How do certain atmospheric aerosols affect Cu-binding organic ligands in the oligotrophic coastal sea surface microlayer?†
Received
20th September 2023
, Accepted 4th December 2023
First published on 7th December 2023
Abstract
It is still unclear how the chemical speciation of Cu in surface seawater is impacted by aerosols from various sources deposited on the sea surface, which is surprising, considering the environmental importance of Cu. Therefore, we used voltammetry to investigate Cu complexing capacity (CuCC) in the sea surface microlayer (SML) and in the underlying water (ULW) of the oligotrophic middle Adriatic Sea during February–July 2019. The focus was on the impacts of specific atmospheric processes such as open-fire biomass burning (BB), pollination season and Saharan dust intrusion. The presence of ligand class L2 (19.9–392.0, average 63.8, median 43.1) nM; log
K2 (8.3–10.2, average 9.6, median 9.6) was observed in all samples, while ligand class L1 (40.5–76.1, average 53.6, median 48.9) nM; log
K1 (10.3–11.1, average 10.6, median 10.5) was found in only 25% of SML samples. Throughout the period, the SML was enriched with organic ligands by a factor of up to 9.1 compared to the ULW, mainly due to the high sensitivity of the SML to specific atmospheric depositions. In addition, measurements with corresponding specific model aerosols were conducted to analyse their impacts on CuCC. Pollen directly affected CuCC in the SML by increasing the concentration of allochthonous ligands such as proteins. The deposition of BB aerosols rich in nutrients and trace metals stimulated the biological production of organic ligands, showing an indirect effect on CuCC delayed by up to two weeks. Finally, Saharan dust had a negligible impact on CuCC. This study illustrates the susceptibility of oligotrophic coastal area to the effects of pollen and open-fire BB aerosols in altering the Cu-binding organic ligands in the SML.
Environmental significance
It is still unclear how aerosols of pollen, biomass burning (BB) or Saharan dust affect the chemical speciation of Cu(II) in the sea surface microlayer (SML). The interaction of Cu(II) with organic ligands is very important because it controls bioavailable Cu(II) concentration, balancing its beneficial nutritive and harmful toxic effects for marine microorganisms. After deposition on the SML, pollen directly affected the Cu-binding ligand pool by increasing the concentration of allochthonous ligands such as proteins, open-fire BB aerosols rich in inorganic nutrients showed an indirect effect by stimulating the biological production of autochthonous organic ligands, while Saharan dust intrusion showed a negligible effect on Cu-binding ligands. The atmospheric input of pollen and BB aerosols affects Cu speciation in oligotrophic coastal seas.
|
1. Introduction
The atmosphere represents one of the most important transport pathways of various natural and anthropogenic aerosols from the continents to the oceans.1 Through atmospheric deposition, aerosols, a complex mixture of organic and inorganic compounds, directly affect marine biochemical processes such as primary and secondary production, and indirectly influence the atmospheric carbon dioxide budget and the global climate. Aerosols can supply seawater with macronutrients (N, P, Si), trace metals,2–4 and a wide range of organic compounds.5 Some trace metals are micronutrients and essential for phytoplankton growth (e.g. Fe, Cu, Mn, Zn, Co, …), while some others can induce toxic effects (e.g. Hg, Pb, Cd). The biological availability of trace metals entering seawater via atmospheric deposition is regulated by their chemical speciation, interactions (with organic matter, interfaces, biota),6 chemical transformations, solubility,2,7,8 leaching9 and uptake kinetics.10 Atmospheric deposition is one of the most important sources of water-soluble organic carbon in seawater,11,12 which serves as a carbon source for heterotrophic bacteria and thus plays a role in the biogeochemical carbon cycle.13 Due to the ubiquitous global function of phytoplankton, which is responsible for the sequestration of about half of atmospheric carbon dioxide through photosynthesis, the utilization of supplied external inorganic nutrients14 and resilience to pressures from externally supplied anthropogenic organic pollutants15,16 appear to be very important for its growth and reproduction. In general, the response of phytoplankton and heterotrophic bacteria to atmospheric deposition depends on the trophic status of the marine environment. Oligotrophic marine areas, where primary production is limited in terms of available nutrients, are particularly sensitive to atmosphere-water interactions, especially to the fertilizing potential of aerosols.5,17,18
The first direct contact of atmospheric aerosols with seawater occurs within the sea surface microlayer (SML), the largest natural boundary layer placed within the uppermost 1 mm of the sea surface that connects the atmosphere and the ocean. Due to the direct solar radiation exposure, the SML is a specific biogeochemical environment with a broad spectrum of aquatic microorganisms (neuston) the metabolic activity of which determines the production, degradation and modification of organic matter within the SML.19 The SML is a specific region of the water column dominated by different types of organic substances, which can be produced in situ within the SML,20 transported from subsurface waters21 or originate from the atmospheric and terrestrial input.22,23 The surface-active organic matter readily accumulates at the air–water interface, causing a decrease in surface tension24 and influencing the flux of energy and gasses.
Copper is one of the most studied and monitored trace metals in seawater due to its nutritive as well as, depending on the concentration, harmful effect to certain phytoplankton species.1,25 Atmospheric deposition to seawater represents one of the exogenous sources of Cu,4,26 influencing biochemical processes in seawater.1,27 The chemical speciation of Cu in seawater is mainly controlled by interactions with dissolved organic ligands via complex formation.28 Different organic molecules could serve as Cu-binding organic ligands in seawater. Field studies29 supported by laboratory bioassay experiments with phytoplankton cultures30,31 and macroalgal species32 point that autochthonous ligands of biological origin, generally belonging to the L1 ligand group, bind Cu in surface seawater. The exudation of organic ligands to form inert Cu complexes in seawater may be one of the strategic ways for phytoplankton to reduce the concentration of bioavailable (free/hydrated) Cu2+ to very low levels (0.001–10 pM).30 On the other hand, L2 ligands are usually found in the deep waters, where they originate from passive production associated with grazing, decay of organic matter and bacterial transformations.29 In addition, allochthonous ligands such as terrestrial humic substances appear as relatively strong Cu-binding ligands in coastal and estuarine waters.33
In this study, we investigated the influence of atmospheric deposition on the organic Cu complexing capacity (CuCC) parameters (concentration of organic ligand classes, Li, and their apparent stability constants, Ki) of the surface layers (SML and underlying water, ULW from ∼1 m depth) at the central Adriatic coast. The objective was to answer the following questions: (i) how do the CuCC parameters change in the upper surface layers of the coastal waters from winter to summer, and (ii) to what extent were Cu-binding ligands affected by specific atmospheric depositions related to registered atmospheric events such as open-fires of local vegetation, pollination period and Saharan dust intrusion. To address the possible extent and nature of the influence of each specific event typical for the entire Mediterranean coastal area on the CuCC parameters in the SML, we additionally analysed water leachate of filter-collected aerosols of (i) simulated biomass burning (BB) and (ii) Saharan dust, as well as (iii) model solutions of different pollen from anemophilous plants characteristic for the middle Adriatic coast. Based on the redox processes of organic molecules in the field samples and in specific leachate solutions detected by electrochemical methods on the working electrode, we identified protein-like material and reduced sulphur species (RSS) as organic ligands that specifically bind Cu in the SML. In addition, we discussed the environmental role of atmospheric deposition in the interactions between Cu and organic matter as well as in the biogeochemical processes of organic matter and Cu ions in the SML of oligotrophic coastal marine areas relevant for the Adriatic Sea and the entire Mediterranean region.
2. Materials and methods
2.1. Sampling area and environmental conditions
Seawater samples were collected between February 6th and July 10th, 2019 at Jadrija (J) and Martinska (M) stations in the Šibenik archipelago's coastal zone at the eastern part of the middle Adriatic Sea (Croatia, Fig. 1). This marine area is characterized by oligotrophic conditions with poor biological production,34 with low influence from the karstic Krka River runoff, and with Šibenik town (population 34
000) having low industrial activity.
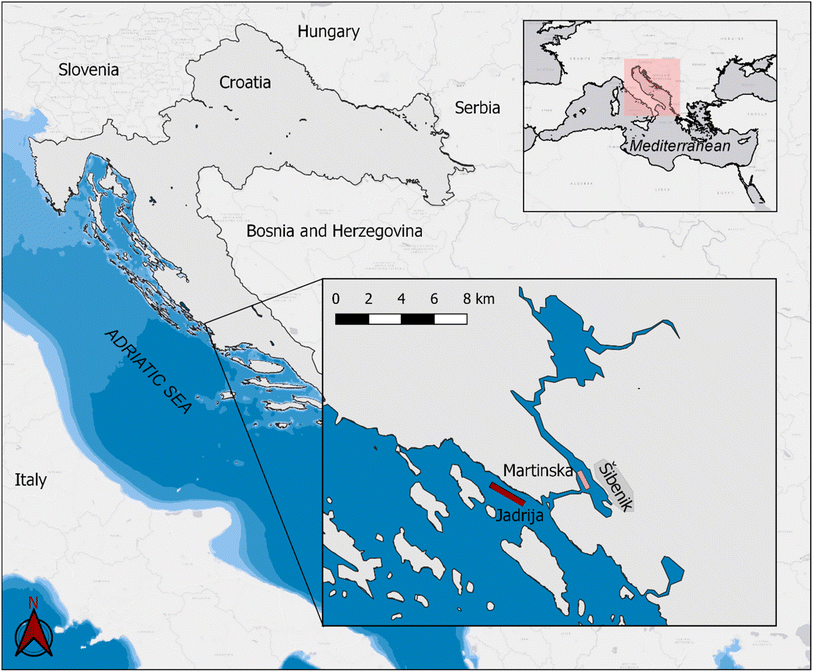 |
| Fig. 1 Jadrija and Martinska stations in the coastal zone of Šibenik archipelago in the middle Adriatic Sea. The map was created using open source QGIS (http://qgis.osgeo.org/). | |
Environmental conditions on the days of sampling are listed in Table 1. Sampling was mostly conducted in sunny and calm weather (wind speed ranged from 0.6 to 4.2 m s−1), with no or little precipitation on the day of sampling (<7.6 mm), ensuring stability of the SML and no depletion of organic matter. Salinity in the SML and ULW samples at Jadrija station ranged between 29.3 and 38.5 psu, while at Martinska station, located in the Krka River estuary, it was between 5.5 psu in the SML and 22.6 psu in the ULW. Water temperature ranged between 6.2 °C in winter and 26.3 °C in summer for both stations.
Table 1 Environmental conditions at the days of sampling during the middle Adriatic Sea campaign in 2019
Date |
Sample station |
Weather condition |
Wind speed m s−1 |
Wind direction |
Precipitation mm |
SML |
ULW |
S/psu |
T/°C |
S/psu |
T/°C |
06.02 |
J |
Sunny |
0.9 |
NW |
0 |
38.06 |
12.82 |
36.20 |
13.20 |
20.02 |
J |
Sunny |
3.8 |
SW |
0.2 |
29.30 |
12.40 |
36.20 |
13.25 |
05.03 |
J |
Sunny |
0.6 |
N/NW |
0.3 |
37.94 |
12.62 |
38.15 |
12.88 |
20.03 |
J |
Sunny |
4.2 |
NE |
0 |
38.38 |
13.59 |
38.38 |
13.58 |
01.04 |
J |
Sunny |
0.8 |
NE |
0 |
37.79 |
14.38 |
37.96 |
14.41 |
16.04 |
J |
Sunny |
0.9 |
SE |
0 |
36.48 |
14.67 |
36.94 |
14.74 |
29.04 |
J |
Cloudy |
2.6 |
N |
0 |
37.92 |
16.22 |
37.92 |
16.23 |
15.05 |
J |
Sunny |
3.8 |
N/NE |
0 |
38.42 |
16.12 |
38.45 |
16.08 |
29.05 |
J |
Sunny |
3.8 |
S |
7.6 |
36.88 |
19.23 |
37.14 |
18.55 |
12.06 |
J |
Sunny |
3.7 |
S |
0 |
31.31 |
22.93 |
33.88 |
23.01 |
27.06 |
J |
Sunny |
1.6 |
NE |
0 |
36.89 |
23.88 |
37.22 |
23.46 |
10.07 |
J |
Sunny |
3.7 |
S/SW |
5.9 |
34.79 |
25.41 |
34.75 |
25.41 |
06.02 |
M |
Sunny |
0.9 |
NW |
0 |
5.53 |
9.44 |
6.23 |
6.20 |
01.04 |
M |
Sunny |
2.8 |
NE/SE |
0 |
14.88 |
13.22 |
22.60 |
14.38 |
29.05 |
M |
Sunny |
2.5 |
S |
7.6 |
9.12 |
19.96 |
11.16 |
19.79 |
10.07 |
M |
Sunny |
2.8 |
S |
5.9 |
21.25 |
26.16 |
22.53 |
26.29 |
2.2. Meteorological situation and specific events
The area is under the influence of Mediterranean climate with temperate, wet winters and warm to hot, dry summers, with high to very high fire risk. According to Köppen's classification, the researched area belongs to the Csa climate, with the immediate hinterland characterised by the Cfa climate.35 The temporal variations of the average daily meteorological parameters such as wind speed, air temperature, precipitation, and relative humidity (Fig. 2A) were measured at the Martinska marine station.4,20 Briefly, the air temperature showed a clear seasonal pattern with a cold winter and a warm summer, with strong N to N/NE bora winds in winter and southerly winds in spring/summer. Most precipitation occurred in April and May, while relative humidity ranged from 24 to 89% (Fig. 2A).
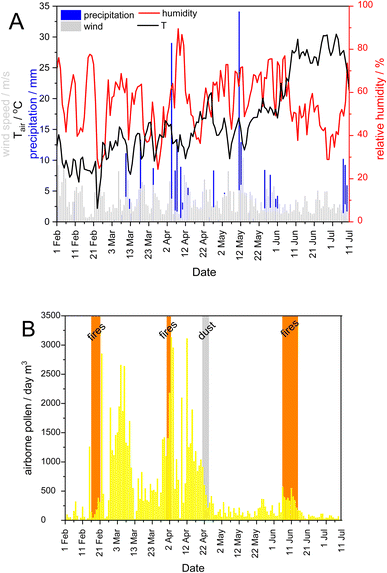 |
| Fig. 2 (A) Daily average meteorological parameters recorded at Martinska marine station and (B) airborne pollen concentrations (yellow) with marked open-fire BBs (orange) and Saharan dust (grey) events identified during the sampling campaign in the middle Adriatic coastal area. (For interpretation of the references to colour in this figure legend, the reader is referred to the Web version of this article.) | |
Three types of specific atmospheric events were registered during our sampling campaign: open-fire biomass burnings (BB), Saharan dust intrusion and pollination season (Fig. 2B). According to the air-mass backward trajectories, the period of Saharan dust intrusion was identified on April 21st–25th, 2019 (Fig. S1†). Three intense open-fire BB events in winter (February 16th–21st) and spring (March 31st–April 2nd, June 6th–15th) were based on air-mass backward trajectory analysis and archival data from the Šibenik County Fire Department (http://www.vatrogastvo-sibenik-knin.hr/). The latter data usually contain information on the type of operation, the duration of the fire, the area attacked, and the type of vegetation affected. The sampling campaign coincided with the main pollination period of the trees and the highest airborne pollen concentrations in March and April (https://www.plivazdravlje.hr/alergije/kalendar/21/Sibenik.html). Local wind speed, air temperature, precipitation, and/or relative humidity appeared to be directly responsible for the occurrence and permanence of extreme pollen events characterised by high airborne pollen concentrations over a period of time in the Mediterranean area.36–38
2.3. Seawater sample collection and treatment
The SML samples (microlayer thickness δ = 20 ± 3 μm) were collected using an in-house constructed rotating drum sampler made of polymethyl methacrylate (PMMA, plexiglas) driven by a battery-powered geared electric motor (Fig. S2†) and stripped via a PTFE wiper into pre-cleaned (nitric acid, p.a.) PE bottles. The drum dimensions are as follows: length = 49.8 cm, diameter = 39.6 cm, with a rotation speed of 8 rpm. The sampler is constructed in a way that the drum is placed a few cm below the water level to ensure that the whole surface of the drum crosses through the interface upon rotation. The ULW was collected directly from the rubber boat from a depth of about 1 m, also in PE bottles. All bottles were sealed in zip-lock plastic bags and immediately frozen until analysed. Prior to analysis, samples were thawed and filtered through pre-cleaned (180 mL MQ water + 180 mL UV-irradiated seawater) cellulose acetate (CA) capsule filters (0.45 μm pore size, Minisart, Sartorius, Göttingen, Germany) mounted on a syringe. Freezing/thawing could lead to the release of organic molecules from the cells, which in turn could alter the amount of Cu-binding ligands, probably by increasing their concentration in the dissolved fraction. If so, the ligand concentrations determined in this study might have been overestimated to a small extent. However, their trend over the studied period will most probably have remained the same because all samples were treated in the same way. Additionally, our results are highly comparable to the CuCC determined in the SML in the eastern Mediterranean, as discussed in Section 3.2.1, and they also fit well with the other results obtained during the 2019 BiREADI campaign.4,20,39 Seawater salinity and temperature were measured using a SeaBird 25 CTD profiler.
2.4. Specific aerosol sampling and analysis: simulated BB and Saharan dust
The PM10 aerosols (particulate matter with an aerodynamic diameter da < 10 μm) produced by the combustion of dry oak firewood as a simulation of a BB emission were collected on pre-combusted quartz fiber filters (d = 140 mm, Whatman) using a high-volume aerosol sampler (flow rate 32 m3 h−1, Kalman System Co., Hungary). The firewood logs were burnt in a Servant S114 cast iron stove with a heating capacity of 5 kW. The stove was connected to a stack about 8 m high with an internal diameter of 120 mm. At the end of the stack a small amount of the flue gas was introduced into a dilution unit in which the sampling head of the high-volume aerosol sampler was placed. The filter sections containing 8.07 mg, 12.20 mg and 9.34 mg of aerosols collected in different phases of combustion were leached separately in UV-irradiated seawater by ultrasonic agitation for 20 min and subsequent storage at +4 °C for 24 h. The obtained solutions were filtered through a pre-cleaned 0.45 μm CA filter (Sartorius) and immediately analysed for organic components (CuCC, RSS, and protein-like material).
The PM10 aerosols of Saharan dust were collected on the Adriatic coast during a dust yield in June 2021 (Fig. S1†) on pre-combusted (450 °C for 4 h) quartz fiber filters (d = 47 mm, Pall Life Sciences, Port Washington, New York) using an automatic sequential low-volume reference sampler PNS 18T-DM-3.1 (according to EN 12341:2014, Comde-Derenda, Stahnsdorf, Germany). The sampler was operated at a volume airflow rate of 2.3 m3 h−1. A filter section containing 0.23 mg of the collected dust was leached in UV-irradiated seawater (ultrasonic agitation for 20 min and subsequent storage at +4 °C for 24 h) and the leachate was then filtered in the same way as the BB leachates, followed by the analyses of organic components (CuCC, RSS, and protein-like material). The water-soluble organic carbon of the corresponding unexposed filters (blanks) was subtracted from the one of exposed filters.
2.5. Sampling and analysis of tree pollen
Pollen was collected during the pollination season from the taxa Cupressus sempervirens L. and Juniperus communis L. (both from the family Cupressaceae), and Pinus nigra J. F. Arnold (family Pinaceae) in the Botanical Garden (Faculty of Science, University of Zagreb, Croatia). The selected species were chosen because the dominance of cypress and pine pollen had been confirmed for the middle Adriatic coastal area and the immediate hinterland.37 The mature anthers were collected directly from the plants in a paper bag and dried at room temperature. Pollen powder was separated from the anthers, weighed, and dissolved in UV-irradiated seawater to a concentration of 80 mg L−1 each to reach dissolved organic carbon (DOC) of 6.387, 7.351 and 7.117 mg C L−1 in order to reflect the DOC values measured in the SML samples on April 1st, 2019 (6.762 mg C L−1 at J and 7.250 mg C L−1 at M station), when airborne pollen concentration in Šibenik area was very high (>1500 day−1 m−3) and coincided with the BB event (Fig. 2B). The solution was filtered through a pre-cleaned 0.45 μm CA filter (Sartorius) and immediately analysed for organic matter components (CuCC, RSS, and protein-like material).
2.6. Instrumentation and reagents
The electrochemical instrumentation consisted of a μAUTOLAB potentiostat type II (EcoChemie, The Netherlands), a 663VA Stand three-electrode system (Metrohm, Herisau, Switzerland), and an Interface for Mercury Electrode (IME) module. A PC equipped with GPES 4.9 software was used for the automatic measurements and data acquisition. A static mercury drop electrode (SMDE) with a surface area of 0.52 mm2 served as the working electrode. Commercially available Ag/AgCl (3 M KCl) was used as the reference electrode and a glassy carbon rod as the counter electrode. Dissolved oxygen was removed from the samples by introducing nitrogen for 15 min before analysis. The deoxygenation and accumulation steps were accompanied by stirring at 3000 rpm with a Teflon stirrer. All measurements were performed at room temperature (23 ± 1 °C).
Stock solutions of Cu2+ were prepared by dilution of standard solution (Cu(NO3)2 in 0.5 M HNO3, traceable to SRM from NIST, 1000 mg L−1 Cu2+Certipur®, Merck-Millipore). Triton-X-100 (T-X-100), bovine serum albumin (BSA, lyophilised), and glutathione (GSH) were purchased from Sigma-Aldrich, Steinheim, Germany. Nitric acid of analytical purity (pro analysis, p.a.) and high purity (suprapur, s.p.) was bought from Fisher Scientific, UK. Deionised ultrapure Milli-Q water (18.2 MΩ cm resistivity) (Millipore, Burlington MA, USA) was used to prepare the diluted solutions and to rinse the cell and electrode system. The UV-irradiated seawater, free of organic matter, was prepared from the Adriatic seawater stored in the dark at +4 °C for at least 3 months. Filtered seawater (0.7 μm glass fiber filter, GF/F, Whatman) was irradiated for 24 h with a homebuilt UV system (250 W high-pressure mercury vapour lamp) to decompose natural organic matter.
2.7. Determination of CuCC parameters
Up to 99.6% of total dissolved Cu2+ (dCu; including organic Cu2+ complexes, inorganic Cu2+ complexes and free/hydrated Cu2+) in seawater is in the form of organic complexes.29 Their concentrations and apparent stability constants are determined by mathematical modelling after complexometric titration.40 Organic ligands with different Cu binding strengths contribute to CuCC, and according to a “discrete model” they are grouped based on similar affinities of Cu-binding functional groups. Therefore, the CuCC does not usually provide information about the structure of the ligands or complexes, but the concentrations of the individual groups (classes) of organic ligands (Li) and their corresponding conditional stability constants (Ki) are the main parameters describing the CuCC. The obtained K-s are only valid under the physicochemical conditions (temperature, salinity) of the analysed samples (Table 1).
Two aliquots of each of the filtered seawater sample, the UV-irradiated seawater-leached dust, the UV-irradiated seawater-leached BB aerosols, and the UV-irradiated seawater solution of model pollen were prepared for CuCC study. The concentration of total dCu was determined by differential pulse anodic stripping voltammetry (DPASV) in one aliquot and complexometric titration was done in the other. Before determination of total dCu, nitric acid (68% w/w HNO3, s.p., Merck) was added to the first filtrate aliquot to achieve a pH < 2, after which the samples were exposed to UV irradiation for 24 h to decompose organic ligands. The samples were then titrated with an increased concentration of Cu(NO3)2 standard solution and the peak current was measured. The DPASV measurement conditions were: accumulation potential, Ea −0.85 V, accumulation time, ta 300 s, step potential, Es 1.95 mV, amplitude, a 19.95 mV, modulation time, tmod 0.04 s, and interval time, tint 0.1 s. The total dCu concentration in the samples was calculated using the standard addition method. The accuracy of the DPASV method was verified using the Open Ocean Seawater Reference Material for Trace Metals (NASS-6), of the National Research Council of Canada, with a certified Cu value of 0.248 ± 0.025 μg L−1. The measured total dCu concentration was within 5% of the certified value.
The second filtrate aliquot (at natural pH) was also titrated with Cu2+ standard solution. A period of 15 min was allowed after each Cu2+ addition for its distribution to reach equilibrium. The DPASV was applied under the following conditions: Ea −0.60 V, ta 120 s, Es 4.05 mV, a 25.02 mV, tmod 0.05 s, and tint 0.61 s. The obtained titration curves (peak current vs. (dCu + added Cu)) were mathematically transformed into the Ružić-van den Berg, Langmuir/Gerringa and Scatchard complexation models embedded in the ProMCC software41 in order to calculate the CuCC parameters: Li and corresponding log
Ki of the complexes. A linear shape of the Scatchard plot indicated the presence of 1 class of ligands, while a convex shape suggested the presence of 2 classes of ligands.40
2.8. Determination of protein-like ligands
Constant current chronopotentiometric stripping (CPS) was used for the detection of protein-like material in the dissolved seawater fraction. The CPS analysis is based on the detection of “peak H” at highly negative potentials around −1.85 V (vs. Ag/AgCl reference electrode).42,43 Peak H appears in a slightly alkaline solution such as seawater due to the hydrogen evolution reaction catalysed only by the adsorbed protein-like material.44 CPS was performed under the following conditions: Ea −0.6 V, ta 60 s, 120 s or 180 s, stripping current Istr = −3 μA, −4 μA, −5 μA (seawater samples, simulated BB and dust leachate), −15 μA (model pollen solution) and maximum measurement time 3 s. In contrast to previous CPS quantifications of protein-like material in seawater samples using a calibration plot constructed with the concentration range of a standard protein,42,43 here we used the BSA standard addition method for the first time. In this way, peak H was successfully detected by adjusting ta and Istr for each sample, which would not have been possible using a preconstructed calibration plot, because of the unexpectedly high amounts of protein-like material in SML samples. The protein-like material is expressed in the equivalent concentration of BSA, a common protein standard used in many analytical protocols, including the analysis of dissolved proteins and commassie-stained protein particles (CSP) in seawater.
2.9. Determination of reduced sulphur species (RSS)
The electrochemical determination of RSS is based on the reaction of sulfhydryl groups with the mercury working electrode forming Hg-SR, followed by its cathodic stripping reduction and the appearance of a peak around −0.6 V.45 Square wave voltammetry (SWV) was applied to detect dissolved RSS under the following conditions: Ea −0.2 V, ta 180 s, Es 4.05 mV, a 25.05 mV and f 50 Hz. Reduced glutathione (GSH) was used for calibration, with a detection limit of 5.3 nM. GSH is found in oxic seawater layers at the surface at nM concentrations,45,46 which makes it a good model for the quantification of RSS in the SML and ULW samples.
2.10. Surface-active substances (SAS) determination
SAS were quantified in the dissolved seawater fraction using alternating current (AC) voltammetry with an out of phase mode (phase angle 90°), Ea −0.6 V, ta 30 s, f 77 Hz, a 10 mV, and Es 40 mV. The method detects the decrease in capacitive current due to the adsorption of SAS at the electrode–electrolyte interface and the perturbation of the double layer capacitance.47 The non-ionic surfactant T-X-100 was used as a model calibration compound for SAS with a detection limit of 0.02 mg L−1. T-X-100 is commonly used for the quantification of SAS in marine SML and ULW samples.24,48–50
2.11. Dissolved organic carbon (DOC) measurement
For the analysis of DOC and water-soluble organic carbon, samples were filtered using pre-combusted (450 °C for 4 h) glass fiber filters (GF/F 0.7 μm Whatman, Maidestone, UK) in an all-glass filtering system (Millipore, Burlington MA, USA). Filtrates for the analysis were collected in duplicates in 20 mL glass tubes precleaned with chromium-sulfuric acid, rinsed with Milli-Q water, and pre-combusted at 450 °C for 4 h. Samples were preserved with HgCl2 (final concentration 10 mg L−1) and stored in the dark at 4 °C until analysis. Measurements were performed using a TOC-VCPH analyser (Shimadzu) with platinum silica catalyst and nondispersive infrared (NDIR) detector for CO2 measurements. The concentration of organic carbon was calculated as an average of 2 replicate samples. The average of the instrument and the Milli-Q blank sample corresponded to 0.03 mg L−1 (n = 33) with high reproducibility (1.6%).
2.12. Data analysis and interpretation
The analysis of backward trajectories of air masses was performed using the NOAA Hybrid Single-Particle Lagrangian Integrated Trajectory (HYSPLIT) transport model,51,52 with Global Data Assimilation System (GDAS; 1°, global, 2006 – present). The back trajectories were calculated for 72 h time intervals with a 24 h frequency (Fig. S1†). The plots represent a trajectory ensemble of 3 individual backward trajectories, terminating at the sampling point at 5 m above ground level.
All graphs were created in OriginPro 9.0. The strength and direction of linear correlation was expressed by the Pearson correlation coefficient (r), with a statistical significance level p < 0.05.
The enrichment factors (EF), indicating a degree to which the SML is enriched in a specific analyte (X) relative to the ULW, were calculated as the ratio of concentration of X in the SML and ULW:
EF > 1 indicates the enrichment in the SML due to accumulation of material at the air–sea interface transported either from the water column or deposited from the atmosphere, while EF < 1 indicates the depletion of a certain analyte in the SML compared to the ULW.
3. Results
3.1. Overcoming organic surfactants' interference on Cu redox processes on the electrode
SAS usually account for a significant fraction of the natural organic matter pool in a seawater column,53 with the SML representing a top layer, which is especially dominated by SAS.24 In the middle Adriatic's SML and ULW samples, surface-active electro(in)active organic substances (SAS) were present in the range 0.028–68.4 and 0.005–3.6 eq. mg T-X-100 L−1, respectively (Tables 2 and 3). Using DPASV, we examined complexometric titrations of Cu in two selected samples whose DOC and SAS concentrations were higher than the average DOC and SAS values found in the SML (0.798 mg C L−1; eq. 0.179 mg T-X-100 L−1) and in the ULW (0.878 mg C L−1; eq. 0.106 mg T-X-100 L−1). The selected samples were: the SML sample collected on April 16th (1.16 mg C L−1, eq. 0.196 mg T-X-100 L−1) and the ULW sample on April 1st (0.971 mg C L−1, eq. 0.398 mg T-X-100 L−1). Three very high DOC and SAS values determined for the SML at both stations in April (Table 2) were excluded from the calculated average DOC and SAS values. SAS interferences were significant during DPASV measurements in both samples, which resulted in a lower sensitivity of the technique. To overcome this, we considered the application of a desorption step applied after the accumulation54 and the addition of competing hydrophobic surfactant such as T-X-100.33 SAS normally desorb from the electrode surface at potentials more negative than −1.3 V.24 We applied desorption potential, Ed −1.4 V for 1 s (Fig. S3A†), which provided higher Cu reoxidation peak and this step was therefore used in all ASV measurements. Moreover, according to the dependence of the Cu peak intensity on the range of T-X-100 concentrations (0.2–10 mg L−1, Fig. S3B†), 1 mg L−1 of T-X-100 was added to all our samples prior to ASV measurement, ensuring much higher sensitivity. An adsorbed layer of T-X-100 does not affect the electrochemical processes of Cu, but increases the peak of Cu reoxidation.33,55 The pseudopolarograms observed in UV-irradiated seawater and in the selected SML and ULW samples showed substantial differences when recorded with and without the addition of T-X-100 (Fig. S3C†). At the Ea = −0.6 V, which was chosen for the selective detection of labile Cu species, the peak current was higher for all three electrolytes, showing the benefit of T-X-100 addition.
Table 2 Concentrations of L1 and L2 class of ligands with corresponding K-s, concentrations of DOC, SAS, dCu, normalized Ltot/DOC values, RSS and protein-like material in SML samples. LOD-limit of detection
Date |
SML station |
[L1]/nM |
log K1 |
[L2]/nM |
log K2 |
DOC mg C L−1 |
SAS eq. mg T-X-100 L−1 |
[dCu]/nM |
[Ltot]/DOC μmol mg−1 C−1 |
RSS eq. nM GSH |
Protein material eq. nM BSA |
06.02 |
J |
|
|
119.0 ± 4.2 |
9.6 ± 0.1 |
0.985 |
0.168 |
20.12 ± 0.84 |
0.12 |
6.9 ± 3.1 |
<LOD |
20.02 |
J |
|
|
28.4 ± 0.7 |
9.7 ± 0.0 |
0.793 |
0.028 |
2.98 ± 0.14 |
0.04 |
<LOD |
<LOD |
05.03 |
J |
40.5 ± 14.6 |
11.1 ± 2.8 |
42.9 ± 12.8 |
8.9 ± 0.3 |
1.521 |
0.658 |
22.52 ± 0.78 |
0.05 |
5.9 ± 3.2 |
<LOD |
20.03 |
J |
|
|
64.1 ± 2.8 |
9.3 ± 0.1 |
0.877 |
0.028 |
5.14 ± 0.30 |
0.07 |
<LOD |
<LOD |
01.04 |
J |
|
|
392.0 ± 8.3 |
9.1 ± 0.1 |
6.762 |
58.279 |
11.53 ± 0.49 |
0.06 |
76.5 ± 6.5 |
0.40 ± 0.31 |
16.04 |
J |
52.8 ± 9.9 |
10.3 ± 0.4 |
66.8 ± 8.2 |
8.6 ± 0.2 |
1.160 |
0.196 |
13.20 ± 1.19 |
0.10 |
26.1 ± 2.7 |
1.63 ± 0.56 |
29.04 |
J |
|
|
46.8 ± 1.2 |
9.48 ± 0.05 |
1.026 |
0.166 |
3.82 ± 0.22 |
0.05 |
<LOD |
<LOD |
15.05 |
J |
|
|
36.2 ± 1.6 |
9.5 ± 0.1 |
1.002 |
0.034 |
3.27 ± 0.35 |
0.04 |
<LOD |
<LOD |
29.05 |
J |
|
|
41.0 ± 1.1 |
9.6 ± 0.0 |
0.420 |
0.028 |
2.38 ± 0.42 |
0.10 |
<LOD |
<LOD |
12.06 |
J |
|
|
44.2 ± 1.8 |
9.7 ± 0.1 |
0.670 |
0.080 |
4.84 ± 0.17 |
0.07 |
<LOD |
<LOD |
27.06 |
J |
45.0 ± 14.5 |
10.4 ± 0.4 |
49.4 ± 11.0 |
8.8 ± 0.4 |
0.920 |
0.263 |
12.09 ± 1.12 |
0.10 |
6.3 ± 3.2 |
<LOD |
10.07 |
J |
|
|
40.5 ± 1.5 |
10.0 ± 0.1 |
0.205 |
0.054 |
7.83 ± 0.88 |
0.20 |
<LOD |
<LOD |
06.02 |
M |
|
|
78.2 ± 2.3 |
9.5 ± 0.1 |
0.807 |
0.427 |
4.52 ± 0.21 |
0.10 |
23.2 ± 2.7 |
<LOD |
01.04 |
M |
76.1 ± 22.5 |
10.7 ± 1.6 |
200.0 ± 18.3 |
8.3 ± 0.2 |
7.250 |
68.425 |
7.34 ± 0.64 |
0.04 |
34.8 ± 2.8 |
2.17 ± 0.30 |
29.05 |
M |
|
|
91.0 ± 1.7 |
9.6 ± 0.1 |
0.445 |
0.122 |
6.79 ± 0.36 |
0.20 |
20.0 ± 2.7 |
<LOD |
10.07 |
M |
|
|
48.4 ± 1.1 |
9.8 ± 0.1 |
0.345 |
0.254 |
9.07 ± 0.82 |
0.14 |
7.4 ± 3.1 |
<LOD |
Table 3 Concentrations of ligands with corresponding K-s, concentrations of DOC, SAS, dCu, normalized Ltot/DOC values, RSS and protein-like material in ULW samples. LOD-limit of detection,/-not measured
Date |
ULW station |
[L2]/nM |
log K2 |
DOC mg C L−1 |
SAS eq. mg T-X-100 L−1 |
[dCu]/nM |
[Ltot]/DOC μmol mg−1 C−1 |
RSS eq. nM GSH |
Protein material eq. nM BSA |
06.02 |
J |
19.9 ± 1.0 |
9.6 ± 0.2 |
0.821 |
0.184 |
2.92 ± 0.13 |
0.02 |
<LOD |
<LOD |
20.02 |
J |
33.2 ± 0.7 |
9.9 ± 0.1 |
0.809 |
0.155 |
2.85 ± 0.32 |
0.04 |
<LOD |
<LOD |
05.03 |
J |
26.4 ± 0.7 |
10.2 ± 0.2 |
0.790 |
0.031 |
2.88 ± 0.21 |
0.03 |
<LOD |
<LOD |
20.03 |
J |
33.7 ± 1.0 |
9.9 ± 0.1 |
0.847 |
0.119 |
4.75 ± 0.14 |
0.04 |
<LOD |
<LOD |
01.04 |
J |
43.1 ± 2.5 |
9.8 ± 0.1 |
0.971 |
0.398 |
4.10 ± 0.15 |
0.04 |
<LOD |
<LOD |
16.04 |
J |
62.1 ± 2.7 |
9.5 ± 0.1 |
0.965 |
0.190 |
3.97 ± 0.28 |
0.06 |
6.3 ± 3.2 |
<LOD |
29.04 |
J |
25.8 ± 1.1 |
10.0 ± 0.2 |
0.969 |
0.028 |
2.62 ± 0.18 |
0.03 |
<LOD |
<LOD |
15.05 |
J |
26.9 ± 1.0 |
9.8 ± 0.1 |
0.972 |
0.028 |
2.15 ± 0.19 |
0.03 |
<LOD |
<LOD |
29.05 |
J |
31.2 ± 0.8 |
9.6 ± 0.0 |
0.900 |
0.049 |
2.73 ± 0.10 |
0.03 |
<LOD |
<LOD |
12.06 |
J |
36.4 ± 1.2 |
9.6 ± 0.1 |
0.940 |
0.107 |
4.06 ± 0.21 |
0.04 |
<LOD |
<LOD |
27.06 |
J |
37.5 ± 2.1 |
9.8 ± 0.1 |
0.835 |
0.070 |
3.57 ± 0.23 |
0.04 |
<LOD |
<LOD |
10.07 |
J |
35.3 ± 1.9 |
10.0 ± 0.1 |
0.895 |
0.005 |
6.57 ± 0.47 |
0.04 |
<LOD |
<LOD |
06.02 |
M |
— |
— |
0.628 |
0.028 |
— |
— |
— |
— |
01.04 |
M |
60.4 ± 1.8 |
9.7 ± 0.1 |
0.837 |
3.615 |
5.63 ± 0.28 |
0.07 |
6.8 ± 3.6 |
<LOD |
29.05 |
M |
69.6 ± 2.9 |
9.5 ± 0.1 |
0.921 |
0.093 |
3.21 ± 0.14 |
0.08 |
7.6 ± 3.1 |
<LOD |
10.07 |
M |
47.5 ± 1.2 |
9.8 ± 0.0 |
0.945 |
0.109 |
5.44 ± 0.33 |
0.05 |
8.1 ± 3.1 |
<LOD |
3.2. CuCC in the surface seawater of the middle Adriatic Sea
The mathematical transformations of the Cu complexometric curves obtained for the SML and ULW samples in the ProMCC software illustrated the capacity of one (L2) or two classes of organic ligands (L1, L2) to bind Cu2+. The SML contained ligand class L2 (28.4 ± 0.7 to 392.0 ± 8.3 nM) in all analysed samples, while ligand class L1 (40.5 ± 14.6 to 76.1 ± 22.5 nM) was present in only four SML samples; three of those were from Jadrija station (March 5th, April 16th, June 27th, Table 2) and one sample from Martinska station (April 1st, Table 2). In the ULW samples, only ligands of class L2 were present, in the range 19.9 ± 1.0–69.6 ± 2.8 nM (Table 3). For the SML samples, log
K1 values were 10.3 ± 0.4–11.1 ± 2.8 with an average value of 10.6 ± 1.3 and log
K2 values were 8.3 ± 0.2–10.0 ± 0.1 with an average value of 9.4 ± 0.1. The log
K2 values for the ULW samples ranged from 9.5 ± 0.1 to 10.2 ± 0.2 with an average value of 9.8 ± 0.1.
Fig. 3 shows the change in total concentration of ligands and Cu, with atmospheric events during the sampling period in the background. Over the entire sampling period from February to July 2019, higher CuCC was detected in the SML than in the ULW samples. The EF for Ltot in the SML ranged from 0.9 to 9.1 with a mean of 2.6 (Fig. 4) and a median of 2.0. The SML was enriched in Ltot at both stations (EF > 1), with the exception of the Jadrija station on February 20th (EF = 0.9). Significant enrichment of organic ligands (EF > 2) was detected in 31% of the SML samples. Enrichment factor for DOC ranged from 0.2 to 8.7 with an average value of 1.8 and a median of 1.0. The samples from April 1st with EF = 9.1 in Jadrija and EF = 4.6 in Martinska stood out probably due to the highest values of [Ltot] and DOC determined in the SML. Sampling on April 1st coincided with episodic atmospheric pollen input as well as nearby open-fire event (Fig. 2B), indicating that the dissolved organic ligands contained in the SML may be of atmospheric origin. A very high enrichment of Ltot (EF = 6.0) and dCu (EF = 8.0) in the SML was also detected on February 6th at the Jadrija station (Fig. 4), but we could not identify any atmospheric event associated with an intense input before the start of our campaign.
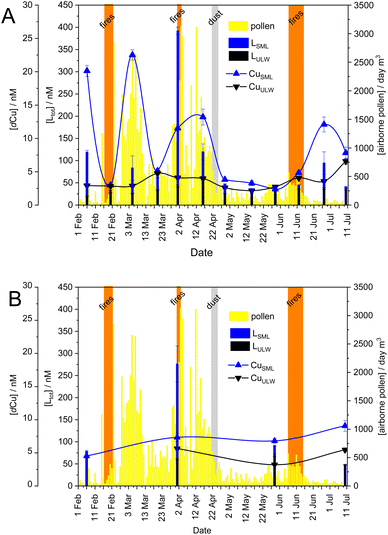 |
| Fig. 3 Concentrations of Ltot and dCu in the SML and ULW samples for Jadrija (A) and Martinska (B) station with marked specific atmospheric events: orange – open-fire BB, yellow – airborne pollen, grey – Saharan dust. | |
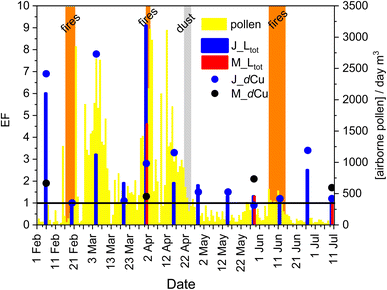 |
| Fig. 4 Enrichment factor (EF) for Ltot and dCu concentrations at Jadrija (J) and Martinska (M) stations in the period February–July 2019. The black horizontal line indicates EF = 1, specific atmospheric events are: orange – open-fire BB, yellow – airborne pollen concentration, grey – Saharan dust. | |
3.2.1. Cu-binding organic ligands.
Since the coastal SML represents a very specific environment in terms of specific phytoplankton, photochemical processes influenced by direct solar radiation and anthropogenic impacts, the structure of Cu-binding organic ligands found there could be very specific. Being focused on the certain atmospheric events and the air–sea interactions followed during our campaign by comparing the SML and the corresponding ULW samples, we analysed protein-like material as allochthonous (atmospheric) Cu-binding ligands connected to the intensive pollen deposition, and RSS as both autochthonous (biological) ligands and allochthonous (atmospheric) ligands in some samples. In a dissolved fraction of the SML samples from April at both stations, protein-like material was quantified by the detection of peak H applying the CPS method (Table 2). The concentration in Jadrija SML samples was eq. 0.40 ± 0.31 nM BSA on April 1st and eq. 1.63 ± 0.56 nM BSA on April 16th, while at Martinska station, the concentration was eq. 2.17 ± 0.30 nM BSA on April 1st. Chronopotentiograms and accompanying BSA standard additions to the SML sample from April 16th are shown in Fig. S4.† In addition, CuCC parameters for 1 mg L−1 BSA, a model protein, in UV-irradiated seawater electrolyte were determined from the complexometric titration curve data (Fig. S5†). BSA bound Cu2+ ions at a concentration of 152.0 ± 3.1 nM and formed complexes with log
K = 9.29 ± 0.56. This is comparable to the concentrations of the ligand class L2 and the log
K2 values determined in the SML, justifying BSA as a good model for protein-like ligands.
RSS concentrations at both stations ranged from eq. 5.9 ± 3.2–76.5 ± 6.5 nM GSH and accounted for 6–30% of the ligands in the samples. The highest RSS concentrations were found in the SML of Jadrija (eq. 76.5 ± 6.5 nM GSH) and Martinska (eq. 34.8 ± 2.8 nM GSH) on April 1st, while their concentration was lower in the corresponding ULW samples, indicating possible atmospheric impact via deposition of various aerosols such as pollen and/or BB in the SML. Moreover, RSS were detected in all SML and ULW samples at Martinska station situated in the Šibenik Bay (in Krka River estuary; Tables 2 and 3), but at higher levels than previously reported for the water column in the upper reach of the Krka River,56 possibly due to atmospheric impact.
Normalization of Ltot concentrations to the DOC values provides information on the amount of Cu-binding organic ligands per unit mass of organic carbon, [Ltot]/DOC (μmol mg−1 C−1).50 The concentrations of Ltot were not found in a constant ratio in the DOC (Tables 2 and 3). The amount of organic ligands by mass unit of DOC ranged from 0.04 to 0.20 μmol mg−1 C−1 with an average value of 0.09 μmol mg−1 C−1 in the SML samples, while the values for the ULW varied to a lesser extent, between 0.02–0.08 μmol mg−1 C−1 with an average value of 0.04 μmol mg−1 C−1. These results indicate a higher number of Cu-binding organic ligands per organic C mass unit in the SML, showing that SML is a specific environment where allochthonous and autochthonous organic ligands accumulate.
To our knowledge, the electrochemical study of Cu-binding organic ligands in the SML has only been reported for a few marine areas. In Table 4, we compared obtained Middle Adriatic CuCC parameters, EF-s, and Ltot/DOC ratios for the SML and corresponding ULW with those published for the Mediterranean Sea,49,50,57 the Norwegian fjords,48 and the Yellow Sea,58 taking into account their specific environment and trophic conditions. The range of Ltot concentrations found for the SML in the middle Adriatic Sea is comparable to the values reported for the oligotrophic E Mediterranean Sea, but is lower than the Ltot concentrations in the SML in the mesotrophic NW Mediterranean Sea, which is considered the second most productive part of the Mediterranean area due to nutrient supply from the Rhone and Ebro rivers.59 The biological molecules and products formed during the rapid transformation of fresh organic matter are the predominant Cu-binding ligands in the SML of the E Mediterranean,50,57 while there may be autochthonous as well as additional sources of ligands such as atmospheric inputs in the SML of the NW Mediterranean.49 The average values for Ltot concentrations in the ULW of the Adriatic Sea were up to five times lower than those in the E and NW Mediterranean, confirming the low autochthonous production of ligands. The highest Ltot concentrations were determined in the SML in subarctic Norwegian fjords, characterized by local remineralisation of sediments,60 where ULW was identified as the only source of Cu-binding ligands that eventually reach the SML.48 In the eutrophic Jiaozhou Bay, where river and sewage discharges stimulate primary production61 and the influence of wet atmospheric deposition is negligible,62 higher average Ltot concentrations were identified than in the oligotrophic Adriatic and E Mediterranean. Stability constants were higher in the middle Adriatic area, with stronger L1 class ligands (log
K1 > 10) detected for the first time in certain SML samples under the influence of specific atmospheric events. In all the areas compared, an average EF > 1 was determined for Ltot, suggesting that SML was enriched with Cu-binding organic ligands compared to ULW. While in the NW and E Mediterranean the average EF was 1.2, in the middle Adriatic Sea it was higher, 2.6, due to significant atmospheric input of ligands in the SML. However, this value is comparable to the EF in the fjords. The density of Cu-binding organic ligands per organic C mass unit was very similar in the Adriatic and Mediterranean Sea, while values up to three times higher were calculated for the SML and ULW of the Norwegian fjords, suggesting more specific functional groups for Cu binding.
Table 4 Comparison of the middle Adriatic Sea CuCC with the values for the SML and ULW of E and NW Mediterranean, subarctic Norwegian fjords and Jiaozhou Bay (Yellow Sea, North China)
Coastal sea |
|
[Ltot]/nM |
EFLtot |
log K |
L
tot/DOC μmol mg−1 C−1 |
This paper.
Karavoltsos et al., 2022.57
Karavoltsos et al., 2015.50
Plavšić et al., 2007.49
Gašparović et al., 2007.48
Chen et al., 2006.58
Average values.
|
Middle Adriatic Seaa |
SML |
28.4–392 |
0.9–9.1 |
8.3–11.1 |
0.04–0.20 |
100.2g |
9.4g |
0.09g |
ULW |
19.9–69.6 |
2.6g |
9.5–10.2 |
0.02–0.08 |
39.3g |
9.8g |
0.04g |
E Mediterraneanb |
SML |
29–201 |
0.8–2.0 |
7.5–8.3 |
|
114g |
8.0g |
ULW |
22–136 |
1.2g |
7.6–8.5 |
93g |
7.9g |
E Mediterraneanc |
SML |
51.5–679.6 |
0.3–2.5 |
6.6–7.9 |
0.03–0.17 |
|
229.6g |
|
55.2–346.4 |
1.2g |
ULW |
208.5g |
NW Mediterraneand |
SML |
280–940 |
1.2g |
|
0.10–0.29 |
ULW |
100–370 |
0.10–0.20 |
Norwegian fjordse |
SML |
230–1790 |
1.3–5.1 |
6.8–7.8 |
0.11–0.64 |
ULW |
90–370 |
2.5g |
0.07–0.24 |
Jiaozhou Bay (Yellow Sea)f |
SML |
385g |
1.56g |
7.21g |
|
ULW |
245g |
3.3. Impact of specific atmospheric aerosols on surface seawater
The SML samples from April 1st at Jadrija and Martinska stations showed the highest Ltot, 392.0 ± 8.3 nM and 276.1 ± 40.8 nM, respectively, followed by Jadrija SML samples with 119.6 ± 18.1 nM (April 16th) and 119.0 ± 4.2 nM (February 6th) (Fig. 3). While such extremely high ligand concentration was found in the SML samples at both stations (Table 2), relatively low values and no major change throughout the seasons was characteristic of Cu-binding ligands in ULW samples (Table 3), pointing to the impact of external processes/events from the atmosphere to the SML.
3.3.1. The biomass burning (BB) events.
Within ∼20 km of the Jadrija and Martinska sampling stations, three intense open-fire BB episodes occurred during February–July 2019 campaign,4,16,20 caused by burning of agricultural waste and pine forests (February 16th–21st, March 31st–April 2nd), and low vegetation and pine and olive tree forests (June 6th–15th). The BB event on March 31st–April 2nd coincided with a high concentration of airborne pollen (Fig. 2B) and a yellow blanket of deposited pollen particles covering the sea surface at both stations. The exact contribution of each of the two events to the Ltot pool in the SML cannot be unambiguously discerned. However, the other two BB events provided an opportunity to further analyse the impact of such events on the Cu chemistry/complexing processes in the SML. The results indicated that atmospheric deposition of BB aerosols in February and June did not show direct effect on the SML chemistry, especially concerning direct input of organic ligands from the air, so we could hypothesize the same for the BB event in April. To clarify the hypothesis of an indirect effect, we analysed the model leachates of the filter-collected simulated BB aerosols for organic matter components (Table 5). The aerosol mass of the simulated BB was chosen in a way that the concentrations of leached water-soluble organic carbon from BB aerosols (7.155 mg C L−1, 7.368 mg C L−1, and 6.326 mg C L−1, respectively) closely matched the DOC values determined in the SML samples from April 1st, 2019 (6.762 mg C L−1 at J station and 7.250 mg C L−1 at M station, Table 2). The leached organic material of the simulated BB aerosols did not show capacity to complex Cu ions, and no specific ligands such as protein-like material or RSS were detected (Table 5). This indicates that BB did not bring these allochthonous ligands into the SML on April 1st.
Table 5 Dissolved organic matter components (water-soluble organic carbon (WSOC), L, proteins and reduced sulphur species (RSS)) and dCu concentrations in model aerosol leachate of simulated BB, Saharan dust (collected in 2021), and pollen solutions
Leachate/solution |
WSOC mg C L−1 |
[dCu]/nM |
[L2]/nM |
log K2 |
Proteins eq. nM BSA |
RSS eq. nM GSH |
Values lower than corresponding limit of detection.
|
Simulated BB |
AH-7 |
7.155 |
16.25 ± 0.6 |
0 |
— |
0 |
0 |
AH-18 |
7.368 |
1.50 ± 0.11 |
0 |
— |
0 |
0 |
AH-20 |
6.326 |
17.8 ± 1.01 |
0 |
— |
0 |
0 |
Pollen |
Cupressus sempervirens
|
7.117 |
13.6 ± 1.78 |
103 ± 2.90 |
9.94 ± 0.10 |
10.6 ± 2.0 |
3.0a |
Juniperus communis
|
6.387 |
61.9 ± 5.9 |
172 ± 2.20 |
9.90 ± 0.02 |
3.8 ± 0.9 |
3.6a |
Pinus nigra
|
7.351 |
57.4 ± 4.75 |
211 ± 4.04 |
9.54 ± 0.07 |
4.6 ± 1.1 |
13.52 ± 2.85 |
Dust |
Saharan dust |
0.910 |
26.8 ± 5.55 |
0 |
— |
0 |
0 |
3.3.2. The pollination period.
Very high airborne pollen concentrations were measured in late March and early April (>1500 day−1 m−3, Fig. 2B) resulting in a yellow blanket of pollen particles on the sea surface at both stations on April 1st at the time of sampling. This event could be classified as an extreme pollen event,36 indicating a possible contribution of pollen's organic material to Cu-binding organic ligand pool in the SML. The highest concentrations of Ltot (392.0 nM at Jadrija station and 276.1 nM at Martinska station), and the corresponding very high DOC values (6.762 mg C L−1 and 7.250 mg C L−1, respectively) were found in the SML samples on April 1st (Table 2 and Fig. 3). Since an open-fire episode that occurred at the same time in proximity (∼1 km) to the sampling sites (Fig. 2B) was not relevant for the obtained extreme results (as evidenced in 3.3.1.), and such a high pollen input was not registered on any other sampling day, deposited pollen appeared to be a major source of the Cu-binding organic ligands in the SML on April 1st. To confirm that, we determined the CuCC parameters and protein-like material in solutions of model pollens characteristic of the middle Adriatic coastal area, namely Cupressus sempervirens, Juniperus communis, and Pinus nigra (Table 5). The concentration of water-soluble organic carbon in the generated model solutions was consistent with the values of DOC detected in the SML samples from April 1st, 2019 (Table 2). Then, we compared CuCC results with the values obtained in the SML samples. The model pollen solutions had a high concentration of ligand class L2 (>100 nM), with log
K2 < 10, which were comparable to the L2 and log
K2 values determined for the SML of the middle Adriatic Sea (Table 2), confirming pollen as a source of Cu-binding organic ligands. The protein-like material was detected in all pollen solutions tested, up to an amount eq. 10.6 ± 2.0 nM BSA, confirming its Cu complexing properties.
RSS were also detected in the Pinus nigra sample, but not in the simulated BB samples (Table 5). It is possible that tree pollen such as Pinus pollen, which accounted for an average of 49.9% of the total pollen concentration in the first half of April 2019 (Fig. S6†), introduced also some RSS into the SML on April 1st. RSS were previously quantified in the water-soluble fraction of fine aerosols collected at the middle Adriatic coast, where two types of non-volatile RSS were confirmed, but were not associated with any specific atmospheric event.63 In addition, sulphur-containing oxygenated organic aerosols comprise up to 19% of the total organic aerosols in this coastal area.64
3.3.3. The Saharan dust intrusion.
Following the Saharan dust event on April 21st–25th, 2019, seawater samples were taken on April 29th and the EF obtained was 1.1 for DOC, indicating a negligible influence of the Saharan dust event on dissolved organic matter in the SML in the middle Adriatic Sea. To check this in more details, we prepared a leachate from the Saharan dust aerosol with a water-soluble organic carbon of 0.910 mg C L−1, which was comparable to the DOC of the SML sample collected on April 29th, 2019, and analysed it for organic matter components. No capacity to bind Cu was obtain in the dust leachate and no specific Cu-binding organic molecules such as RSS or protein-like material were detected (Table 5). A delayed effect, as in the case of the open-fire BB impact, was also not evident in the subsequent samplings. This could be due to our sampling frequency since the next sampling was a few days after the intrusion and every 2 weeks thereafter, so the immediate consequences might simply have been missed. As hypothesised by Penezić et al. (2021),4 the deposited material might have been removed by physical processes and/or promptly processed by microorganisms before our sampling took place. Moreover, the adsorption of organic molecules on the dust mineral particles during their transport in the atmosphere is not typical; in some cases Saharan dust may supply the Mediterranean Sea with DOC, but it has also been shown that high levels of dust could be associated with low values of DOC in seawater.5 Saharan dust generally supplies the Mediterranean Sea with macronutrients65 as well as major and trace metals such as V, Cr, Mn, Fe, Ni, Cu, Zn, Cd and Pb66 or Co,4 which influence phytoplankton production. However, only West African Saharan dust aerosol has been previously identified as a direct source of organic complexing ligands for Cu, Pb, Cd, Ni and Co in seawater.6
4. Discussion
4.1. Biomass burning impacted CuCC in the SML of the coastal sea
Biomass burning emission represents a dominant source of various chemical species67 such as different organic aerosols68,69 and inorganic compounds such as nutrients and trace metals.70 Nevertheless, our results indicated that water-soluble organic molecules from open-fire BB in the middle Adriatic did not directly alter the amount of organic matter components such as Cu-binding organic ligands in the SML. However, high Ltot concentrations (Fig. 3) and a corresponding SML enrichment with EF > 2 (Fig. 4) were determined in subsequent post-fire samplings (March 5th, April 16th, and June 27th), suggesting that BB aerosols may have had an indirect and up to two weeks delayed influence on Ltot concentrations in the SML. According to the other results of the Middle Adriatic campaign in 2019,20 open-fire BB events in February, April and June were accompanied by wet deposition that caused an extensive input of dissolved inorganic nitrogen into the SML and induced phytoplankton growth which resulted with an up to two-week delayed increase in concentrations of DOC, as well as in dissolved lipids and dissolved carbohydrates, autochthonous molecules relevant for the binding of Cu ions.71,72
RSS such as sulphides and thiols have been identified as one of the strongest marine Cu-binding ligands73 that may account for the majority of Cu-binding organic ligands in the photic seawater layer.46 Stronger ligands of the L1 class (log
K1 > 10), usually biologically produced in the upper photic zone,74 were registered only in the SML samples from March 5th, April 16th, and June 27th at Jadrija (Table 2). The RSS detected on March 5th, April 16th, and June 27th in the SML at the Jadrija station (Table 2) overlapped with the detection of these L1 ligands. Therefore, the detected RSS could be part of the L1 ligand class, produced by phytoplankton, possibly purposely as a response to elevated dCu concentrations.30 Indeed, the average dCu concentration was 15.90 nM in the SML from Jadrija collected on February 6th, March 5th, April 1st and 16th, and June 27th, while in the other SML samples it was 4.44 nM dCu, and this is 3.5 times lower. The EF of dCu was also the highest (up to 8.0) on the mentioned dates (Fig. 4). Except for February 6th, those samplings correspond to the post-BB periods when heavy rain events caused deposition maximums and wet atmospheric deposition was the most significant source of anthropogenic Cu for the SML of the coastal Adriatic Sea.4 The concentrations of Cu in PM10 samples increased throughout the intense open-fire BB episodes, especially in winter, and to a lesser extent during spring events, compared to the corresponding seasonal background levels.4 Such a delayed impact of BB aerosols is not unusual because residence time of supplied bioactive trace metals is long enough to allow their chemical and biological utilisation and transformation.75
In the era of global climate change, the Mediterranean region is predicted to experience more frequent droughts in the coming decades,76,77 which will favour extreme vegetation wildfires. The Mediterranean Sea has an exceptionally low biological productivity and is classified as an oligotrophic water body,78 which makes it a very sensitive system79 that can easily respond to various extreme environmental changes/processes, such as episodic heavy nutrient loadings by rivers from the land and/or by wet precipitation from the atmosphere.17,20
4.2. Airborne pollen impacted CuCC in the SML of the coastal sea
Pollen particles are one of the largest bioaerosols with diameters of up to 300 μm and belong to the fraction of coarse aerosols (>2 μm).80,81 Pollen is usually produced by direct emission into the atmosphere and is one of the primary organic bioaerosols.82 Atmospheric transport and deposition of pollen is seasonal and particularly pronounced in spring and summer, when pollen is produced, but less so in autumn and winter.5,37 Pollen of various plants is very rich in organic compounds such as proteins (up to 61%),83–85 which are water-soluble molecules comprising amino acid residues that have a high affinity for binding Cu, thus contributing to CuCC.31 Pollen powder also consists of lipids, carbohydrates, and carotenoids,85 which are also known to be Cu complexing ligands.71,72
Our results confirmed the dominant influence of pollen organic ligands to the CuCC in the SML on April 1st. In addition, a statistically significant strong and positive correlation was found between airborne pollen concentration and DOC (r = 0.767, p = 0.0036) as well as [Ltot] (r = 0.749, p = 0.0051) for the SML samples (N = 12), suggesting not only an extreme but possibly a longer and significant influence of pollen organic material on the trace metal chemistry in the SML. Although a long-term analysis of the meteorological conditions and high airborne pollen concentration in the central Adriatic coastal area revealed a positive correlation between air temperature and airborne pollen concentration and a negative correlation between relative humidity/precipitation and airborne pollen concentration,37 we did not obtain statistically significant correlation during our 5 months long campaign. The reason for this could be the relatively short time in which the field campaign was conducted.
Although different pollen types can be transported over long distances,38,86 based on the amounts of pollen found in the air and on the sea surface of the study area, we assume that the coarse-grained pollen found in the SML of the middle Adriatic originated mostly from cypress and pine trees and were probably transported from the local terrestrial ecosystems into the seawater. A similar situation has already been confirmed for the neighbouring area of the city of Split.37 Low to high pollen concentrations are generally found in the air of the study area throughout the year (Fig. 2B), with cypress and pine pollen being most abundant tree pollen in March and April (Fig. S6,†https://www.plivazdravlje.hr/alergije/kalendar/21/Sibenik.html). Furthermore, air masses from rural areas (such as the middle Adriatic coast) have higher concentrations of larger biological particles such as pollen grains than air masses from urban and industrial areas,80 which supports our assumption of a local origin of the pollen found in the SML.
4.3. Strengthens and weaknesses of research work
In the present study, we have tackled an interdisciplinary and complex topic of air-seawater interactions through the specific atmospheric depositions, which affected the Cu-binding organic ligands in seawater in very different ways. These findings are the main strength of our work. However, we have also identified some weaknesses. The relevant weakness is related to the frequency of seawater sampling and the choice of model biomass for the simulation of BB. Sampling every two weeks could miss relevant atmospheric impacts related to the fast response of the seawater system to atmospheric deposition. It is possible that this was one reason why the effect of Saharan dust was not detected, as discussed in Section 3.3.3. The simulated BB from dry oak does not exactly match the mixed vegetation involved in the open-fire events in 2019, and also, the aerosols of the simulated BB were not exposed to ambient air and therefore did not undergo secondary ageing processes. However, since both the real and the simulated BB originate from vegetation, we can consider that our model experiment with simulated BB supported the interpretation of the field results in a relevant way. We are aware, however, that further work should be initiated to clarify the different or similar effects of both primary and secondary aerosols released by the burning of different biomasses in coastal areas on the chemistry of the surface seawater layers. These weaknesses will serve as guidelines for the improvement of further fieldwork design and research activities within the given topic.
5. Conclusions
During the field campaign of the BiREADI project in the period February–July 2019 at Jadrija and Martinska stations situated in the coastal zone of the middle Adriatic Sea, we studied the temporal changes in CuCC parameters in the SML and ULW samples influenced by the specific effects of airborne pollen, open-fire BB aerosols, and Saharan dust intrusion. While no atmospheric impacts were evident in the ULW samples, the SML samples exhibited the highest Cu-binding ligand concentrations and their enrichment by a factor of up to 9.1 due to responses to the specific atmospheric depositions. The open-fire BBs identified in winter and spring of 2019 did not supply SML with Cu-binding organic ligands, as confirmed by analysis of leachate from the simulated BB aerosols, which showed no capacity to complex Cu ions. The open-fire BB inputs showed an indirect effect on CuCC via wet deposition by introducing fire-born dissolved inorganic nutrients and trace metals into the SML via wet deposition. Consequently, fertilised primary production was accompanied by the production of various Cu-binding organic ligands, which was reflected in increased CuCC up to two weeks after the intrusion. The higher dCu concentrations introduced by wet depositions of BB aerosols in the SML were accompanied by the biological production of specific stronger L1 organic ligands such as RSS. Ligand class L2, typically thought to be the major Cu-buffering substance, was quantified in all SML and ULW samples. An extreme pollen input was recorded during the sampling on April 1st at both stations, accompanied by a direct increase of allochthonous Cu-binding ligands in the SML (Fig. 3). Thus, the organic molecules of pollen, especially the protein-like ones, proved to be very good complexing agents for Cu ions, as confirmed by analysis of leachates of model pollen (Table 5). With respect to pollen and BBs, Saharan dust intrusion in spring showed no obvious effects on the Cu-binding organic ligands in the SML sample taken four days after its intrusion (Fig. 3 and 4). The analysis of the selected leachate of the Saharan dust aerosols agrees with this observation (Table 5). However, further studies should focus on the analysis of wet and dry deposition samples associated with specific atmospheric events to assess their exact contribution to Cu-binding capacity in seawater.
This work represents a first electrochemical study of terrestrial sources such as pollination and BB as very important external forces that could significantly alter, directly and indirectly, the chemical speciation of Cu ions in the SML of the Adriatic Sea and all other oligotrophic coastal areas of the Mediterranean Sea. The study of extreme events and the evaluation of their impact on the biogeochemical processes in seawater is very important for future monitoring of Cu chemical speciation in coastal marine areas, as atmospheric pressure is expected to intensify in the future. Moreover, studies on Cu speciation in seawater are also important considering future predictions of an environment overloaded with elevated Cu concentrations of anthropogenic origin and the (in)ability of marine systems to cope with it, as the global demand for Cu has almost doubled between 2000 and 2020.87
Author contributions
S. Strmečki: conceptualization, investigation, supervision, formal analysis, validation, visualization, writing – original draft, writing – review & editing; I. Dešpoja: investigation, formal analysis, visualization, writing – review & editing; A. Penezić: investigation, formal analysis, validation, visualization, writing – review & editing; A. Milinković: investigation, formal analysis, writing – review & editing; S. Bakija Alempijević: investigation, formal analysis, writing – review & editing; G. Kiss: investigation, writing – review & editing; A. Hoffer: investigation, writing – review & editing; B. Mitić: investigation, formal analysis, writing – review & editing; D. Hruševar: investigation, formal analysis, writing – review & editing; S. Frka: project administration, funding acquisition, resources, writing – review & editing.
Conflicts of interest
There are no conflicts to declare.
Acknowledgements
We thank Dr D. Omanović for constructing the sea surface microlayer sampler and providing it for this study, and for help in using the ProMCC. We thank Z. Zovko and Dr J. Dautović for DOC measurements, N. Vuletin from the Institute of Public Health of Šibenik - Knin County for providing the airborne pollen concentrations, and T. Bulat for help during the field campaign. We also thank Dr B. Gašparović for critical reading of the manuscript. This research was funded by the BiREADI project (IP-2018-01-3105: Biochemical responses of oligotrophic Adriatic surface ecosystems to atmospheric deposition inputs) of the Croatian Science Foundation.
References
- T. J. Yang, Y. Chen, S. Q. Zhou and H. W. Li, Impacts of Aerosol Copper on Marine Phytoplankton: A Review, Atmosphere, 2019, 10, 414 CrossRef CAS.
- T. Jickells, Atmospheric Inputs of Metals and Nutrients to the Oceans - Their Magnitude and Effects, Mar. Chem., 1995, 48, 199–214 CrossRef CAS.
- A. R. Baker, K. Weston, S. D. Kelly, M. Voss, P. Streu and J. N. Cape, Dry and wet deposition of nutrients from the tropical Atlantic atmosphere: Links to primary productivity and nitrogen fixation, Deep Sea Res. Part I, 2007, 54, 1704–1720 CrossRef CAS.
- A. Penezić, A. Milinković, S. B. Alempijević, S. Žužul and S. Frka, Atmospheric deposition of biologically relevant trace metals in the eastern Adriatic coastal area, Chemosphere, 2021, 283, 131178 CrossRef PubMed.
- Y. Galletti, S. Becagli, A. di Sarra, M. Gonnelli, E. Pulido-Villena, D. M. Sferlazzo, R. Traversi, S. Vestri and C. Santinelli, Atmospheric deposition of organic matter at a remote site in the central Mediterranean Sea: implications for the marine ecosystem, Biogeosciences, 2020, 17, 3669–3684 CrossRef CAS.
- M. Nimmo, G. R. Fones and R. Chester, Atmospheric deposition: A potential source of trace metal organic complexing ligands to the marine environment, Croat. Chem. Acta, 1998, 71, 323–341 CAS.
- K. R. M. Mackey, C. T. Chien, A. F. Post, M. A. Saito and A. Paytan, Rapid and gradual modes of aerosol trace metal dissolution in seawater, Front. Microbiol., 2015, 5, 794 Search PubMed.
- B. M. Angel, S. C. Apte, G. E. Batley and M. Raven, Geochemical factors affecting the solubility of copper in seawater, Environ. Chem., 2021, 18, 1–11 CrossRef CAS.
- N. M. Mahowald, D. S. Hamilton, K. R. M. Mackey, J. K. Moore, A. R. Baker, R. A. Scanza and Y. Zhang, Aerosol trace metal leaching and impacts on marine microorganisms, Nat. Commun., 2018, 9, 2614 CrossRef PubMed.
- A. Quigg, J. R. Reinfelder and N. S. Fisher, Copper uptake kinetics in diverse marine phytoplankton, Limnol. Oceanogr., 2006, 51, 893–899 CrossRef CAS.
- L. Iavorivska, E. W. Boyer and D. R. DeWalle, Atmospheric deposition of organic carbon via precipitation, Atmos. Environ., 2016, 146, 153–163 CrossRef CAS.
- L. Xie, X. L. Gao, Y. L. Liu, B. Yang, X. Q. Lv, J. M. Zhao and Q. G. Xing, Atmospheric dry deposition of water-soluble organic matter: An underestimated carbon source to the coastal waters in North China, Sci. Total Environ., 2022, 818, 151772 CrossRef CAS PubMed.
- K. Djaoudi, F. Van Wambeke, A. Barani, N. Bhairy, S. Chevaillier, K. Desboeufs, S. Nunige, M. Labiadh, T. H. des Tureaux, D. Lefevre, A. Nouara, C. Panagiotopoulos, M. Tedetti and E. Pulido-Villena, Potential bioavailability of organic matter from atmospheric particles to marine heterotrophic bacteria, Biogeosciences, 2020, 17, 6271–6285 CrossRef CAS.
- T. M. Tsagaraki, B. Herut, E. Rahav, I. R. B. Frank, A. Tsiola, M. Tsapakis, A. Giannakourou, A. Gogou, C. Panagiotopoulos, K. Violaki, S. Psarra, A. Lagaria, E. D. Christou, N. Papageorgiou, S. Zervoudaki, M. L. F. de Puelles, N. Nikolioudakis, T. B. Meador, T. Tanaka, M. L. Pedrotti, M. D. Krom and P. Pitta, Atmospheric Deposition Effects on Plankton Communities in the Eastern Mediterranean: A Mesocosm Experimental Approach, Front. Mar. Sci., 2017, 4, 210 CrossRef.
- M. Pflieger and A. Kroflič, Acute toxicity of emerging atmospheric pollutants from wood lignin due to biomass burning, J. Hazard. Mater., 2017, 338, 132–139 CrossRef CAS PubMed.
- I. Jakovljević, I. Šimić, G. Mendas, Z. S. Štrukil, S. Žužul, V. Gluščić, R. Godec, G. Pehnec, I. Bešlić, A. Milinković, S. B. Alempijević, M. Šala, M. Ogrizek and S. Frka, Pollution levels and deposition processes of airborne organic pollutants over the central Adriatic area: Temporal variabilities and source identification, Mar. Pollut. Bull., 2021, 172, 112873 CrossRef PubMed.
- S. Guerzoni, R. Chester, F. Dulac, B. Herut, M. D. Loye-Pilot, C. Measures, C. Migon, E. Molinaroli, C. Moulin, P. Rossini, C. Saydam, A. Soudine and P. Ziveri, The role of atmospheric deposition in the biogeochemistry of the Mediterranean Sea, Prog. Oceanogr., 1999, 44, 147–190 CrossRef.
- N. J. P. Owens, J. N. Galloway and R. A. Duce, Episodic Atmospheric Nitrogen Deposition to Oligotrophic Oceans, Nature, 1992, 357, 397–399 CrossRef CAS.
- M. Cunliffe, R. C. Upstill-Goddard and J. C. Murrell, Microbiology of aquatic surface microlayers, FEMS Microbiol. Rev., 2011, 35, 233–246 CrossRef CAS PubMed.
- A. Milinković, A. Penezić, A. Cvitešić Kušan, V. Gluščić, S. Žužul, S. Skejić, D. Šantić, R. Godec, G. Pehnec, D. Omanović, A. Engel and S. Frka, Variabilities of biochemical properties of the sea surface microlayer: Insights to the atmospheric deposition impacts, Sci. Total Environ., 2022, 838, 156440 CrossRef PubMed.
- Y. Chen, G. P. Yang, Q. Y. Xia and G. W. Wu, Enrichment and characterization of dissolved organic matter in the surface microlayer and subsurface water of the South Yellow Sea, Mar. Chem., 2016, 182, 1–13 CrossRef CAS.
- C. X. Ji, G. P. Yang, Y. Chen and P. Y. Zhang, Distribution, degradation and bioavailability of dissolved organic matter in the East China Sea, Biogeochemistry, 2019, 142, 189–207 CrossRef CAS.
- V. Drozdowska and M. Józefowicz, Spectrophotometric studies of marine surfactants in the southern Baltic Sea, Oceanologia, 2015, 57, 159–167 CrossRef.
- S. Frka, Z. Kozarac and B. Ćosović, Characterization and seasonal variations of surface active substances in the natural sea surface micro-layers of the coastal Middle Adriatic stations, Estuarine, Coastal Shelf Sci., 2009, 85, 555–564 CrossRef CAS.
- J. S. Lopez, L. Lee and K. R. M. Mackey, The Toxicity of Copper to Crocosphaera watsonii and Other Marine Phytoplankton: A Systematic Review, Front. Mar. Sci., 2019, 5, 511 CrossRef.
- T. J. Yang, Y. Chen, S. Q. Zhou, H. W. Li, F. H. Wang and Y. C. Zhu, Solubilities and deposition fluxes of atmospheric Fe and Cu over the Northwest Pacific and its marginal seas, Atmos. Environ., 2020, 239, 117763 CrossRef CAS.
- J. S. Yang, J. Cao, G. L. Xing and H. L. Yuan, Lipid production combined with biosorption and bioaccumulation of cadmium, copper, manganese and zinc by oleaginous microalgae Chlorella minutissima UTEX2341, Bioresour. Technol., 2015, 175, 537–544 CrossRef CAS PubMed.
- R. Nicolau, Y. Louis, D. Omanović, C. Garnier, S. Mounier and I. Pižeta, Study of interactions of concentrated marine dissolved organic matter with copper and zinc by pseudopolarography, Anal. Chim. Acta, 2008, 618, 35–42 CrossRef CAS PubMed.
- H. Whitby, A. M. Posacka, M. T. Maldonado and C. M. G. van den Berg, Copper-binding ligands in the NE Pacific, Mar. Chem., 2018, 204, 36–48 CrossRef CAS.
- M. F. C. Leal, M. T. S. D. Vasconcelos and C. M. G. van den Berg, Copper-induced release
of complexing ligands similar to thiols by Emiliania huxleyi in seawater cultures, Limnol. Oceanogr., 1999, 44, 1750–1762 CrossRef CAS.
- J. I. Lorenzo, M. Nieto-Cid, X. A. Alvarez-Salgado, P. Perez and R. Beiras, Contrasting complexing capacity of dissolved organic matter produced during the onset, development and decay of a simulated bloom of the marine diatom Skeletonema costatum, Mar. Chem., 2007, 103, 61–75 CrossRef CAS.
- S. Karavoltsos, A. Sakellari, S. Strmečki, M. Plavšić, E. Ioannou, V. Roussis, M. Dassenakis and M. Scoullos, Copper Complexing Properties of Exudates and Metabolites of Macroalgae from the Aegean Sea, Chemosphere, 2013, 91, 1590–1595 CrossRef CAS PubMed.
- J. Pađan, S. Marcinek, A. M. Cindrić, C. Santinelli, S. R. Brogi, O. Radakovitch, C. Garnier and D. Omanović, Organic Copper Speciation by Anodic Stripping Voltammetry in Estuarine Waters With High Dissolved Organic Matter, Front. Chem., 2021, 8, 628749 CrossRef PubMed.
- D. Viličić, Specific Oceanological Characteristics of the Croatian Part of the Adriatic, Hrvat. Vode, 2014, 22, 297–314 Search PubMed.
- T. Šegota and A. Filipčić, Köppen’s Classification of Climates and the Problem of Corresponding Croatian Terminology, Geoadria, 2003, 8(1), 17–37 CrossRef.
- P. Carinanos, J. L. Guerrero-Rascado, A. M. Valle, A. Cazorla, G. Titos, I. Foyo-Moreno, L. Alados-Arboledas and C. D. de la Guardia, Assessing pollen extreme events over a Mediterranean site: Role of local surface meteorology, Atmos. Environ., 2022, 272, 118928 CrossRef CAS.
- T. Puljak, M. Mamić, B. Mitić, I. Hrga and D. Hruševar, First aerobiological study in Mediterranean part of Croatia (Dalmatia): pollen spectrum and seasonal dynamics in the air of Split, Aerobiologia, 2016, 32, 709–723 CrossRef.
- A. Vucić, A. Večenaj, I. Hrga, D. Peroš-Pucar, B. Stjepanović, D. Hruševar and B. Mitić, Comparative study of ragweed pollen seasons (2008-2017) in the air of Croatian tourist cities of Zagreb (continental area) and Zadar (Mediterranean area), Aerobiologia, 2019, 35, 765–770 CrossRef.
- A. V. Tomaš, D. Šantić, M. Šolić, S. Skejić, A. Milinković, A. Cvitešić Kušan, B. Gašparović, S. Šestanović and S. Frka, How do open coastal fire episodes’ impact sea surface microlayer neuston communities?, Sci. Total Environ., 2023, 861, 160593 CrossRef PubMed.
- I. Pižeta, S. G. Sander, R. J. M. Hudson, D. Omanović, O. Baars, K. A. Barbeau, K. N. Buck, R. M. Bundy, G. Carrasco, P. L. Croot, C. Garnier, L. J. A. Gerringa, M. Gledhill, K. Hirose, Y. Kondo, L. M. Laglera, J. Nuester, M. J. A. Rijkenberg, S. Takeda, B. S. Twining and M. Wells, Interpretation of complexometric titration data: An intercomparison of methods for estimating models of trace metal complexation by natural organic ligands, Mar. Chem., 2015, 173, 3–24 CrossRef.
- D. Omanović, C. Garnier and I. Pižeta, ProMCC: An all-in-one tool for trace metal complexation studies, Mar. Chem., 2015, 173, 25–39 CrossRef.
- S. Strmečki, M. Plavšić and B. Ćosović, Constant current chronopotentiometric stripping analysis of 'N-catalyst' in sodium chloride solution and seawater, Electroanalysis, 2010, 22, 91–98 CrossRef.
- S. Strmečki, J. Dautović and M. Plavšić, Constant current chronopotentiometric stripping characterisation of organic matter in seawater from the northern Adriatic, Croatia, Environ. Chem., 2014, 11, 158–166 CrossRef.
- S. Strmečki and L. Pereža, Electrochemistry of chitosan
amino-glycan and BSA protein mixture under seawater conditions, J. Electroanal. Chem., 2021, 898, 115630 CrossRef.
- P. J. Superville, I. Pižeta, D. Omanović and G. Billon, Identification and on-line monitoring of reduced sulphur species (RSS) by voltammetry in oxic waters, Talanta, 2013, 112, 55–62 CrossRef CAS PubMed.
- D. G. Tang, K. W. Warnken and P. H. Santschi, Organic complexation of copper in surface waters of Galveston Bay, Limnol. Oceanogr., 2001, 46, 321–330 CrossRef CAS.
- V. Cuculić, N. Cukrov, Z. Kwokal, S. Strmečki and M. Plavšić, Assessing trace metal contamination and organic matter in the brackish lakes as the major source of potable water, Environ. Geochem. Health, 2018, 40, 489–503 CrossRef PubMed.
- B. Gašparović, M. Plavšić, B. Ćosović and A. Saliot, Organic matter characterization in the sea surface microlayers in the subarctic Norwegian fjords region, Mar. Chem., 2007, 105, 1–14 CrossRef.
- M. Plavšić, B. Gašparović and B. Ćosović, Copper complexation and surfactant activity of organic matter in coastal seawater and surface microlayer samples from north Norwegian fjords and NW Mediterranean region, Fresenius Environ. Bull., 2007, 16, 372–378 Search PubMed.
- S. Karavoltsos, E. Kalambokis, A. Sakellari, M. Plavsic, E. Dotsika, P. Karalis, L. Leondiadis, M. Dassenakis and M. Scoullos, Organic matter characterization and copper complexing capacity in the sea surface microlayer of coastal areas of the Eastern Mediterranean, Mar. Chem., 2015, 173, 234–243 CrossRef CAS.
- R. R. Draxler and G. D. Hess, An overview of the HYSPLIT_4 modelling system for trajectories, dispersion and deposition, Aust. Meteorol. Mag., 1998, 47, 295–308 Search PubMed.
- A. F. Stein, R. R. Draxler, G. D. Rolph, B. J. B. Stunder, M. D. Cohen and F. Ngan, NOAA's HYSPLIT Atmospheric Transport and Dispersion Modeling System, Bull. Am. Meteorol. Soc., 2015, 96, 2059–2077 CrossRef.
- M. Plavšić, B. Gašparović, S. Strmečki, V. Vojvodić and N. Tepić, Copper complexing ligands and organic matter characterization in the northern Adriatic Sea, Estuarine, Coastal Shelf Sci., 2009, 85, 299–306 CrossRef.
- Y. Louis, P. Cmuk, D. Omanović, C. Garnier, V. Lenoble, S. Mounier and I. Pižeta, Speciation of trace metals in natural waters: The influence of an adsorbed layer of natural organic matter (NOM) on voltammetric behaviour of copper, Anal. Chim. Acta, 2008, 606, 37–44 CrossRef CAS PubMed.
- M. Plavšić, D. Krznarić and B. Ćosović, The Electrochemical Processes of Copper in the Presence of Triton X-100, Electroanal, 1994, 6, 469–474 CrossRef.
- S. Strmečki, I. Ciglenečki, M. Gligora Udovič, M. Marguš, E. Bura-Nakić, J. Dautović and M. Plavšić, Voltammetric Study of Organic Matter Components in the Upper Reach of the Krka River, Croatia, Croat. Chem. Acta, 2018, 91, 447–454 CrossRef.
- S. Karavoltsos, A. Sakellari, M. Plavšić, G. Bekiaris, D. Tagkouli, A. Triantafyllidis, A. Giannakourou, S. Zervoudaki, I. Gkikopoulos and N. Kalogeropoulos, Metal complexation, FT-IR characterization, and plankton abundance in the marine surface microlayer of coastal areas in the Eastern Mediterranean, Front. Mar. Sci., 2022, 9, 932446 CrossRef.
- X. R. Chen, H. D. Gong and C. Y. Liu, Copper complexing capacities of seawater in surface microlayer in Jiaozhou Bay, Environ. Sci., 2006, 27, 885–889 CAS.
- M. Estrada, Primary production in the northwestern Mediterranean, Sci. Mar., 1996, 60, 55–64 Search PubMed.
- P. Wassmann, M. Reigstad, S. Oygarden and F. Rey, Seasonal variation in hydrography, nutrients, and suspended biomass in a subarctic fjord: applying hydrographic features and biological markers to trace water masses and circulation
significant for phytoplankton production, Sarsia, 2000, 85, 237–249 CrossRef.
- S. M. Liu, J. Zhang, H. T. Chen and G. S. Zhang, Factors influencing nutrient dynamics in the eutrophic Jiaozhou Bay, North China, Prog. Oceanogr., 2005, 66, 66–85 CrossRef.
- L. Zou, H. T. Chen and J. Zhang, Experimental examination of the effects of atmospheric wet deposition on primary production in the Yellow Sea, J. Exp. Mar. Biol. Ecol., 2000, 249, 111–121 CrossRef CAS PubMed.
- A. Cvitešić Kušan, S. Frka and I. Ciglenečki, Electrochemical Evidence of non-Volatile Reduced Sulfur Species in Water-Soluble Fraction of Fine Marine Aerosols, Atmosphere, 2019, 10, 674 CrossRef.
- R. Casotto, A. C. Kušan, D. Bhattu, T. Cui, M. I. Manousakas, S. Frka, A. Kroflič, I. Grgić, I. Ciglenečki, U. Baltensperger, J. G. Slowik, K. R. Daellenbach and A. S. H. Prevot, Chemical composition and sources of organic aerosol on the Adriatic coast in Croatia, Atmos. Environ.: X, 2022, 13, 100159 CAS.
- R. Gallisai, F. Peters, G. Volpe, S. Basart and J. M. Baldasano, Saharan Dust Deposition May Affect Phytoplankton Growth in the Mediterranean Sea at Ecological Time Scales, PLoS One, 2014, 9, e110762 CrossRef PubMed.
- C. Theodosi, Z. Markaki and N. Mihalopoulos, Iron speciation, solubility and temporal variability in wet and dry deposition in the Eastern Mediterranean, Mar. Chem., 2010, 120, 100–107 CrossRef CAS.
- M. O. Andreae, Emission of trace gases and aerosols from biomass burning - an updated assessment, Atmos. Chem. Phys., 2019, 19, 8523–8546 CrossRef CAS.
- L. A. Garofalo, M. A. Pothier, E. J. T. Levin, T. Campos, S. M. Kreidenweis and D. K. Farmer, Emission and Evolution of Submicron Organic Aerosol in Smoke from Wildfires in the Western United States, ACS Earth Space Chem., 2019, 3, 1237–1247 CrossRef CAS.
- P. Rajput and M. M. Sarin, Polar and non-polar organic aerosols from large-scale agricultural-waste burning emissions in Northern India: Implications to organic mass-to-organic carbon ratio, Chemosphere, 2014, 103, 74–79 CrossRef CAS PubMed.
- D. S. Hamilton, M. M. G. Perron, T. C. Bond, A. R. Bowie, R. R. Buchholz, C. Guieu, A. Ito, W. Maenhaut, S. Myriokefalitakis, N. Olgun, S. D. Rathod, K. Schepanski, A. Tagliabue, R. Wagner and N. M. Mahowald, Earth, Wind, Fire, and Pollution: Aerosol Nutrient Sources and Impacts on Ocean Biogeochemistry, Ann. Rev. Mar. Sci., 2022, 14, 303–330 CrossRef PubMed.
- M. Mlakar, V. Cuculić, S. Frka and B. Gašparović, Copper-phospholipid interaction at cell membrane model hydrophobic surfaces, Bioelectrochemistry, 2018, 120, 10–17 CrossRef CAS PubMed.
- M. Plavšić and S. Strmečki, Carbohydrate polymers as constituents of exopolymer substances in seawater, their complexing properties towards copper ions, surface and catalytic activity determined by electrochemical methods, Carbohydr. Polym., 2016, 135, 48–56 CrossRef PubMed.
- L. M. Laglera and C. M. G. van den Berg, Copper complexation by thiol compounds in estuarine waters, Mar. Chem., 2003, 82, 71–89 CrossRef CAS.
- H. Whitby, J. T. Hollibaugh and C. M. G. van den Berg, Chemical Speciation of Copper in a Salt Marsh Estuary and Bioavailability to Thaumarchaeota, Front. Mar. Sci., 2017, 4, 178 CrossRef.
- A. M. Ebling and W. M. Landing, Trace elements in the sea surface microlayer: rapid responses to changes in aerosol deposition, Elem. Sci. Anth., 2017, 5, 42 CrossRef.
- B. I. Cook, J. E. Smerdon, R. Seager and S. Coats, Global warming and 21st century drying, Clim. Dyn., 2014, 43, 2607–2627 CrossRef.
- R. Seager, T. J. Osborn, Y. Kushnir, I. R. Simpson, J. Nakamura and H. B. Liu, Climate Variability and Change of Mediterranean-Type
Climates, J. Clim., 2019, 32, 2887–2915 Search PubMed.
- H. R. Powley, M. D. Krom and P. Van Cappellen, Understanding the unique biogeochemistry of the Mediterranean Sea: Insights from a coupled phosphorus and nitrogen model, Global Biogeochem. Cycles, 2017, 31, 1010–1031 CrossRef CAS.
- C. M. Turley, The changing Mediterranean Sea - a sensitive ecosystem?, Prog. Oceanogr., 1999, 44, 387–400 CrossRef.
- S. Matthias-Maser and R. Jaenicke, The size distribution of primary biological aerosol particles with radii >0.2 μm in an urban rural influenced region, Atmos. Res., 1995, 39, 279–286 CrossRef.
- S. Matthias-Maser and R. Jaenicke, A Method to Identify Biological Aerosol-Particles with Radius Greater-Than 0.3-μm for the Determination of Their Size Distribution, J. Aerosol Sci., 1991, 22, S849–S852 CrossRef CAS.
- A. Laskin, J. Laskin and S. A. Nizkorodov, Mass spectrometric approaches for chemical characterisation of atmospheric aerosols: critical review of the most recent advances, Environ. Chem., 2012, 9, 163–189 CrossRef CAS.
- T. H. Roulston, J. H. Cane and S. L. Buchmann, What governs protein content of pollen: Pollinator preferences, pollen-pistil interactions, or phylogeny?, Ecol. Monogr., 2000, 70, 617–643 Search PubMed.
- M. D. de Sa-Otero, S. Armesto-Baztan and E. Diaz-Losada, Analysis of protein content in pollen loads produced in north-west Spain, Grana, 2009, 48, 290–296 CrossRef.
- A. Kenđel and B. Zimmermann, Chemical Analysis of Pollen by FT-Raman and FTIR Spectroscopies, Front. Plant Sci., 2020, 11, 352 CrossRef PubMed.
- P. Carinanos, C. Galan, P. Alcazar and E. Dominguez, Analysis of the particles transported with dust-clouds reaching Cordoba, southwestern Spain, Arch. Environ. Contam. Toxicol., 2004, 46, 141–146 CrossRef CAS PubMed.
- T. Watari, K. Nansai and K. Nakajima, Major metals demand, supply, and environmental impacts to 2100: A critical review, Resour., Conserv. Recycl., 2021, 164, 105107 CrossRef CAS.
|
This journal is © The Royal Society of Chemistry 2024 |