DOI:
10.1039/D3BM01746J
(Paper)
Biomater. Sci., 2024,
12, 1490-1501
Preparation of glycopeptide-modified pH-sensitive liposomes for promoting antigen cross-presentation and induction of antigen-specific cellular immunity†
Received
26th October 2023
, Accepted 4th February 2024
First published on 5th February 2024
Abstract
Cross-presentation, exogenous antigen presentation onto major histocompatibility complex class I molecules on antigen presenting cells, is crucially important for inducing antigen-specific cellular immune responses for cancer immunotherapy and for the treatment of infectious diseases. One strategy to induce cross-presentation is cytosolic delivery of an exogenous antigen using fusogenic or endosomolytic molecule-introduced nanocarriers. Earlier, we reported liposomes modified with pH-responsive polymers to achieve cytosolic delivery of an antigen. Polyglycidol-based or polysaccharide-based pH-responsive polymers can provide liposomes with delivery performance of antigenic proteins into cytosol via membrane fusion with endosomes responding to acidic pH, leading to induction of cross-presentation. Mannose residue was introduced to pH-responsive polysaccharides to increase uptake selectivity to antigen presenting cells and to improve cross-presentation efficiency. However, direct introduction of mannose residue into pH-responsive polysaccharides suppressed cytoplasmic delivery performance of liposomes. To avoid such interference, for this study, mannose-containing glycans were incorporated separately into pH-responsive polysaccharide-modified liposomes. Soybean agglutinin-derived glycopeptide was used as a ligand for lectins on antigen presenting cells. Incorporation of glycopeptide significantly increased the cellular uptake of liposomes by dendritic cell lines and increased cross-presentation efficiency. Liposomes incorporated both glycopeptide and pH-responsive polysaccharides exhibited strong adjuvant effects in vitro and induced the increase of dendritic cells, M1 macrophages, and effector T cells in the spleen. Subcutaneous administration of these liposomes induced antigen-specific cellular immunity, resulting in strong therapeutic effects in tumor-bearing mice. These results suggest that separate incorporation of glycopeptides and pH-responsive polysaccharides into antigen-loaded liposomes is an effective strategy to produce liposome-based nanovaccines to achieve antigen cross-presentation and induction of cellular immunity towards cancer immunotherapy.
1. Introduction
Immune systems can recognize pathogens or abnormal cells within the body and can eliminate them via specific mechanisms. By using and/or activating such specific mechanisms, effective treatment modalities and prophylactic vaccines have been developed for cancer, infectious diseases, and other immunity-relating diseases.1–3 In addition to conventional protein-based vaccines, mRNA-based vaccines emerged during the coronavirus pandemic.4–6 In the case of mRNA-based COVID-19 vaccines, mRNA molecules are encapsulated in lipid-based nanoparticles to protect them from degradation and to deliver them into intracellular spaces for mRNA translation to antigenic proteins. These lipid-based nanoparticles are also regarded as acting as an adjuvant by damaging or killing the cells at injection sites to release damage-associated molecular patterns (DAMPs).7,8 Such DAMP release is expected to promote antigen-specific immune responses further after vaccination.
For vaccination, the production of antigen-specific antibodies is crucially important for protection against infection by pathogens. It is induced by the presentation of an antigen onto major histocompatibility complex (MHC) class II molecules of antigen presenting cells (APCs). By contrast, cellular immune responses must occur to eliminate infected cells or cancerous cells within the body, which are induced by the presentation of an antigen onto MHC class I molecules. Generally, exogenous antigenic proteins are internalized into APCs via endocytosis. They are degraded within the endosome/lysosome, which engenders antigen presentation onto MHC class II and induction of humoral immunity. For the presentation of an exogenous antigen onto MHC class I, a phenomenon designated as cross-presentation, control of the uptake mode and the intracellular fate of antigen are extremely important.9–11 Cytosolic antigen delivery, antigen delivery into weakly acidic intracellular compartments, induction of specific receptor-mediated antigen uptake, and other antigen delivery routes have been investigated for the induction of cross-presentation of an exogenous antigen.12–15 However, the comprehensive picture of cross-presentation, including the relation between antigen delivery routes and cross-presentation efficacy, detailed molecular mechanisms, and cell phenotype dependence, remains unclear. Therefore, the collection of basic research data related to cross-presentation using various antigen carriers is necessary for designing efficient antigen delivery systems to induce antigen-specific cellular immunity.
As explained above, cytosolic delivery of antigen is an effective approach to induce cross-presentation because an antigen delivered into cytosol would behave as an endogenous antigen.10–12 To date, various antigen delivery systems have been reported as promoting the cytosolic delivery of an antigen, such as virus-derived fusogenic protein-introduced liposomes, cell-penetrating peptide-introduced carriers, and endosomolytic molecule-introduced carriers.16–19 The authors have also reported several antigen delivery systems using liposomes modified with pH-responsive polymers.20 Carboxylated polyglycidol-modified liposomes have delivered model antigenic protein (ovalbumin, OVA) successfully into cytosol of murine dendritic cell line or bone marrow-derived dendritic cells, resulting in induction of cross-presentation of OVA.21 Polysaccharides such as dextran or β-glucans can also be used as a backbone of pH-responsive polymers. pKa of carboxy groups for these polysaccharide derivatives is 6–6.5, indicating that carboxy groups are deprotonated in physiological conditions but are protonated in endosomal acidic pH conditions. After protonation of carboxy groups, these polysaccharide derivatives form hydrophobic domains, which could destabilize co-existing liposomal membranes only at acidic pH.22–24 These polysaccharide-based pH-sensitive polymer-modified liposomes induced fusion with endosomal membrane, resulting in successful cytosolic delivery of OVA and induction of OVA-specific cellular immune responses in vivo.22–24 However, the cross-presentation efficiency of these polysaccharide derivative-modified liposomes is still moderate. To improve cross-presentation efficiency, mannose residues were introduced into carboxylated polysaccharide derivatives to promote their uptake selectivity to APCs and mannose receptor (MR)-mediated cross-presentation.25 The mannose residue introduction increased the uptake selectivity considerably, but cross-presentation efficiency was not so improved, probably because the introduction of mannose residue into polysaccharide derivatives might have decreased the cytosolic delivery performance.25
Considering the findings obtained from those earlier studies, in this study, a pH-responsive polysaccharide derivative and a glycopeptide containing several mannose residues were introduced separately to antigen-loaded liposomes to balance the promotion of cell uptake via lectins and the cytosolic antigen delivery performance of pH-responsive polysaccharide derivatives (Fig. 1). Here, soybean agglutinin (SBA)-derived glycopeptide (Man9GlcNAc2-Asn) was selected as a ligand for lectins expressing on APCs. Man9GlcNAc2-Asn from SBA is a homogenous ligand used to study the binding of C-type lectins, DC-SIGN and DC-SIGNR. Both receptors bind to N-linked high mannose oligosaccharide and the highest level of binding was found for Man9GlcNAc2-Asn-glycopeptide which decreased with smaller glycans.26 Binding affinity of Man9GlcNAc2 glycopeptide to DC-SIGN was found to be more than 50-fold higher than mannose.27 Glycan array screening data showed that Man9 and other high mannose oligosaccharides are the best ligands for langerin, another C-type lectin.28 A solid-phase binding competition assay also showed C-type carbohydrate recognition domain 4 (CRD4) of MR binds Man9 oligosaccharide with more than 40-fold higher affinity than mannose.29 These studies indicate the potential of Man9GlcNAc2 as an ideal ligand for targeting these lectins on APCs.26–31 For the present study, the effects of SBA glycopeptide and pH-responsive dextran derivative modification to liposomes on cell uptake specificity, cross-presentation efficiency, and adjuvant effect were investigated. Furthermore, the performances of these liposomes for induction of antigen-specific immune responses were evaluated, as were their therapeutic effects on tumor-bearing mice.
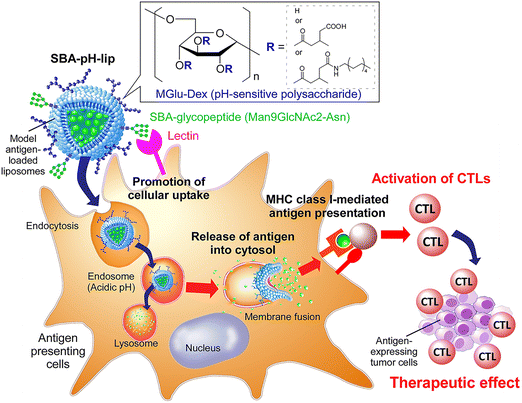 |
| Fig. 1 Soybean agglutinin-derived glycopeptide-introduced pH-responsive polysaccharide-modified liposomes promote the cross-presentation and induction of antigen-specific cellular immune responses. | |
2. Materials and methods
2.1. Materials
Egg yolk phosphatidylcholine (EYPC) was kindly donated by NOF Co. (Tokyo, Japan). 1,2-Dipalmitoyl-sn-glycero-3-phosphothioethanol (DPPTE) was bought from Avanti Polar Lipids (Birmingham, AL, USA). OVA and fetal bovine serum (FBS) were purchased from Sigma-Aldrich (St Louis, MO). Phospholipid C test-Wako was from Wako Pure Chemical Industries Ltd (Osaka, Japan). 1,1′-Dioctadecyl-3,3,3′,3′-tetramethylindocarbocyanine perchlorate (DiI) was from Life Technologies (Carlsbad, CA). N-[(4-Maleimidomethyl)cyclohexyl carbonyloxy]sulfosuccinimide, sodium salt (sulfo-SMCC) was obtained from Dojindo Laboratories (Kumamoto, Japan). Tween20 and lipopolysaccharide (LPS) were from nacalai tesque (Kyoto, Japan). Bio-Gel P-100, Bio-Gel P-4 and Precision Plus Protein™ Dual Color Standards were from Bio-Rad, USA. Pronase was from Roche, Germany. Coomassie (Bradford) protein assay kit was from Thermo Fisher Scientific K.K. (Tokyo, Japan). pH-Responsive dextran derivative with carboxylated unit: 58% and anchor unit: 8% (MGlu58-Dex-A8) was synthesized as previously described.24 Soybean agglutinin was purified as described previously using affinity chromatography on lactosylamine-Bio-Gel P-100 column.32,33 For the preparation of SBA glycopeptide Man9GlcNAc2-Asn, denatured affinity purified soybean agglutinin was digested with Pronase and glycopeptide separated on Bio-Gel P-4 as reported previously.34,35 Free amino group contents in SBA aqueous solution were determined by a fluorescamine assay.
2.2. Preparation of liposomes
EYPC (20 mg) and DPPTE (0.996 mg, 5 mol%) dissolved in 3 mL of chloroform was added to a 10 mL round-bottom flask. After evaporation of chloroform, MGlu58-Dex-A8 (9 mg, lipids/polymer = 7/3, w/w) dissolved in 0.9 mL of methanol were added to the flask and the solvent was evaporated. The remaining organic solvent was further removed under vacuum for >2 h. Obtained mixed thin film (total lipids: 1.25 × 10−5 mol) was hydrated by 4 mg mL−1 OVA in phosphate-buffered saline (PBS) by 2 min-sonication using a bath-type ultrasonic cleaner (AS ONE, ASU-10). The liposome suspension was further treated by 5 times cycles of freezing and thawing and was extruded 31 times through a polycarbonate membrane with a pore size of 100 nm. The liposome suspension was centrifuged (163
383g, 1 h) at 4 °C to remove unencapsulated OVA. For conjugation of SBA glycopeptide onto the liposomes, 5 equiv. of sulfo-SMCC was reacted with amino groups within SBA glycopeptide for 2 h at room temperature, then reaction mixture was purified by G-25 column. Subsequently, purified maleimide group-introduced SBA glycopeptides were mixed with DPPTE-introduced liposomes and were incubated for 24 h at 4 °C. The liposome suspension was centrifuged (163
383g, 1 h, twice) at 4 °C to remove unconjugated SBA glycopeptide.
2.3. Characterization of liposomes
Diameters and zeta potentials of the liposomes (0.1 mM of lipid) were measured using a Zetasizer Nano ZS (Malvern Instruments Ltd, Worcestershire, UK). Data were obtained as an average of more than three measurements on different samples. The concentrations of lipid and OVA in liposome suspension were measured using phospholipid C test-Wako and Coomassie protein assay reagent, respectively.
2.4. Cellular association of liposomes
DiI-labeled liposomes were prepared as described above except that a mixed thin film containing DiI (0.1 mol% for total lipids) was dispersed in PBS containing OVA. DC2.4 cells, a murine dendritic cell line, (1.5 × 105 cells) cultured for 2 days in a 12-well plate were washed twice with PBS and then incubated in serum-free medium (0.95 mL). DiI-labeled liposomes (6 mM lipid concentration, 0.05 mL) were added gently to the cells and then incubated for 4 h at 37 °C. After incubation, the cells were washed with PBS three times. Fluorescence intensity of the cells was determined via a flow cytometric analysis (CytoFLEX, Beckman Coulter, Inc.). Fluorescence intensity for untreated cells was subtracted from each data to remove autofluorescence of cells. DiI-derived fluorescence intensity per lipid for each liposome was also measured using a spectrofluorometer and was compensated.
2.5. Confocal laser scanning microcopic analysis
DC2.4 cells (3 × 105 cells) cultured for 2 days in a glass-bottom dish were washed twice with PBS and then incubated in a serum-free medium (1.9 mL). DiI-labeled liposomes (6 mM lipid concentration, 0.1 mL) were added gently to the cells and incubated for 5 h at 37 °C. After incubation, the cells were washed with PBS three times and observed using a confocal laser scanning microscopy LSM5 EXCITER (Carl Zeiss).
2.6. Evaluation of antigen presentation
DC2.4 cells (1.5 × 105 cells) cultured for 2 days in a 6-well plate were washed with PBS twice and then incubated in culture medium. OVA-loaded liposomes (final OVA concentration: 30 μg mL−1) or OVA class I epitope peptide (SIINFEKL) were added gently to the cells, followed by incubation for 24 h at 37 °C. After incubation, the cells were transferred to the round-bottom 96-well plate and then washed with 2% FBS-containing PBS (FACS buffer) twice. The cells were treated with anti-mouse CD16/32 antibody (0.5 μg/0.1 mL, eBioscience, 93) for 30 min on ice to block Fc receptors. After three times washing by FACS buffer, cells were further incubated with biotinylated anti-mouse OVA254–264 (SIINFEKL) peptide bound to H-2Kb (0.125 μg/0.1 mL, eBioscience, 25-D1.16) for 30 min on ice. After three times washing by FACS buffer, cells were stained with streptavidin-PE (Sigma, St Louis, MO, USA) for 30 min on ice. After three times washing by FACS buffer, cellular fluorescence was detected by a flow cytometer (CytoFLEX, Beckman Coulter, Inc.).
2.7. Cytokine production
DC2.4 cells (2 × 104 cells) cultured for 2 days in a 96-well plate were washed with PBS twice and then incubated in serum-free medium. OVA-loaded liposomes (final lipid concentration: 0.1 mM) or SBA glycopeptide (0.1 mg mL−1) were added gently to the cells, followed by incubation for 36 h at 37 °C. After incubation, plates were centrifuged (1500 rpm, 5 min) to remove cell debris and the supernatants were collected. Cytokine production in the supernatants was measured using mouse uncoated ELISA kit (for TNF-α, IL-1β or IL-12, Invitrogen) according to the manufacturer's instruction.
2.8. Animals
Seven-week-old female C57BL/6 mice (H-2b) were purchased from Oriental Yeast Co., Ltd (Tokyo, Japan). All animal experiments were approved by the Institutional animal experimentation committee in Osaka Metropolitan University (approval no. 22-1) and were performed in compliance with the institutional guidelines of animal care and use.
2.9. Analysis of immune cell composition in the spleen and in vitro stimulation of splenocytes
On days 7 and 14, 50 μg of OVA-loaded liposomes were subcutaneously injected to C57BL/6 mice under anesthesia with isoflurane. After 24 h of each injection, spleen was harvested from the mice and single cell suspension of the spleen was prepared by gentle mashing and passing through a 70 μm mesh Cellstrainer™ (Falcon®). Erythrocytes were removed by incubating the cell pellet for 5 min in RBC lysis buffer (pluriSelect Life Science UG & Co. KG) at room temperature. Single cells from spleen were seeded into 96-well plates with 1.0 × 106 cells per well and washed by FACS buffer. Antibodies against the following molecules were used with a given combination of fluorescence-labeling: B220 (RA3-6B2, BioLegend), CD3ε (145-2C11, BD Biosciences), CD4 (RM4-5, BD Biosciences), CD8α (53-6.7, BD Biosciences), CD11b (M1/70, BioLegend), CD11c (N418, BioLegend), CD44 (IM7, BD Biosciences), CD45 (30-F11, BD Biosciences), CD62L (MEL-14, BD Biosciences), CD206 (C068C2, BioLegend), F4/80 (BM8, BioLegend), MHC II (AF6-120.1, BioLegend). Staining was carried out on ice for 20 min (each diluted 1
:
200 in FACS buffer). After washing twice, cell populations were analyzed via a flow cytometric analysis (CytoFLEX, Beckman Coulter, Inc.) using a CytoExpert software.
Single cells from spleen (2 × 106 per 2 mL) were incubated with various concentrations of OVA solution (0, 50, 100 μg mL−1) for 4 days. After the incubation, the concentration of IFN-γ was measured using mouse uncoated ELISA kit (for IFN-γ, Invitrogen) according to the manufacturer's instruction.
2.10. Induction of antitumor immunity
E.G7-OVA cells, OVA-expressing T-lymphoma, (5 × 105 cells per mouse) were subcutaneously inoculated into the left back of C57BL/6 mice under anesthesia with isoflurane. On days 7 and 14, 50 μg of OVA-loaded liposomes were injected subcutaneously into the right backs of the mice under anesthesia with isoflurane. Tumor sizes were monitored from the day of tumor inoculation. Mice immunized with PBS were used as a control to confirm the development of tumors following the first inoculation of E.G7-OVA cells. Mice were sacrificed when tumor volumes became over 2000 mm3. All treated groups contained 5 mice.
2.11. Statistical analysis
Statistically significant differences between experimental groups were determined using Prism software (v9, GraphPad). Where one-way ANOVA followed by Tukey's HSD post hoc test was used, variance between groups was found to be similar by Brown–Forsythe test. A log-rank test was employed for analyzing mouse survival. The symbols *, **, ***, and **** indicate P values less than 0.05, 0.01, 0.001, and 0.0001, respectively.
3. Results and discussion
3.1. Preparing glycopeptide derivative-modified and polysaccharide derivative-modified liposomes
Model antigenic protein-loaded liposomes modified with both pH-responsive polysaccharide derivatives and SBA-derived glycopeptides were prepared by the hydration of a mixed thin film composed of lipids and polysaccharide derivatives and subsequent extrusion to adjust the liposome size. As a lipid component, DPPTE was incorporated to present thiol groups on the liposome surface. Amino groups in SBA glycopeptide were reacted with sulfo-SMCC for the modification of maleimide groups. Maleimide-introduced SBA glycopeptides were conjugated with liposomal surface via thiol–maleimide reaction. The prepared liposome sizes were approximately 90–110 nm (Fig. 2A), which corresponds to the pore size of the polycarbonate membrane during extrusion. Their polydispersity index values were around 0.10 (Fig. 2B), indicating that these liposomes have a narrow size distribution irrespective of their modification of the pH-responsive polysaccharide derivative or glycopeptides. Modification of pH-responsive polysaccharide derivatives decreased the zeta potential of the liposomes (Fig. 2C), suggesting modification of the carboxylated polymers on the liposomal surface. Model antigenic protein (OVA) loading amounts and loading efficiencies were approximately 80–100 g mol−1 lipids and 20–25% (Fig. 2D), which are almost identical to findings reported from our earlier study.23 These data suggest the successful preparation of antigen-loaded liposomes. To confirm that the pH-responsive property of these liposomes is retained after SBA glycopeptide modification, liposomes encapsulating a fluorescent dye (pyranine) and its quencher (p-xylene-bis-pyridinium bromide) were prepared. As shown in Fig. S1,† liposomes stably retained the contents at pH 7.4. In contrast, remarkable pyranine leakage from liposomes was observed below pH 6.5. At pH 5.5, which corresponds to endosomal pH, almost 100% of the contents were released within 30 min. These results indicate that pH-responsive polysaccharide derivative (MGlu58-Dex-A8) on the liposome surface does not interact with liposomal membrane at a physiological condition, whereas protonated MGlu58-Dex-A8 at acidic condition could disrupt liposomal membrane immediately. In addition, SBA modification did not interfere pH-responsiveness of polysaccharide derivatives.
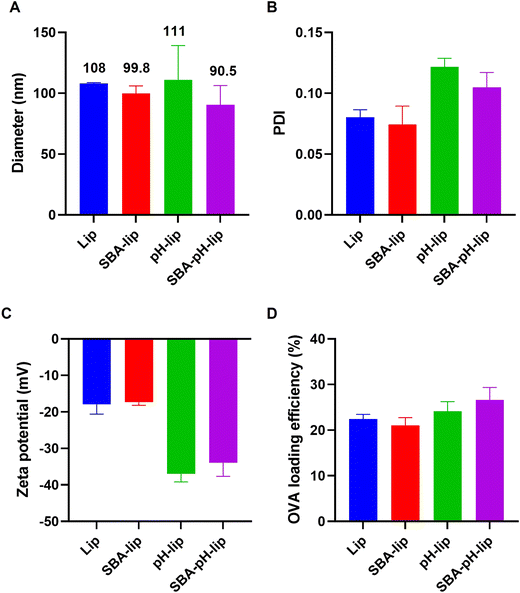 |
| Fig. 2 (A) Diameter, (B) polydispersity index (PDI), (C) zeta potential and OVA-loading efficiency (D) of egg PC liposomes modified with and without SBA and/or pH-sensitive polysaccharide derivative (MGlu58-Dex-A8). Each bar shows the mean ± SEM (n = 3). | |
3.2. Glycopeptide incorporation effects on antigen delivery and cross-presentation performance of liposomes
Next, the effects of modification of polysaccharide derivatives and/or SBA glycopeptide on cellular association of liposomes were investigated. DiI-labeled liposomes were applied to a murine dendritic cell line (DC2.4 cell). Then the cell fluorescence and the intracellular distribution of liposomes were measured respectively using a flow cytometer and confocal laser scanning microscopy (CLSM) (Fig. 3). For SBA glycopeptide-modified liposomes (SBA-lip), the average fluorescence intensity was slightly high but it was almost equal to that of cells treated with unmodified liposomes. By contrast, pH-responsive polysaccharide modification (pH-lip) showed significantly increased cellular association of liposomes with dendritic cell lines, which might result from the recognition of carboxylates on the liposome surface by scavenger receptors.21,36–38 Moreover, both modification of SBA glycopeptides and pH-responsive polysaccharide derivatives (SBA-pH-lip) further enhanced the cellular association of the liposomes. This tendency was also confirmed using CLSM analysis (Fig. 3B). Internalization of SBA-pH-lip within cells was evaluated further using a Z-stacked image (Fig. S2†). To analyze the effect of lectin on the cellular association of these liposomes, cellular association of liposomes in the presence of excess free SBA was evaluated (Fig. S3†). Cellular association of SBA-lip was slightly decreased in the presence of free SBA (Fig. S3A,†P = 0.10). Considering that the SBA glycopeptide maximum density on the liposome surface derived from DPPTE content (5%) is not so high, single introduction of SBA glycopeptide onto the liposomes is not so effective to promote the interaction of glycans with lectins on the dendritic cell surface. In contrast, cellular association of SBA-pH-lip was significantly reduced in the presence of excess SBA (Fig. S3B†), suggesting the recognition of SBA-pH-lip by lectins. The high density of carboxylates and high molecular weight (approx. 70 kDa of dextran main chain) of pH-responsive polysaccharide on the liposomes might support the approach of liposomes on the surface of dendritic cell lines via multivalent interactions. In turn, that approach might promote the access of mannose residues within SBA glycopeptide into binding sites of lectins. Thus, both modification of SBA glycopeptide and pH-responsive polysaccharide might cooperatively promote the cellular association of liposomes via lectin binding and recognition by scavenger receptors.
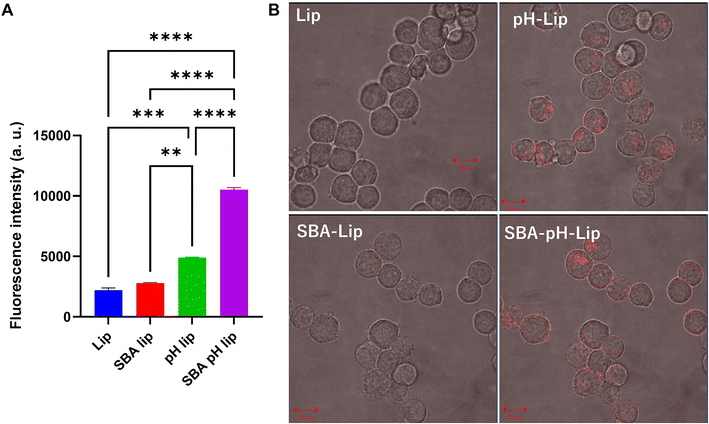 |
| Fig. 3 (A) Relative fluorescence intensity of DC2.4 cells treated with various DiI-labeled liposomes for 4 h (0.3 mM lipid concentration). Fluorescence intensity for the untreated cells was subtracted. Statistical analyses were done using analysis of variance (ANOVA) with Tukey's test: **P < 0.01, ***P < 0.001 and ****P < 0.0001. (B) Confocal laser scanning microscopic (CLSM) images of DC2.4 cells treated with DiI-labeled liposomes. DC2.4 cells were incubated in the presence of liposomes (0.3 mM lipids) for 5 h at 37 °C. The intracellular distribution of lipids (red) was found using CLSM. Scale bars = 10 μm. | |
Antigen cross-presentation, which is a phenomenon by which an exogenous antigen is presented onto MHC class I molecules of APCs,9–11 is necessary for inducing cellular immune responses. After DC2.4 cells were incubated with OVA-loaded liposomes, the cross-presentation capabilities of various liposomes were evaluated using a specific antibody that recognizes OVA class I epitope peptide (SIINFEKL)/MHC class I complexes. As Fig. 4 shows, modification of SBA glycopeptide and/or pH-responsive polysaccharide derivatives exhibited higher percentages and higher fluorescence intensity of SIINFEKL/MHC-I complex presentation than those of unmodified liposome-treated cells. Especially, SBA-pH-lip showed a significantly higher cross-presentation property than unmodified liposomes about both percentage and fluorescence intensity. As reported in earlier reports,21–24 this finding might be attributable to enhanced cellular association of SBA-pH-lip (Fig. 3) and cytosolic release of OVA molecules the via pH-responsive endosomal membrane disruption property of the pH-responsive polysaccharide derivative. Therefore, these results suggest that double modification of SBA glycopeptide and pH-responsive polysaccharide derivative is an efficient approach to promote antigen cross-presentation.
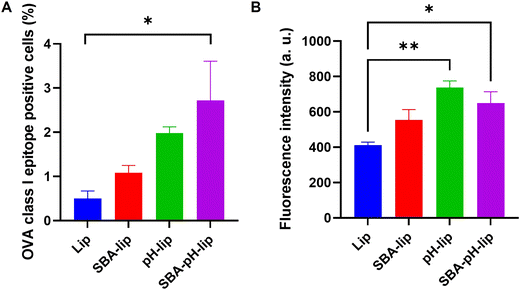 |
| Fig. 4 (A) Percentage and (B) fluorescence intensity of MHC class I/OVA epitope peptide (SIINFEKL) complex-expressing DC2.4 cells treated with various OVA-loaded liposomes modified with or without SBA and/or pH-sensitive polysaccharides. Statistical analysis was done using ANOVA with Tukey's test: *P < 0.05 and **P < 0.01 (n = 3). | |
3.3. Adjuvant property of glycopeptide derivative-modified and polysaccharide derivative-modified liposomes
Not only antigen delivery performance, but also the adjuvant property of antigen carriers is important for inducing antigen-specific immune responses.39,40 Various toll-like receptor (TLR) ligands such as monophosphoryl lipid A, CpG-DNA, and other adjuvant molecules can be incorporated into the liposomes to provide the adjuvant property. Reportedly, pH-responsive polysaccharide derivatives themselves also have adjuvant properties that can induce pro-inflammatory cytokine production from dendritic cells or macrophages.22,41 In addition, SBA glycopeptide itself can induce cytokine production from a dendritic cell line (Fig. 5A–C). Here, the effects of SBA glycopeptide and/or pH-responsive polysaccharide modification on the adjuvant property of the liposomes were investigated by measuring inflammatory cytokine production from the dendritic cell line. As expected, both SBA-lip and SBA-pH-lip induced significantly higher TNF-α and IL-6 productions from DC2.4 cells than PBS-treated cells did, which might result from the adjuvant property of SBA glycopeptide. For IL-1β and IL-12, pH-lip and SBA-pH-lip promoted the secretion of these pro-inflammatory cytokines. IL-1β production is known to be promoted by the activation of inflammasomes through intracellular release of the contents within a lysosome such as cathepsin.42 Both pH-lip and SBA-pH-lip exhibited pH-responsive content release property (Fig. S1†). In addition, pH-responsive polysaccharide derivatives on the liposomes can promote the cytosol release of liposomal contents via destabilization of endosomal and lysosomal membranes after protonation of carboxylates within these weakly acidic intracellular compartments.21,22 For that reason, modification of pH-responsive polysaccharide derivatives might also promote an inflammasome-pathway to promote the secretion of IL-1β and/or other inflammatory cytokines. IL-12 secretion is known to be promoted TLR pathways.43 Considering that adjuvant effect of pH-responsive polysaccharides is inhibited by TLR4 agonist (data not shown), pH-lip might induce TLR4-mediated IL-12 secretion from DC2.4 cells. In addition, SBA glycopeptide induced TNF-α and IL-6 productions (Fig. 5A and C), suggesting the recognition of SBA glycopeptide via TLR4. High cellular association of SBA-pH-lip (Fig. 3A) might promote TLR4 recognition of SBA glycopeptide on the liposomes, leading to significantly higher IL-12 production from DC2.4 cells. Thus, double modification of SBA and pH-responsive polysaccharide derivatives onto the liposome would be an effective approach to obtain the synergy of adjuvant effects derived from each component and thereby promote pro-inflammatory cytokines of various kinds.
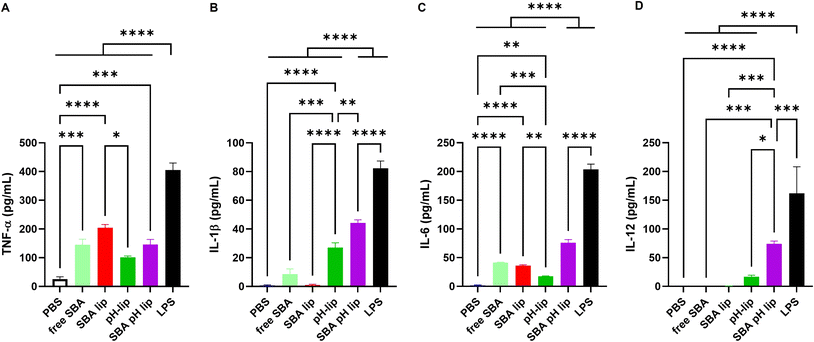 |
| Fig. 5 (A) TNF-α, (B) IL-1β, (C) IL-6, or (D) IL-12 production from DC2.4 cells treated for 36 h with various liposomes (0.1 mM lipids). Free SBA (0.1 mg mL−1) and LPS (5 μg mL−1) were used as controls. Statistical analyses were done using ANOVA with Tukey's test: *P < 0.05, **P < 0.01, ***P < 0.001, and ****P < 0.0001. | |
3.4. Effects of subcutaneous injection of liposomes on immune cell populations in the spleen
Considering the highly adjuvant effect of SBA-pH-lip, we investigated the change in immune cell population in the spleen after liposome injection. First, OVA-loaded various liposomes were administered subcutaneously to the mice once a week. After 24 h following the second injection, the spleen of each was harvested. Single spleen cells were stained with various antibodies to bind specific surface markers of each immune cell population. Fig. S4† portrays results for dendritic cells, monocytes, and macrophage populations. Injection of pH-lip and SBA-pH-lip increased dendritic cells significantly within the spleen (Fig. S4A†), which might reflect the strong adjuvant effects of these liposomes. The percentages of monocytes and macrophages were not altered after liposome injection (Fig. S4B and S4C†), but all liposomes significantly increased M1 macrophages and decreased M2 macrophages (Fig. S4D–S4F†). Considering that these liposomes promoted TNF-α production from the dendritic cell line (Fig. 5A), liposomes of all types might polarize macrophage populations effectively into immunity-activating M1 phenotypes in vivo. Fig. S5† presents changes in the T cell and B cell populations after liposome injection. Whole T cell population tended to decrease (Fig. S5A†), and the B cell population tended to increase after injection of pH-lip and SBA-pH-lip (Fig. S5B†). CD4-positive T cells decreased significantly after injection of pH-lip and SBA-pH-lip (Fig. S5D†), but no effect was found on the CD8/CD4 ratio in the spleen by liposome injection (Fig. S5E†). As shown in Fig. 6, change in the T-cell subpopulation was evaluated further. For CD8-positive T cell populations, central memory T cells tended to decrease after liposome injection, but administration of pH-lip and SBA-pH-lip significantly increased the percentages of effector T cells in the spleen (Fig. 6B–D), suggesting that cellular immune responses were induced by the efficient cross-presentation capability and highly adjuvant effects of these liposomes. In the case of CD4-positive T cell populations, naïve T cells were decreased significantly (Fig. 6E), which might correspond to the decrease of CD4-positive cells within T cells, as shown in Fig. S5D.† Injection of liposomes, especially SBA-pH-lip, significantly increased both central memory and effector T cells (Fig. 6F and G). These findings suggest that SBA-pH-lip can induce not only CD8-positive T cell-based cellular immune responses but also CD4-positive T cell-based humoral immune responses.
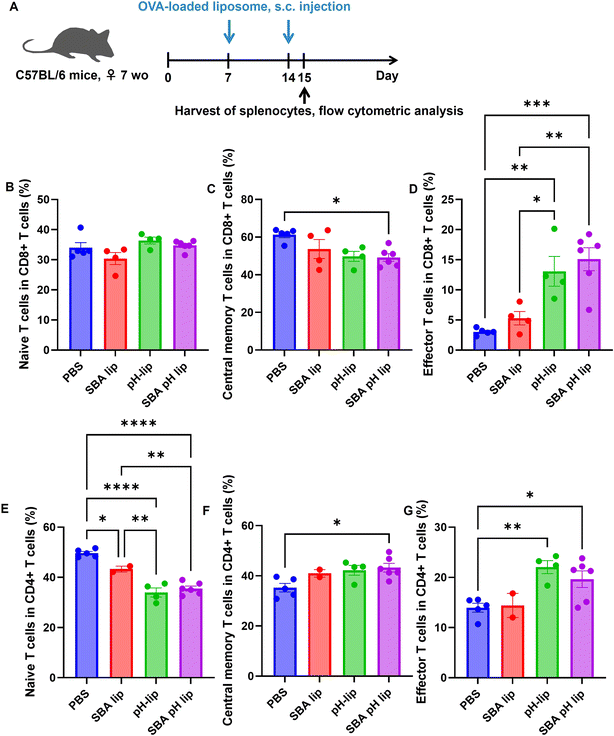 |
| Fig. 6 Effects of subcutaneous injection of liposomes on T cell populations in the spleen. (A) Experimental schedule. Mice were injected subcutaneously with PBS or liposomes on days 7 and 14. Single cells were extracted from the spleen on day 15, followed by flow cytometric analysis. Graphs depict the frequency of (B) naïve CD8+ T cells (CD62L+ CD44−) of CD3+ cells, (C) central memory CD8+ T cells (CD62L+ CD44+) of CD3+ cells, (D) effector memory CD8+ T cells (CD62L− CD44+) of CD3+ cells, (E) naïve CD4+ T cells (CD62L+ CD44−) of CD3+ cells, (F) central memory CD4+ T cells (CD62L+ CD44+) of CD3+ cells, and (G) effector memory CD4+ T cells (CD62L− CD44+) of CD3+ cells (mean ± SEM; n = 4–6). Statistical analyses were conducted using ANOVA with Tukey's test: *P < 0.05, **P < 0.01, ***P < 0.001, and ****P < 0.0001. | |
3.5. Induction of antitumor immunity by glycopeptide derivative-modified and polysaccharide derivative-modified liposomes
To investigate the capabilities of liposomes for induction of antigen-specific immune responses, splenocytes harvested from OVA-loaded liposome-immunized mice were stimulated by OVA during in vitro culture (Fig. 7A). When compared to PBS-treated and SBA-lip-injected groups, pH-lip-treated and SBA-pH-lip-treated groups showed significantly higher IFN-γ secretion by splenocytes only in the presence of antigen stimulation (Fig. 7B). These results indicate that OVA-specific cellular immune responses were induced by the subcutaneous administration of OVA-loaded pH-lip or SBA-pH-lip because these liposomes can enhance effector T cell populations within the spleen (Fig. 6D and G), cytotoxic CD8-positive T cells, and/or Th1 cells secreted IFN-γ in response to OVA stimulation.
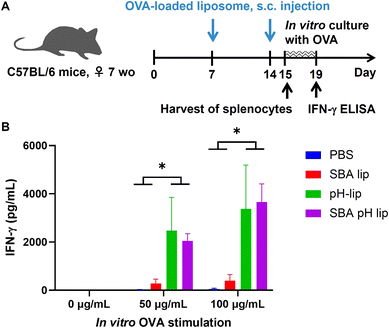 |
| Fig. 7
In vitro stimulation of splenocytes from mice immunized with OVA-loaded liposomes. (A) Experimental schedule. Splenocytes (2 × 106 per 2 mL) isolated from the mice immunized with PBS or various OVA-loaded liposomes twice were incubated with various concentrations of OVA for 4 days. IFN-γ secretion in the supernatant was detected using ELISA (B). Splenocytes were collected at 24 h after final immunization. Statistical analyses were done using ANOVA with Tukey's test *P < 0.05 (n = 5). | |
Finally, antitumor effects of these liposomes on tumor-bearing mice were evaluated. OVA-expressing T lymphoma cells (E.G7-OVA cells) were injected to the mice for the establishment of tumor-bearing mice. On days 7 and 14 after tumor cell inoculation, OVA-loaded liposomes were injected into the mice subcutaneously. Then, tumor growth and survival were monitored (Fig. 8A and S6†). When PBS was injected as a control, tumor volumes grew remarkably. All mice reached the predetermined endpoint tumor volume (2000 mm3) within 25 days. If OVA-loaded SBA-lip was injected into the mice, then tumor growth was suppressed slightly (Fig. 8B and S6†), but the mice survival was not prolonged significantly (Fig. 8C). In fact, SBA-lip can induce immunity-activating M1 macrophages (Fig. S4D†) but not effector T cells in the spleen (Fig. 6D and G). Therefore, cellular immune response induced by SBA-lip injection might be insufficient to suppress tumor growth. By contrast, administration of pH-lip or SBA-pH-lip apparently decreased the tumor volume from 5 days after first injection of these liposomes (Fig. 8B and S6†). Mice survival was also prolonged significantly by these liposomes (Fig. 8C). It is noteworthy that 40% of mice treated with SBA-pH-lip became completely tumor-free. These results are attributable to the effective activation of dendritic cells, M1 macrophages, and effector T cells and to the simultaneous induction of OVA-specific cellular immune responses. To investigate immune responses after subcutaneous injection of liposomes more directly, immune cell populations within tumor were analyzed. Tumor-bearing mice were treated with OVA-loaded liposomes at 7 days after E.G7-OVA cell inoculation. Tumor was excised on day 13 to obtain enough size of tissue even in pH-lip and SBA-pH-lip groups. Pictures of excised tumor was shown in Fig. S7,† suggesting that pH-lip and SBA-pH-lip groups tended to decrease tumor size compared with PBS or SBA-lip groups even on day 13. Single live cells within tumor were stained with antibodies for various cell surface markers and were analyzed using a flow cytometry (Fig. S8–S10†). As almost same with analyses in spleen (Fig. S4, S5† and Fig. 6), the increase in dendritic cell, M1 macrophage and effector CD8+ T cell populations and the decrease in CD4+ T cells were observed in pH-lip or SBA-pH-lip groups compared with PBS or SBA-lip groups. These data suggest that effector CD8+ T cell-based cellular immune responses were effectively induced within tumor in pH-lip or SBA-pH-lip groups. These responses were further supported by dendritic cells and M1 macrophages within tumor microenvironments, resulting in effective tumor growth suppression and extension of mouse survival (Fig. 8). However, the difference in therapeutic effects between pH-lip and SBA-pH-lip was not significant at present experimental setting (Fig. 8). Considering significantly higher cellular association (Fig. 3A), in vitro adjuvant effect (Fig. 5) and in vivo performance of SBA-pH-lip compared with pH-lip, further optimization of SBA glycopeptide conjugation amounts and types of glycopeptides with higher affinity to the lectins will promote the immunity-inducing effect of these liposomes in not only adjuvant property but also in vivo therapeutic effect level.
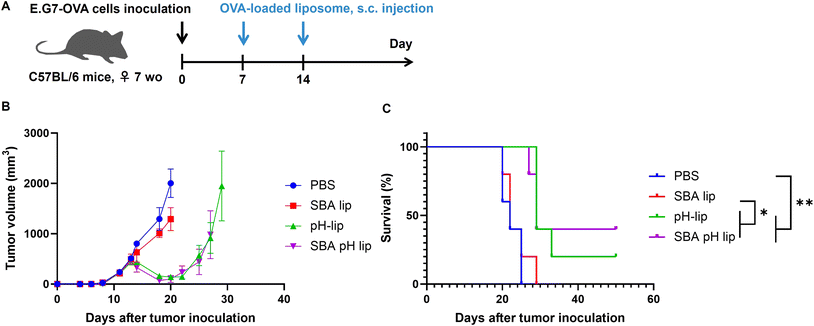 |
| Fig. 8 Antitumor effects induced by various liposomes. (A) Experimental schedule. E.G7-OVA (5 × 105 per mouse) were inoculated subcutaneously into the left backs of C57BL/6 mice. Then the tumor volume (B) and survival of mice (C) were monitored. Mice were injected subcutaneously with 50 μg of OVA-loaded liposomes on days 7 and 14. Mice treated with PBS were used as controls. Statistical analysis was done using Log-rank tests for (B): *P < 0.05 and **P < 0.01 (n = 5). | |
4. Conclusion
To promote the cellular association of liposomes and intracellular delivery of antigenic proteins, SBA-derived glycopeptides and pH-responsive polysaccharide derivatives were modified respectively onto antigen-loaded liposomes. As expected, modification of the SBA glycopeptides enhanced liposome uptake by dendritic cell lines. Further modification of pH-responsive polysaccharide derivatives promoted the cross-presentation of exogenous antigen successfully. These liposomes exhibited a strong adjuvant property, resulting in induction of antigen-specific effector T cells in vivo and exerting highly therapeutic effects on tumor-bearing mice. Therefore, the results presented herein are expected to provide a useful design of glycan and/or lipid-based antigen carriers for the induction of cross-presentation and antigen-specific cellular immune responses for use with cancer immunotherapy or vaccines against infectious diseases.
Author contributions
Eiji Yuba: conceptualization, data curation, formal analysis, funding acquisition, investigation, methodology, project administration, supervision, validation, writing – original draft, writing – review & editing, Rajesh Kumar Gupta: conceptualization, data curation, formal analysis, funding acquisition, investigation, validation, writing – review & editing.
Conflicts of interest
There are no conflicts to declare.
Acknowledgements
This research was funded by the India-Japan collaborative project on DST and JSPS grant number: DST/INT/JSPS/P-307/2020 and JPJSBP120207716. This research was partly supported by JSPS KAKENHI grant number JP21H03822 and JP23H04087. The authors thank Shin Yanagihara (Osaka Prefecture University) for his kind support on liposome preparation.
References
- J. Liu, M. Fu, M. Wang, D. Wan, Y. Wei and X. Wei, Cancer vaccines as promising immuno-therapeutics: platforms and current progress, J. Hematol. Oncol., 2022, 15, 28 CrossRef PubMed.
- S. D. Jazayeri, H. X. Lim, K. Shameli, S. K. Yeap and C. L. Poh, Nano and microparticles as potential oral vaccine carriers and adjuvants against infectious diseases, Front. Pharmacol., 2021, 12, 682286 CrossRef CAS PubMed.
- H. B. Streeter and D. C. Wraith, Manipulating antigen presentation for antigen-specific immunotherapy of autoimmune diseases, Curr. Opin. Immunol., 2021, 70, 75–81 CrossRef CAS PubMed.
- L. Zhang, K. R. More, A. Ojha, C. B. Jackson, B. D. Quinlan, H. Li, W. He, M. Farzan and N. Pardi, Effect of mRNA-LNP components of two globally-marketed COVID-19 vaccines on efficacy and stability, npj Vaccines, 2023, 8, 156 CrossRef CAS PubMed.
- G. T. Szabó, A. J. Mahiny and I. Vlatkovic, COVID-19 mRNA vaccines: Platforms and current developments, Mol. Ther., 2022, 30, 1850–1868 CrossRef PubMed.
- R. Tenchov, R. Bird, A. E. Curtze and Q. Zhou, Lipid nanoparticles-from liposomes to mRNA vaccine delivery, a landscape of research diversity and advancement, ACS Nano, 2021, 15, 16982–17015 CrossRef CAS PubMed.
- H. Zhang, X. You, X. Wang, L. Cui, Z. Wang, F. Xu, M. Li, Z. Yang, J. Liu, P. Huang, Y. Kang, J. Wu and X. Xia, Delivery of mRNA vaccine with a lipid-like material potentiates antitumor efficacy through Toll-like receptor 4 signaling, Proc. Natl. Acad. Sci. U. S. A., 2021, 118, e2005191118 CrossRef CAS PubMed.
- K. Kobiyama and K. J. Ishii, Making innate sense of mRNA vaccine adjuvanticity, Nat. Immunol., 2022, 23, 474–476 CrossRef CAS PubMed.
- K. L. Rock and L. Shen, Cross-presentation: underlying mechanisms and role in immune surveillance, Immunol. Rev., 2005, 207, 166–183 CrossRef CAS PubMed.
- O. P. Joffre, E. Segura, A. Savina and S. Amigorena, Cross-presentation by dendritic cells, Nat. Rev. Immunol., 2012, 12, 557–569 CrossRef CAS PubMed.
- M. Embgenbroich and S. Burgdorf, Current concepts of antigen cross-presentation, Front. Immunol., 2018, 9, 1643 CrossRef PubMed.
- M. Gros and S. Amigorena, Regulation of antigen export to the cytosol during cross-presentation, Front. Immunol., 2019, 10, 41 CrossRef CAS PubMed.
- C. Hotta, H. Fujimaki, M. Yoshinari, M. Nakazawa and M. Minami, The delivery of an antigen from the endocytic compartment into the cytosol for cross-presentation is restricted to early immature dendritic cells, Immunology, 2006, 117, 97–107 CrossRef CAS PubMed.
- S. Burgdorf, V. Lukacs-Kornek and C. Kurts, The mannose receptor mediates uptake of soluble but not of cell-associated antigen for cross-presentation, J. Immunol., 2006, 176, 6770–6776 CrossRef CAS PubMed.
- H. J. P. van der Zande, D. Nitsche, L. Schlautmann, B. Guigas and S. Burgdorf, The mannose receptor: from endocytic receptor and biomarker to regulator of (meta)inflammation, Front. Immunol., 2021, 12, 765034 CrossRef CAS PubMed.
- L. Bungener, K. Serre, L. Bijl, L. Leserman, J. Wilschut, T. Daemen and P. Machy, Virosome-mediated delivery of protein antigens to dendritic cells, Vaccine, 2002, 20, 2287–2295 CrossRef CAS PubMed.
- T. Nakamura, R. Moriguchi, K. Kogure, N. Shastri and H. Harashima, Efficient MHC class I presentation by controlled intracellular trafficking of antigens in octaarginine-modified liposomes, Mol. Ther., 2008, 16, 1507–1514 CrossRef CAS PubMed.
- K. Song, D. C. Nguyen, T. Luu, O. Yazdani, D. Roy, P. S. Stayton and S. H. Pun, A mannosylated polymer with endosomal release properties for peptide antigen delivery, J. Controlled Release, 2023, 356, 232–241 CrossRef CAS PubMed.
- J. T. Wilson, S. Keller, M. J. Manganiello, C. Cheng, C.-C. Lee, C. Opara, A. Convertine and P. S. Stayton, pH-Responsive nanoparticle vaccines for dual-delivery of antigens and immunostimulatory oligonucleotides, ACS Nano, 2013, 7, 3912–3925 CrossRef CAS PubMed.
- E. Yuba, Development of functional liposomes by modification of stimuli-responsive materials and their biomedical applications, J. Mater. Chem. B, 2020, 8, 1093–1107 RSC.
- E. Yuba, A. Harada, Y. Sakanishi, S. Watarai and K. Kono, A liposome-based antigen delivery system using pH-sensitive fusogenic polymers for cancer immunotherapy, Biomaterials, 2013, 34, 3042–3052 CrossRef CAS PubMed.
- E. Yuba, A. Yamaguchi, Y. Yoshizaki, A. Harada and K. Kono, Bioactive polysaccharide-based pH-sensitive polymers for cytoplasmic delivery of antigen and activation of antigen-specific immunity, Biomaterials, 2017, 120, 32–45 CrossRef CAS PubMed.
- S. Yanagihara, N. Kasho, K. Sasaki, N. Shironaka, Y. Kitayama, E. Yuba and A. Harada, pH-Sensitive branched β-glucan-modified liposomes for activation of antigen presenting cells and induction of antitumor immunity, J. Mater. Chem. B, 2021, 9, 7713–7724 RSC.
- E. Yuba, N. Tajima, Y. Yoshizaki, A. Harada, H. Hayashi and K. Kono, Dextran derivative-based pH-sensitive liposomes for cancer immunotherapy, Biomaterials, 2014, 35, 3091–3101 CrossRef CAS PubMed.
- E. Yuba, Y. Fukaya, S. Yanagihara, N. Kasho and A. Harada, Development of mannose-modified carboxylated curdlan-coated liposomes for antigen presenting cell targeted antigen delivery, Pharmaceutics, 2020, 12, 754 CrossRef CAS PubMed.
- Y. Guo, H. Feinberg, E. Conroy, D. A. Mitchell, R. Alvarez, O. Blixt, M. E. Taylor, W. I. Weis and K. Drickamer, Structural basis for distinct ligand-binding and targeting properties of the receptors DC-SIGN and DC-SIGNR, Nat. Struct. Mol. Biol., 2004, 11, 591–598 CrossRef CAS PubMed.
- P. J. Coombs, R. Harrison, S. Pemberton, A. Quintero-Martinez, S. Parry, S. M. Haslam, A. Dell, M. E. Taylor and K. Drickamer, Identification of novel contributions to high-affinity glycoprotein-receptor interactions using engineered ligands, J. Mol. Biol., 2010, 396, 685–696 CrossRef CAS PubMed.
- H. Feinberg, A. S. Powlesland, M. E. Taylor and W. I. Weis, Trimeric structure of langerin, J. Biol. Chem., 2010, 285, 13285–13293 CrossRef CAS PubMed.
- H. Feinberg, S. A. F. Jégouzo, Y. Lasanajak, D. F. Smith, K. Drickamer, W. I. Weis and M. E. Taylor, Structural analysis of carbohydrate binding by the macrophage mannose receptor CD206, J. Biol. Chem., 2021, 296, 100368 CrossRef CAS PubMed.
- L. Dorland, H. van Halbeek, J. F. Vleigenthart, H. Lis and N. Sharon, Primary structure of the carbohydrate chain of soybean agglutinin. A reinvestigation by high resolution 1H NMR spectroscopy, J. Biol. Chem., 1981, 256, 7708–7711 CrossRef CAS PubMed.
- H. Feinberg, R. Castelli, K. Drickamer, P. H. Seeberger and W. I. Weis, Multiple modes of binding enhance the affinity of DC-SIGN for high mannose N-linked glycans found on viral glycoproteins, J. Biol. Chem., 2007, 282, 4202–4209 CrossRef CAS PubMed.
- R. K. Gupta, D. G. Goswami, R. R. Singh, A. Surolia and A. K. Panda, Soybean agglutinin coated PLA particles entrapping candidate vaccines induces enhanced primary and sustained secondary antibody response from single point immunization, Eur. J. Pharm. Sci., 2012, 45, 282–295 CrossRef CAS PubMed.
- S. Sinha, N. Mitra, G. Kumar, K. Bajaj and A. Surolia, Unfolding studies on soybean agglutinin and concanavalin a tetramers: a comparative account, Biophys. J., 2005, 88, 1300–1310 CrossRef CAS PubMed.
- H. Lis and N. Sharon, Soybean agglutinin–a plant glycoprotein. Structure of the carboxydrate unit, J. Biol. Chem., 1978, 253, 3468–3476 CrossRef CAS PubMed.
- D. Ashford, R. A. Dwek, J. K. Welply, S. Amatayakul, S. W. Homans, H. Lis, G. N. Taylor, N. Sharon and T. W. Rademacher, The beta 1->2-D-xylose and alpha 1->3-L-fucose substituted N-linked oligosaccharides from Erythrina cristagalli lectin, Eur. J. Biochem., 1987, 166, 311–320 CrossRef CAS PubMed.
- M. T. Huynh, C. Mikoryak, P. Pantano and R. Draper, Scavenger receptor A1 mediates the uptake of carboxylated and pristine multi-walled carbon nanotubes coated with bovine serum albumin, Nanomaterials, 2021, 11, 539 CrossRef CAS PubMed.
- M. G. Villegas, M. T. Ceballos, J. Urquijo, E. Y. Torres, B. L. Ortiz-Reyes, O. L. Arnache-Olmos and M. R. López, Poly(acrylic acid)-coated iron oxide nanoparticles interact with mononuclear phagocytes and decrease platelet aggregation, Cell. Immunol., 2019, 338, 51–62 CrossRef CAS PubMed.
- M. Fujiwara, J. D. Baldeschwieler and R. H. Grubbs, Receptor-mediated endocytosis of poly(acrylic acid)-conjugated liposomes by macrophages, Biochim. Biophys. Acta, 1996, 1278, 59–67 CrossRef PubMed.
- Y. Hou, Y. Wang, Y. Tang, Z. Zhou, L. Tan, T. Gong, L. Zhang and X. Sun, Co-delivery of antigen and dual adjuvants by aluminum hydroxide nanoparticles for enhanced immune responses, J. Controlled Release, 2020, 326, 120–130 CrossRef CAS PubMed.
- J.-W. Lim, W. Na, H.-O. Kim, M. Yeom, A. Kang, G. Park, C. Park, J. Ki, S. Lee, B. Jung, H. H. Jeong, D. Park, D. Song and S. Haam, Co-delivery of antigens and immunostimulants via a polymersome for improvement of antigen-specific immune response, J. Mater. Chem. B, 2020, 8, 5620–5626 RSC.
- M. Miyazaki, E. Yuba, H. Hayashi, A. Harada and K. Kono, Development of pH-responsive hyaluronic acid-based antigen carriers for induction of antigen-specific cellular immune responses, ACS Biomater. Sci. Eng., 2019, 5, 5790–5797 CrossRef CAS PubMed.
- V. Hornung, F. Bauernfeind, A. Halle, E. O. Samstad, H. Kono, K. L. Rock, K. A. Fitzgerald and E. Latz, Silica crystals and aluminum salts activate the NALP3 inflammasome through phagosomal destabilization, Nat. Immunol., 2008, 9, 847–856 CrossRef CAS PubMed.
- H. S. Kim and D. H. Chung, TLR4-mediated IL-12 production enhances IFN-γ and IL-1β production, which inhibits TGF-β production and promotes antibody-induced joint inflammation, Arthritis Res. Ther., 2012, 14, R210 CrossRef CAS PubMed.
|
This journal is © The Royal Society of Chemistry 2024 |