DOI:
10.1039/D3SU00174A
(Perspective)
RSC Sustain., 2023,
1, 1722-1736
White & grey biotechnologies for shaping a sustainable future
Received
2nd June 2023
, Accepted 8th August 2023
First published on 9th August 2023
Abstract
Biocatalysis plays a prominent role in both white and grey biotechnologies. Some well-known and a few lesser-known developments in biocatalysis have made it align even better with principles of green chemistry and green engineering and hence contributed to both white and grey biotechnologies in shaping a sustainable future. These include more efficient production of enzymes, use of process intensification techniques such as microwaves, ultrasonics, high pressure and flow catalysis. The market size of enzymes was estimated to be about USD 12.3 billion in 2022. Discovery of enzyme non-specificity as seen in their moonlighting and promiscuous behaviour has far-reaching consequences in biotechnology. The important roles of biocatalysis in production of biodiesel and bioethanol; valorization of whey and food waste in general are discussed. The waste generated in processing of coffee beans and coffee brewing, pegged globally at 10 million tons each year, deserves more attention as a part of the biorefinery concept.
Sustainability spotlight
Two shades of biotechnologies, white and grey, are concerned with the impact of our anthropogenic activities on the environment. The original premise of white biotechnology was to develop processes which did not need “end-of-pipe solutions”. This has been possible in many cases by following the rules of green chemistry and green engineering. However, this perspective points out that in many other cases, the solution has turned out to be waste valorization rather than its abolition. Thus, bioremediation (the mandate of grey biotechnology) and waste valorization complement each other well as twin approaches and continue to fashion our quest for development of sustainable processes. Just like in green chemistry, biocatalysts are the lynchpin in the development of many of these processes related to waste valorization and bioremediation. For example, biofuels cannot become economical without the concept of biorefinery which essentially seeks innovative exploitation of by-products/waste. This perspective discusses developments in biocatalysis which have turned them into even more practical and economical drivers of waste valorization. The perspective then goes on to discuss some case histories to illustrate this. The message is that biocatalysts have a tremendous impact on the energy sector and prevention of environmental deterioration.
|
1. Introduction
It has become popular to classify biotechnology in terms of a colour code.1,2 Biotechnology, as such, is about the use of biological cells/molecules for process/product development. So, a common thread runs through all classes of biotechnology. Worries about our climate, environment and overdependence on fossil fuels have led biotechnologists to focus on sustainability of industrial processes/practices. Fossil fuels are not renewable in a practical sense; their extraction and use affect both the climate and environment. Thus, the three worries are rather related. Besides, many anthropogenic activities generate waste. So, sustainability can be achieved by either using renewables/waste as raw materials or/and by switching from harsh chemistry to biological strategies with the aim of minimising waste generation. These thoughts led to the concept of white biotechnology. It is common to use it as a synonym for the pre-existing discipline of industrial biotechnology. It is that but with a focus on reliance on sustainable practices. What was earlier called environmental biotechnology (largely dominated by bioremediation) is now subsumed in the concept of grey biotechnology.3 This perspective is about how both white biotechnology and grey biotechnology complement each other in the quest for sustainability.
Chemists had taken the lead in the development of sustainable methods. Anastas and Warner enunciated the twelve rules of green chemistry.4 Almost, half a century later, Anastas and Zimmerman added twelve rules of green engineering.5 Technology is, in a way, about developing engineering solutions to turn ideas from basic science into practical applications, especially at the industrial level. So, it is but natural that strategies of white and grey biotechnologies have considerable overlap with these rules of green chemistry and engineering.
White biotechnology shared a vision with green chemistry that it would develop processes which would minimize/abolish waste generation.6 The ambitious philosophy of white biotechnology was captured in the key phrase that “no end of pipe solution” should be needed. Ideas, just because they are noble, do not necessarily find traction in industry. In this case, industry saw a way out of saving money on bioremediation and dealing with environmental regulations of the local/regional authorities. Undoubtedly, both green chemistry and white biotechnology have impacted industrial processes substantially for the better. Kazlauskas and Kim list industrial innovations which have won the US presidential green chemistry award during the 2004–2010 period.7 It is noteworthy that every innovation on the list rests on biocatalysis. The market size of enzymes was estimated to be about USD 12.3 billion in 2022. The revenue forecast for 2030 is estimated to be USD 20.3 billion, with the maximum share being contributed by the pharmaceutical sector.
Sheldon has pointed out that “biocatalysis conforms to 10 of the 12 principles of green chemistry”.8 Hence, it would not be an over-statement to say that biocatalysis is the pivot of white biotechnology. Biocatalysis is an enabler of white technology in its aim to conform to green chemistry.7,9,10 It is not surprising that, quite early, attention was paid to obtaining/designing enzymes dedicated to the application of biocatalysis in white biotechnology.11 A later review focussed on obtaining enzymes for biorefineries.12 When the process moves beyond a laboratory, the scaled-up version has to comply with the rules of green engineering as well.
More specifically, this perspective (a) provides an overview of significant changes in process designs which have come about as a result of applications of biocatalysis in line with the basic philosophy of white biotechnology and (b) critically analyses how much of white biotechnology has turned out to be about waste valorisation leading to a useful product and not strictly about waste abolition as such. In these cases, it is often found that biocatalysis has facilitated the discovery of a route for waste valorisation. A few examples of this are discussed as well in this article. Where no valorisation of a waste is possible, bioremediation/grey biotechnology has also benefitted from advances in biocatalysis.13 Interestingly enough, the terms “waste valorisation” and “bioremediation” continue to be far more popular among research workers as compared to “white biotechnology” or “sustainable biotechnology (Fig. 1).
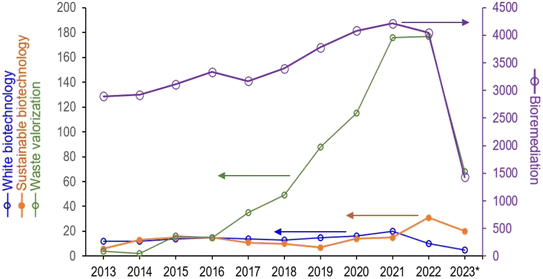 |
| Fig. 1 Analysis of publications in PubMed for estimating the number of times using the keywords “white biotechnology”, “sustainable biotechnology”, “waste valorization” and “bioremediation” that occurred in publications in recent years. For 2023, the data collected were until April 15, 2023. | |
2. Metrics of greenness
Given the shared vision of green chemistry and white biotechnology, a discussion on the metrics of greenness for processes is a good way to start our narrative.
Woodley has pointed out that the pharmaceutical industry, with 25–100 kg waste generated per kg of product, leads the chemical industry in dealing with production of bulk chemicals which produce waste of about >0.1 kg per kg product.14 It is noteworthy that pharmaceuticals is probably one sector which has been closer to the “no end of pipe solution” ideal rather than strategic shifts such as the biorefinery concept, which is more a story of waste valorisation.8 This article does not discuss those well-known successes in the pharma sector in the post-white biotechnology era, although there too, a combination of chemical catalysis and biocatalysis has played a major role.14,15
Several metrics for evaluating/grading the greenness of a process have been suggested.8,16 Some are based directly on the principles of green chemistry. A simple one is atom economy (AE) which evaluates how many atoms of the reactants ended up in the product (and not in a by-product). The corresponding quantitative parameter is AE%, which is defined as the ratio of molecular weights of the desired products to those of the reactants multiplied by 100. It is an oversimplified parameter as the solvent and the stoichiometry of the reaction are not taken into account. Reaction mass efficiency (RME) replaces the molecular weights with the actual masses of the reactants used and product(s) obtained. On the other hand, carbon efficiency just takes into account the ratio of amounts of carbon in the products to that in the reactants. Neither of the last two parameters takes into account the environmental concerns related to the solvents (used as the reaction medium or during work-up) or the energy consumed. Some other green matrices have also been proposed from time to time.17
The general consensus appears to be that the E-factor is the best parameter to evaluate greenness.17 The environment factor (EF, E-factor) has the virtue of being flexible to incorporate additional parameters. Defined simply, it is the ratio of kg of total waste to kg of product; it can even be used to estimate greenness of each step in a multi-step process.18 Both the simple EF (ignoring solvents and water) and complete EF (factoring in solvents and water footprints, assuming no recycling of either) have been described.8 It has been pointed out that a few catalytic processes, while having high AE, are actually low E-factor processes.8 Calculation of the E-factor also resulted in the surprising finding that the oil-refining industry had E-factor <0.1 whereas processes in the fine chemical and pharmaceutical industries had E-factors in the range of 5–>100.19 Sheldon points out that pharmaceutical industries have paid the necessary attention to greening of their manufacturing processes since these E-factors were published.8
An E+-factor, which also takes into account greenhouse gas emissions, has been proposed; this way the kind/amount of energy used is also estimated to get a holistic idea of the greenness of the process.20 Sheldon has also discussed the challenge of using green matrices when a starting material is obtained from outside or its synthesis is outsourced.8
Maria has discussed the use of the E-factor and a gate-to-gate strategy to assess the greenness of the process of hydration to oleic acid by oleate hydratase.21 Consideration of the E-factor and estimation of carbon dioxide release (total and in kg per kg of product) led to identification of “hot spots” in the process which enabled improvement in the greenness of the process. In a more recent article, the same author has emphasised the need to look at the ecological footprint of the use of organic solvent at all stages of a biocatalyst process: in extraction of substrates from the raw material, in the synthesis of solvent as such, in the actual biocatalytic step and during disposal as waste at the end of the process.22 The article also points out the positive impact of process intensification (such as the use of higher substrate concentrations) and solvent recycling.22
Life cycle assessment (LCA) which looks at “cradle to grave” greenness is complex but undoubtedly the most accurate parameter to judge the true greenness of a process/product.23 LCA also includes the water footprint (WFP) of a process. The use of ground and surface water is coded blue; rainwater is coded green. A grey WFP is the volume of water needed to dilute the waste stream to conform to international standards such as those set by the EC or US Congress.24 A good discussion on the synergy of green chemistry and green engineering rules in enhancing sustainability of processes is available.25
3. How biocatalysts have emerged as enablers in white and grey biotechnologies
There have been some paradigm shifts over the years which have made biocatalysis align even more with the rules of green chemistry and green engineering.26,27
3.1 Economics of biocatalytic processes
The first one is the cost of enzymes. Cheetham, in an insightful article on economics of using a biocatalyst in an industrial process, pointed out that “the microorganisms or enzymes used constitute only a small proportion of the total running costs of the process”.28 The situation, if anything, has become even better. Earlier, a significant fraction of the production cost of the enzyme used to go towards its downstream processing.29–32 The trend has increasingly been to shorten purification protocols. A major enabler has been developments in affinity-based processes.32–35 While earlier affinity ligands (such as enzyme inhibitors, lectins and antibodies) were mostly of biological origin (and hence expensive), ‘pseudoaffinity ligands’ such as textile dyes and metal chelates made affinity media much cheaper. A notable move was the use of recombinant technology to produce proteins with fusion tags such as polyhistidine. Such proteins could be easily purified using immobilized metal ion affinity chromatography (IMAC), which uses immobilised columns of chelated nickel, zinc ions, etc.36–39 Today, there are many technologies, such as (strept)avidin-biotin or INTEIN, built around this concept and a huge variety of fusion tags have been described in the literature.40–42 This is a key driver for abolishing the distinction between upstream and downstream stages of protein purification. Not all proteins/enzymes need to meet the same purity levels. Some enzymes (such as asparaginase, streptokinase, etc.) and proteins (such as hormones, cytokines, vaccines and antibodies) which have therapeutic applications have to be not only “pure” (in the sense of being free of other proteins and isoproteins), but also have to be analysed to ensure that molecules such as endotoxins, nucleic acid fragments, etc. are not present. Besides, even the presence of modified forms of the desired protein (aggregates, with oxidised methionines, deaminated asparagine/glutamine, incorrect disulphide bonds, and proteolytic fragments with different levels of post-translational modifications) has to be ruled out.43,44 It is for these reasons that advances in protein production have not resulted in lowering the cost of therapeutic proteins/enzymes drastically. Enzymes used in diagnostics have to be free of other enzymes/inhibitors with interfering activities but their cost tends to be higher (than what are called industrial enzymes) as their market size is smaller. As pointed out by Cheetham,28 market size/volume produced affects the cost of production.
Enzymes which play a greater role in white and grey technologies (sometimes referred to as industrial enzymes) generally do not need to have high purity and are produced at much larger scales. These are not so expensive anymore. Proteases, amylases, cellulases, hemi-cellulases, pectinases, and lipases find applications in detergent formulations, the starch and textile industry, the oil and fat industry, the food and beverage industry, cheese making, animal and poultry feed formulations and leather processing. Specific enzymes such as glucose isomerase (for production of high fructose containing syrup) and penicillin acylase (for obtaining semi-synthetic penicillins) have been game-changers single-handedly.28,43,45 Cheetham has provided interesting data about how the cost of penicillins has come down by over a thousand times between 1943 and the 1950s.28 More relevant is the impact of replacing chemical deacylation with penicillin G acylase.8
Industrial enzymology also has a different toolbox for purifying proteins. These are scalable processes such as aqueous two-phase separations (ATPS) and expanded bed chromatography (EBC). These are also unique because neither requires the solid–liquid separation step in which the feed can be cleared of cell debris. This necessitates the use of industrial level centrifugation or membrane-based separation. Both ATPS and EBC can also be integrated with an inbuilt affinity interaction.33,34,37,46–49 Precipitation can be combined with smart affinity ligands, in a process called affinity precipitation. Affinity interaction can also be incorporated into a technique called three phase partitioning (TPP) to design a process called macro-[affinity ligand] facilitated three phase partitioning (MLFTPP) which is the third process which can directly handle suspensions as feed for purification. These partitioning processes are also useful in protein refolding in cases where the products of the recombinant protein are inactive solids called “inclusion bodies”.50,51 Both TPP and MLFTPP have yet to be tried at the industrial level but show considerable promise as approaches which merge upstream and downstream stages to create streamlined and shorter purification protocols.
To sum up, most of the enzymes used in white biotechnology can be produced fairly cheaply. Furthermore, protein engineering, directed evolution and clustered regularly interspaced short palindromic repeats (CRISPR) technology have made it possible to tailor their specificities and storage or operational stabilities.52–55
Apart from the use of recombinant technology, sourcing enzymes from extremophiles has played an important role.56,57 Enzymes from psychrophiles can operate at much lower temperature, obviating the need for sanitisation between production cycles in food/milk processing industries.58–64 Enzymes from psychrophiles are also useful in washing machine detergents. According to Novozyme, “if everybody in Europe turned down the wash temperature from 60 to 40 °C and from 40 to 30 °C, the carbon dioxide reduction would equal the annual carbon emissions from 3 million cars” (Biotimes, no. 04, December 2009, Novozyme).
3.2 Non-aqueous enzymology, medium engineering and green solvents
While early enzymology was almost exclusively carried out in aqueous buffers, currently it is common to use aqueous-organic cosolvent mixtures, reverse micelles, or low water containing non-aqueous media as the reaction medium.65 In recent years, lyophilised enzymes, which give poor catalytic rates, have been largely replaced with better enzyme formulations such as propanol rinsed enzyme preparations (PREPs) or enzyme precipitated and rinsed with n-propanol (EPRP), cross-linked enzyme aggregates (CLEAs), cross-linked enzyme crystals (CLECs) and protein coated micro-crystals (PCMCs).66–72 While earlier highly polar and water miscible organic solvents were reported to be not practical as reaction media, this problem could be easily solved by a simple chemical modification of the enzyme so that it resisted stripping of necessary water molecules by such solvents.73 This was a rather critical finding as highly polar organic solvents are often the preferred reaction media as these dissolve most of the organic compounds.
The option of being able to use different media has opened up possibilities for changing the yield and selectivity of biocatalytic reactions; this is sometimes referred to as medium engineering.73–75 A relevant issue is being able to select a “green solvent”. Ionic liquids and deep eutectic solvents have emerged as good options for further greening of biocatalysis.76 At one time, it appeared as if some high-performance formulations such as CLEA would not work there but soon enough it was shown that this is not so.73 Supercritical/subcritical and fluorous solvents have also been used as reaction media for enzyme-catalysed reactions.77 While carbon dioxide, ethane, propane, trifluoromethane, n-butane and freons have all been used, carbon dioxide as a supercritical fluid has shown sufficient promise as a green solvent. One factor to watch out for is the drop in pH around the hydration layer of the enzyme as the locally produced carbonic acid can affect the enzyme activity. Enzymes such as lipases, glucoamylase, cellulase, many proteases etc. have been used in supercritical carbon dioxide.78 Solvent free conditions mean that one of the substrates itself is used as a solvent during the reaction. Alcohols have been sometimes used in preparation of biodiesel by lipase catalysed transesterification reactions with fats/oils.79
The concept of green solvents tends to be controversial. When it comes to organic solvents at least, a good view is “while there is no complete consensus on which solvents are “green”, there is some reasonable agreement on what cannot be used (e.g. chlorinated solvents), and on what could be used (low molecular weight alcohols, esters, some ketones, etc.)”.22 Water is a good choice from a green point of view. A challenge in carrying out biocatalysis in aqueous media is posed by substrates which are poorly soluble in such media. Padrosa et al. reported that adding a surfactant was an efficient strategy to solve this problem in the case of hydrolysis of naproxen ester in a flow reactor.80
Lipschutz's group has been focussing on micellar catalysis, i.e. carrying out reactions in core organic solvents to ensure solubility of substrates which are otherwise poorly soluble in outside water medium. An earlier overview by this group discussed ideal designs for catalysis by organometallics.81 The concept is extended to biocatalysis/chemoenzymatic catalysis in other published studies which is part of a recent review.82 The important feature of micellar catalysis is that micelles are not insulated from the external water medium. The organic solvent is just 1–5% (w/w) of the total medium. The species compete for the inner core of organic solvent. While micellar catalysis is not new, this recent focus on it has led to the development of designer surfactants and carrying out chemoenzymatic synthesis in a “single pot” fashion. The reaction rates for enzymatic reactions are much faster than in neat organic solvents. The applications at the industrial level have already been reported.82
3.3 Promiscuity enables repurposing of enzymes
While drug repurposing has become a popular phrase in recent years,83 repurposing of usual enzymes especially inexpensive ones (hydrolases such as amylases, proteases and lipases being important examples) is becoming increasingly important in many areas of biotechnology. These unusual applications mostly become possible because of what is called enzyme promiscuity.26,83–90 Substrate promiscuity refers to what has been known as the broad specificity of enzymes. A lipase may hydrolyse fats and oils made up of different fatty acids.91 Alcohol dehydrogenases can catalyse redox reactions with diverse alcohols.92–94
Catalytic promiscuity, on the other hand is sort of a game changer. It refers to the catalysis of a reaction by an enzyme which is otherwise normally catalysed by an enzyme from another class among the six classes under EC classification.90,95,96 An important example of catalytic promiscuity is catalysis of C–C bond formation in aqueous media, aqueous-organic cosolvent mixtures and low water containing non-aqueous media. So much of organic synthesis involves C–C bond formation. Enzymes which can catalyse C–C bond formation, such as aldolases, transketolases and thiamine pyrophosphate-dependent carboxylases are relatively expensive.97 Formation of C–C bonds by lipases has been reported extensively and illustrates catalytic promiscuity.65,92,98–100 If this C–C bond formation is carried out in a non-aqueous medium, it becomes an example of both condition promiscuity and catalytic promiscuity operating simultaneously.85,101–103
3.4 Process intensification in biocatalysis
The process intensification concept was originally developed in the 1970s at Imperial Chemical Industries. While the aim was cost reduction by innovation, a useful unintended consequence was that it led to moving closer to the goals of green chemistry and green engineering besides better safety.104 Currently, it “aims to make dramatic reductions in plant volume, ideally between 100- and 1000-fold, by replacing traditional unit operations with novel, usually very compact designs by combining them into one hybrid unit”.104
An early review on microwave-assisted enzymology is by Roy and Gupta.105 While microwave-assisted extractions have been more common, their use in accelerating catalytic reaction rates, especially in low water enzymology, has also been explored.106,107 Microwave irradiated pre-treatment has been shown to significantly enhance the yield from biomass-derived sugars and other chemicals.108 An intriguing approach has been to improve enzyme performance by using magnetic fields. Wasak et al., who worked with laccase, have referred to a few earlier reports on how static magnetic fields affected the catalytic activities of amylase, superoxide dismutase, peroxidase and tyrosinase and the influence of the rotating magnetic field (RMF) on reactions catalysed by chitinase and lipase.109 In their work, they report a frequency-dependent effect of RMF on an increase in laccase activity by up to 11% with broadening of the pH range. They speculated that eddy currents reduce mass-transfer constraints. Laccase is used for bio-pulping and bio-bleaching of textiles, delignification and organic synthesis.110 Horikoshi et al. also report that the magnetic field generated by microwaves facilitates hydrolysis of casein catalysed by papain and decomposition of hydrogen peroxide by catalase.111 While the mechanism of the effect(s) of magnetism on biocatalysis is far from clear, interest in this seems to be picking up. Recently, Dik et al. reported that an externally applied magnetic field improved the activity of L-asparaginase adsorbed on iron oxide nanoparticles.112 Extensive work has been carried out on enzyme immobilization on iron oxide nanoparticles;113–117 it may be interesting to investigate this phenomenon with a wider range of immobilised enzymes.
Ultrasonics has also been used for biocatalysis, mostly in low water media.66,118,119 Activation of proteases for food processing has also been attempted using ultrasound.120 Şener et al. described results on lactose hydrolysis by lactase and reported significant enzyme inactivation upon pulsing of ultrasonic waves.121 As process intensification tools for biocatalytic reactions and extractive processes, a recent update on the use of microwaves and ultrasound has been published.122 At the commercial level, microwaves have been used by the food industry but these large size units tend to be rather expensive. With both ultrasonics and microwaves, enzyme stability in aqueous media continues to be a challenge.
Membranes in recent years have become cheaper and reactor design has minimised the fouling problem. So, membrane reactors are being increasingly used in biocatalysis.123
One area which is less known is that of baroenzymology. A recent book is dedicated to high pressure technologies related to enzymes.124 While most applications of high pressure (as a process intensification method) relate to the food, beverage and dairy industries, it is a promising approach as results with many microbial enzymes such as amylases, asparaginase, glucose oxidase, lactase and proteases illustrate. The effect of high pressure on enzyme catalysis in ionic liquids is of direct relevance to white biotechnology.124 High pressure reactors are also used when supercritical fluids are employed as the reaction medium (see the earlier brief discussion on supercritical fluids in this article).
3.5 Enzyme immobilization
Enzyme immobilization is an old area where innovations continue to happen ceaselessly. As enzymes are generally costlier than chemical catalysts, their recovery and reusability were considered important very early. Two fairly comprehensive resources on enzyme immobilization are books edited by Cao125 and Ferreira.126 Immobilization, whether on solid matrices or smart polymers, makes it possible to recover and reuse biocatalysts after the reaction.91,110,111,122–135 The use of nanomaterials including nano-composites as carriers of enzymes has been extensively reported in recent times.114,117,136–142 Horseradish peroxidase (HRP) has been frequently used for bioremediation.143 HRP was immobilized in a magnetic hybrid nanocomposite and worked well in this case though the intended application was conversion of a prodrug (Fig. 2).144 At the same time, green methods for synthesis of nanomaterials have also been reported.145 Some materials such as egg shells, agricultural waste, etc. have been reported as carriers.125 Of particular interest are carrier-free immobilisation strategies.88,146–151 Without a carrier, the immobilized enzyme occupies a much smaller volume, making it possible to use smaller reactors. Two approaches to preparing aggregates of multiple enzymes to catalyse either cascade or non-cascade reactions deserve a mention here.68,152–154 Another strategy is the gentler method of affinity immobilization, especially if coupled with oriented immobilization to obtain high activity preparations.155 Affinity immobilization also makes it possible to deposit a higher amount of activity on small surfaces.143,156–158 An interesting application is treatment of waste water with peroxidase immobilized by affinity layering.143
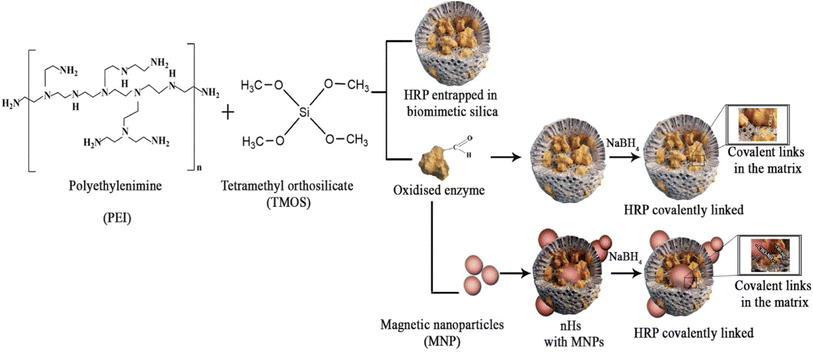 |
| Fig. 2 Magnetic biocatalyst. Horseradish peroxidase was coimmobilized along with magnetic nanoparticles in a preparation of biomimetic silica. Taken from ref. 144. This is an open access article distributed under the terms of the Creative Commons Attribution License, which permits unrestricted use, distribution, and reproduction in any medium, provided the original author and source are credited. | |
A recent paper illustrates a few interesting trends vis-a-vis applications relevant to white biotechnology.159 Waste cooking oil continues to attract attention as a feedstock for producing biodiesel. Magnetic supports for enzyme immobilization are becoming more popular; this is reflected in more publications on magnetic CLEAs as well.145 In the case of biodiesel, especially, magnetic biocatalysts make sense as the generation of glycerol during biodiesel production turns the reaction medium viscous and using a magnet is an efficient way for recovery/recycling of the biocatalyst. Thirdly, composite nanomaterials based on graphene are becoming more common as supports for enzyme immobilization; a paper uses titanium oxide coated iron oxide nanoparticle doped graphene oxide as a carrier for enzyme immobilization for biodiesel preparation.159 While reusability and enhanced stability are often mentioned as desirable outcomes of enzyme immobilization, an equally (and possibly even more) important outcome is that it allows enzymes to be used in all kinds of formats/reactor design. An important illustration of this is the area of flow biocatalysis which is also considered a process intensification strategy but deserves a separate discussion.
3.6 Flow biocatalysis
Perhaps the use of immobilized enzymes in flow systems started with the availability of a simple method to immobilize urease on nylon tubing.160 The early applications of flow systems were in designing flow analysers as this allowed fast analysis of multiple systems such as those in a clinical laboratory. Both “online” (where an aliquot is analysed without manual sampling) and “inline” (all whatever is flowing through is analysed) options are possible.161 Multiple (practically all) spectroscopic techniques can be used for monitoring the reaction. An interesting application was design of an online biosensor for lactate during open heart surgery. Lactate oxidase and catalase were immobilised on porous glass beads to detect the lactate level in blood in real time.162
Flow catalysis became a powerful approach in sustainable chemistry as it enabled the use of smaller reactors (less energy intensive) which can be used in parallel for scaling up. The continuous flow does away with the limitations of equilibrium-controlled reactions and product inhibition.163 Flow biocatalysis in microfluidic systems (including paper-based formats) has quickly emerged as a versatile method in the context of both biosensor designs and preparative processes. It has been adopted by enzymologists for many processes and approaches such as coenzyme regeneration,164 employing enzyme cascades,165 carrying out photobiocatalysis166 and process intensification.167 A few years back, Paradisi edited a special issue of the journal Catalysts containing recent applications of flow biocatalysis.168 As pointed out therein, shifting from batch to continuous operation helps increase industrial productivity. A recent review summarises some of the recent developments and results.169
3.7 Whole cell biocatalysis and co-enzyme regeneration
Cells as such have been used for biocatalysis since early days. This avoids separation and purification of enzymes. To improve mass transfer of substrates and products, it has been common to permeabilize cells before using them as biocatalysts.170 A good update on whole cell biocatalysis for obtaining both bulk and fine chemicals is available.171
One advantage of using live whole cells as biocatalysts is in coenzyme-dependent reactions. Coenzymes such as NAD, NADP, etc. are costly. In recent years, many coenzyme regeneration strategies have been developed while using isolated enzymes (rather than whole cells).172 The most common co-substrates which act as “sacrificial electron donors” are ethanol and isopropanol.172 As a reaction with a co-substrate is thermodynamically unfavourable, molar excess of the co-substrate generates “waste”. Worth mentioning is the discovery of 1,4 butanediol as a co-substrate. Not only can the waste generated be reduced 40-fold, but the diol is also available from green routes.172 An unusual strategy has also been described in which the cells are gradually permeabilized to increasing levels, resulting in enzymes of increasing molecular weights/sizes that could be “secreted” in a fairly purified form.173
Before we conclude this discussion on biocatalysis, it is worth mentioning that a recent special issue of Chemical Reviews includes some updates on the current strategies in biocatalysis.174 Metal-enzyme cascade reactions have been comprehensively reviewed.175 Gantz et al. discussed the use of water-in-oil emulsion droplets for screening biocatalysts.176 Working with picolitre volumes means a tremendous reduction in volumes over the popular 96-well plate format. Combining with microfluidics, this screening approach is much faster and results in a significant improvement in screening efficiency over other currently practiced methods.
After the above discussion related to the state of the art of biocatalysis, we can now discuss few case histories of waste valorization in white biotechnology.
4. Whey as a biomass: a classical but ongoing story in waste valorisation
Much before the concept of white biotechnology was enunciated, whey obtained in the dairy industry was shown to be converted into value-added products. So, rather than bioremediation, valorization led to development of routes for producing bioethanol. Annual production of whey is estimated to be about 160 million tons, increasing by 2% per year.177 On a dry w/w basis, 70% of whey is lactose and 14% is protein; some free amino acids, minerals and a small amount of fat constitute the rest. The presence of lactose was a hindrance in discharging the whey as a waste in water bodies since it has a high BOD (biological oxygen demand) value and would affect marine and freshwater organisms negatively. Early efforts to use lactose as a sweetener in ice creams, etc. were not successful as it felt like sand on the tongue. This led to the use of beta-galactosidase (also known as lactase) for hydrolysis of lactose. The development of this technology was also spurred on by the discovery of low lactose tolerance. Early pilot plant level technologies to reduce lactose content were developed at Corning (USA) (and naturally used glass beads) and Snam Progetti (Italy) which used fibre entrapped lactase.178,179 While Corning technology was developed for acid whey, the Italian group focussed on milk. Valio in Finland got into this sector as a result of a Master's thesis and initially used membrane-based technology. Their web-page describes an interesting story of how the company was reluctant to market low lactose milk (https://www.valio.com/food-solutions-for-companies/articles/through-fire-and-water-overcoming-obstacles-in-the-creation-of-lactose-free-products/, accessed on Feb. 27, 2023). The board's perception was that people would reject milk tampered with by technology. They actually sold 1 million litres of low lactose milk in the first two months. Later, Valio also used lactase immobilised on phenol-formaldehyde resin.100 This tells us how the acceptance of products created by biotechnological interventions has shaped up. A 1980 review provides a good account of application of immobilised lactase in hydrolysis of lactose in milk and whey.180 However, Dekker et al. describe that commercial use of free enzymes was the general practice as immobilized enzymes were not viable until recently.181
Lactose present in whey can also be converted to galactooligosaccharides (GOS) by transgalactosylation with beta-galactosidases.182 GOS have 2–8 sugar molecules with all galactose residues except the terminal one which is glucose. These are well known prebiotics with a multibillion USD market. High temperature, two phase systems of aqueous-organic cosolvents and reverse micelles have been used to favour transgalactosylation over hydrolysis in enzyme catalysed reactions. Apart from immobilized beta-galactosidases, whole cells as such or immobilised by surface display technology have been used as biocatalysts.183,184 An important prebiotic called lactulose is just a disaccharide of galactose and fructose and has been produced using beta-galactosidase, both in free and immobilised forms. Apart from its use as a prebiotic, it has been used to improve sensory characteristics of food products.177
Bovine milk proteins consist of 80% caseins and 20% whey proteins. Whey contains proteins such as alpha- and beta-lactalbumins, immunoglobulins, lactoferrin, lactoperoxidase and glycomacropeptide.185 Apart from their use as protein supplements, whey proteins have also been explored for treatment of some cancers and HIV. Extensive studies have been reported on obtaining bioactive peptides of different lengths and sequences from enzymatic hydrolysis of whey proteins from the milks of buffalo, camel and donkey.186,187 These peptides have shown anti-bacterial, anti-inflammatory, hypotensive, anti-diabetic and angiotensin-converting enzyme inhibitory activities. Many have been found to be antioxidants, anticancer agents and immunomodulators. Others are opioid-like and satiety hormone-inducing (making them useful in controlling obesity).186,187
5. Food waste valorisation: challenges & opportunities
Food waste originates at all stages of production, storage, transport, processing and consumption. Right at the farm level, a fair% of produce may not meet the desired level of quality. Seasonal weather changes are not unknown and can affect the crop. In many countries, cold storage at the place of production and a cold chain during transport are not always available. Occasionally, an imbalance in supply and demand exacerbates the situation. Some workers like to distinguish between ‘food waste’ (“industrial, commercial and domestic mixed food residues and bread and other bakery wastes meant for human consumption”) and ‘organic waste’ (“e.g. peels and rapeseed meal not meant for human consumption”).188 In this article, we will use the term food waste (FW) to include both.
It has been rightly pointed out that, in terms of desirability, the ranking of strategies to deal with FW is reuse > recycle for nutrient recovery > recycle for energy > disposal.189 FW/year is estimated to be a few billion tons per year. In the EU, it is around 89–145 megatons year−1. An FAO estimate is that about one-third of the food produced just for humans ends up as waste at one of the stages mentioned above.177,188,190 FW is reported to have an average composition of 30–60% starch, 5–10% proteins and 10–40% lipids (w/w%). This makes it a potentially valuable resource.188 A consortium of hydrolases in one case converted the FW to 0.63 g glucose per g of solid FW. In another attempt, a mixture of alpha-amylase and glucoamylase hydrolysed starch in FW almost completely.188 Bread waste particles incorporated in the growth medium could be used to grow S. mangrovei to obtain PUFA docosahexanoic acid which is now regarded as an important food supplement and is in high demand.188 FW hydrolysates in sea water could be used to grow known freshwater algae, which is remarkable as it avoids consumption of normal water.188 Similarly, microalgae which do not require aerable lands and can be grown in ponds or bioreactors, can utilize FW as a nutrient. In turn, microalgal biomass can be a source of diverse kinds of chemicals and materials.188 The use of waste from kitchens and restaurants to obtain biodiesel has been mentioned elsewhere.164
One powerful technique for waste valorisation of agro-industrial waste is solid state fermentation (SSF), or more accurately called solid substrate fermentation (when waste itself is used as both the support and nutrient).191 Brans of wheat and rice or sugarcane bagasse have been frequently used but utilization of diverse agro-industrial wastes has been reported.191,192 Fermentation takes place in the presence of low moisture content (does not consume water or generate waste water), is scalable with high volumetric productivity, and does not require high sterile conditions (so expensive fermenters are not required). SSF does suffer from poor heat and mass transfer. Air pulsation and agitation solve these problems to a certain extent. As heat is generated, SSF is particularly suitable for growing thermophilic microorganisms. SSF has been used to produce enzymes, animal feed and chemical/pharmaceutical intermediates. As filamentous fungi can access nutrients in solid waste more easily, SSF has been particularly useful for growing fungi. A recent book reports extensive applications of fungi and their enzymes in white biotechnology.193 The microbes secrete enzymes (which themselves are valuable products) to convert waste into more easily extractable metabolites. Cerda-Cejudo et al. discussed how SSF can be used to obtain polyphenols including tannins, flavonoids, and anthocyanins from SSF of peels, seeds and pulp of fruits and vegetables.194 Li et al. described conversion of FW to a product which can partially replace and improve commercial feed for crayfish.195 Blue biotechnology includes biotechnology dealing with marine and freshwater resources. Hence this kind of work in fact overlaps with both grey and blue biotechnologies.
One application of SSF, detoxification of agro-industrial waste, deserves special mention.196 Ricin (one of the most toxic substances) in castor bean cake, phorbol esters in jatropha seed cake, HCN in cassava peels and gossypol in cotton seed meal are some of the many examples of how SSF has detoxified these waste products and enabled their use as animal feed free of toxic substances. Some well-known environmental hazards such as the herbicide atrazine and chlorophenols such as pentachlorophenol (a known endocrine disruptor) can be degraded via SSF. Textile dyes adsorbed on FW have been successfully degraded using the SSF approach.
6. Usefulness of coffee beyond the last sip
About 10 million tons of coffee waste per year are reported to be produced globally.197 Nearly, 0.6 ton per ton of coffee produced is spent coffee grounds (SCGs); this is the waste generated during brewing and manufacturing instant coffee. This is approximately estimated at 6 million tons per year. SCG contains carbohydrates, lipids and proteins; polyphenolics and many bioactive compounds. It has been reported to be useful in producing biofuels (bioethanol, biodiesel, hydrogen and biogas), biomaterials such as polyhydroxyalkanoates and many bioactive compounds.197 So, SCG is worth mentioning in the context of waste valorization and the circular economy. Here, we will discuss further only its use in enzymatic production of biodiesel.
After coffee is brewed, spent coffee grounds contain about 10–15% (w/w) oil. To put this in perspective, among some common feedstocks for producing biodiesel, contents of oil in palm, soybean and rapeseed are 20%, 20% and 37–50%, respectively. It is observed that biodiesel from coffee oil has better stability against oxidation, and as in other cases, the rest of the solids after oil extraction can be used as a feedstock for ethanol.198 It was reported that the enzymatic route for obtaining biodiesel gave 98.5% conversion whereas alkali-catalysed conversion preceded by acid-catalysed esterification (in order to reduce free fatty acid content of coffee oil) led to poor conversion.199 Earlier, too, it was shown that oils containing high levels of free fatty acids can be efficiently converted into biodiesel by the enzymatic route.151,200 Burton et al. used one of the most expensive immobilized lipases, Novozyme 435, in their work.199
While enzymatic routes for conversion of oils/fats to diverse products have been mentioned in various contexts in this article and elsewhere,91 a mixture of lipases to obtain the “best trade-off between conversion and cost” of the enzymes (with different specificities and costs) was described for conversion of oil to biodiesel.72 The results illustrate the benefit of simple biocatalyst designs for large improvements in lipase performance in low water media.66 Both relatively expensive and cheaper free lipases in an optimised ratio (of activity units) were co-immobilised on an inexpensive core of potassium sulphate microcrystals.72 About 83% conversion in 48 hours could be obtained by this inexpensive immobilized biocatalyst.
Spent coffee grounds constitute an important system to build a biorefinery based on them. Kitchen/restaurant waste is considered a viable starting material. A similar approach to collect spent coffee grounds from cafes in a region should be possible. Unlike kitchen/restaurant waste which may be a quite heterogeneous waste, spent coffee grounds can be more homogeneous if care is taken to identify the origin of coffee beans used during brewing.
7. Glycerol: a C3 compound as a “waste” with multiple routes for valorization
For every molecule of fat/oil converted to biodiesel, a molecule of glycerol is generated. Glycerol, even prior to its availability in large amounts as a byproduct of biodiesel production, was used in the cosmetics, food and beverage industries, and for synthesis of trinitroglycerine and polymers.201–203 However, the amount of glycerol suddenly available in large amounts in crude form led to supply far outstripping demand.202
In the biorefinery concept, C3 molecules such as lactic acid, glycerol, 3-hydroxypropionic acid, 1,3 propanediol and acrylic acid are considered to be important building blocks for synthesis of multiple organic compounds (“platform chemicals”).2,204 The glycerol story is an example of how quickly innovative routes to conversion of an industrial by-product into such diverse products can be developed. In the USA alone, its generation was reported to be 250 million gallons in 2006, and by 2007, interesting leads for converting it to bioethanol, butanol, bioplastics, etc. were available.202,205 Further developments saw its use as a C-source for microbial production of value-added chemicals such as organic acids and polyols and many platform chemicals.206,207 It is interesting that in spite of its high viscosity, it could be used as such for some interesting organic reactions.208 Later, an immobilized lipase could convert glycerol into glycerol carbonate by a reaction with dimethyl carbonate in a solvent-free medium.209 Glycerol carbonate is an important green solvent. Glycerol-based deep eutectic solvents constitute another use of converting glycerol into a green solvent for an enzymatic reaction in which glycerol was a substrate as well.210
8. How cultural and regional factors can dictate waste valorization
It may be interesting to compare ground realities in two very different cultures and economies. Klein et al. discussed the waste from potato and rapeseed production in Lower Saxony, Germany.189 Many feel that biogas, because of its low energy efficiency and transport costs, is not a very attractive option. Most of the waste is used for livestock feeding in dairy and cattle farming. Waste water from potato processing has been used as a high-quality fertilizer and pet food. An interesting situation is that rapeseed cake has even been exported to Austria profitably in spite of the transportation cost. Taxation on biodiesel after 2006 has made the use of rapeseed oil for biodiesel production unattractive and several plants set up for this have had to shut down.
In a survey in India, some different features emerged.211 Household waste is estimated to be 68.7 million tons per year. One notable source of household FW was reported to be due to confusion about labels indicating storage conditions. A simple solution may be to use labels in both English and the local language. Waste due to an inadequate cold chain is also a big factor. The Indian experience with biogas has been more positive. An important finding mentioned is the high risk of disease transmission when waste is used as feed. For example, untreated swill fed to pigs was found to lead to higher incidences of hepatitis E.211
Not just with respect to waste valorization but regional factors are important for biotechnology as a whole. For example, adoption of biotechnology is quite critical for societal progress in developing countries. A recent report by Shah-Neville provides some insights into the opportunities and challenges in the biotechnology sector in developing countries in general.212 Sharma and Swarup had pointed out the role of biotechnology in national progress in India; much of this may be common to other developing countries.213 In line with the core theme of this article, Gupta and Mukherjee discussed at length how important training scientists in biocatalysis is for harnessing the benefits of biotechnology in such countries.214
9. Summary and future possibilities
Two distinct approaches to white biotechnology can be seen. The first is the synthesis of compounds or chemical intermediates used in industries related to pharmaceuticals, agriculture and other sectors. This exploits green chemistry but uses biocatalysts. This is where waste minimization or the idea of not needing “end of pipe solutions” for waste treatment has been reasonably successful. It appears that it is possible to identify/design an enzyme for practically any reaction. While specific examples of products/processes have not been discussed here, the evolution of the power of biocatalysis has been pointed out. The second approach, the focus of this article, is waste valorization.215 This is now frequently referred to as the biorefinery concept.216 The philosophy is that the products obtained ultimately from petroleum refining should be obtained from wastes/by-products of existing processes.217 The key is to get crucial building blocks, so called platform chemicals. Attempts are made to create diverse routes for valorisation and to make use of everything as far as possible. The first generation of biorefineries was designed around edible crops, the second generation around lignocellulosic wastes, and the third generation exploits micro- or macro-algae.
With reasonable success with the biorefinery concept, it is now common to hear words such as bioeconomy (economy built around biobased products and processes) or bio-circular economy which is essentially fairly close to the ideals of white biotechnology.218 Ideally, this should have abolished the need for bioremediation (grey biotechnology). In the real world, as technology makes our anthropogenic activities more complex and diverse, waste treatment will continue to be needed. A case in point is waste water.
What do we see in the future based on the current trends? First, the trends in biorefinery based on published work plus patent search have been discussed recently (Fig. 3).219 The amount and quality of water used in any bio-based process would become increasingly crucial.220 Enzymes from halophiles or engineered biocatalysts which can work in salty environments and hence with sea water are likely to be important. We have over-focussed on enzyme specificity even though so much has been possible in biotechnology because of non-specificity.26,83–92,97,98 With a change in mindset, more innovations would be possible. A good example of this is the use of inexpensive hydrolases such as amylase in bioremediation of alkanes.221
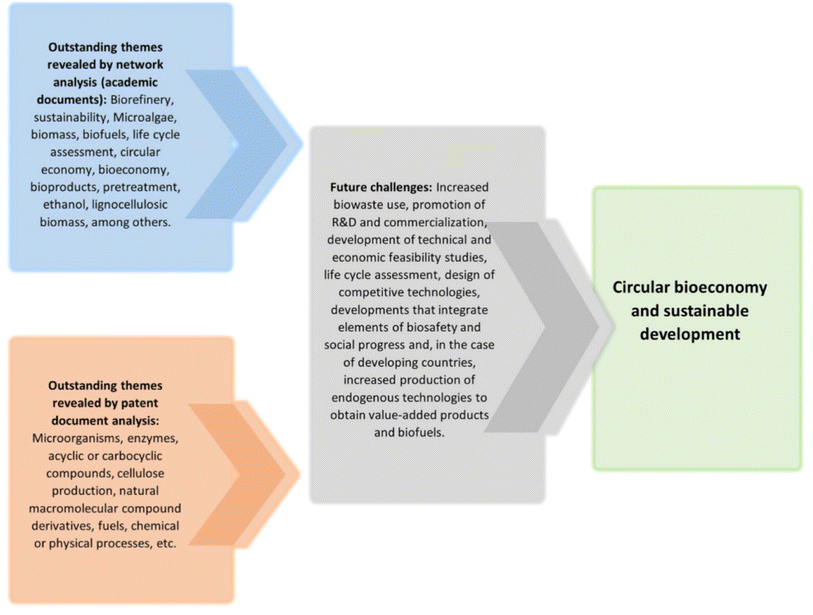 |
| Fig. 3 Analysis of scientific and technological trends in a biorefinery and its sustainable development. This is based on identification of nodes and thematic clusters in network analysis bibliographic data. The patent document search in the Parentscope database was carried out and relevant technological trends were extracted. Taken from ref. 219. This is an open access article distributed under the terms of the Creative Commons Attribution License, which permits unrestricted use, distribution, and reproduction in any medium with due credit to the source. | |
Also, we have restricted ourselves to structure–function relationships while designing/engineering enzymes. The intrinsic disorder as a design element may pay dividends in the future.87,222 Related to this is our increasing understanding of protein condensates and protein–membrane interactions. Another dimension yet to be exploited is the translation of our growing understanding of enzyme behaviour in crowded environments to biotechnological applications.223 Real systems are not dilute substrate solutions in clean aqueous buffers but substrates which are often dirty suspensions/concentrated solutions and multiphasic in nature. It is highly likely that robotics and artificial intelligence/machine learning tools will play a role in shaping the bioeconomy in the future.224
Conflicts of interest
There are no conflicts to declare.
References
- P. Kafarski, Chemik, 2012, 66, 811–816 Search PubMed.
- M. C. S. Barcelos, F. B. Lupki, G. A. Campolina, D. L. Nelson and G. Molina, FEMS Microb. Lett., 2018, 365, fny239 CrossRef CAS PubMed.
- F. O. Adepoju, M. N. Ivantsova and O. N. Kanwugu, AIP Conf. Proc., 2019, 2174, 020199 CrossRef CAS.
-
P. T. Anastas and J. C. Warner, Green Chemistry: Theory and Practice, Oxford University Press, New York, 1958, p. 30 Search PubMed.
- P. T. Anastas and J. B. Zimmerman, Environ. Sci. Technol., 2003, 37, 94A–101A CrossRef PubMed.
- M. N. Gupta and S. Raghava, Chem. Central J., 2007, 1, 17 CrossRef PubMed.
-
R. J. Kazlauskas and B.-G. Kim, in Biocatalysis for Green Chemistry and Chemical Process Development, ed. J. A. Tao and R. Kazlauskas, Wiley, Hoboken, 2011, pp. 3–22 Search PubMed.
-
R. A. Sheldon, in Biocatalysis in Organic Solvents, ed. P. Lozano, Elsevier, Cambridge, USA, 2022, pp. 1–22 Search PubMed.
- H. E. Shoemaker, D. Mink and M. G. Wubbolts, Science, 2003, 299, 1694–1696 CrossRef PubMed.
- R. Wohlgemuth, Curr. Opin. Biotechnol., 2010, 21, 713–724 CrossRef CAS PubMed.
- T. Drepper, T. Eggert, W. Hummel, C. Leggewie, M. Pohl, F. Rosenau, S. Wilhelm and K.-E. Jaeger, Biotechnol. J., 2006, 1, 777–786 CrossRef CAS PubMed.
- S. Heux, I. Meynial-Salles, M. J. O'Donohue and C. Dumon, Biotechnol. Adv., 2015, 33, 1653–1670 CrossRef CAS PubMed.
- A. K. Pandey, V. K. Gaur, A. Udayan, S. Varjani, S.-H. Kim and J. W. C. Wong, Chemosphere, 2021, 272, 129936 CrossRef CAS PubMed.
- J. M. Woodley, Trends Biotechnol., 2008, 26, 321–327 CrossRef CAS PubMed.
-
Asymmetric Synthesis with Chemical and Biological Methods, ed. D. Enders and K.-J. Jaeger, Wiley-VCH, Weinheim, 2007 Search PubMed.
-
Green Chemistry Metrics: Measuring and Monitoring Sustainable Processes, ed. A. Lapkin and D. Constable, Wiley-Blackwell, Oxford, 2008 Search PubMed.
-
J. Clark, D. Macquarri, M. Gronnow and V. Budarin, in Process Intensification for Green Chemistry: Engineering Solutions for Sustainable Chemical Processing, ed. K. Boodhoo and A. Harvey, 2013, pp. 33–58 Search PubMed.
- R. A. Sheldon, Chem. Ind., 1992, 903–906 CAS.
- F. Roschangar, R. A. Sheldon and C. H. Senanayake, Green Chem., 2015, 17, 752–768 RSC.
- F. Tieves, F. Tonin, E. Fernández-Fueyo, J. M. Robbins, B. Bommarius, A. S. Bommarius, M. Alcalde and F. Hollmann, Tetrahedron, 2019, 75, 1311–1314 CrossRef CAS.
- P. D. de María, Green Chem., 2022, 24, 9620–9628 RSC.
- P. D. de María, EFB Bioecon. J., 2023, 3, 100056 CrossRef.
-
M. Lancaster, Green Chemistry: An Introductory Text, RSC Publishing, Cambridge, 2010 Search PubMed.
- J. J. Klemes, Curr. Opin. Chem. Eng., 2012, 1, 238–245 CrossRef CAS.
- K. K. Mulholland, R. W. Sylvester and J. A. Dyer, Environ. Prog., 2000, 19, 260 CrossRef CAS.
- M. N. Gupta and J. Mukherjee, Curr. Sci., 2013, 104, 1178–1186 CAS.
- J. Mukherjee and M. N. Gupta, Sustainable Chem. Proc., 2015, 3, 1–5 CrossRef CAS.
-
P. S. J. Cheetham, in Applied Biocatalysis, eds. A. J. J. Straathof and P. Adlercruetz, Harwood Academic Publishers, Amsterdam, 2000, pp. 399–434 Search PubMed.
- K. Mondal, I. Roy and M. N. Gupta, Anal. Chem., 2006, 78, 3499–3504 CrossRef CAS PubMed.
- S. Raghava, B. Barua, P. K. Singh, M. Das, L. Madan, S. Bhattacharyya, K. Bajaj, B. Gopal, R. Varadarajan and M. N. Gupta, Protein Sci., 2008, 17, 1987–1997 CrossRef CAS PubMed.
-
R. Datar and C.-G. Rosén, in Separation Processes in Biotechnology, ed. J. A. Asenjo, CRC Press, ebook, 2020, pp. 741–794 Search PubMed.
-
I. Roy, and M. N. Gupta, in Three Phase Partitioning. Applications in Separation and Purification of Biological Molecules and Natural Products, ed. M. N. Gupta and I. Roy, Elsevier, Amsterdam, 2021, pp. 175–196 Search PubMed.
- I. Roy, M. Sardar and M. N. Gupta, Biochem. Eng. J., 2005, 23, 193–198 CrossRef CAS.
- S. Teotia, K. Mondal and M. N. Gupta, Food Bioprod. Proc., 2006, 84, 37–43 CrossRef CAS.
- S. Gautam, P. Dubey, P. Singh, R. Varadarajan and M. N. Gupta, Anal. Biochem., 2012, 430, 56–64 CrossRef CAS PubMed.
- J. Porath, Protein Expr. Purif., 1992, 3, 263–281 CrossRef CAS PubMed.
- I. Roy and M. N. Gupta, Biotechnol. Appl. Biochem., 2000, 32, 81–87 CrossRef CAS PubMed.
- M. N. Gupta, S. Jain and I. Roy, Biotechnol. Prog., 2002, 18, 78–81 CrossRef CAS PubMed.
- C. L. Kielkopf, W. Bauer and I. L. Urbatsch, Cold Spring Harb. Protoc., 2020, 102194 CrossRef PubMed.
- T. G. Schmidt and A. Skerra, Nat. Protoc., 2007, 2, 1528–1535 CrossRef CAS PubMed.
- V. Mishra, Curr. Protein Pept. Sci., 2020, 21, 821–830 CrossRef CAS PubMed.
- F. Leonhardt, A. Gennari, G. B. Paludo, C. Schmitz, F. X. da Silveira, D. C. D. A. Moura, G. Renard, G. Volpato and C. F. Volken de Souza, 3 Biotech., 2023, 13, 186 CrossRef PubMed.
-
G. Walsh and D. R. Headon, Protein Biotechnology, Wiley, New York, 1994 Search PubMed.
- N. I. Majewska, M. L. Tejada, M. J. Betenbaugh and N. Agarwal, Annu. Rev. Chem. Biomol. Eng., 2020, 11, 311–338 CrossRef CAS PubMed.
-
G. Walsh, in Proteins: Biochemistry and Biotechnology, John Wiley & Sons, Ltd., Chichester, 2nd edn, 2014, pp. 311–326 Search PubMed.
- I. Roy, M. Sardar and M. N. Gupta, Enzyme Microb. Technol., 2000, 27, 53–65 CrossRef CAS PubMed.
- S. Teotia and M. N. Gupta, J. Chromatogr. A, 2004, 1025, 297–301 CrossRef CAS PubMed.
- S. Teotia, R. Lata and M. N. Gupta, J. Chromatogr. A, 2004, 1052, 85–91 CrossRef CAS PubMed.
- I. Roy, S. Jain, S. Teotia and M. N. Gupta, Biotechnol. Prog., 2004, 20, 1490–1495 CrossRef CAS PubMed.
- J. Mukherjee and M. N. Gupta, Int. J. Biol. Macromol., 2017, 97, 778–789 CrossRef CAS PubMed.
-
Three Phase Partitioning. Applications in Separation and Purification of Biological Molecules and Natural Products, ed. M. N. Gupta and I. Roy, Elsevier, Amsterdam, 2021 Search PubMed.
- A. Gershenson and F. H. Arnold, Genet. Eng., 2000, 22, 55–76 CAS.
- L. Dong, X. Lin, D. Yu, L. Huang, B. Wang and L. Pan, J. Ind. Microbiol. Biotechnol., 2020, 47, 133–144 CrossRef CAS PubMed.
- S. Gargiulo and P. Soumillion, Curr. Opin. Chem. Biol., 2021, 61, 107–113 CrossRef CAS PubMed.
- I. Victorino da Silva Amatto, N. Gonsales da Rosa-Garzon, F. Antônio de Oliveira Simões, F. Santiago, N. Pereira da Silva Leite, J. Raspante Martins and H. Cabral, Biotechnol. Appl. Biochem., 2022, 69, 389–409 CrossRef CAS PubMed.
- G. Feller, J. Phys. Condens. Matter, 2010, 22, 323101 CrossRef PubMed.
- C. Brininger, S. Spradlin, L. Cobani and C. Evilia, Semin. Cell Dev. Biol., 2018, 84, 158–169 CrossRef CAS PubMed.
- G. Feller and C. Gerday, Nat. Rev. Microbiol., 2003, 1, 200–208 CrossRef CAS PubMed.
- C. Struvay and G. Feller, Int. J. Mol. Sci., 2012, 13, 11643–11665 CrossRef CAS PubMed.
- S. Sahay and D. Chouhan, J. Genet. Eng. Biotechnol., 2018, 16, 319–325 CrossRef PubMed.
- V. L. Arcus and A. J. Mulholland, Annu. Rev. Biophys., 2020, 49, 163–180 CrossRef CAS PubMed.
- A. Kumar, S. Mukhia and R. Kumar, 3 Biotech, 2021, 11, 426 CrossRef PubMed.
- M. Kumari, S. Padhi, S. Sharma, L. C. Phukon, S. P. Singh and A. K. Rai, 3 Biotech, 2021, 11, 479 CrossRef PubMed.
- S. Tendulkar, A. Hattiholi, M. Chavadar and S. Dodamani, J. Biosci., 2021, 46, 64 CrossRef CAS PubMed.
-
R. N. Patel, Stereoselective Biocatalysis, Marcel Dekker, New York, 2000 Search PubMed.
- M. N. Gupta and I. Roy, Eur. J. Biochem., 2004, 271, 2575–2583 CrossRef CAS PubMed.
- S. Shah, A. Sharma and M. N. Gupta, Anal. Biochem., 2006, 351, 207–213 CrossRef CAS PubMed.
- S. Dalal, M. Kapoor and M. N. Gupta, J. Mol. Catal. B: Enzym., 2007, 44, 128–132 CrossRef CAS.
- A. B. Majumder, S. Shah and M. N. Gupta, Chem. Cent. J., 2007, 1, 10 CrossRef PubMed.
- S. Shah and M. N. Gupta, Bioorg. Med. Chem. Lett., 2007, 17, 921–924 CrossRef CAS PubMed.
- K. Solanki, M. N. Gupta and P. J. Halling, Bioresour. Technol., 2012, 115, 147–151 CrossRef CAS PubMed.
- A. Banerjee, V. Singh, K. Solanki, J. Mukherjee and M. N. Gupta, Sustainable Chem. Proc., 2013, 1, 14 CrossRef.
- K. Solanki and M. N. Gupta, Chem. Cent. J., 2008, 2, 2 CrossRef PubMed.
- M. N. Gupta, Eur. J. Biochem., 1992, 203, 25–32 CrossRef CAS PubMed.
- L. Cicco, G. Dilaurio, F. M. Perna, P. Vitali and V. Capriati, Org. Biomol. Chem., 2021, 19, 2558–2577 RSC.
-
P. Lozano, Biocatalysis in Green Solvents, Elsevier, Inc., London, 2022 Search PubMed.
- H. R. Hobbs and N. R. Thomas, Chem. Rev., 2007, 107, 2786–2820 CrossRef CAS PubMed.
- Z. Knez, J. Supercrit. Fluids, 2018, 134, 133–140 CrossRef CAS.
- S. Shah and M. N. Gupta, Process Biochem., 2007, 42, 409–414 CrossRef CAS.
- D. R. Padrosa, V. de Vitis, M. L. Contente, F. Molinari and F. Paradisi, Catalysts, 2019, 9, 232 CrossRef.
- B. H. Lipshutz, Curr. Opin. Green Sustain. Chem., 2018, 11, 1–8 CrossRef.
- H. Gröger, F. Gallou and B. H. Lipshutz, Chem. Rev., 2023, 123, 5262–5296 CrossRef PubMed.
- M. N. Gupta and I. Roy, Biol. Rev., 2021, 96, 205–222 CrossRef CAS PubMed.
- B. Arora, J. Mukherjee and M. N. Gupta, Sustainable Chem. Proc., 2014, 2, 25 CrossRef.
- M. Kapoor, A. B. Majumder and M. N. Gupta, Catal. Lett., 2015, 145, 527–532 CrossRef CAS.
- J. Mukherjee and M. N. Gupta, Biotechnol. Rep., 2015, 6, 119–123 CrossRef PubMed.
- T. L. Blundell, M. N. Gupta and S. E. Hasnain, Prog. Biophys. Mol. Biol., 2020, 156, 34–42 CrossRef CAS PubMed.
- M. N. Gupta, A. Alam and S. E. Hasnain, Biochimie, 2020, 175, 50–57 CrossRef CAS PubMed.
-
M. N. Gupta and V. N. Uversky, in Structure and Intrinsic Disorder in Enzymology, ed. M. N. Gupta and V. N. Uversky, Academic Press, London, 2023, pp. 241–277 Search PubMed.
- M. N. Gupta and V. N. Uversky, Cell. Mol. Life Sci., 2023, 80, 130 CrossRef CAS PubMed.
- M. Kapoor and M. N. Gupta, Process Biochem., 2012, 47, 555–569 CrossRef CAS.
- H. Yang, A. Jonsson, E. Wehtje, P. Adlercreutz and B. Mattiasson, Biochim. Biophys. Acta, 1997, 1336, 51–58 CrossRef CAS PubMed.
- A. Jonsson, W. van Breukelen, E. Wehtje, P. Adlercreutz and B. Mattiasson, J. Mol. Catal. B: Enzym., 1998, 5, 273–276 CrossRef CAS.
- A. Jonsson, E. Wehtje, P. Adlercreutz and B. Mattiasson, Biochim. Biophys. Acta, 1999, 1430, 313–322 CrossRef CAS PubMed.
-
M. N. Gupta and V. N. Uversky, in Structure and Intrinsic Disorder in Enzymology, ed. M. N. Gupta and V. N. Uversky, Academic Press, London, 2023, pp. 279–301 Search PubMed.
- M. N. Gupta, M. Kaloti, M. Kapoor and K. Solanki, Artif. Cells Blood Substit. Immobil. Biotechnol., 2011, 39, 98–109 CrossRef CAS PubMed.
-
P. Clapés, in Organic Synthesis Using Biocatalysis, ed. A. Goswami and J. D. Stewart, Academic Press, Cambridge, USA, 2015, pp. 285–337 Search PubMed.
-
Methods in Non-aqueous Enzymology, ed. M. N. Gupta, Birkhäuser Verlag, Basel-Boston-Berlin, 2000 Search PubMed.
-
R. Patel, Biocatalysis in the Pharmaceutical and Biotechnological Industries, CRC Press, Boca Raton, 2007 Search PubMed.
-
T. Godfrey and S. West, Industrial Enzymology, McMillan, London, 1996, pp. 146–149 Search PubMed.
- C. Branneby, P. Carlqvist, A. Magnusson, K. Hult, T. Brinck and P. Berglund, J. Am. Chem. Soc., 2003, 125, 874–875 CrossRef CAS PubMed.
- E. Busto, V. Gotor-Fernandez and V. Gotor, Chem. Soc. Rev., 2010, 39, 4504–4523 RSC.
- A. Patti and C. Sanfilippo, Int. J. Mol. Sci., 2022, 23, 2675 CrossRef CAS PubMed.
-
Process Intensification for Green Chemistry, ed. K. Boodhoo and A. Harvey, John Wiley & Sons, Ltd., Chichester, 2013 Search PubMed.
- I. Roy and M. N. Gupta, Curr. Sci., 2003, 85, 1685–1693 CAS.
- I. Roy and M. N. Gupta, Tetrahedron, 2003, 59, 5431–5436 CrossRef CAS.
- V. Singh, K. Solanki and M. N. Gupta, Proc. Indian Natl. Sci. Acad., 2012, 78, 629–635 CAS.
- Z. Zhu, Y. Liu, X. Yang, S. J. McQueen-Mason, L. D. Gomez and D. J. Macquarrie, Biomass Conv. Bioref., 2021, 11, 2681–2693 CrossRef CAS.
- A. Wasak, R. Drozd, D. Jankowiak and R. Rakoczy, Sci. Rep., 2019, 9, 3707 CrossRef PubMed.
- P. Giardina, V. Faraco, C. Pezzella, A. Piscitelli, S. Vanhulle and G. Sannia, Cell. Mol. Life Sci., 2010, 67, 369–385 CrossRef CAS PubMed.
- S. Horikoshi, K. Nakamura, M. Yashiro, K. Kadomatsu and N. Serpone, Sci. Rep., 2019, 9, 8945 CrossRef PubMed.
- G. Dik, A. Ulu, O. O. Inan, S. Atalay and B. Ates, Catal. Lett., 2023, 153, 1250–1264 CrossRef CAS.
- M. N. Gupta, M. Kapoor, A. B. Majumder and V. Singh, Curr. Sci., 2011, 100, 1152–1162 CAS.
- K. Kannan, J. Mukherjee and M. N. Gupta, Sci. Adv. Mater., 2013, 5, 1477–1484 CrossRef CAS.
- J. Mukherjee and M. N. Gupta, Chem. Centr. J., 2012, 6, 133 CrossRef CAS PubMed.
- J. Mukherjee, K. Solanki and M. N. Gupta, Methods Mol. Biol., 2013, 1051, 117–127 CrossRef CAS PubMed.
- J. Mukherjee and M. N. Gupta, Bioresour. Technol., 2016, 209, 166–171 CrossRef CAS PubMed.
- J. V. Sinisterra, Ultrasonics, 1992, 30, 180–185 CrossRef CAS PubMed.
- S. Neito, R. Villa, A. Donaire and P. Lozano, Ultrason. Sonochem., 2021, 75, 105606 CrossRef PubMed.
- A. F. C. Pacheco, L. B. de Souza, P. H. C. Paiva, C. A. Lelis, E. N. R. Vieira, A. A. L. Tribst and B. R. de Castro Leite Júnior, Appl. Food Res., 2023, 3, 100281 CrossRef CAS.
- N. Şener, D. K. Apar and B. Özbek, Process Biochem., 2008, 41, 1493–1500 CrossRef.
- K. V. K. Boodhoo, M. C. Flickinger, J. M. Woodley and E. A. C. Emanuelsson, Chem. Eng. Process., 2022, 172, 108793 CrossRef CAS.
-
K. Belafi-Bako and P. Bakonyi, in Biotechnology and Bioengineering, ed. E. Jacob-Lopes and L. Q. Zepka, IntechOpen, 2019, DOI:10.5772/intechopen.84513.
-
Effect of High-Pressure Technologies on Enzymes, ed. B. R. de Castro Leite Júnior and A. A. L. Tribst, Elsevier, Cambridge, USA, 2023 Search PubMed.
-
Carrier-bound Immobilized Enzymes. Principles, Applications and Design, ed. L. Cao, Wiley-VCH, Weinheim, 2005 Search PubMed.
-
Biocatalyst Immobilization: Foundations and Applications, ed. M. L. Ferreira, Elsevier, Cambridge, USA, 2023 Search PubMed.
- R. Tyagi, I. Roy, R. Agarwal and M. N. Gupta, Biotechnol. Appl. Biochem., 1998, 28, 201–206 CAS.
- I. Roy and M. N. Gupta, Chem. Biol., 2003, 10, 1161–1171 CrossRef CAS PubMed.
- I. Roy and M. N. Gupta, Biocatal. Biotransf., 2003, 21, 297–304 CrossRef CAS.
- M. Sardar, I. Roy and M. N. Gupta, Biotechnol. Prog., 2003, 19, 1654–1658 CrossRef CAS PubMed.
- S. Jain, I. Roy and M. N. Gupta, Artif. Cells Blood Substit. Immobil. Biotechnol., 2004, 32, 325–337 CrossRef CAS PubMed.
- I. Roy, M. Sardar and M. N. Gupta, Biochem. Eng. J., 2003, 16, 329–335 CrossRef CAS.
- I. Roy, S. Sharma and M. N. Gupta, Adv. Biochem. Eng. Biotechnol., 2004, 86, 159–189 CrossRef CAS PubMed.
-
I. Roy and M. N. Gupta, in Immobilization of Enzymes and Cells, ed. J. M. Guisan, Humana Press, Totowa, 2nd edn, 2006, pp. 87–96 Search PubMed.
- K. Mondal, P. Mehta, B. R. Mehta, D. Varandani and M. N. Gupta, Biochim. Biophys. Acta, 2006, 1764, 1080–1086 CrossRef CAS PubMed.
- K. Kannan, J. Mukherjee and M. N. Gupta, Chem. Lett., 2014, 43, 1064–1066 CrossRef CAS.
- M. N. Gupta, M. Perwez and M. Sardar, Biocatal. Biotransf., 2020, 38, 178–201 CrossRef CAS.
- J. Mukherjee, D. Malhotra, S. Gautam and M. N. Gupta, Ultrasonic. Sonochem., 2013, 20, 1054–1061 CrossRef CAS PubMed.
- M. Perwez, R. Ahmad and M. Sardar, Int. J. Biol. Macromol., 2017, 103, 16–24 CrossRef CAS PubMed.
- G. Orfanakis, M. Patila, A. V. Catzikonstantinou, K.-M. Lyra, A. Kouloumpis, K. Spyrou, P. Katapodis, A. Paipetis, P. Rudolf, D. Gournis and H. Stamatis, Front. Mater., 2018, 5, 25 CrossRef.
-
M. Sardar, M. Perwez, R. Ahmad, J. Mukherjee and M. N. Gupta, in Encyclopedia of Nanoscience and Nanotechnology, ed. H. S. Nalwa, 2018, vol. 28, pp. 1–30 Search PubMed.
- Y. Li, Y. Wu, S. A. Delbari, A. Kim, N. A. Sabahi, Q. Le, C. Xia, R. Luque, H. Jang, M. Shokouhimehr and R. Varma, Mol. Catal., 2023, 538, 113003 CrossRef CAS.
- S. Dalal and M. N. Gupta, Chemosphere, 2007, 67, 741–747 CrossRef CAS PubMed.
- S. Correa, S. Puertas, L. Gutiérrez, L. Asín, J. Martínez de la Fuente, V. Grazú and L. Betancor, PLoS One, 2019, 14, e0214004 CrossRef CAS PubMed.
-
Interfaces between Nanomaterials and Microbes, ed. M. N. Gupta, S. K. Khare and R. Sinha, CRC Press, Boca Raton, 2021 Search PubMed.
- I. Roy, J. Mukherjee and M. N. Gupta, Methods Mol. Biol., 2017, 1504, 109–123 CrossRef CAS PubMed.
- M. N. Gupta and V. S. Bisaria, J. Biosci. Bioeng., 2018, 126, 445–450 CrossRef PubMed.
- T. E. Abraham, J. R. Joseph, L. B. Bindhu and K. K. Jayakumar, Carbohydr. Res., 2004, 339, 1099–1104 CrossRef CAS PubMed.
- A. B. Majumder, K. Mondal, T. P. Singh and M. N. Gupta, Biocatal. Biotransf., 2008, 26, 235–242 CrossRef CAS.
- H. Tirunagari, S. Basetty, H. B. Rode and N. W. Fadnavis, J. Biotechnol., 2018, 282, 67–69 CrossRef CAS PubMed.
- R. O. Sena, C. Carneiro, M. V. H. Moura, G. C. Brêda, M. C. C. Pinto, L. X. S. G. M. Fé, R. Fernandez-Lafuente, E. A. Manoel, R. V. Almeida, D. M. G. Freire and E. P. Cipolatti, Int. J. Biol. Macromol., 2021, 189, 734–743 CrossRef CAS PubMed.
- R. A. Sheldon, R. Schoevaart and L. M. van Langen, Biocatal. Biotransform., 2005, 23, 141–147 CrossRef CAS.
- S. Dalal, A. Sharma and M. N. Gupta, Chem. Cent. J., 2007, 1, 16 CrossRef PubMed.
- P. F. Ávila, A. H. F. de Mélo and R. Goldbeck, Innov. Food Sci. Emerg. Technol., 2023, 85, 103335 CrossRef.
- B. Solomon, R. Koppel, G. Pines and E. Katchalski-Katzir, Biotechnol. Bioeng., 1986, 28, 1213–1221 CrossRef CAS PubMed.
- B. Mattiasson, Methods Enzymol., 1988, 137, 647–656 CAS.
- M. Saleemuddin, Adv. Biochem. Eng. Biotechnol., 1999, 64, 203–226 CrossRef CAS PubMed.
- M. Sardar, D. Varandani, B. Mehta and M. N. Gupta, Biocatal. Biotransf., 2008, 26, 313–320 CrossRef CAS.
- E. Parandi, M. Safaripour, N. Mosleh, M. Saidi, H. R. Nodeh, B. Oryani and S. Rezania, Biomass Bioenergy, 2023, 173, 106794 CrossRef CAS.
- P. Sundaram and W. Hornby, FEBS Lett., 1970, 10, 325–327 CrossRef CAS PubMed.
-
N. Holmes and R. A. Bourne, in Chemical Process Technology for a Sustainable Future, ed. T. M. Letcher, J. L. Scott and D. A. Paterson, RSC Publishing, Cambridge, 1st edn, 2014, pp. 28–45 Search PubMed.
- M. Kyrolainen, H. Hakonson, R. Ekorth and B. Mattiasson, Anal. Chim. Acta, 1993, 279, 149–153 CrossRef.
- J. Britton, S. Majumdar and G. A. Weiss, Chem. Soc. Rev., 2018, 47, 5891–5918 RSC.
- B. Baumer, T. Classen, M. Pohl and J. Pietruszka, Adv. Synth. Catal., 2020, 362, 2894–2901 CrossRef CAS.
- A. I. Benítez-Mateos, D. Roura Padrosa and F. Paradisi, Nat. Chem., 2022, 14, 489–499 CrossRef PubMed.
- S. N. Chanquia, A. Valotta, H. Gruber-Woel and S. Kara, Front. Catal., 2022, 1, 816538 CrossRef.
- L.-E. Meyer, M. Hobisch and S. Kara, Curr. Opin. Biotechnol., 2022, 78, 102835 CrossRef CAS PubMed.
- F. Paradisi, Catalysts, 2020, 10, 645 CrossRef CAS.
- M. Crotti, M. S. Robescu, J. M. Bolivar, D. Ubiali, L. Wilson and M. L. Contente, Front. Catal., 2023, 3, 1154452 CrossRef.
- H. Felix, Methods Enzymol., 1988, 137, 637–641 CAS.
- B. Lin and Y. Tao, Microb. Cell Fact., 2017, 16, 106 CrossRef PubMed.
- G. Torrelo, U. Hanefeld and F. Hollmann, Catal. Lett., 2015, 145, 309–345 CrossRef CAS.
- S. Raghava and M. N. Gupta, Anal. Biochem., 2009, 385, 20–25 CrossRef CAS PubMed.
- T. R. Ward and C. Copéret, Chem. Rev., 2023, 123, 5221–5224 CrossRef CAS PubMed.
- S. González-Granda, J. Albarrán-Velo, I. Lavandera and V. Gotor-Fernández, Chem. Rev., 2023, 123, 5297–5346 CrossRef PubMed.
- M. Gantz, S. Neun, E. J. Medcalf, L. D. van Vliet and F. Hollfelder, Chem. Rev., 2023, 123, 5571–5611 CrossRef CAS PubMed.
- A. Karim and M. Aider, Int. Dairy J., 2022, 126, 105249 CrossRef CAS.
- M. Pastore and F. Moorisi, Methods Enzymol., 1976, 44, 822–830 CAS.
- W. H. Pitcher Junior, J. R. Ford and H. H. Weetall, Methods Enzymol., 1976, 44, 792–809 Search PubMed.
- T. Finocchiaro, N. F. Olson and T. Richardson, Adv. Biochem. Eng., 1980, 15, 71–88 CAS.
- P. J. T. Dekker, D. Koenders and M. J. Bruins, Nutrients, 2019, 11, 551 CrossRef CAS PubMed.
- L. Lu, L. Guo, K. Wang, Y. Liu and M. Xiao, Biotechnol. Adv., 2020, 39, 107465 CrossRef CAS PubMed.
- C. Vera, C. Guerrero, C. Aburto, A. Cordova and A. Illanes, Biochim. Biophys. Acta, 2020, 1861, 140271 CrossRef PubMed.
- R. Dorau, P. Ruhdal and J. C. Solem, Appl. Microbiol. Biotechnol., 2021, 105, 4943–4955 CrossRef CAS PubMed.
- A. R. Madureira, C. I. Pereira, A. M. P. Gomes, M. E. Pintado and F. Xavier Malcata, Food Res. Int., 2007, 40, 1197–1211 CrossRef CAS.
- E. Akan, J. Food Sci. Technol., 2021, 58, 3743–3751 CrossRef CAS PubMed.
-
M. Santos-Hernandez, T. Kleekayal and R. J. FitzGerald, in Enzymes beyond Traditional Applications in Dairy Science and Technology, ed. Y. S. Rajput and R. Sharma, Elsevier, Cambridge, USA, 2023, pp. 189–232 Search PubMed.
- D. Pleissner and C. S. K. Lin, Sustainable Chem. Proc., 2013, 1, 21 CrossRef.
- O. Klein, S. Nier and C. Tamásy, Tijdschr Econ Soc Geogr, 2020, 113, 194–210 CrossRef.
-
United Nations Environment Programme, Food Waste Index Report 2021, Nairobi, 2021 Search PubMed.
-
A. Pandey, C. R. Soccol and C. Larroche, Current Developments in Solid-State Fermentation, Asiatech, New Delhi, 2007 Search PubMed.
-
J. P. López-Gómez, M. A. Manan and C. Webb, in Food Industry Wastes, ed. M. R. Kosseva and C. Webb, Elsevier, Inc., Amsterdam, 2nd edn, 2020, pp. 135–161 Search PubMed.
-
Recent Advances in White Biotechnology through Fungi, ed. A. N. Yadav, S. Singh, S. Mishra and A. Gupta, Springer, Cham, 2020 Search PubMed.
- N. D. Cerda-Cejudo, J. J. Buenrostro-Figueroa, L. Sepúlveda-Torre, C. Torres-León, M. L. Chávez-González, J. A. Ascacio-Valdés and C. N. Aguilar, Resources, 2023, 12, 36 CrossRef.
- Q. Li, P. Yi, J. Zhang, Y. Shan, Y. Lin, M. Wu, K. Wang, G. Tian, J. Li and T. Zhu, Environ. Sci. Pollut. Res., 2023, 30, 15325–15334 CrossRef CAS PubMed.
- C. Joshi, S. K. Khare and M. N. Gupta, Curr. Biochem. Eng., 2014, 1, 35–49 CrossRef.
- Y.-G. Lee, E.-J. Cho, S. Maskey, D.-T. Nguyen and H.-J. Bae, Molecules, 2023, 28, 3562 CrossRef CAS PubMed.
- N. Kondamudi, S. K. Mohapatra and M. Mishra, J. Agric. Food Chem., 2008, 56, 11757–11760 CrossRef CAS PubMed.
- R. Burton, X. Fan and G. Austic, Int. J. Green Energy, 2010, 7, 530–536 CrossRef CAS.
- V. Kumari, S. Shah and M. N. Gupta, Energy Fuels, 2007, 21, 368–372 CrossRef CAS.
- D. T. Johnson and K. A. Taconi, Environ. Prog., 2007, 26, 338–348 CrossRef CAS.
- M. Pagliaro, R. Ciriminna, H. Kimura, M. Rossi and C. D. Pina, Angew. Chem., Int. Ed., 2007, 46, 4434–4440 CrossRef CAS PubMed.
-
M. Pagliaro and M. Rossi, The Future of Glycerol, RSC Publishing, Cambridge, 2nd edn, 2010 Search PubMed.
-
White Biotechnology, ed. R. Ulber and D. Sell, Springer Verlag, Berlin, 2007 Search PubMed.
- A. Coombs, Nat. Biotechnol., 2007, 25, 953–954 CrossRef CAS PubMed.
- R. Dobson, V. Gray and K. Rumbold, J. Ind. Microbiol. Biotechnol., 2012, 39, 2017–2226 CrossRef PubMed.
- J. Pradima, M. R. Kulkarni and Archna, Resource-Efficient Technol., 2017, 3, 394–405 CrossRef.
- Y. Gu, J. Barrault and F. Jerome, Adv. Synth. Catal., 2008, 350, 2007–2012 CrossRef CAS.
- Y. Du, J. Gao, W. Kong, L. Zhou, L. Ma, Y. He, Z. Huang and Y. Jiang, ACS Omega, 2018, 3, 6642–6650 CrossRef CAS PubMed.
- S. Sun, Y. Lv and G. Wang, Ind. Crops Prod., 2020, 151, 112470 CrossRef CAS.
- S. Sinha and P. Tripathi, Case Stud. Chem. Environ. Eng., 2021, 4, 100162 CrossRef CAS.
-
W. Shah-Neville, https://www.labiotech.eu/in-depth/biotechnology-developing-countries/, accessed on August 2, 2023.
- M. Sharma and R. Swarup, Adv. Biochem. Eng. Biotechnol., 2003, 84, 1–48 CrossRef PubMed.
- M. N. Gupta and J. Mukherjee, Proc. Indian Natl. Sci. Acad., 2015, 81, 1113–1132 Search PubMed.
- H. Kawaguchi, K. Takada, T. Elkasaby, R. Pangestu, M. Toyoshima, P. Kahar, C. Ogino, T. Kaneko and A. Kondo, Bioresour. Technol., 2022, 344, 126165 CrossRef CAS PubMed.
-
C. Dalastra, N. Klanovicz, S. Kubeneck, F. S. Stefanski, D. F. Argenta, G. S. Rauber, T. Caon, R. D. Cadamuro, G. Fongaro and H. Treichel, in Polysaccharide- Degrading Biocatalysts, ed. R. Goldbeck and P. Poletto, Elsevier, Cambridge, USA, 2023, pp. 409–434 Search PubMed.
- M. Kordi, R. Salami, P. Bolouri and N. Delangiz, Syst. Microbiol. Biomanuf., 2022, 2, 413–429 CrossRef CAS.
- K. Subramanian, M. K. Sarkar, H. Wang, Z. H. Qin, S. S. Chopra, M. Jin, V. Kumar, C. Chen, C. W. Tsang and C. S. K. Lin, Crit. Rev. Env. Sci. Technol., 2021, 52, 3921–3942 CrossRef.
- A. Barragán-Ocaña, H. Merritt, O. E. Sánchez-Estrada, J. L. Méndez-Becerril and M. del Pilar Longar-Blanco, PLoS One, 2023, 18, e0279659 CrossRef.
- P. W. Gerbens-Leenes, Environ. Process, 2018, 5, 167–180 CrossRef CAS.
- É. S. M. Pinto, M. Dorn and B. C. Feltes, Chemosphere, 2020, 250, 126202 CrossRef PubMed.
-
Structure and Intrinsic Disorder in Enzymology, ed. M. N. Gupta and V. N. Uversky, Academic Press, London, 2023 Search PubMed.
- M. N. Gupta and V. N. Uversky, Int. J. Mol. Sci., 2023, 24, 2424 CrossRef CAS PubMed.
- T. H. Tsui, M. C. M. van Loosdrecht, Y. Dai and Y. W. Tong, Bioresour. Technol., 2023, 369, 128445 CrossRef CAS PubMed.
Footnote |
† Current address: 508, Block 3, Kirti Apartments, Mayur Vihar Phase 1 Extension, Delhi 110091, India. |
|
This journal is © The Royal Society of Chemistry 2023 |