DOI:
10.1039/D4BM00054D
(Review Article)
Biomater. Sci., 2024,
12, 3480-3499
Functionalized extracellular nanovesicles as advanced CRISPR delivery systems
Received
11th January 2024
, Accepted 7th May 2024
First published on 7th May 2024
Abstract
The clustered regularly interspaced short palindromic repeat (CRISPR) system, an emerging tool for genome editing, has garnered significant public interest for its potential in treating genetic diseases. Despite the rapid advancements in CRISPR technology, the progress in developing effective delivery strategies lags, impeding its clinical application. Extracellular nanovesicles (EVs), either in their endogenous forms or with engineered modifications, have emerged as a promising solution for CRISPR delivery. These EVs offer several advantages, including high biocompatibility, biological permeability, negligible immunogenicity, and straightforward production. Herein, we first summarize various types of functional EVs for CRISPR delivery, such as unmodified, modified, engineered virus-like particles (VLPs), and exosome-liposome hybrid vesicles, and examine their distinct intracellular pathways. Then, we outline the cutting-edge techniques for functionalizing extracellular vesicles, involving producer cell engineering, vesicle engineering, and virus-like particle engineering, emphasizing the diverse CRISPR delivery capabilities of these nanovesicles. Lastly, we address the current challenges and propose rational design strategies for their clinical translation, offering future perspectives on the development of functionalized EVs.
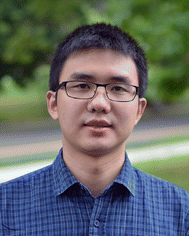 Mingqiang Li | Mingqiang Li is a professor of Molecular Medicine at Sun Yat-sen University. He received his B.S. degree from University of Science and Technology of China in 2009 and obtained his Ph.D. degree under the supervision of Prof. Xuesi Chen from Changchun Institute of Applied Chemistry, Chinese Academy of Sciences, in 2015. From 2015 to 2018, he carried out postdoctoral research with Prof. Kam W. Leong at Columbia University. He serves on the editorial board of Biomaterials, Journal of Tissue Engineering, Chinese Chemical Letters, and Med-X. His current research is mainly focused on biomaterials and nanomedicines. |
1. Introduction
The clustered regularly interspaced short palindromic repeat (CRISPR) genome editing system has significantly advanced the field of gene engineering. Its operation primarily hinges on the single guide RNA (sgRNA), which identifies the target genomic sequences through complementary base pairing, leading to the activation of Cas nucleases.1–3 This activation results in precise cleavage at specific gene loci.4,5 With the ability to precisely cleave and genetically modify the target DNA locus, the CRISPR/Cas system is a promising molecular technology for gene therapy of cancers, infectious diseases, liver diseases, and genetic diseases.6–9
Despite holding significant potential, CRISPR technology necessitates the development of effective delivery systems for CRISPR components.10 To date, a diverse array of nanotechnological tools has been explored for delivering CRISPR systems,11–14 encompassing viral vectors (lentiviral, adenoviral, and adeno-associated virus (AAV) vectors),15 liposomes,16,17 mesoporous silica nanoparticles,18 gold nanoparticles,19,20 and so on. These tools are instrumental in advancing CRISPR applications. Nonetheless, the progression in developing these delivery systems is not keeping pace with the demands of CRISPR technology for clinical use. A major challenge lies in the absence of an optimized carrier that is efficient, targeted, and safe for delivering CRISPR components in vivo.21,22 Such a carrier must address several critical issues: the packaging of the large-sized CRISPR/Cas systems, the complexity of encapsulation processes, precise targeting and accumulation at specific locations, mitigating immunogenicity and toxicity,13 and reducing rapid degradation and clearance in the circulatory system.23–28
Addressing the challenges in CRISPR delivery, functionalized extracellular nanovesicles (EVs) emerge as promising carriers due to their high biocompatibility,29 straightforward production, efficient transfection, low cytotoxicity, negligible immunogenicity, and the ability to penetrate the blood–brain barrier.30 EVs are nanosized lipid bilayer vesicles that cells actively secrete into various biological fluids, including blood, urine, and saliva.31,32 Generally, EVs are categorized into exosomes (30–100 nm) and microvesicles (50–1000 nm) based on their size.33 EVs are known to carry a diverse array of detectable biomarkers, making them highly effective at delivering nucleic acids, proteins, and lipids to target cells. This capability facilitates intercellular communication, coordinates biological functions, and helps maintain homeostasis.34,35 In the realm of therapeutic applications, researchers have explored the use of EVs derived from macrophages and dendritic cells for immunotherapies. These therapeutic EVs are particularly noted for their role in modulating inflammation and immune responses.36 EVs’ ample internal space and specialized cavity structure enable EVs to effectively encapsulate CRISPR/Cas9 systems, simplifying the packaging of large-sized genetic materials. Additionally, the natural lipid bilayer membranes of EVs facilitate delivery, particularly through homologous tropism targeting. The surface proteins on EVs also play a crucial role in mitigating immune responses and reducing clearance from circulation.37,38 Despite these advantages, certain challenges persist in using EVs for CRISPR delivery.39,40 Unmodified EVs from certain cell types may exhibit limited targeting capacity, raising concerns about off-target effects.41 Furthermore, the electrostatic repulsion between the negatively charged EV and cell membrane can impede efficient cellular uptake. Another complication arises when EVs are internalized via endocytosis, as some may be degraded in lysosomes, leading to reduced intracellular delivery efficiency.42
Significant strides are being made by scientists in applying chemical and physical modifications to EVs, aiming to create advanced functional carriers for optimal CRISPR delivery (Fig. 1). Central to this development are key engineering strategies: producer cell engineering, direct vesicle modification, and virus-like particle engineering. These approaches significantly influence the physicochemical properties and biological functions of EVs, including their cellular entry mechanisms. Various EV types, utilizing methods such as membrane fusion, endocytosis, and virus-like infection for CRISPR delivery, benefit from these strategies. The objectives of these engineering efforts are threefold: to extend EV circulation time for effective tissue targeting, to enhance precision in targeting specific tissues or organs, and to improve cellular uptake and lysosomal evasion for efficient delivery into the cytoplasm.43,44 A notable example is the use of common scaffolding proteins from EV membranes (CD63, CD9, Lamp-2b, etc.) in combination with specific functional molecules to increase CRISPR loading efficiency. Additionally, innovative cell membrane engineering techniques in producer cells, such as the transfection of viral vesicular stomatitis virus G (VSV-G) proteins, are being explored to enhance the targeting capability and uptake efficiency of the engineered EVs.45
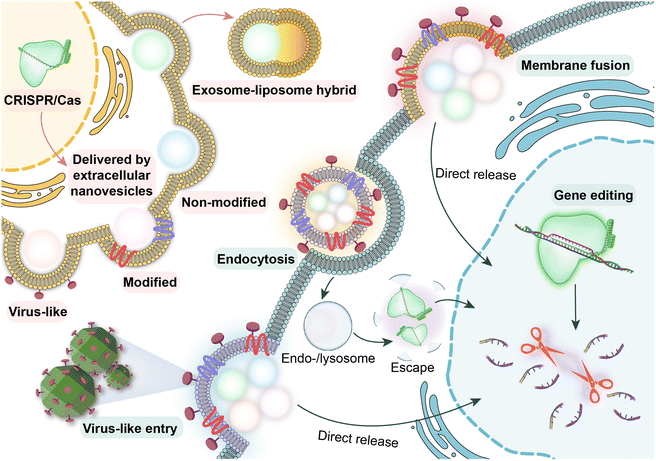 |
| Fig. 1 This review primarily examines four types of functionalized EVs as advanced delivery tools for the CRISPR system in gene therapy. These include natural EVs, modified EVs, exosome-liposome hybrid vesicles, and virus-like EVs, each employing distinct engineering strategies. Additionally, the uptake of these EVs by recipient cells occurs through various mechanisms: membrane fusion, endocytosis, and virus-like infection. | |
In this review, we examine the role of advanced EVs in CRISPR delivery, offering a comprehensive overview of recent advances on the use of CRISPR-loaded EVs for a variety of biomedical applications. We then provide an in-depth analysis of the cellular uptake mechanisms for these EVs, including membrane fusion, endocytosis, and virus-like infection. Additionally, we discuss and emphasize innovative engineering strategies aimed at enhancing the functionality of EVs for more efficient delivery. Finally, we discuss the current challenges and potential strategies for overcoming these obstacles in EV-based CRISPR delivery, aiming to inform the development of next-generation tools with potential for broader application and clinical implementation.
2. EVs-based CRISPR delivery systems
EVs, present in various bodily fluids, are a diverse mix of nanoscale and micrometer-scale membrane vesicles released by a wide range of cells into the extracellular matrix. They play a crucial role in cell communication, migration, tumor growth, and other physiological processes.46 EVs are primarily classified into three categories based on their size and biogenesis: exosomes, microvesicles (MVs), and apoptotic bodies,37,47 which are surrounded by lipid bilayer membranes with the same topological orientation as the plasma membrane.30 Research indicates that EVs play a crucial role in various intercellular signaling pathways, mediating a wide range of physiological and pathological cellular processes through the transport of different biomolecules and facilitating the exchange of intercellular components.48 As a result, EVs are increasingly being investigated as potential therapeutic agents, particularly in cancer treatment.49 Typically, EVs are utilized in two primary contexts: first, as agents of natural reparative processes that help reduce pathological signaling; second, as highly effective delivery carriers that target specific tissues in vivo due to their high biocompatibility, permeability, non-immunogenicity, and low cytotoxicity.49 Consequently, there is growing interest in the development of EV-mediated drug delivery systems.
Owing to their inherent endogenous properties, such as high biocompatibility, permeability, non-immunogenicity, and low cytotoxicity, EVs are emerging as promising nanocarriers for the CRISPR system (Fig. 2).50 Additionally, they provide robust protection to CRISPR agents during circulation, ensuring delivery to target tissues. The widespread presence of EVs in body fluids like blood plasma and saliva, coupled with their large-scale availability and ease of collection, enhances their utility as carriers for the CRISPR system. They can efficiently transport CRISPR components from secreting cells to distant recipient cells through bodily fluids. Furthermore, EVs can enter target cells via endocytosis, virus-like entry, or fusion with the plasma membrane, thereby facilitating the effective transportation of CRISPR components into the cytoplasm.21 Currently, research has identified four main forms of EVs: non-modified extracellular vesicles, modified extracellular vesicles, exosome-liposome hybrid vesicles, and engineered virus-like particles (VLPs) (Fig. 3). For clarity and consistency in this discussion, we use the term “EVs” to collectively refer to all these cell-derived nanoparticles. We will explore the characteristics, advantages, and limitations of these four types of EVs in the context of CRISPR system delivery.
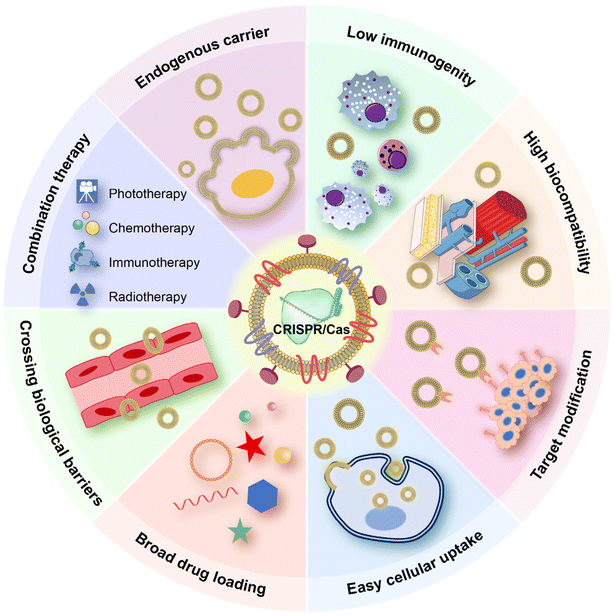 |
| Fig. 2 Illustration of the potential and advantages of functionalized EVs as nanocarriers for the CRISPR gene-editing system. | |
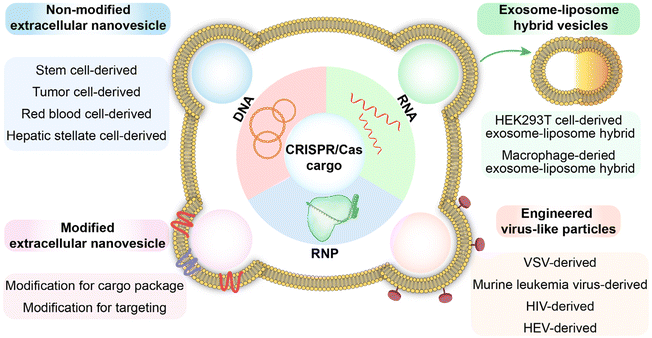 |
| Fig. 3 Overview of four varieties of EVs. This figure concisely summarizes the characteristics of four types of EVs: non-modified EVs, modified EVs, exosome-liposome hybrid vesicles, and engineered VLPs. | |
2.1 Unmodified EVs
Unmodified EVs, also known as natural extracellular vesicles, are produced directly by parental cells without any engineering modifications. These unmodified EVs are capable of transporting cargo with high efficiency, primarily through endocytosis.51 Their high biological compatibility and low immunogenicity, coupled with their natural presence in body fluids, make them particularly suitable for CRISPR delivery applications. Furthermore, research has shown that these EVs often exhibit a propensity for interaction and combination with their parental cells, a phenomenon referred to as “cell tropism”. This property can be advantageous in enhancing targeted interactions, thereby improving the specificity and effectiveness of CRISPR delivery.52
For instance, Usman et al. pioneered the use of red blood cell-derived extracellular vesicles (RBC EVs) for the delivery of Cas9 mRNA and sgRNA, aiming to inhibit microRNA expression in leukemia therapy.53 This approach leverages the abundant presence of RBC EVs in body fluids. In their method, MOLM13 cells were incubated with RBC EVs containing electroporated Cas9 mRNA for 48 hours. The characterization of this process revealed that approximately 50% of the Cas9 proteins were effectively expressed in the nuclei of MOLM13 cells. This led to successful therapeutic gene editing, evidenced by a significant 98% reduction in the expression of miR-125b, an oncogenic microRNA in leukemia cells, in vitro.
Chen et al. leveraged the innate homing properties of EVs, using unmodified endogenous EVs as carriers for delivering Cas9 protein and HBV-specific sgRNA to target HBV-related genes in Huh7 cells.54 Similarly, Kim et al. employed cancer-derived EVs as natural vehicles to transport CRISPR/Cas9 plasmids targeting the poly (ADP-ribose) polymerase-1 (PARP-1) gene.55 Notably, these ovarian tumor-secreted EVs demonstrated cell tropism, selectively accumulating in ovarian tumors in SKOV3 xenograft mice. This led to substantial PARP-1 suppression through effective CRISPR/Cas9 gene editing, inducing apoptosis in ovarian cancer cells. In a more recent study, He et al. reported that cancer-produced EVs showed a cell tropism-dependent targeting efficiency for CRISPR/Cas9 delivery.56 These CRISPR/Cas9-loaded EVs exhibited a higher affinity for adjacent cancer cells in vitro compared to MVs secreted by epithelial cells and selectively accumulated in tumors in HepG2 xenograft mice in vivo. This targeted delivery resulted in efficient proto-oncogene downregulation and enhanced anti-cancer effects, underscoring the potential of unmodified EVs as carriers for the CRISPR/Cas9 system in gene therapy. In another application, Wan et al. used EVs secreted by hepatic stellate cells (LX-2) to deliver Cas9 ribonucleoprotein (RNP) for treating liver disorders.57 By optimizing electroporation, they successfully loaded Cas9 RNP into LX-2 EVs, achieving significant liver-specific genome-editing activity (Fig. 4A). This approach led to indel frequencies of 26.1% and 21.3% in vivo for the treatment of acute liver failure and hepatocellular carcinoma (HCC), respectively, demonstrating the efficacy of EVs as delivery vehicles for CRISPR RNPs in liver disease therapy.57
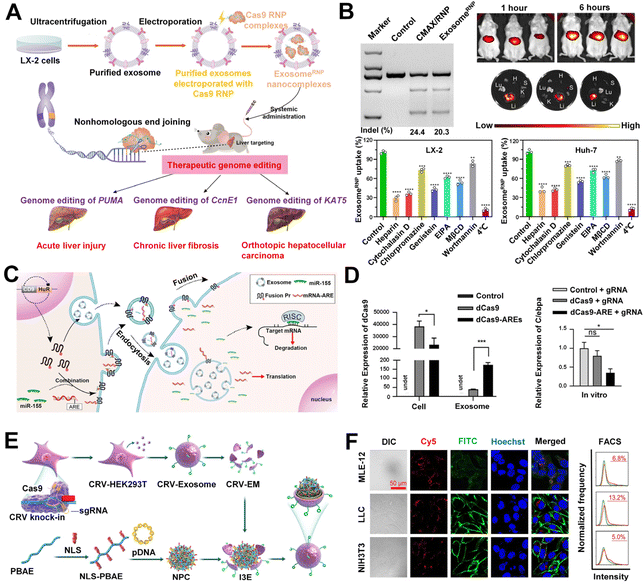 |
| Fig. 4 Unmodified and modified EVs for CRISPR delivery system. (A) Schematic illustration of endogenous EVs for targeting delivery Cas9 RNP for liver-related disease therapy. (B) KAT5 indel mutation frequency was detected by T7E1 experiments. DiR-labeled exosome biodistribution was observed in vivo, and cell uptake of exosomeRNP mechanism for LX-2 and Huh-7 was characterized. Reproduced with permission under a Creative Commons CC BY-NC License.57 Copyright 2022, American Association for the Advancement of Science. (C) A diagrammatic depiction of EVs functionalized with CD9-HuR fusion proteins, which recruit miRNA or dCas9 mRNA. The dCas9 mRNA would be modified with ARE which can combine with HuR. (D) Analysis of dCas9 protein expression and the interference efficiency of dCas9 on C/ebpα expression in the recipient adipogenic stem cells are characterized. Reproduced with permission.62 Copyright 2019, American Chemical Society. (E) Construction of M2 TAMs-targeting nanovesicles for CRISPR system delivery. (F) Confocal microscopy images display uptake of I3E in different cell lines (MLE-12, LLC, NIH 3T3), with plasmids labeled by Cy5, demonstrating the specific targeting capacity of CRV. FACS data includes a green curve representing untreated cells and a red curve for Cy5-positive cells. Reproduced with permission.70 Copyright 2023, Wiley-VCH. | |
2.2 Modified EVs
The development of modified EVs for CRISPR delivery has accelerated recently, focusing on enhancing encapsulation efficiency and targeting specificity. Researchers have identified functionalized EVs as an effective method to improve the loading of CRISPR components.58 Endogenous loading techniques allow for the integration of various sgRNAs and Cas proteins into EVs, facilitating the mass production of diverse, function-specific EVs tailored for targeted tissue gene editing.22 However, physical exogenous loading methods like sonication, passive loading, and electroporation may compromise the structural integrity and properties of EVs. As a result, gene engineering and chemical modification of EVs are preferred to enhance packaging efficiency. For instance, Wang et al. utilized arrestin domain-containing protein 1 (ARRDC1)-mediated microvesicles (ARMMs) as a platform for high-performance intracellular delivery of the CRISPR/Cas9 RNP complex, leveraging the interaction between ARRDC1 and the WW domain, which is rich in proline and short linear peptide motifs.59 The PPXY motifs of ARRDC1 specifically bind to the WW domain of the neural precursor cell-expressed developmentally downregulated gene 4 (NEDD4) family proteins, facilitating the recruitment of Cas9 proteins into EVs.
Furthermore, Dooley et al. identified two “scaffold” proteins, PTGFRN and BASP1, that are preferentially sorted into EVs and can efficiently bind to Cas9, enhancing packaging capacity.58 Zhang and colleagues introduced a novel approach by co-encapsulating VSV-G with the CRISPR/Cas9 system to improve cargo activity and packaging capacity.60 Ye et al. improved the encapsulation efficiency of the CRISPR/Cas9 system by integrating GFP and GFP nanobodies with the EV membrane protein CD63 and the Cas9 protein, utilizing the affinity of the GFP-GFP body.61 Recently, Li et al. achieved high RNA loading efficiency in EVs by fusing the EV membrane marker CD63 with HuR, an RNA-binding protein.62 They also discovered that dCas9 mRNA modified with AU-rich elements was readily encapsulated into this EV system, enhancing gene editing efficiency (Fig. 4B).
Additionally, genetic engineering and chemical modifications can endow EVs with novel functions and properties.63,64 One significant challenge in CRISPR technology is the precise targeting of specific loci in vivo. To address this, post-production genetic engineering and chemical modifications are employed to introduce functional ligands and surface proteins, enhancing the transfection potential and targeting ability of the CRISPR system. For instance, Whitely et al. modified the surface of EVs encapsulating Cas9/sgRNA-eGFP with VSV-G, achieving a 42% efficiency in eGFP knockout with minimal off-target effects in HEK293T cells.23 The addition of VSV-G notably improved transfection efficiency, offering insights into targeted delivery optimization. Previous research has demonstrated the feasibility of engineering EVs with specific ligands for targeted delivery, such as using galactose or glycoconjugates to target the asialoglycoprotein receptor.65 Xu et al. utilized a chimeric-antigen receptor (CAR) to enhance the selective tropism of EVs derived from epithelial cells, creating a safer transport platform for the CRISPR/Cas9 system.66 CAR-EVs exhibited stronger transfection capabilities in tumor cells compared to normal epithelial EVs and demonstrated superior performance in targeting the MYC oncogene both in vitro and in vivo. Zhang and colleagues recently synthesized valency-controlled tetrahedral DNA nanostructures with DNA aptamers, modifying EV surface proteins through cholesterol anchoring.67 This modification significantly enhanced the targeting potential of the EVs, showing a pronounced anti-tumor effect on hepatocellular cancer by downregulating Wnt Family Member 10B protein expression. Furthermore, Ohno et al. developed various EVs modified with GE11 peptides or epidermal growth factor (EGF) for targeting EGFR-overexpressing tumor cells with high efficiency.68 Gulei and coworkers engineered EVs expressing TNF-α proteins on the surface, secreted by tumor cells transfected with the pDisplay plasmid.69 These modified EVs efficiently targeted the IAP1/2 gene and Caspase 8 molecules for anti-tumor therapy. Jiang's group prepared CRISPRi delivery exosomes with the peptide CRVLRSGSC (CRV) for effective targeting of M2-type tumor-associated macrophages (TAMs).70 The targeting peptide CRV was continuously expressed by integrating the CRV-Lamp2b sequence into HEK293T cells, enabling precise and efficient in situ TAM reprogramming (Fig. 4C).
2.3 Engineered VLPs
VLPs, which are composed of viral proteins but lack viral genetic material, are increasingly recognized as promising carriers for CRISPR/Cas9 delivery. Their lack of a pathogenic viral genome categorizes them alongside EVs, endowing VLPs with the combined benefits of both EVs and viral vectors.71–75 VLPs exploit the natural capabilities of viruses, such as cargo packaging, endosome escape, and the ability to be reprogrammed for targeting various cell types,71 making them a valuable option for CRISPR/Cas system delivery.64,76 Notably, VLPs differ from viral vectors in that they transiently deliver gene editing tools using mRNA or proteins, thereby reducing the risks of off-target effects and genome integration. VLPs have become a focal point in nanocarrier research for gene therapy. Montagna and colleagues successfully employed the fusogenic glycoprotein VSV-G to develop a VLP vehicle that effectively delivers CRISPR/Cas9 RNP. This approach effectively downregulated 50% of EGFP expression in EGFP-expressing HEK293T cells (Fig. 5A).77 Mangeot's group engineered VLPs derived from murine leukemia virus to transport Cas9/sgRNA RNPs, achieving efficient genome editing in various cell lines and primary cells, including human induced pluripotent stem cells, human hematopoietic stem cells, and mouse bone marrow cells.78 Yin et al. encapsulated SpCas9 mRNA within HIV-1 VLPs for treating ocular diseases in vivo.79 More recently, Gee et al. developed nanomembrane-derived extracellular vesicles for the delivery of macromolecular cargo (NanoMEDIC), a novel transient delivery system based on EV engineering (Fig. 5B).31 This system integrates SpCas9 into EVs void of pathogenic viral genomes using the FKBP12 and FRB dimerization system. They further modified the VLP surface with HIV gag and VSV-G proteins to target HEK293T cells for Duchenne muscular dystrophy (DMD) therapy. Similarly, Lee et al. prepared hepatotropic hepatitis E VLPs (HEV-LPs), specifically targeting liver tissues for gene editing.76 These HEV-LPs are formed through self-assembly of the N-terminal truncated second open reading frame of HEV. However, a limitation of VLP-mediated delivery is its inefficiency in specifically targeting tissues. Nikolic et al. discovered that VLP glycoproteins could bind to low-density-lipoprotein receptors, resulting in low specificity.80 This finding highlights the need for more targeted proteins and engineering strategies to enhance the tissue-specific targeting capabilities of VLPs.
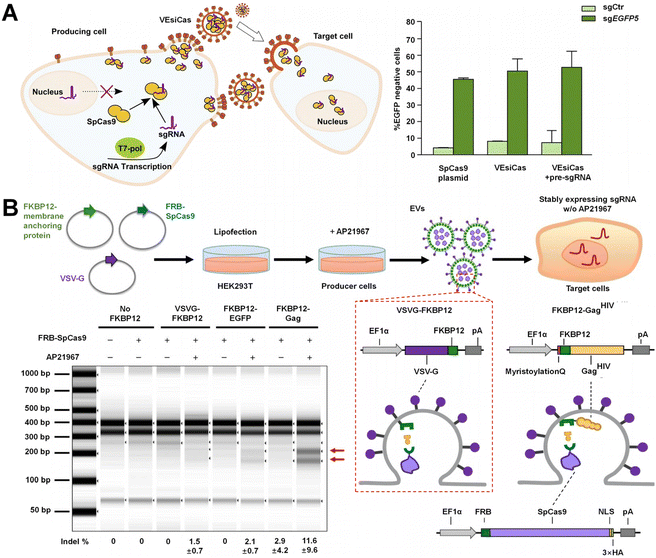 |
| Fig. 5 Utilization of engineered VLPs for CRISPR delivery systems. (A) Synthesis process of VEsiCas, a type of VLP. This process involves the production of EVs by parental cells, modified with VSV-G for targeted tissue specificity. The figure also includes a quantitative analysis of VEsiCas's efficiency in targeting and editing EGFP loci. Reproduced with permission.77 Copyright 2018, Elsevier. (B) Schematic illustration of NanoMEDIC CRISPR delivery platform, encompassing its production by parental cells and subsequent delivery into recipient cells. The figure compares the efficiency of different VLP membrane-anchoring constructs, assessed using T7E1 experiments. Comparing different VLP membrane-anchoring constructs delivery efficiency to DMD1 in the HEK293T cells by T7E1 experiments. Reproduced with permission under a Creative Commons Attribution 4.0 International License.31 Copyright 2020, Springer Nature. | |
2.4 Exosome-liposome hybrid vesicles
Exosome-liposome hybrid vesicles effectively merge the inherent properties of exosomes with the versatility of liposomes,81–83 potentially overcoming the limitations of exosomes in therapy and the drawbacks of liposomes, such as toxicity and prolonged circulation.84 This recent advancement has positioned these hybrid vesicles as a promising option for CRISPR system delivery.85 They offer the controlled size and surface modification flexibility of liposomes,86 along with the natural characteristics of exosomes.81 Recent studies have seen success in creating EV-azide lipids hybrid vesicles in a controlled manner while maintaining the integrity of EVs. These hybrid vesicles, integrated with targeting peptides using copper-free click chemistry, have shown potential for specific target therapy, making them suitable for CRISPR technology applications.87 Rayamajhi and colleagues developed hybrid exosomes combining EVs from mouse macrophages and synthetic liposomes, demonstrating efficient tumor cell targeting in acidic cancer microenvironments and enhanced drug loading capabilities, thereby providing a viable platform for CRISPR delivery.88 Lin et al. encapsulated dCas9/sgRNA complexes in exosome-liposome hybrid vesicles, effectively downregulating the Runx2 gene in mesenchymal stem cells, underscoring the potential of these hybrid vesicles as CRISPR system carriers.89 Moreover, the membrane fusion process in creating these hybrid vesicles does not alter the intrinsic properties of the EVs, such as cell tropism or specific targeting capabilities. In Liang's research, CRISPR/Cas plasmids were transported in chondrocyte-targeting exosomes (CAP-Exo) merged with liposomes to form hybrid CAP-Exos via membrane fusion.90 This approach successfully delivered Cas9 and sgRNA-MMP-13 specifically to chondrocytes, leading to a marked decrease in MMP-13 protein expression and alleviating osteoarthritis symptoms (Fig. 6).
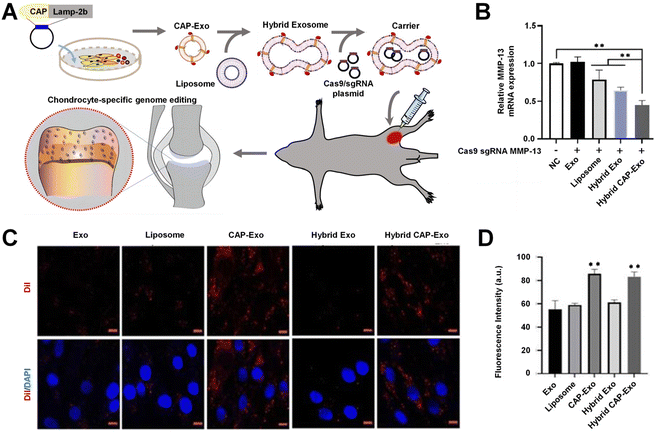 |
| Fig. 6 Exosome-liposome hybrid vesicles for CRISPR delivery system. (A) Schematic illustration of chondrocyte-specific genome editing delivered by hybrid exosomes. The process involves dendritic cells co-incubating with plasmids encoding Lamp2b and a chondrocyte affinity peptide (CAP). These CAP-tagged exosomes undergo membrane fusion with liposomes, resulting in hybrid exosomes that offer increased capacity for CRISPR agent loading. Administered intra-articularly, these hybrid CAP-Exos deliver the Cas9/gRNA complex targeting matrix metalloproteinase 13 (MMP-13) into rat chondrocytes, thereby attenuating cartilage degradation. (B) The graph depicts quantitative real-time PCR (qRT-PCR) results, highlighting changes in MMP-13 mRNA levels in rat chondrocytes following treatment. (C) Confocal microscopy images of EVs, demonstrating the targeting efficacy of CAP-Exo and hybrid CAP-Exo. (D) Quantitative analysis of fluorescence intensity, as measured by ImageJ software. Reproduced with permission.90 Copyright 2022, Ivyspring International Publisher. | |
2.5 Comparison of different EVs
Recent in-depth research and exploration have established EVs as viable vehicles for CRISPR system delivery. These vehicles can be broadly categorized into natural EVs, modified EVs, VLPs, and exosome-liposome hybrid vesicles. Due to the potential safety concerns associated with viral delivery systems, such as high immunogenicity and the risk of viral gene integration,91 non-viral CRISPR delivery systems are gaining prominence. These systems offer significant advantages, including high loading capacity, stability, and biocompatibility, thereby ensuring safe and efficient delivery. Notably, EVs closely resemble biological cell membranes in composition, which reduces obstacles to in vivo applications, making them less susceptible to immune system recognition and clearance. Additionally, innovative functional engineering strategies applied to EVs can further enhance their CRISPR delivery capabilities. Such enhancements include improved targeting ability and delivery efficiency, positioning EVs as highly promising candidates for clinical translation.92
Generally, employing chemical modifications or molecular engineering strategies to enhance the functionalities of natural EV membranes,93 such as in VLPs and hybrid EVs, has been shown to improve CRISPR delivery. This includes enhancements in CRISPR agent loading capacity, intracellular delivery efficiency, and specific targeting ability. However, it is important to note that the use of viral fusogens, coat proteins, and other functional ligands in these processes can potentially lead to immunogenicity.94 A comprehensive overview of these different types of EVs has been provided in the preceding sections. Based on this detailed discussion, their respective advantages and disadvantages have been contrasted and summarized in Table 1. This comparison provides insights into the potential opportunities for further clinical applications of these nanovesicles in CRISPR delivery.
Table 1 Comparison of different EVs
Types of EVs |
Advantages |
Limitations |
Ref. |
Natural EVs |
• High compatibility and biological permeability |
• Low intracellular delivery efficiency |
30, 38 and 40 |
• Low cytotoxicity and immunogenicity |
• Limited cell tropism for off-target risk |
• Easy production |
|
Modified EVs |
• High CRISPR agent's loading efficiency |
• Immunogenicity |
22 and 56 |
• Improving transfection potential and targeting ability |
• Risk of off-target editing |
|
• Complicated and high cost |
VLPs |
• Efficient intracellular delivery |
• Immunogenicity |
73 and 74 |
• Ability to encapsulate cargo and escape endosomes |
• Complicated |
• Low viral genome integration risk |
|
Exosome-liposome hybrid vesicles |
• Keeping EVs intactness and functionality |
• Immunogenicity |
81 and 85 |
• Combining the endogenous nature of exosomes with the flexibility of liposomes |
|
3. Cellular entry pathways of EVs
Given their exceptional delivery capabilities, EVs are increasingly utilized as carriers for CRISPR systems. Understanding the specific interactions between EVs and recipient cells is of paramount importance. This section discusses three primary cellular uptake pathways of EVs: membrane fusion, endocytosis, and viral-mimetic infection. Each pathway's advantages and disadvantages are explored to provide insight into their potential applications.
3.1 Membrane fusion
Membrane fusion, a common physiological process, involves the merging of two separate lipid membranes into a single bilayer.95–97 Typically, upon recognizing recipient cells, EVs initiate signal transduction, leading to membrane fusion, a key interaction method.98 This allows for the discharge of cargoes directly into the cytoplasm of target cells, circumventing lysosomal degradation.99 As a result, the membrane fusion process, which facilitates direct cell–cell contact and immediate CRISPR cargo release into the cytoplasm, demonstrates high delivery efficiency for CRISPR systems.99 This mechanism is also applicable in clinical settings involving CRISPR.
For instance, Tian and colleagues isolated EVs from a human neural progenitor cell line for delivering miRNA drugs with anti-inflammatory properties.100 They engineered a recombinant fusion protein to enhance targeting ability and facilitate membrane fusion. Similarly, Lee et al. explored membrane incorporation between the plasma membrane and EVs secreted by tumor cells (Fig. 7A).101 This approach significantly enhanced the penetration of hydrophobic photosensitizers in both spheroids and in vivo tumors. These findings suggest that EVs derived from cancer cells, equipped with targeting capability and high delivery efficiency, could be an effective vehicle for CRISPR system delivery via a fusion-dependent uptake pathway.
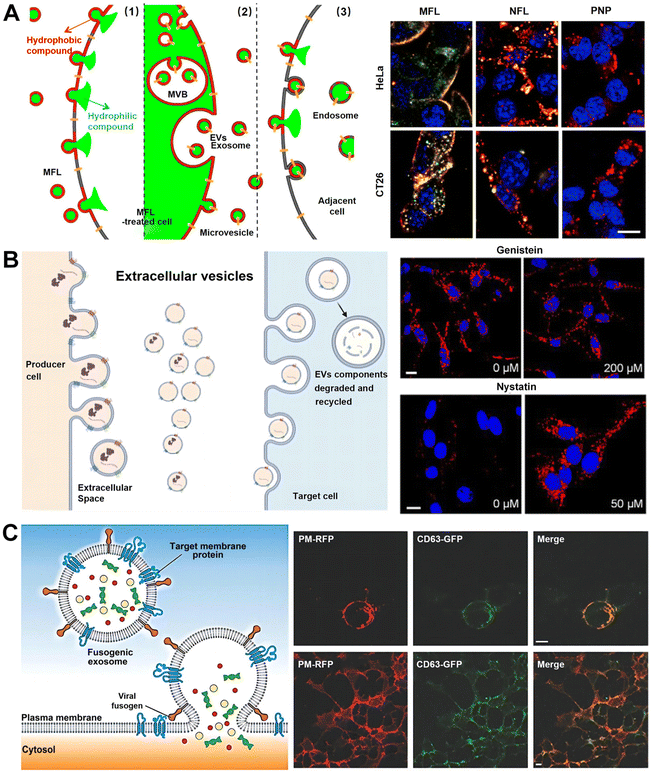 |
| Fig. 7 Cellular entry pathways of EVs. (A) Membrane fusion pathway: EVs are secreted from the tumor to deliver hydrophobic and hydrophilic components via membrane fusion. Confocal microscopy images display the co-localization of fluorescence in HeLa and CT26 cells treated with EVs. Reproduced with permission.101 Copyright 2015, American Chemical Society. (B) Endocytosis pathway: EVs are captured through the endocytosis approach. Confocal microscopy images of PC12 cells pretreated with various concentrations of genistein and nystatin to investigate the principles of endocytosis. Reproduced with permission.104 Copyright 2014, Elsevier. (C) Mimetic infection pathway: modified exosomes with VSV-G can target recipient cells in a virus-mimetic manner. Confocal microscopy images demonstrate this interaction through fluorescence co-localization. Reproduced with permission.45 Copyright 2017, Wiley-VCH. | |
3.2 Endocytosis pathway
Endocytosis, a conventional cellular internalization pathway, is recognized as the predominant route for cell uptake of EVs.22,51,101 This process in the context of EV-mediated CRISPR system delivery typically involves three steps: (1) target ligands on the EV surface bind to receptor proteins on the recipient cell, initiating cellular uptake signals; (2) this binding triggers invagination of the recipient cell membrane, forming endosomes that contain the CRISPR-loaded EVs; (3) subsequently, the CRISPR cargoes are released following successful escape from the endosome/lysosome.102,103
Recent studies have delved into the intricacies of the EV endocytosis process. Costa Verdera et al. investigated EV uptake mechanisms using fluorescent labeling in different cell models, uncovering a link to clathrin-independent endocytosis.51 Tian and colleagues employed high-throughput microscopy to study the uptake of EVs derived from PC12 cells, concluding that uptake occurred through clathrin-mediated endocytosis and micropinocytosis (Fig. 7B).104 Additionally, Svensson's group demonstrated that mammalian cells internalize exosomes primarily through lipid raft-mediated endocytosis, a process inversely regulated by caveolin-1, providing valuable insights into the main cellular entry methods for EVs.105 Heusermann et al. explored the efficiency of EV internalization by recipient cells, identifying endocytosis as the principal internalization pathway.106 Joshi et al. utilized advanced molecular tools and correlative light and electron microscopy to further analyze EV endocytosis and cargo release mechanisms, with results showing EV entry into recipient cells via endocytosis. In summary, endocytosis is a vital pathway for the internalization of target cells and the release of CRISPR cargoes. Nonetheless, effective lysosomal escape, timely release, and precise intracellular localization of the CRISPR system necessitate further modification and engineering of EVs.
3.3 Virus-mimetic infection
Recently, there has been significant progress in the design of VLPs for CRISPR delivery. These functionally advanced extracellular vesicles mimic viral infection mechanisms to enter cells, garnering increased interest in their transfection capabilities. Characterized by their well-defined geometry, stable structure, high efficiency, and specific infection ability, virus-mimetic infection represents a promising method for CRISPR system delivery.107 This approach typically involves viral encapsulation, transport, and the controlled release of nucleic acids into host cells.108 Niu et al. created nanoparticles that imitate the surface topography of enveloped viruses, exhibiting a rough surface similar to a virus interacting with a cell membrane.109 They discovered that the surface roughness and complexity of these nanoparticles enhance biomolecule binding and cellular uptake, significantly increasing delivery efficiency. The high surface energy of the rough surface contributes to strong binding between nanoparticles and cell membrane, thereby improving adhesion to cell surfaces and enhancing delivery efficiency.110 Mangeot et al. developed a novel VLP based on the Friend murine leukemia virus, delivering Cas9/sgRNA RNP to target the EMX1 gene. This VLP showed high transduction efficiency in human-induced pluripotent stem cells, achieving a 67% gene indel rateat the EMX1 locus. Similarly, Yang and colleagues produced exosomes expressing viral fusogen VSV-G proteins on their surface, offering a novel approach for CRISPR delivery (Fig. 7C).45 These EVs enter recipient cells through virus-mimetic infection. Freire and coworkers used a penetrating peptide derived from virus capsid proteins as an siRNA delivery tool to leukemia cells, effectively downregulating Bcr-Abl protooncogene expression.111 Vindry and colleagues utilized murine leukemia VLPs loaded with CRISPR RNPs targeting the Sec-tRNA[Ser]Sec gene in human cell lines, achieving high delivery and genome editing efficiency in various cell lines including HEK293T, HepG2, HaCaT, HAP1, HeLa, and LNCaP.112 Hamilton et al. ingeniously designed a hybrid VLP containing Cas9/sgRNA RNPs targeted to the TRAC gene.113 These Cas9-VLPs, equipped with CD4-tropic HIV-1 envelope glycoprotein, demonstrated a high specific transduction efficiency of 53.2% through a virus-like infection manner. Overall, the aforementioned VLPs exhibit remarkable efficiency in CRISPR delivery, primarily attributed to their virus-like infection entry mechanism. This approach holds significant potential for future clinical applications.
3.4 Comparison of different cellular entry pathways
The efficiency of CRISPR system editing is highly dependent on the method of entry into the target cell. In the membrane fusion pathway, CRISPR cargoes are directly released into the cytoplasm, facilitating immediate gene editing. However, this approach does carry a risk of off-targeting. Virus-like transfection, modified and engineered with targeted peptides and viral fusion factors, typically demonstrates the highest efficiency among these methods. Yet, this approach poses potential risks in generating immunogenicity. Selection of an appropriate EV-mediated route for delivering CRISPR ‘scissors’ to target cells is crucial. Each pathway has its own set of advantages and disadvantages, which are comprehensively compared and summarized in Table 2. This comparison is vital for guiding the design of efficient and safe CRISPR delivery systems for clinical applications.
Table 2 Comparison of different cellular entry pathways
Cellular entry pathways |
Advantages |
Disadvantages |
Ref. |
Membrane fusion |
• Contributes to cell–cell contact and intracellular signal pathway |
• Risk of off-targeting |
96, 97 and 140 |
• Bypasses endosomes to promote CRISPR agent's editing efficiency |
|
|
|
Endocytosis |
• Targeting specificity can be improved through EV functionalization |
• Part of the EVs internalized would be degraded by endosomes, which decreases CRISPR agents’ gene-editing efficiency |
103 and 106 |
Virus-mimetic infection |
• High CRISPR system intracellular delivery efficiency |
• Complicated and cumbersome operations |
108, 141 and 142 |
• Better targeting specificity |
• Risk in generating immunogenicity |
4. Enhancing EVs delivery through engineering techniques
While EVs hold significant promise for delivering CRISPR systems in gene therapy, challenges such as lysosomal degradation, inefficient cytoplasmic delivery, and limited recipient cell uptake impede their clinical applications. One issue is the repulsive interaction between the negatively charged EV membrane and the cell membrane, which can hinder uptake. Furthermore, the natural cell tropism characteristic of EVs does not always meet the requirements for precise and efficient targeting of CRISPR systems in vivo. To overcome these hurdles, advanced engineering strategies and techniques are being developed to enhance the next generation of functional extracellular vesicles. By employing these engineering approaches, both the tissue-specific targeting ability and uptake efficiency of EVs can be significantly improved at the cellular and molecular levels. These enhance the intracellular delivery of CRISPR agents, thereby increasing the overall efficiency of genome editing. This focus on refining EV delivery properties is crucial for realizing the full potential of EV-mediated CRISPR systems in therapeutic applications.
4.1 Producer cell engineering
EVs are naturally secreted by a variety of cell types. Engineering of parental cells to produce multifunctional and modified EVs opens up new possibilities for the clinical application of EV-based CRISPR delivery. The strategy known as “producer cell engineering” involves applying biotechnological or chemical techniques to modify and engineer the surfaces of cells that produce these vesicles. This approach aims to enhance the functionality and targeting capabilities of the EVs they secrete, aligning them more effectively with specific clinical applications and delivery needs of the CRISPR system.
Lee and colleagues have demonstrated the successful engineering of EV producer cells using synthetic membrane fusogenic liposomes (MFLs), which facilitated the functionalization of EVs (Fig. 8A).114 This process, detailed in their research, involves the integration of lipophilic cargo and membrane proteins from MFLs into the plasma membrane of parental cells. Simultaneously, hydrophilic agents are delivered into the cytosol. The EVs secreted by these engineered cells not only retain their original membrane protein functions but also acquire the properties of the lipophilic cargoes, such as specific targeting and signal transduction. This membrane fusion approach emerges as an effective method for engineering parental cells to produce functionally enhanced EVs while preserving their inherent membrane characteristics. Confocal microscopy observations revealed that both lipophilic and hydrophilic compounds could be co-packaged into the secreted EVs more efficiently via MFL formation (Fig. 8A). This indicates that liposome-based cellular engineering technology can generate versatile EVs, tailored for specific surface functionalizations, to optimize the delivery of therapeutic CRISPR systems.114
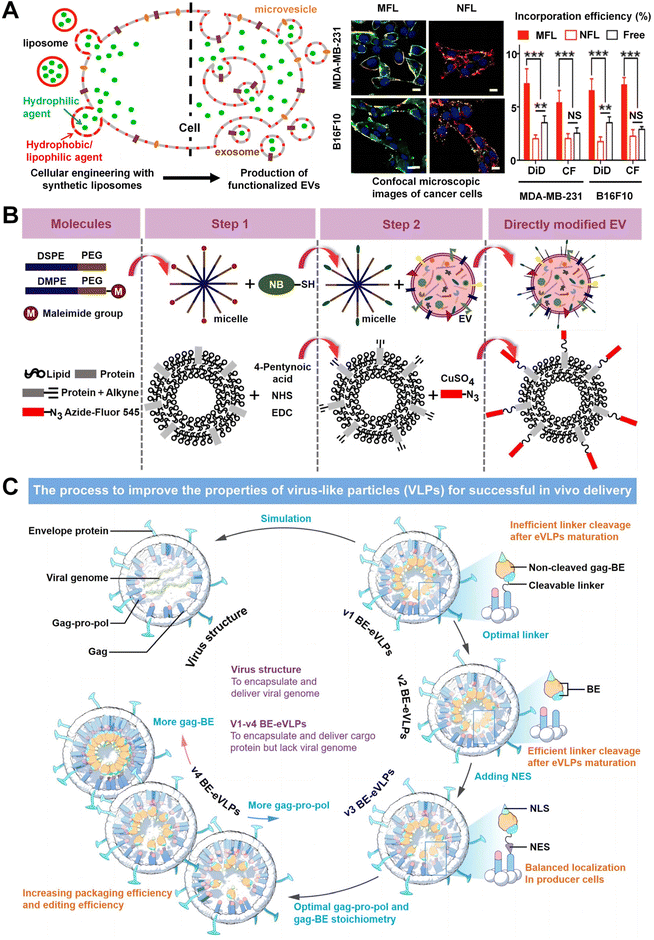 |
| Fig. 8 Engineered techniques to enhance EVs delivery properties. (A) Producing cell engineering: this panel illustrates a strategy for EV functionalization, achieved by engineering parent cells with MFLs that carry both hydrophilic and lipophilic functional agents. Confocal microscopy images substantiate the enhanced co-packaging efficiency of these compounds into secreted EVs. Reproduced with permission.114 Copyright 2016, American Chemical Society. (B) Extracellular vesicle engineering: this schematic depicts the modification of extracellular vesicles using lipid-PEG micelles incorporated with nanobodies (NB) post-insertion. It also illustrates the use of click chemistry technology for EV engineering, exemplified by the conjugation of azide-fluor 545. Reproduced with permission.117 Copyright 2016, Elsevier. (C) Virus-like engineering: this section describes the development of four generations of BE-eVLPs. The engineering process involved optimizing a cleavable linker, the localization of the gag–BE fusion, and the stoichiometry of gag–BE:gag-pro-pol. Reproduced with permission.139 Copyright 2022, Springer Nature. | |
Utilizing genetic engineering to modify EV-producing cells is another effective method for functionalizing EVs. Building upon earlier research that co-transfected plasmids encoding CD63-GFP and VSV-G into HEK293T cells to produce EVs targeting acidic environments, Yang and colleagues developed fusogenic exosomes equipped with VSV-G and GLUT4 proteins. GLUT4 is instrumental in glucose transport into cells, and its overexpression in HEK293T cells targeted these exosomes to C2C12 cells.45 Through characterization and analysis, it was shown that the fusogenic exosomes secreted from HEK293T cells incorporated GLUT4, enabling their transfer into the target cell membrane. This process of “membrane editing” in parental cells effectively generated functional EVs. In acidic environments, VSV-G expression facilitated the fusion of C2C12 cell plasma membranes with the modified exosomes, significantly enhancing the glucose uptake capacity of C2C12 cells.
In pursuit of muscle and brain tissue-specific, non-immunogenic RNA delivery, Alvarez-Erviti et al. employed a producer cell engineering approach.115 They transfected dendritic cells with plasmids encoding targeting peptides like rabies viral glycoprotein (RVG) peptide, muscle-specific peptide (MSP), and FLAG epitope. Additionally, these cells were engineered to express Lamp-2b proteins, which bind to the neuron-specific RVG peptide, ensuring targeted delivery. This strategy of engineering parental cells showcases its suitability for the delivery of CRISPR/Cas cargos and facilitating communication between adjacent cells. Overall, through producer cell engineering, EVs that stably express specific functions can be derived from donor cells. This approach enhances their biocompatibility and safety for in vivo applications.
4.2 Extracellular vesicle engineering
Addressing the challenges associated with the complex and time-consuming nature of cell engineering methods, such as production inefficiencies, a more manageable and straightforward approach involves directly loading exogenous agents or ligands onto isolated EVs. This can be achieved through methods like coincubation or electroporation, allowing for more controlled integration of functional groups into the EVs.67 This strategy is not only conducive to the efficient production of EVs loaded with the CRISPR system but also ensures the preservation of the original membrane protein structures and the integrity of the EVs.116
Kooijmans et al. developed an innovative noncovalent modification technique for the surface of EVs, termed “post-insertion”, to enhance tumor-targeting specificity and extend the circulation time of EVs (Fig. 8B).117 Initially, nanobodies targeting the EGFR (EGa1) were conjugated with phospholipid (DMPE)-PEG derivatives to create nanobody-PEG-micelles through chemical coupling. Recognizing that the integration of PEG-lipids into liposomes is temperature-dependent, these nanobody-PEG micelles were incubated with EVs derived from Neuro2A cells at various temperatures to elucidate the post-insertion mechanism. Characterization results indicated that the optimal temperature for integrating EGa1-PEG-micelles into the EVs was 40 °C, a condition that preserved the original properties of the EVs. This post-insertion engineering approach for EVs not only facilitates targeted drug delivery to specific locations but also shows great promise for transporting CRISPR systems efficiently.117
Expanding on prior research that investigated drug accumulation in parenchymal hepatocytes using pullulan modification, Tamura and colleagues developed a technique to conjugate negatively charged exosomes, secreted from mesenchymal stem cells, with cationic pullulan through electrostatic interactions.118 This approach leveraged pullulan as a targeting ligand for asialoglycoprotein receptors on hepatocytes, significantly enhancing the specific accumulation and targeted delivery capabilities of exosomes for liver therapy. Direct engineering of EVs can also be accomplished through covalent bioconjugation. Smyth et al. utilized a highly efficient, rapid-click chemistry reaction to incorporate azide-fluor 545 into exosomes.119 This method of chemical modification not only preserves the structural integrity of the EVs but also maintains high reactivity, allowing for large-scale production (Fig. 8B). This advancement in EV engineering demonstrates the potential for precise, targeted delivery of therapeutic agents, including CRISPR systems. Generally, most engineering methods for EVs are conducted through chemical processes.48 These methods not only preserve the structural integrity of the EVs but also maintain high reactivity, facilitating large-scale production. However, the biocompatibility of chemically modified EVs may be compromised, necessitating further evaluation of their safety for in vivo applications.
4.3 Virus-like particle engineering
Viral vectors, including AAVs and adenoviral vectors, are currently among the most efficient carriers for delivering CRISPR systems.120 However, their use often leads to challenges such as prolonged gene expression and genomic integration, raising concerns about off-target effects and adverse immunogenic responses. VLPs present an innovative solution by harnessing virus-like properties, including the ability to escape endosomes, package cargo, and target specific cells. These attributes significantly reduce the risks associated with off-target effects and genomic integration. As a result, virus-mimetic engineering holds considerable promise for advancing gene-editing therapeutics using the CRISPR system, combining the efficiency of viral delivery with a safer profile.
The host specificity of viral vectors is largely influenced by their envelope glycoproteins. By engineering various envelope glycoproteins, researchers can impart specific cell-targeting capabilities to pseudotyped particles. For instance, the broad tropism and immunogenicity of VSV-G-pseudotyped lentiviral vectors limit their application. Studies have highlighted the significance of HCV/E1E2 glycoproteins in viral invasion, particularly their liver-targeting properties, which have garnered considerable interest.121 Capitalizing on this, Lee and colleagues developed HCV/E1E2-pseudotyped lentiviral vectors as an alternative to VSV-G-pseudotyping for the targeted delivery of the CRISPR/Cas system in HCC therapy.122 Unlike VSV-G-pseudotyping particles, HCV/E1E2 targets Huh7 HCC cells specifically, which highly express CD81 and claudin-1 receptors, and efficiently accumulates at Huh7 tumor sites. Comparative studies of E1E2-LV/GFP and VSV-G-LV/GFP vectors across different HCC cell lines revealed that E1E2-LV showed highly selective transduction in Huh7 cells. The use of E1E2-LV pseudoparticles to transport Cas9 and sgRNA targeting the kinesin spindle protein gene resulted in effective apoptosis, demonstrating the selective targeting ability of E1E2-LV/sgKSP and its potential as a CRISPR/Cas9 system carrier.122 Furthermore, Prel et al. successfully loaded mRNA drugs onto modified HIV-1 vectors using a dimerization-independent MS2-driven RNA packaging system.123 This involved engineering the ZF2 domain of the HIV-1 nucleocapsid protein with the MS2 coat protein (MS2cp). MS2 aptamer (MS2apt) was incorporated into mRNA drugs, and through the dimerization interaction between MS2cp and MS2apt, mRNAs were efficiently packaged into engineered VLPs. This method not only demonstrated high loading efficiency but also presented a viable approach for encapsulating CRISPR systems in VLPs.
Liu's group successfully synthesized the first generation of engineered base-editor VLPs.124 They conjugated the highly active adenine base editor ABE8e and sgRNA to the C-terminus of the Friend murine leukemia virus (FMLV) gag polyprotein using a linker peptide. By modifying these VLPs with the VSV-G envelope glycoprotein, they were able to specifically target HEK293T cells and achieve genomic editing with an efficiency exceeding 97%. The base-editor cargo was designed for release through linker cleavage. To enhance cargo release and loading into the engineered VLPs, the researchers screened various protease-cleavable linker sequences between the MLV gag and ABE8e, incorporating linkers with nuclear export signals (NESs) to facilitate BE incorporation.124,125 Following VLP maturation, these linkers were degraded by proteases, allowing the base editor to localize to the nucleus in transduced cells. The team optimized the architecture of the VLPs by balancing the gag-cargo:gag–pro–pol stoichiometry, effectively managing the trade-off between active base editors incorporated per VLP, total VLP titers, and other factors impacting VLP efficiency. Additionally, the tissue specificity of these BE-VLPs was made adjustable through the engineering of various fusogens (Fig. 8C). Compared to their first-generation counterparts, the latest engineered VLPs have shown a dozen-fold improvement in loading capacity and gene editing efficiency. This represents a promising approach for the specific delivery of CRISPR RNPs to mediate genome editing in vivo.124 However, it's important to note that while modifications to extracellular vesicles or parental cells can improve delivery efficiency and targeting capacity, different engineering strategies come with their own set of advantages and limitations in clinical applications, as summarized in Table 3.
Table 3 Comparison of “producer cell engineering”, “extracellular vesicles engineering” and “virus-like engineering” techniques
Engineering techniques |
Advantages |
Limitations |
Ref. |
Producer cell engineering |
• Guarantees EVs structure intactness and functionalization |
• Time-consuming and complicated |
54, 143 and 144 |
|
• Universally applicable |
|
|
Extracellular vesicles engineering |
|
• Difficult to achieve industrial production |
53, 55, 67 and 116 |
• Better control of cargo loading and modification |
• Further purification is required |
|
|
VLP engineering |
• Dramatically decreases off-target effect and genome integration risk |
• Expression of related proteins is not enough for assembly |
71, 120 and 124 |
• Strengthens CRISPR agent's loading capacity and targeting ability. |
• Complicated purification |
VLP engineering harnesses the inherent capabilities of viruses, including cargo packaging, endosome escape, and the potential for reprogramming to target various cell types, rendering it a valuable method for delivering the CRISPR/Cas system. However, this approach is constrained by the high immunogenicity associated with viral vectors, which limits its application in vivo.94
5. Critical barriers to clinical translation
Over the past decade, the CRISPR gene editing system has significantly advanced and is now poised to make a substantial impact.126 EVs, with their biocompatibility and high transfection efficiency, are ideal vectors for CRISPR gene editing. Nevertheless, the clinical translation of EV-based delivery systems faces major challenges, particularly in terms of stability during internal transport, targeting ability during internal transport, and safety. These critical barriers must be addressed to realize the full potential of EVs as vectors in clinical settings.127 This review aims to summarize the current challenges and provide insights into the future of EV-based delivery, with a detailed discussion to follow (Fig. 9).
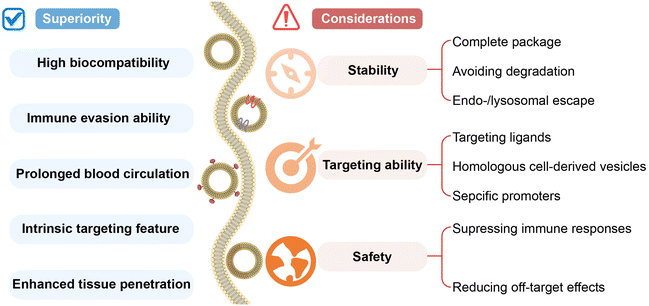 |
| Fig. 9 The superiorities and challenges of functionalized EVs as CRISPR genome editing technology nanocarrier. | |
5.1 Stability during internal transport
Throughout the EV production process, functional elements like proteins or nucleic acids can be deliberately incorporated into EVs.128,129 Common physical methods for embedding CRISPR systems into EVs, including electroporation, sonication, pH gradient modification, and extrusion, may compromise the stability and integrity of EVs. These techniques can significantly alter the components of EVs, potentially creating large gaps in the lipid bilayer membranes, leading to the loss of EV markers, and causing aggregation. Additionally, they might damage the cargo contained within the EVs.22 Furthermore, endocytosis is the primary method by which recipient cells internalize EVs. Once internalized, the EVs are transported into endosomes, from which some may escape to release CRISPR cargos into the cytoplasm and ultimately to the target site. However, a portion of these EVs could be degraded by lysosomes. Enhancing endosomal escape is therefore crucial for successful CRISPR delivery. Recent studies suggest that incorporating pH-sensitive GALA peptides and melittin can improve endo/lysosomal escape capabilities.130,131 Moreover, as carriers of CRISPR drugs, EVs may trigger immune responses or undergo enzymatic degradation during transit. Particularly if the production source differs from the recipient species, immune reactions can occur, underscoring the need for careful consideration of the source in EV-based CRISPR delivery.132
5.2 Targeting ability during internal transport
The targeting efficacy of EVs during internal transport is a critical aspect of CRISPR system delivery. Research indicates that natural EVs tend to predominantly accumulate in the liver (approximately 84%) and spleen (around 14%).22 This organ-specific accumulation presents a challenge in directing CRISPR systems precisely and specifically to intended target tissues other than the liver and spleen. Off-target effects pose significant risks, including gene toxicity or unintended gene editing at non-target loci. A particularly concerning issue is the potential for non-specific editing of generative cells, which could lead to heritable mutations and genetic diseases in future generations.133 This raises not only technical challenges but also ethical considerations. Therefore, minimizing off-target risks associated with EV-mediated CRISPR delivery is an essential prerequisite for advancing gene-editing applications in clinical settings.
5.3 Safety in the clinical applications
The safety of EV nanotherapeutics is closely tied to the origin of the parental cell lines, as this determines their intra-vesicular contents. EVs secreted from tumor cells, for instance, may contain cancer-related factors that could promote immunosuppressive phenotypes, immune escape, tumor growth, or even cell transformation.134 Therefore, the safety profile of EVs is influenced both by their cellular origin and their specific compositions. It is crucial to exercise stringent control over the EV secretion process. Furthermore, modifications to EVs that extend their circulation time can potentially elicit immune responses and raise safety concerns. A primary step in advancing modified EVs towards clinical usage is a thorough assessment of their functionality and potential immunogenicity. Due to their biological origin, EVs are likely to elicit minimal immune reactions. For example, during blood transfusions, thousands of EVs are introduced into patients without apparent adverse effects.135 Furthermore, EVs are inherently non-replicative and non-mutagenic, mitigating regulatory concerns related to adverse effects or neoplasia formation, unlike virus-derived vehicles or cell therapies.135 Studies have demonstrated that EVs, even when externally engineered and modified, do not provoke toxicity or inflammatory responses.135 A case in point involves C2C12 cell-derived EVs that were modified with anchor peptides and splice-switching oligonucleotides for muscular dystrophy treatment, exhibiting no detectable toxicity or inflammation in the liver, kidneys, or muscles of mice after intravenous injection. Therefore, EV engineering holds significant promise for delivering therapeutic molecules. Nonetheless, given the variety of engineering strategies, safety studies conducted in animal models should be interpreted cautiously. Effective management of parental cell line selection and the post-production process is vital, as these factors significantly impact the quality of cargos and the biological properties of EVs.136 Another pressing issue regarding the clinical translation of CRISPR systems is the moral and ethical considerations involved.137 While the technological advancements in CRISPR over the years have the potential to significantly benefit our lives, it is imperative to develop more comprehensive ethical and legal frameworks.138 In cases involving somatic cells, the level of risk assessment is similar to that of standard biomedical testing, but the ethical implications still require careful consideration and management.
6. Conclusion and outlook
In recent years, the CRISPR system has emerged as a revolutionary gene-editing tool, showing great promise in treating genetic diseases. Its rapid advancement highlights a critical need for parallel development in delivery technologies. Given their high biocompatibility, low immunogenicity, and excellent penetrability, EVs stand out as promising carriers for CRISPR delivery in future clinical applications. This potential positions EVs as a key focus for further research and development in the field of gene therapy, aligning with the ongoing evolution of CRISPR technology. With dedicated efforts from scientists worldwide, a range of functional EVs has been developed for CRISPR delivery. These include unmodified EVs, modified EVs, engineered VLPs, and exosome-liposome hybrid vesicles. These nanovesicles, entering cells through membrane fusion, endocytosis, or virus-like infection, are undergoing optimization to become ideal carriers for effective CRISPR delivery. However, several challenges persist in the EV-based delivery of CRISPR for clinical applications, notably concerning stability, tissue-specific targeting, and safety. Therefore, careful selection of parental cell lines and stringent post-production regulation are crucial. Despite these hurdles, the potential for improved functionalized EVs in safely and efficiently delivering CRISPR systems to target cells is immense. This advancement holds significant promise for the future of precision medicine.
Conflicts of interest
There are no conflicts to declare.
Acknowledgements
This work was supported by the National Key Research and Development Program of China (2019YFA0111300), the National Natural Science Foundation of China (52373166, 22277155, 32301121), the Natural Science Foundation of Guangdong Province for Distinguished Young Scholar (2024B1515020025), the Science and Technology Program of Guangzhou (2024A04J6572), 2024 Basic and Applied Basic Research Topic of Guangzhou Science and Technology Project (2024A04J4710), the Guangdong Province Basic and Applied Basic Research Foundation (2021A1515110534, 2022A1515110893), the Basic Research Project of Shenzhen Basic Research Special Project (JCYJ20230807110401002), the Guangdong Provincial Pearl River Talents Program (2019QN01Y131), and the China Primary Health Care Foundation (2022-003).
References
- M. An, A. Raguram, S. W. Du, S. Banskota, J. R. Davis, G. A. Newby, P. Z. Chen, K. Palczewski and D. R. Liu, Nat. Biotechnol., 2024 DOI:10.1038/s41587-023-02078-y.
- X. Meng, D. Yao, K. Imaizumi, X. Chen, K. W. Kelley, N. Reis, M. V. Thete, A. Arjun McKinney, S. Kulkarni, G. Panagiotakos, M. C. Bassik and S. P. Paşca, Nature, 2023, 622, 359–366 CrossRef CAS PubMed.
- A. Katti, B. J. Diaz, C. M. Caragine, N. E. Sanjana and L. E. Dow, Nat. Rev. Cancer, 2022, 22, 259–279 CrossRef CAS PubMed.
- W. Zeng, L. Zheng, Y. Li, J. Yang, T. Mao, J. Zhang, Y. Liu, J. Ning, T. Zhang, H. Huang, X. Chen and F. Lu, Emerging Microbes Infect., 2024, 13, 2284286 CrossRef PubMed.
- S. Dong, X. Liu, Y. Bi, Y. Wang, A. Antony, D. Lee, K. Huntoon, S. Jeong, Y. Ma, X. Li, W. Deng, B. R. Schrank, A. J. Grippin, J. Ha, M. Kang, M. Chang, Y. Zhao, R. Sun, X. Sun, J. Yang, J. Chen, S. K. Tang, L. J. Lee, A. S. Lee, L. Teng, S. Wang, L. Teng, B. Y. S. Kim, Z. Yang and W. Jiang, Nat. Commun., 2023, 14, 6610 CrossRef CAS PubMed.
- Y. Tao, K. Yi, H. Wang, H.-W. Kim, K. Li, X. Zhu and M. Li, J. Colloid Interface Sci., 2022, 613, 406–414 CrossRef CAS PubMed.
- H. Kong, C. Zhuo, K. Yi, C. Zheng, R. L. Mintz, Y.-H. Lao, Q. Zhong, E. Ju, H. Wang, D. Shao, H. Xiao, Y. Tao and M. Li, Nano Today, 2023, 53, 102040 CrossRef CAS.
- J. Y. Wang and J. A. Doudna, Science, 2023, 379, eadd8643 CrossRef CAS PubMed.
- H. Kong, E. Ju, K. Yi, W. Xu, Y.-H. Lao, D. Cheng, Q. Zhang, Y. Tao, M. Li and J. Ding, Adv. Sci., 2021, 8, 2102051 CrossRef CAS PubMed.
- Z. Xu, Q. Wang, H. Zhong, Y. Jiang, X. Shi, B. Yuan, N. Yu, S. Zhang, X. Yuan, S. Guo and Y. Yang, Exploration, 2022, 2, 20210081 CrossRef CAS PubMed.
- F. Chen, M. Alphonse and Q. Liu, Wiley Interdiscip. Rev.: Nanomed. Nanobiotechnol., 2020, 12, e1609 Search PubMed.
- C. Liu, L. Zhang, H. Liu and K. Cheng, J. Controlled Release, 2017, 266, 17–26 CrossRef CAS PubMed.
- Y. Tao, K. Yi, H. Hu, D. Shao and M. Li, J. Mater. Chem. B, 2021, 9, 94–100 RSC.
- L. Li, S. Hu and X. Chen, Biomaterials, 2018, 171, 207–218 CrossRef CAS PubMed.
- F. Li, J. Zhang, K. Yi, H. Wang, H. Wei, H. F. Chan, Y. Tao and M. Li, ACS Appl. Bio Mater., 2022, 5, 2009–2030 CrossRef CAS PubMed.
- J. A. Zuris, D. B. Thompson, Y. Shu, J. P. Guilinger, J. L. Bessen, J. H. Hu, M. L. Maeder, J. K. Joung, Z. Y. Chen and D. R. Liu, Nat. Biotechnol., 2015, 33, 73–80 CrossRef CAS PubMed.
- C. Zhuo, H. Kong, K. Yi, C.-W. Chi, J. Zhang, R. Chen, H. Wang, C. Wu, Y.-H. Lao, Y. Tao and M. Li, Adv. Funct. Mater., 2023, 33, 2210860 CrossRef CAS.
- Q. Liu, C. Wang, Y. Zheng, Y. Zhao, Y. Wang, J. Hao, X. Zhao, K. Yi, L. Shi, C. Kang and Y. Liu, Biomaterials, 2020, 258, 120275 CrossRef CAS PubMed.
- K. Lee, M. Conboy, H. M. Park, F. Jiang, H. J. Kim, M. A. Dewitt, V. A. Mackley, K. Chang, A. Rao, C. Skinner, T. Shobha, M. Mehdipour, H. Liu, W. C. Huang, F. Lan, N. L. Bray, S. Li, J. E. Corn, K. Kataoka, J. A. Doudna, I. Conboy and N. Murthy, Nat. Biomed. Eng., 2017, 1, 889–901 CrossRef CAS PubMed.
- M. Hansen-Bruhn, B. E. de Avila, M. Beltran-Gastelum, J. Zhao, D. E. Ramirez-Herrera, P. Angsantikul, K. Vesterager Gothelf, L. Zhang and J. Wang, Angew. Chem., Int. Ed., 2018, 57, 2657–2661 CrossRef CAS PubMed.
- K. Horodecka and M. Düchler, Int. J. Mol. Sci., 2021, 22, 6072–6097 CrossRef CAS PubMed.
- D. Kostyushev, A. Kostyusheva, S. Brezgin, V. Smirnov, E. Volchkova, A. Lukashev and V. Chulanov, Int. J. Mol. Sci., 2020, 21, 7362 CrossRef CAS PubMed.
- J. A. Whitley, S. Kim, L. Lou, C. Ye, O. A. Alsaidan, E. Sulejmani, J. Cai, E. G. Desrochers, Z. Beharry, C. B. Rickman, M. Klingeborn, Y. Liu, Z. R. Xie and H. Cai, J. Extracell. Vesicles, 2022, 11, e12196 CrossRef CAS PubMed.
- R. Mout, M. Ray, Y. W. Lee, F. Scaletti and V. M. Rotello, Bioconjugate Chem., 2017, 28, 880–884 CrossRef CAS PubMed.
- C. T. Charlesworth, P. S. Deshpande, D. P. Dever, J. Camarena, V. T. Lemgart, M. K. Cromer, C. A. Vakulskas, M. A. Collingwood, L. Zhang, N. M. Bode, M. A. Behlke, B. Dejene, B. Cieniewicz, R. Romano, B. J. Lesch, N. Gomez-Ospina, S. Mantri, M. Pavel-Dinu, K. I. Weinberg and M. H. Porteus, Nat. Med., 2019, 25, 249–254 CrossRef CAS PubMed.
- D. L. Wagner, L. Amini, D. J. Wendering, L. M. Burkhardt, L. Akyuz, P. Reinke, H. D. Volk and M. Schmueck-Henneresse, Nat. Med., 2019, 25, 242–248 CrossRef CAS PubMed.
- S. Kim, T. Koo, H.-G. Jee, H.-Y. Cho, G. Lee, D.-G. Lim, H. S. Shin and J.-S. Kim, Genome Res., 2018, 28, 367–373 CrossRef CAS PubMed.
- K. S. Makarova, Y. I. Wolf, J. Iranzo, S. A. Shmakov, O. S. Alkhnbashi, S. J. J. Brouns, E. Charpentier, D. Cheng, D. H. Haft, P. Horvath, S. Moineau, F. J. M. Mojica, D. Scott, S. A. Shah, V. Siksnys, M. P. Terns, C. Venclovas, M. F. White, A. F. Yakunin, W. Yan, F. Zhang, R. A. Garrett, R. Backofen, J. van der Oost, R. Barrangou and E. V. Koonin, Nat. Rev. Microbiol., 2020, 18, 67–83 CrossRef CAS PubMed.
- X. Huang, A. Li, P. Xu, Y. Yu, S. Li, L. Hu and S. Feng, J. Nanobiotechnol., 2023, 21, 184 CrossRef PubMed.
- S. EL Andaloussi, I. Mäger, X. O. Breakefield and M. J. A. Wood, Nat. Rev. Drug Discovery, 2013, 12, 347–357 CrossRef CAS PubMed.
- P. Gee, M. S. Y. Lung, Y. Okuzaki, N. Sasakawa, T. Iguchi, Y. Makita, H. Hozumi, Y. Miura, L. F. Yang, M. Iwasaki, X. H. Wang, M. A. Waller, N. Shirai, Y. O. Abe, Y. Fujita, K. Watanabe, A. Kagita, K. A. Iwabuchi, M. Yasuda, H. Xu, T. Noda, J. Komano, H. Sakurai, N. Inukai and A. Hotta, Nat. Commun., 2020, 11, 1334 CrossRef CAS PubMed.
- W. S. de Voogt, M. E. Tanenbaum and P. Vader, Adv. Drug Delivery Rev., 2021, 174, 250–264 CrossRef CAS PubMed.
- J. Johnston, T. Stone Jr. and Y. Wang, Biomater. Sci., 2023, 11, 4055–4072 RSC.
- S. Yang, B. Zheng, F. Raza, S. Zhang, W. E. Yuan, J. Su and M. Qiu, Biomater. Sci., 2024, 12, 1131–1150 RSC.
- F. Raza, H. Zafar, L. Jiang, J. Su, W. Yuan, M. Qiu and A. C. Paiva-Santos, Biomater. Sci., 2023, 12, 57–91 RSC.
- M. Zhang, L. Wan, R. Li, X. Li, T. Zhu and H. Lu, Biomater. Sci., 2023, 11, 7247–7267 RSC.
- G. Raposo and W. Stoorvogel, J. Cell Biol., 2013, 200, 373–383 CrossRef CAS PubMed.
- S. Kamerkar, V. S. LeBleu, H. Sugimoto, S. Yang, C. F. Ruivo, S. A. Melo, J. J. Lee and R. Kalluri, Nature, 2017, 546, 498–503 CrossRef CAS PubMed.
- S. Fais, L. O'Driscoll, F. E. Borras, E. Buzas, G. Camussi, F. Cappello, J. Carvalho, A. Cordeiro da Silva, H. Del Portillo, S. El Andaloussi, T. Ficko Trcek, R. Furlan, A. Hendrix, I. Gursel, V. Kralj-Iglic, B. Kaeffer, M. Kosanovic, M. E. Lekka, G. Lipps, M. Logozzi, A. Marcilla, M. Sammar, A. Llorente, I. Nazarenko, C. Oliveira, G. Pocsfalvi, L. Rajendran, G. Raposo, E. Rohde, P. Siljander, G. van Niel, M. H. Vasconcelos, M. Yanez-Mo, M. L. Yliperttula, N. Zarovni, A. B. Zavec and B. Giebel, ACS Nano, 2016, 10, 3886–3899 CrossRef CAS PubMed.
- M. Lu, H. Xing, Z. Yang, Y. Sun, T. Yang, X. Zhao, C. Cai, D. Wang and P. Ding, Eur. J. Pharm. Biopharm., 2017, 119, 381–395 CrossRef CAS PubMed.
- L. Barile and G. Vassalli, Pharmacol. Ther., 2017, 174, 63–78 CrossRef CAS PubMed.
- T. Smyth, M. Kullberg, N. Malik, P. Smith-Jones, M. W. Graner and T. J. Anchordoquy, J. Controlled Release, 2015, 199, 145–155 CrossRef CAS PubMed.
- D. Haussecker, J. Controlled Release, 2014, 195, 49–54 CrossRef CAS PubMed.
- M. E. Hung and J. N. Leonard, J. Biol. Chem., 2015, 290, 8166–8172 CrossRef CAS PubMed.
- Y. Yang, Y. Hong, G.-H. Nam, J. H. Chung, E. Koh and I.-S. Kim, Adv. Mater., 2017, 29, 1605604 CrossRef PubMed.
- Y. Tian, L. Ma, M. Gong, G. Su, S. Zhu, W. Zhang, S. Wang, Z. Li, C. Chen, L. Li, L. Wu and X. Yan, ACS Nano, 2018, 12, 671–680 CrossRef CAS PubMed.
- R. Szatanek, M. Baj-Krzyworzeka, J. Zimoch, M. Lekka, M. Siedlar and J. Baran, Int. J. Mol. Sci., 2017, 18, 1153 CrossRef PubMed.
- S. Du, Y. Guan, A. Xie, Z. Yan, S. Gao, W. Li, L. Rao, X. Chen and T. Chen, J. Nanobiotechnol., 2023, 21, 231 CrossRef PubMed.
- H. Lee, H. Park, G. J. Noh and E. S. Lee, Carbohydr. Polym., 2018, 202, 323–333 CrossRef CAS PubMed.
- Y. Liang, Z. Iqbal, J. Wang, L. Xu, X. Xu, K. Ouyang, H. Zhang, J. Lu, L. Duan and J. Xia, Biomater. Sci., 2022, 10, 4095–4106 RSC.
- H. Costa Verdera, J. J. Gitz-Francois, R. M. Schiffelers and P. Vader, J. Controlled Release, 2017, 266, 100–108 CrossRef CAS PubMed.
- L. Duan, K. Ouyang, J. Wang, L. Xu, X. Xu, C. Wen, Y. Xie, Y. Liang and J. Xia, ChemBioChem, 2021, 22, 3360–3368 CrossRef CAS PubMed.
- W. M. Usman, T. C. Pham, Y. Y. Kwok, L. T. Vu, V. Ma, B. Peng, Y. S. Chan, L. Wei, S. M. Chin, A. Azad, A. B. He, A. Y. H. Leung, M. Yang, N. Shyh-Chang, W. C. Cho, J. Shi and M. T. N. Le, Nat. Commun., 2018, 9, 2359 CrossRef PubMed.
- R. Chen, H. Huang, H. Liu, J. Xi, J. Ning, W. Zeng, C. Shen, T. Zhang, G. Yu, Q. Xu, X. Chen, J. Wang and F. Lu, Small, 2019, 15, e1902686 CrossRef PubMed.
- S. M. Kim, Y. Yang, S. J. Oh, Y. Hong, M. Seo and M. Jang, J. Controlled Release, 2017, 266, 8–16 CrossRef CAS PubMed.
- C. He, D. Jaffar Ali, H. Xu, S. Kumaravel, K. Si, Y. Li, B. Sun, J. Ma and Z. Xiao, Exp. Cell Res., 2020, 392, 112040 CrossRef CAS PubMed.
- T. Wan, J. Zhong, Q. Pan, T. Zhou, Y. Ping and X. Liu, Sci. Adv., 2022, 8, eabp9435 CrossRef CAS PubMed.
- K. Dooley, R. E. McConnell, K. Xu, N. D. Lewis, S. Haupt, M. R. Youniss, S. Martin, C. L. Sia, C. McCoy, R. J. Moniz, O. Burenkova, J. Sanchez-Salazar, S. C. Jang, B. Choi, R. A. Harrison, D. Houde, D. Burzyn, C. Leng, K. Kirwin, N. L. Ross, J. D. Finn, L. Gaidukov, K. D. Economides, S. Estes, J. E. Thornton, J. D. Kulman, S. Sathyanarayanan and D. E. Williams, Mol. Ther., 2021, 29, 1729–1743 CrossRef CAS PubMed.
- Q. Wang, J. Yu, T. Kadungure, J. Beyene, H. Zhang and Q. Lu, Nat. Commun., 2018, 9, 960 CrossRef PubMed.
- X. Zhang, Q. Xu, Z. Zi, Z. Liu, C. Wan, L. Crisman, J. Shen and X. Liu, Dev. Cell, 2020, 55, 784–801 CrossRef CAS PubMed.
- Y. Ye, X. Zhang, F. Xie, B. Xu, P. Xie, T. Yang, Q. Shi, C. Y. Zhang, Y. Zhang, J. Chen, X. Jiang and J. Li, Biomater. Sci., 2020, 8, 2966–2976 RSC.
- Z. Li, X. Zhou, M. Wei, X. Gao, L. Zhao, R. Shi, W. Sun, Y. Duan, G. Yang and L. Yuan, Nano Lett., 2019, 19, 19–28 CrossRef CAS PubMed.
- X. Song, C. Liu, N. Wang, H. Huang, S. He, C. Gong and Y. Wei, Adv. Drug Delivery Rev., 2021, 168, 158–180 CrossRef CAS PubMed.
- S. Zhang, J. Shen, D. Li and Y. Cheng, Theranostics, 2021, 11, 614–648 CrossRef CAS PubMed.
- G. Ahn, S. M. Banik, C. L. Miller, N. M. Riley, J. R. Cochran and C. R. Bertozzi, Nat. Chem. Biol., 2021, 17, 937–946 CrossRef CAS PubMed.
- Q. Xu, Z. Zhang, L. Zhao, Y. Qin, H. Cai, Z. Geng, X. Zhu, W. Zhang, Y. Zhang, J. Tan, J. Wang and J. Zhou, J. Controlled Release, 2020, 326, 455–467 CrossRef CAS PubMed.
- J. Zhuang, J. Tan, C. Wu, J. Zhang, T. Liu, C. Fan, J. Li and Y. Zhang, Nucleic Acids Res., 2020, 48, 8870–8882 CrossRef CAS PubMed.
- S. Ohno, M. Takanashi, K. Sudo, S. Ueda, A. Ishikawa, N. Matsuyama, K. Fujita, T. Mizutani, T. Ohgi, T. Ochiya, N. Gotoh and M. Kuroda, Mol. Ther., 2013, 21, 185–191 CrossRef CAS PubMed.
- D. Gulei and I. Berindan-Neagoe, Mol. Ther.–Nucleic Acids, 2019, 17, 448–451 CrossRef CAS PubMed.
- L. Zhang, Y. Lin, S. Li, X. Guan and X. Jiang, Angew. Chem., Int. Ed., 2023, 62, e202217089 CrossRef CAS PubMed.
- A. Raguram, S. Banskota and D. R. Liu, Cell, 2022, 185, 2806–2827 CrossRef CAS PubMed.
- C. F. Xu, G. J. Chen, Y. L. Luo, Y. Zhang, G. Zhao, Z. D. Lu, A. Czarna, Z. Gu and J. Wang, Adv. Drug Delivery Rev., 2021, 168, 3–29 CrossRef CAS PubMed.
- R. J. Chandler, M. S. Sands and C. P. Venditti, Hum. Gene Ther., 2017, 28, 314–322 CrossRef CAS PubMed.
- P. Lyu, L. Wang and B. Lu, Life, 2020, 10, 366 CrossRef CAS PubMed.
- D. Kim, Q. V. Le, Y. Wu, J. Park and Y. K. Oh, Pharmaceutics, 2020, 12, 1233–1275 CrossRef CAS PubMed.
- E. B. Lee, J. H. Kim, W. Hur, J. E. Choi, S. M. Kim, D. J. Park, B. Y. Kang, G. W. Lee and S. K. Yoon, Sci. Rep., 2019, 9, 1616 CrossRef PubMed.
- C. Montagna, G. Petris, A. Casini, G. Maule, G. M. Franceschini, I. Zanella, L. Conti, F. Arnoldi, O. R. Burrone, L. Zentilin, S. Zacchigna, M. Giacca and A. Cereseto, Mol. Ther.–Nucleic Acids, 2018, 12, 453–462 CrossRef CAS PubMed.
- P. E. Mangeot, V. Risson, F. Fusil, A. Marnef, E. Laurent, J. Blin, V. Mournetas, E. Massourides, T. J. M. Sohier, A. Corbin, F. Aube, M. Teixeira, C. Pinset, L. Schaeffer, G. Legube, F. L. Cosset, E. Verhoeyen, T. Ohlmann and E. P. Ricci, Nat. Commun., 2019, 10, 45 CrossRef CAS PubMed.
- D. Yin, S. Ling, D. Wang, Y. Dai, H. Jiang, X. Zhou, S. R. Paludan, J. Hong and Y. Cai, Nat. Biotechnol., 2021, 39, 567–577 CrossRef CAS PubMed.
- J. Nikolic, L. Belot, H. Raux, P. Legrand, Y. Gaudin and A. A. Albertini, Nat. Commun., 2018, 9, 1029 CrossRef PubMed.
- J. Mondal, S. Pillarisetti, V. Junnuthula, M. Saha, S. R. Hwang, I. K. Park and Y. K. Lee, J. Controlled Release, 2022, 353, 1127–1149 CrossRef PubMed.
- Q. Zhong, C. Zheng, K. Yi, R. L. Mintz, S. Lv, Y. Tao and M. Li, Biomater. Sci., 2023, 11, 4774–4788 RSC.
- K. Yi, H. Kong, C. Zheng, C. Zhuo, Y. Jin, Q. Zhong, R. L. Mintz, E. Ju, H. Wang, S. Lv, Y.-H. Lao, Y. Tao and M. Li, Biomaterials, 2023, 302, 122349 CrossRef CAS PubMed.
- S. Sharma, R. Parveen and B. P. Chatterji, Curr. Pathobiol. Rep., 2021, 9, 133–144 CrossRef CAS PubMed.
- B. Yip, Biomolecules, 2020, 10, 839 CrossRef CAS PubMed.
- Y. Li, A. Lu, M. Long, L. Cui, Z. Chen and L. Zhu, Acta Biomater., 2019, 83, 334–348 CrossRef CAS PubMed.
- Y.-H. Ou, J. Liang, B. Czarny, M. G. Wacker, V. Yu, J.-W. Wang and G. Pastorin, Semin. Cancer Biol., 2021, 74, 45–61 CrossRef CAS PubMed.
- S. Rayamajhi, T. D. T. Nguyen, R. Marasini and S. Aryal, Acta Biomater., 2019, 94, 482–494 CrossRef CAS PubMed.
- Y. Lin, J. Wu, W. Gu, Y. Huang, Z. Tong, L. Huang and J. Tan, Adv. Sci., 2018, 5, 1700611 CrossRef PubMed.
- Y. Liang, X. Xu, L. Xu, Z. Iqbal, K. Ouyang, H. Zhang, C. Wen, L. Duan and J. Xia, Theranostics, 2022, 12, 4866–4878 CrossRef CAS PubMed.
- H. Shin and J. Kim, Chem. Commun., 2022, 58, 1860–1870 RSC.
- X. Zhang, H. Zhang, J. Gu, J. Zhang, H. Shi, H. Qian, D. Wang, W. Xu, J. Pan and H. A. Santos, Adv. Mater., 2021, 33, 2005709 CrossRef CAS PubMed.
- C. Zheng, Q. Zhong, K. Yi, H. Kong, F. Cao, C. Zhuo, Y. Xu, R. Shi, E. Ju, W. Song, Y. Tao, X. Chen and M. Li, Sci. Adv., 2023, 9, eadh2413 CrossRef CAS PubMed.
- V. Madigan, F. Zhang and J. E. Dahlman, Nat. Rev. Drug Discovery, 2023, 22, 875–894 CrossRef CAS PubMed.
- T. L. Reinhard Jahn and T. C. Südhof, Cell, 2003, 112, 519–533 CrossRef PubMed.
- X. Ji, Y. Ma, W. Liu, L. Liu, H. Yang, J. Wu, X. Zong, J. Dai and W. Xue, ACS Nano, 2020, 14, 7462–7474 CrossRef CAS PubMed.
- H. Kong, K. Yi, C. Zheng, Y. H. Lao, H. Zhou, H. F. Chan, H. Wang, Y. Tao and M. Li, J. Mater. Chem. B, 2022, 10, 6841–6858 RSC.
- C. Zheng, Q. Zhong, W. Song, K. Yi, H. Kong, H. Wang, Y. Tao, M. Li and X. Chen, Adv. Mater., 2023, 35, 2206989 CrossRef CAS PubMed.
- J. Meldolesi, Curr. Biol., 2018, 28, 435–444 CrossRef PubMed.
- T. Tian, L. Cao, C. He, Q. Ye, R. Liang, W. You, H. Zhang, J. Wu, J. Ye, B. A. Tannous and J. Gao, Theranostics, 2021, 11, 6507–6521 CrossRef CAS PubMed.
- J. Lee, J. Kim, M. Jeong, H. Lee, U. Goh, H. Kim, B. Kim and J. H. Park, Nano Lett., 2015, 15, 2938–2944 CrossRef CAS PubMed.
- R. Cecchin, Z. Troyer, K. Witwer and K. V. Morris, Mol. Ther., 2023, 31, 1255–1230 CrossRef PubMed.
- Y. Ju, H. Guo, M. Edman and S. F. Hamm-Alvarez, Adv. Drug Delivery Rev., 2020, 157, 118–141 CrossRef CAS PubMed.
- T. Tian, Y. L. Zhu, Y. Y. Zhou, G. F. Liang, Y. Y. Wang, F. H. Hu and Z. D. Xiao, J. Biol. Chem., 2014, 289, 22258–22267 CrossRef CAS PubMed.
- K. J. Svensson, H. C. Christianson, A. Wittrup, E. Bourseau-Guilmain, E. Lindqvist, L. M. Svensson, M. Morgelin and M. Belting, J. Biol. Chem., 2013, 288, 17713–17724 CrossRef CAS PubMed.
- W. Heusermann, J. Hean, D. Trojer, E. Steib, S. von Bueren, A. Graff-Meyer, C. Genoud, K. Martin, N. Pizzato, J. Voshol, D. V. Morrissey, S. E. Andaloussi, M. J. Wood and N. C. Meisner-Kober, J. Cell Biol., 2016, 213, 173–184 CrossRef CAS PubMed.
- E. A. Taha, J. Lee and A. Hotta, J. Controlled Release, 2022, 342, 345–361 CrossRef CAS PubMed.
- X. Gao, J. Ding, Q. Long and C. Zhan, Wiley Interdiscip. Rev.: Nanomed. Nanobiotechnol., 2021, 13, e1692 CAS.
- Y. Niu, M. Yu, S. B. Hartono, J. Yang, H. Xu, H. Zhang, J. Zhang, J. Zou, A. Dexter, W. Gu and C. Yu, Adv. Mater., 2013, 25, 6233–6237 CrossRef CAS PubMed.
- W. Wang, P. Wang, X. Tang, A. A. Elzatahry, S. Wang, D. Al-Dahyan, M. Zhao, C. Yao, C. T. Hung, X. Zhu, T. Zhao, X. Li, F. Zhang and D. Zhao, ACS Cent. Sci., 2017, 3, 839–846 CrossRef CAS PubMed.
- J. M. Freire, I. Rego de Figueiredo, J. Valle, A. S. Veiga, D. Andreu, F. J. Enguita and M. A. Castanho, J. Controlled Release, 2017, 245, 127–136 CrossRef CAS PubMed.
- C. Vindry, O. Guillin, P. E. Mangeot, T. Ohlmann and L. Chavatte, Cells, 2019, 8, 574–595 CrossRef CAS PubMed.
- J. R. Hamilton, C. A. Tsuchida, D. N. Nguyen, B. R. Shy, E. R. McGarrigle, C. R. Sandoval Espinoza, D. Carr, F. Blaeschke, A. Marson and J. A. Doudna, Cell Rep., 2021, 35, 109207 CrossRef CAS PubMed.
- J. Lee, H. Lee, U. Goh, J. Kim, M. Jeong, J. Lee and J. H. Park, ACS Appl. Mater. Interfaces, 2016, 8, 6790–6795 CrossRef CAS PubMed.
- L. Alvarez-Erviti, Y. Seow, H. Yin, C. Betts, S. Lakhal and M. J. Wood, Nat. Biotechnol., 2011, 29, 341–345 CrossRef CAS PubMed.
- J. P. Armstrong, M. N. Holme and M. M. Stevens, ACS Nano, 2017, 11, 69–83 CrossRef CAS PubMed.
- S. A. A. Kooijmans, L. A. L. Fliervoet, R. van der Meel, M. Fens, H. F. G. Heijnen, P. M. P. van Bergen En Henegouwen, P. Vader and R. M. Schiffelers, J. Controlled Release, 2016, 224, 77–85 CrossRef CAS PubMed.
- R. Tamura, S. Uemoto and Y. Tabata, Acta Biomater., 2017, 57, 274–284 CrossRef CAS PubMed.
- T. Smyth, K. Petrova, N. M. Payton, I. Persaud, J. S. Redzic, M. W. Graner, P. Smith-Jones and T. J. Anchordoquy, Bioconjugate Chem., 2014, 25, 1777–1784 CrossRef CAS PubMed.
- S. Suh, E. H. Choi, H. Leinonen, A. T. Foik, G. A. Newby, W. H. Yeh, Z. Dong, P. D. Kiser, D. C. Lyon, D. R. Liu and K. Palczewski, Nat. Biomed. Eng., 2021, 5, 169–178 CrossRef CAS PubMed.
- A. Torrents de la Peña, K. Sliepen, L. Eshun-Wilson, M. L. Newby, J. D. Allen, I. Zon, S. Koekkoek, A. Chumbe, M. Crispin, J. Schinkel, G. C. Lander, R. W. Sanders and A. B. Ward, Science, 2022, 378, 263–269 CrossRef PubMed.
- S. Lee, Y. Y. Kim and H. J. Ahn, Biomaterials, 2021, 272, 120793 CrossRef CAS PubMed.
- A. Prel, V. Caval, R. Gayon, P. Ravassard, C. Duthoit, E. Payen, L. Maouche-Chretien, A. Creneguy, T. H. Nguyen, N. Martin, E. Piver, R. Sevrain, L. Lamouroux, P. Leboulch, F. Deschaseaux, P. Bouille, L. Sensebe and J. C. Pages, Mol. Ther.–Methods Clin. Dev., 2015, 2, 15039 CrossRef PubMed.
- S. Banskota, A. Raguram, S. Suh, S. W. Du, J. R. Davis, E. H. Choi, X. Wang, S. C. Nielsen, G. A. Newby, P. B. Randolph, M. J. Osborn, K. Musunuru, K. Palczewski and D. R. Liu, Cell, 2022, 185, 250–265 CrossRef CAS PubMed.
- M. F. Richter, K. T. Zhao, E. Eton, A. Lapinaite, G. A. Newby, B. W. Thuronyi, C. Wilson, L. W. Koblan, J. Zeng, D. E. Bauer, J. A. Doudna and D. R. Liu, Nat. Biotechnol., 2020, 38, 883–891 CrossRef CAS PubMed.
- B. Yan and Y. Liang, Int. J. Mol. Sci., 2022, 23, 15758–15767 CrossRef CAS PubMed.
- C. Zhuo, J. Zhang, J. H. Lee, J. Jiao, D. Cheng, L. Liu, H. W. Kim, Y. Tao and M. Li, Signal Transduction Targeted Ther., 2021, 6, 238 CrossRef CAS PubMed.
- T. Janas, M. M. Janas, K. Sapon and T. Janas, FEBS Lett., 2015, 589, 1391–1398 CrossRef CAS PubMed.
- C. Villarroya-Beltri, C. Gutierrez-Vazquez, F. Sanchez-Cabo, D. Perez-Hernandez, J. Vazquez, N. Martin-Cofreces, D. J. Martinez-Herrera, A. Pascual-Montano, M. Mittelbrunn and F. Sanchez-Madrid, Nat. Commun., 2013, 4, 2980 CrossRef PubMed.
- I. Nakase and S. Futaki, Sci. Rep., 2015, 5, 10112 CrossRef PubMed.
- K. K. Hou, H. Pan, P. H. Schlesinger and S. A. Wickline, Biotechnol. Adv., 2015, 33, 931–940 CrossRef CAS PubMed.
- R. L. Oliveira, P. C. Chagastelles, P. Sesterheim and P. Pranke, Stem Cells Int., 2017, 2017, 9824698 Search PubMed.
- D. Ghosh, P. Venkataramani, S. Nandi and S. Bhattacharjee, Cancer Cell Int., 2019, 19, 12–22 CrossRef PubMed.
- A. Ortiz, Semin. Cancer Biol., 2021, 76, 139–142 CrossRef CAS PubMed.
- O. M. Elsharkasy, J. Z. Nordin, D. W. Hagey, O. G. de Jong, R. M. Schiffelers, S. E. L. Andaloussi and P. Vader, Adv. Drug Delivery Rev., 2020, 159, 332–343 CrossRef CAS PubMed.
- D. Zhang, A. Hussain, H. Manghwar, K. Xie, S. Xie, S. Zhao, R. M. Larkin, P. Qing, S. Jin and F. Ding, Plant Biotechnol. J., 2020, 18, 1651–1669 CrossRef PubMed.
- C. Brokowski and M. Adli, J. Mol. Biol., 2019, 431, 88–101 CrossRef CAS PubMed.
- M. Kessel, B. Rana and C. Boehme, Nat. Biotechnol., 2019, 37, 350–351 CrossRef CAS PubMed.
- C. Zhuo, Y. Tao and M. Li, Signal Transduction Targeted Ther., 2022, 7, 279 CrossRef CAS PubMed.
- J. A. Peruzzi, M. L. Jacobs, T. Q. Vu, K. S. Wang and N. P. Kamat, Angew. Chem., Int. Ed., 2019, 58, 18683–18690 CrossRef CAS PubMed.
- Y. Cai, W. Ran, Y. Zhai, J. Wang, C. Zheng, Y. Li and P. Zhang, Biomater. Sci., 2020, 8, 1045–1057 RSC.
- A. Pitchaimani, T. D. T. Nguyen and S. Aryal, Biomaterials, 2018, 160, 124–137 CrossRef CAS PubMed.
- O. G. de Jong, D. E. Murphy, I. Mager, E. Willms, A. Garcia-Guerra, J. J. Gitz-Francois, J. Lefferts, D. Gupta, S. C. Steenbeek, J. van Rheenen, S. El Andaloussi, R. M. Schiffelers, M. J. A. Wood and P. Vader, Nat. Commun., 2020, 11, 1113 CrossRef CAS PubMed.
- N. Luo, J. Li, Y. Chen, Y. Xu, Y. Wei, J. Lu and R. Dong, Drug Delivery, 2021, 28, 10–18 CrossRef CAS PubMed.
Footnote |
† These authors contributed equally to the work. |
|
This journal is © The Royal Society of Chemistry 2024 |