Offenberg, S. J. Eisenreich and B. J. Turpin, Sources of polycyclic aromatic hydrocarbons to the Hudson River Airshed, Atmos. Environ., 2004, 38, 5971–5981 CrossRef CAS.
- M. Al Hello, D. R. Burris, M. Chitsaz and L. A. Rodenburg, Source apportionment of polycyclic aromatic hydrocarbons in New York/New Jersey Harbour sediment, Water Environ. J., 2023, 37, 527–537 CrossRef CAS.
- W. T. Shi, M. Xu, Q. Liu and S. M. Xie, Polycyclic aromatic hydrocarbons in seawater, surface sediment, and marine organisms of Haizhou Bay in Yellow Sea, China: Distribution, source apportionment, and health risk assessment, Mar. Pollut. Bull., 2022, 174, 113280 CrossRef CAS PubMed.
- Y. M. Sun, Y. Z. Tian, Q. Q. Xue, B. Jia, Y. Wei, D. L. Song, F. X. Huang and Y. C. Feng, Source-specific risks of synchronous heavy metals and PAHs in inhalable particles at different pollution levels: Variations and health risks during heavy pollution, Environ. Int., 2021, 146, 106162 CrossRef CAS PubMed.
- C. Men, R. M. Liu, L. B. Xu, Q. R. Wang, L. J. Guo, Y. X. Miao and Z. Y. Shen, Source-specific ecological risk analysis and critical source identification of heavy metals in road dust in Beijing, China, J. Hazard. Mater., 2020, 388, 121763 CrossRef CAS PubMed.
- A. R. Jafarabadi, E. Raudonytė-Svirbutavičienė, A. R. Bakhtiari and A. Kareiva, Polycyclic aromatic hydrocarbons (PAHs) in the non-bleached and bleached corals and their ambient environment: The role of suspended particulate matter, mucus, and positive matrix factorization model for identifying contributions to the carcinogenicity of PAH sources, Sci. Total Environ., 2021, 787, 147688 CrossRef.
- C. Y. Wang, L. J. Yu, Y. M. Zou, L. Ji and Y. W. Li, Sources and historical sedimentary record: Temporal variability of n-alkane and PAHs from the Yellow River Estuary, China, Appl. Geochem., 2020, 114, 104475 CrossRef CAS.
- S. Y. Zhao, C. Li, Z. Wang, S. X. Yu and Z. H. Shi, Industrial polycyclic aromatic hydrocarbons (PAHs) emissions embodied in domestic trade in China in 2012, J. Environ. Manage., 2021, 284, 111994 CrossRef CAS PubMed.
- S. Yang, W. Yang, G. J. Chen, X. Fang, C. F. Lv, J. A. Zhong and L. H. Xue, Greenhouse gas emissions from oilfield-produced water in Shengli Oilfield, Eastern China, J. Environ. Sci., 2016, 46, 101–108 CrossRef CAS PubMed.
-
China Electricity Council (CEC), CEC Released the Annual Report on Development of China's Power Industry 2022, 2022, from. https://english.cec.org.cn/#/newsdetails?id=1550040674299142145 Search PubMed.
- X. B. Liu, D. L. Li and G. S. Song, Assessment of heavy metal levels in surface sediments of estuaries and adjacent coastal areas in China, Front. Earth. Sci., 2017, 11, 85–94 CrossRef CAS.
- M. Wang, C. Y. Wang and Y. W. Li, Petroleum hydrocarbons in a water-sediment system from Yellow River estuary and adjacent coastal area, China: Distribution pattern, risk assessment and sources, Mar. Pollut. Bull., 2017, 122, 139–148 CrossRef CAS PubMed.
-
The People’s Republic National Standards GB 17378.3, The Specification for Marine Monitoring - Part 3: Sample Collection, Storage and Transportation, General Administration of Quality Supervision, Inspection and Quarantine of the People’s Republic of China, Beijing, PRC, 2007 Search PubMed.
-
The People’s Republic National Standards GB 26411, Determination of 16 Polynuclear Aromatic Hydrocarbons in Seawater by GC-MS, General Administration of Quality Supervision, Inspection and Quarantine of the People’s Republic of China, Beijing, PRC, 2010 Search PubMed.
-
The People’s Republic National Standards HJ 805, Soil and Sediment - Determination of Polycyclic Aromatic Hydrocarbons by Gas Chromatography-Mass Spectrometry Method, State Environmental Protection Bureau, Beijing, PRC, 2016 Search PubMed.
-
The People’s Republic National Standards GB 5009.265, National Food Safety Standard - Determination of Polycyclic Aromatic Hydrocarbons in Food, China Food and Drug Administration, Beijing, PRC, 2016 Search PubMed.
-
The People’s Republic National Standards GB 17378.4, The Specification for Marine Monitoring - Part 4: Seawater Analysis, General Administration of Quality Supervision, Inspection and Quarantine of the People’s Republic of China, Beijing, PRC, 2007 Search PubMed.
-
The People’s Republic National Standards GB 17378.5, The Specification for Marine Monitoring - Part 5: Sediment Analysis, General Administration of Quality Supervision, Inspection and Quarantine of the People’s Republic of China, Beijing, PRC, 2007 Search PubMed.
- T. D. Schmittgen and K. J. Livak, Analyzing real-time PCR data by the comparative CT method, Nat. Protoc., 2008, 3, 1101–1108 CrossRef CAS PubMed.
- D. F. Kalf, T. Crommentuijn and E. J. van de Plassche, Environmental quality objectives for 10 polycyclic aromatic hydrocarbons (PAHs), Ecotoxicol. Environ. Saf., 1997, 36, 89–97 CrossRef CAS PubMed.
- US Environmental Protection Agency (US-EPA), Guidelines for Exposure Assessment. EPA/600/Z-92/001, Fed. Regist., 1992, 57, 22888–22938 Search PubMed.
- B. Beliaeff and T. Burgeot, Integrated biomarker response: a useful tool for ecological risk assessment, Environ. Toxicol. Chem., 2002, 21, 1316–1322 CrossRef CAS PubMed.
- J. F. Narbonne, M. Daubèze, C. Clérandeau and P. Garrigues, Scale of classification based on biochemical markers in mussels: application to pollution monitoring in European coasts, Biomarkers, 1999, 4, 415–424 Search PubMed.
- T. S. B. Abd Manan, T. Khan, W. H. M. W. Mohtar, S. Beddu, S. Qazi, Z. S. Khozani, N. L. M. Kamal, A. Sarwono, H. Jusoh, S. Yavari, S. F. Z. Mustafa, Z. Hanafiah, H. F. M. Zaid, A. Machmudah, M. H. Isa, A. Ahmad and A. A. J. Ghanim, Ecological and health risk assessment of polycyclic aromatic hydrocarbons (PAHs) in Sungai Perak, Malaysia, J. Cleaner Prod., 2021, 294, 126124 CrossRef CAS.
- R. C. Qi, L. Q. Pan, T. Liu and Z. Y. Li, Source risk, ecological risk, and bioeffect assessment for polycyclic aromatic hydrocarbons (PAHs) in Laizhou Bay and Jiaozhou Bay of Shandong Peninsula, China, Environ. Sci. Pollut. Res., 2022, 29, 56705–56726 CrossRef CAS PubMed.
- Y. F. Tong, L. Chen, Y. N. Wang and S. Y. Tian, Distribution, sources and ecological risk assessment of PAHs in surface seawater from coastal Bohai Bay, China, Mar. Pollut. Bull., 2019, 142, 520–524 CrossRef CAS PubMed.
- L. X. Niu, H. Y. Cai, P. H. A. J. M. van Gelder, P. Z. Luo, F. Liu and Q. S. Yang, Dynamics of polycyclic aromatic hydrocarbons (PAHs) in water column of Pearl River estuary (China): Seasonal pattern, environmental fate and source implication, Appl. Geochem., 2018, 90, 39–49 CrossRef CAS.
- N. Xiang, C. X. Jiang, T. H. Yang, P. Li, H. H. Wang, Y. L. Xie, S. N. Li, H. L. Zhou and X. P. Diao, Occurrence and distribution of Polycyclic aromatic hydrocarbons (PAHs) in seawater, sediments and corals from Hainan Island, China, Ecotoxicol. Environ. Saf., 2018, 152, 8–15 CrossRef CAS PubMed.
- R. J. Li, H. Gao, Z. Q. Ji, S. C. Jin, L. K. Ge, H. M. Zong, L. P. Jiao, Z. F. Zhang and G. S. Na, Distribution and sources of polycyclic aromatic hydrocarbons in the water column of Kongsfjorden, Arctic, J. Environ. Sci., 2020, 97, 186–193 CrossRef CAS PubMed.
- R. Elmorsi, Distribution of oil, grease and polycyclic aromatic hydrocarbons in coastal water and sediments of Suez Bay, Oceanol. Hydrobiol. Stud., 2021, 50, 373–384 CrossRef CAS.
- H. Agah, A. Mehdinia, K. D. Bastami and S. Rahmanpour, Polycyclic aromatic hydrocarbon pollution in the surface water and sediments of Chabahar Bay, Oman Sea, Mar. Pollut. Bull., 2017, 115, 515–524 CrossRef CAS PubMed.
- Y. M. Hui, M. H. Zheng, Z. T. Liu and L. R. Gao, Distribution of polycyclic aromatic hydrocarbons in sediments from Yellow River Estuary and Yangtze River Estuary, China, J. Environ. Sci., 2009, 21, 1625–1631 CrossRef CAS PubMed.
- B. Ambade, S. S. Sethi, S. Kurwadkar, P. Mishra and L. Tripathee, Accumulation of polycyclic aromatic hydrocarbons (PAHs) in surface sediment residues of Mahanadi River Estuary: Abundance, source, and risk assessment, Mar. Pollut. Bull., 2022, 183, 114073 CrossRef CAS PubMed.
- A. Corminboeuf, J. C. Montero-Serrano, R. St-Louis, A. Dalpe and Y. Gelinas, Pre- and post-industrial levels of polycyclic aromatic hydrocarbons in sediments from the Estuary and Gulf of St. Lawrence (eastern Canada), Mar. Pollut. Bull., 2022, 174, 113219 CrossRef CAS PubMed.
- O. Otim, Examining the correlation between quantifiable SVOCs and organic carbon content or particulate size in benthic sediments as a function of ocean stratum, Environ. Sci.: Processes Impacts, 2020, 22, 812–823 RSC.
- B. M. Gurgatz, M. R. Garcia, A. C. Cabral, A. C. de Souza, R. H. Nagai, R. C. L. Figueira, M. M. de Mahiques and C. C. Martins, Polycyclic aromatic hydrocarbons in a Natural Heritage Estuary influenced by anthropogenic activities in the South Atlantic: Integrating multiple source apportionment approaches, Mar. Pollut. Bull., 2023, 188, 114678 CrossRef CAS PubMed.
- M. H. Devier, S. Augagneur, H. Budzinski, K. Le Menach, P. Mora, J. F. Narbonne and P. Garrigues, One-year monitoring survey of organic compounds (PAHs, PCBs, TBT), heavy metals and biomarkers in blue mussels from the Arcachon Bay, France, J. Environ. Monit., 2005, 7, 224–240 RSC.
- H. H. Wang, W. Huang, Y. Gong, C. M. Chen, T. Y. Zhang and X. P. Diao, Occurrence and potential health risks assessment of polycyclic aromatic hydrocarbons (PAHs) in different tissues of bivalves from Hainan Island, China, Food Chem. Toxicol., 2020, 136, 111108 CrossRef CAS PubMed.
- M. Y. Aziz, A. Piram, L. Asia, A. Salim, N. V. Hidayati, B. Buchari, P. Doumenq and A. D. Syakti, Organic Pollutants Hazard in Sediments and Green Mussels in Jakarta Bay, Indonesia, Soil Sediment Contam., 2021, 30, 862–885 CrossRef CAS.
- H. Palma-Fleming, C. Cornejo, M. Gonzalez, V. Perez, M. Gonzalez, E. Gutierrez, J. L. Sericano and M. Seeger, Polycyclic aromatic hydrocarbons and polychlorinated biphenyls in coastal environments of Valdivia and Valparaiso, Chile, J. Chil. Chem. Soc., 2008, 53, 1533–1538 CAS.
- E. Blanco-Rayon, A. V. Ivanina, I. M. Sokolova, I. Marigomez and U. Izagirre, Sex and sex-related differences in gamete development progression impinge on biomarker responsiveness in sentinel mussels, Sci. Total Environ., 2020, 740, 140178 CrossRef CAS PubMed.
- C. A. A. Elmamy, B. M. L. Abdellahi, H. Er-Raioui, A. Dartige, M. L. Zamel and P. M. V. Deida, Hydrocarbon pollution in Atlantic coast of Mauritania (Levrier Bay Zone): Call for sustainable management, Mar. Pollut. Bull., 2021, 166, 112040 CrossRef CAS PubMed.
- R. M. Atlas, Microbial degradation of petroleum hydrocarbons: an environmental perspective, Microbiol. Rev., 1981, 45, 180–209 CrossRef CAS PubMed.
- H. Y. Wang, H. Y. Liu, H. T. Cui and N. Abrahamsen, Terminal Pleistocene/Holocene palaeoenvironmental changes revealed by mineral-magnetism measurements of lake sediments for Dali Nor area, southeastern Inner Mongolia Plateau, China, Palaeogeogr., Palaeoclimatol., Palaeoecol., 2001, 170, 115–132 CrossRef.
- Y. G. Gu, C. L. Ke, Y. P. Gao, Q. Liu and Y. F. Li, Nonmetric multidimensional scaling and adverse effects on aquatic biota of polycyclic aromatic hydrocarbons in sediments: A case study of a typical aquaculture wetland, China, Environ. Res., 2020, 182, 109119 CrossRef CAS PubMed.
- A. Cravo, C. Pereira, T. Gomes, C. Cardoso, A. Serafim, C. Almeida, T. Rocha, B. Lopes, R. Company, A. Medeiros, R. Norberto, R. Pereira, O. Araujo and M. J. Bebianno, A multibiomarker approach in the clam Ruditapes decussatus to assess the impact of pollution in the Ria Formosa lagoon, South Coast of Portugal, Mar. Environ. Res., 2012, 75, 23–34 CrossRef CAS PubMed.
- I. Martorell, G. Perello, R. Marti-Cid, V. Castell, J. M. Llobet and J. L. Domingo, Polycyclic aromatic hydrocarbons (PAH) in foods and estimated PAH intake by the population of Catalonia, Spain: temporal trend, Environ. Int., 2010, 36, 424–432 CrossRef CAS PubMed.
- K. H. Kim, S. A. Jahan, E. Kabir and R. J. C. Brown, A review of airborne polycyclic aromatic hydrocarbons (PAHs) and their human health effects, Environ. Int., 2013, 60, 71–80 CrossRef CAS PubMed.
- C. Costello, L. Cao, S. Gelcich, M. A. Cisneros-Mata, C. M. Free, H. E. Froehlich, C. D. Golden, G. Ishimura, J. Maier, I. Macadam-Somer, T. Mangin, M. C. Melnychuk, M. Miyahara, C. L. de Moor, R. Naylor, L. Nostbakken, E. Ojea, E. O'Reilly, A. M. Parma, A. J. Plantinga, S. H. Thilsted and J. Lubchenco, The future of food from the sea, Nature, 2020, 588, 95–100 CrossRef CAS PubMed.
- H. L. Jia, Y. Q. Chang, Y. Q. Sun, D. G. Wang, X. J. Liu, M. Yang, D. D. Xu, B. Meng and Y. F. Li, Distribution and Potential Human Risk of Organochlorine Pesticides in Market Mollusks from Dalian, China, Bull. Environ. Contam. Toxicol., 2010, 84, 278–284 CrossRef CAS PubMed.
- B. Markert, S. Wuenschmann, S. Fraenzle, O. Wappelhorst, V. Weckert, G. Breulmann, R. Djingova, U. Herpin, H. Lieth, W. Schroder, U. Siewers, E. Steinnes, B. Wolterbeek and H. Zechmeister, On the road from environmental biomonitoring to human health aspects: monitoring atmospheric heavy metal deposition by epiphytic/epigeic plants: present status and future needs, Int. J. Environ. Pollut., 2008, 32, 486–498 CrossRef CAS.
- S. M. Almeida, H. Wolterbeek, B. Markert and S. Loppi, Biomonitoring of atmospheric pollution: possibilities and future challenges, Environ. Sci. Pollut. Res., 2017, 24, 11865–11866 CrossRef PubMed.
- C. L. V. Bastolla, D. Lima, J. J. Mattos, V. H. V. Dias, B. P. H. Righetti, C. H. A. M. Gomes, H. Cella, I. M. M. Reis, M. Saldaña-Serrano, C. P. Ferreira, M. C. Bícego, S. Taniguchi, F. L. Zacchi and A. C. D. Bainy, Comparative biochemical and molecular responses of biotransformation and antioxidant systems in three species of Crassostrea (Sacco, 1897) oysters exposed to chrysene, Comp. Biochem. Physiol., Part C, 2023, 270, 109642 CAS.
- Y. F. Gao, R. Y. Wang, Y. Y. Li, X. B. Ding, Y. M. Jiang, J. F. Feng and L. Zhu, Trophic transfer of heavy metals in the marine food web based on tissue residuals, Sci. Total Environ., 2021, 772, 145064 CrossRef CAS PubMed.
- R. W. Ji, L. Q. Pan, R. M. Guo, L. Zheng and M. Y. Zhang, Using multi-integrated biomarker indexes approach to assess marine quality and health status of marine organism: a case study of Ruditapes philippinarum in Laizhou Bay, China, Environ. Sci. Pollut. Res., 2019, 26, 9916–9930 CrossRef CAS PubMed.
- P. K. Hopke, Review of receptor modeling methods for source apportionment, J. Air Waste Manage. Assoc., 2016, 66, 237–259 CrossRef PubMed.
- H. Gupta, PAH determination in effluent and sludge samples of paper industry, Environ. Technol. Innovation, 2018, 9, 115–121 CrossRef.
- R. M. Cavalcante, F. W. Sousa, R. F. Nascimento, E. R. Silveira and G. S. S. Freire, The impact of urbanization on tropical mangroves (Fortaleza, Brazil): Evidence from PAH distribution in sediments, J. Environ. Manage., 2009, 91, 328–335 CrossRef CAS PubMed.
- M. M. Ahmed, P. Doumenq, M. O. Awaleh, A. D. Syakti, L. Asia and S. Chiron, Levels and sources of heavy metals and PAHs in sediment of Djibouti-city (Republic of Djibouti), Mar. Pollut. Bull., 2017, 120, 340–346 CrossRef PubMed.
- M. Oliveira, F. Gomes, Á. Torrinha, M. J. Ramalhosa, C. Delerue-Matos and S. Morais, Commercial octopus species from different geographical origins: Levels of polycyclic aromatic hydrocarbons and potential health risks for consumers, Food Chem. Toxicol., 2018, 121, 272–282 CrossRef CAS PubMed.
-
US-EPA, EPA Positive Matrix Factorization (PMF), 5.0 Fundamentals & User Guide, 2014, from. https://www.epa.gov/air-research/positive-matrix-factorization-model-environmental-data-analyses Search PubMed.
- U. M. Sofowote, B. E. Mccarry and C. H. Marvin, Source apportionment of PAH in Hamilton Harbour suspended sediments: comparison of two factor analysis methods, Environ. Sci. Technol., 2008, 42, 6007–6014 CrossRef CAS PubMed.
- D. Wang, F. Tian, M. Yang, C. Liu and Y. F. Li, Application of positive matrix factorization to identify potential sources of PAHs in soil of Dalian, China, Environ. Pollut., 2009, 157, 1559–1564 CrossRef CAS PubMed.
- E. Jang, M. S. Alam and R. M. Harrison, Source apportionment of polycyclic aromatic hydrocarbons in urban air using positive matrix factorization and spatial distribution analysis, Atmos. Environ., 2013, 79, 271–285 CrossRef CAS.
- S. B. Wang, Y. Q. Ji, J. B. Zhao, Y. Lin and Z. Lin, Source apportionment and toxicity assessment of PM2.5-bound PAHs in a typical iron-steel industry city in northeast China by PMF-ILCR, Sci. Total Environ., 2020, 713, 136428 CrossRef CAS PubMed.
- E. Stogiannidis and R. Laane, Source characterization of polycyclic aromatic hydrocarbons by using their molecular indices: an overview of possibilities, Rev. Environ. Contam. Toxicol., 2015, 234, 49–133 CAS.
- X. Qian, B. C. Liang, W. J. Fu, X. H. Liu and B. S. Cui, Polycyclic aromatic hydrocarbons (PAHs) in surface sediments from the intertidal zone of Bohai Bay, Northeast China: spatial distribution, composition, sources and ecological risk assessment, Mar. Pollut. Bull., 2016, 112, 349–358 CrossRef CAS PubMed.
- R. M. Harrison, D. J. T. Smith and L. Luhana, Source apportionment of atmospheric polycyclic aromatic hydrocarbons collected from an urban location in Birmingham, U.K, Environ. Sci. Technol., 1996, 30, 825–832 CrossRef CAS.
- F. Emanuela, A. Anna, B. Mattia, P. Piero, T. Cristina and M. Mauro, Polycyclic aromatic hydrocarbon (PAH) accumulation in different common sole (Solea solea) tissues from the North Adriatic Sea peculiar impacted area, Mar. Pollut. Bull., 2018, 137, 61–68 CrossRef PubMed.
- Y. Ding, Z. Q. Wu, R. J. Zhang, K. F. Yu, Y. H. Wang, Q. Zou, W. B. Zeng and M. W. Han, Organochlorines in fish from the coastal coral reefs of Weizhou Island, south China sea: levels, sources, and bioaccumulation, Chemosphere, 2019, 232, 1–8 CrossRef CAS PubMed.
- R. S. Piazza, R. Trevisan, F. Flores-Nunes, G. Toledo-Silva, N. Wendt, J. J. Mattos, D. Lima, S. Taniguchi, S. T. Sasaki, A. C. P. Mello, F. L. Zacchi, M. A. S. Serrano, C. H. A. M. Gomes, M. C. Bicego, E. A. de Almeida and A. C. D. Bainy, Exposure to phenanthrene and depuration: changes on gene transcription, enzymatic activity and lipid peroxidation in gill of scallops Nodipecten nodosus, Aquat. Toxicol., 2016, 177, 146–155 CrossRef CAS PubMed.
- J. Noh, H. Kim, C. Lee, S. J. Yoon, S. Chu, B. O. Kwon, J. Ryu, J. J. Kim, H. Lee and U. H. Yim, Bioaccumulation of polycyclic aromatic hydrocarbons (PAHs) by the marine clam, mactra veneriformis, chronically exposed to oil-suspended particulate matter aggregates, Environ. Sci. Technol., 2018, 52, 7910–7920 CrossRef CAS PubMed.
- D. Y. Li, T. Liu, L. Q. Pan, F. X. Hu and Q. Jin, Bioaccumulation and oxidative damage of polycyclic aromatic hydrocarbon mixtures in Manila clam Ruditapes philippinarum, Ecotoxicol. Environ. Saf., 2020, 197, 110558 CrossRef CAS PubMed.
- Y. X. Zhang, H. Y. Chen, C. Liu, R. H. Chen, Y. Y. Wang and Y. G. Teng, Developing an integrated framework for source apportionment and source-specific health risk assessment of PAHs in soils: Application to a typical cold region in China, J. Hazard. Mater., 2021, 415, 125730 CrossRef CAS PubMed.
-
National Bureau of Statistics of China, China Statistics Yearbook 2022, 2022, from. http://www.stats.gov.cn/sj/ndsj/2022/indexeh.htm Search PubMed.
- I. C. T. Nisbet and P. K. Lagoy, Toxic equivalency factors (TEFs) for polycyclic aromatic hydrocarbons (PAHs), Regul. Toxicol. Pharmacol., 1992, 16, 290–300 CrossRef CAS PubMed.
- Y. Z. Li, H. Y. Chen and Y. G. Teng, Source apportionment and source-oriented risk assessment of heavy metals in the sediments of an urban river-lake system, Sci. Total Environ., 2020, 737, 140310 CrossRef CAS PubMed.
- R. Fuller, P. J. Landrigan, K. Balakrishnan, G. Bathan, S. Bose-O’Reilly, M. Brauer, J. Caravanos, T. Chiles, A. Cohen, L. Corra, M. Cropper, G. Ferraro, J. Hanna, D. Hanrahan, H. Hu, D. Hunter, G. Janata, R. Kupka, B. Lanphear, M. Lichtveld, K. Martin, A. Mustapha, E. Sanchez-Triana, K. Sandilya, L. Schaefli, J. Shaw, J. Seddon, W. Suk, M. M. Tellez-Rojo and C. H. Yan, Pollution and health: a progress update, Lancet Planet. Health, 2022, 6, e535–e547 CrossRef PubMed.
|
This journal is © The Royal Society of Chemistry 2024 |