DOI:
10.1039/D3SU00126A
(Critical Review)
RSC Sustain., 2023,
1, 2120-2134
A review on polyhydroxyalkanoate (PHA) production through the use of lignocellulosic biomass
Received
26th April 2023
, Accepted 15th September 2023
First published on 20th September 2023
Abstract
Polyhydroxyalkanoates (PHAs) are aliphatic polyesters produced via bacterial fermentation of sugars and fatty acids and are considered to be a promising material to aid in mitigating plastic pollution due to their universal biodegradability. With an expected 12 billion metric tons of plastic to accumulate within landfills and ecosystems by 2050, this mitigation is desperately needed. The major bottleneck in the commercial feasibility of PHAs is the high cost compared to their non-biodegradable synthetic counterparts currently predominant throughout the packaging and single-use goods industry, with PHAs costing 3–12× more than traditional plastics. With approximately 50% of the production costs attributed to the fermentation substrate, a significant opportunity exists to dramatically cut the cost of PHA production through the use of a low or no-cost substrate. These biodegradable polymers can be sustainably produced from a variety of waste materials. Lignocellulosic biomass is the most abundant natural material on earth and can potentially be used for this purpose. This review includes an overview of the PHA production process such as metabolic pathways, reactor configurations, and pre-treatments for lignocellulosic biomass. The results from various studies using lignocellulosic biomass for PHA production were compared. Finally, potential drawbacks and opportunities are discussed for the viability of integrating the sustainable production of PHAs into the global circular economy. This work satisfies the UN's sustainability goal of responsible consumption and production (Sustainable Development Goal 12).
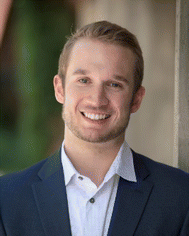 Peter Zytner | Peter Zytner completed his Bachelor of Engineering and Master of Applied Science degrees in Biological Engineering at the University of Guelph. His research interests and area of focus include biopolymer synthesis, biodegradation, sustainable industry development, and polymer processing. Throughout his studies Peter has published six academic journal articles and presented at five conferences. Mr Zytner was awarded an Ontario Ministry of Agriculture, Food, and Rural Affairs (OMAFRA) Highly Qualified Personnel (HQP) scholarship during his graduate studies for his research focus on integrating the Canadian agri-food economy with sustainable plastic use and production. |
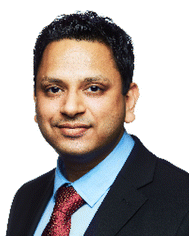 Deepak Kumar | Dr Deepak Kumar is an Assistant Professor in the Chemical Engineering Department at the State University of New York College of Environmental Science and Forestry (SUNY-ESF). His research focuses on the development of sustainable bioprocess technologies for the production of high-value bioproducts, bioplastics, and biofuel from agri-food processing waste and forest residues using integrated experimental and modeling approaches. He has authored more than 70 peer-reviewed research articles and 13 book chapters, with over 3200 citations and an H-index of 26. He has received several awards, including the Bioenergy Society of Singapore (BESS) Achievement Award 2016. |
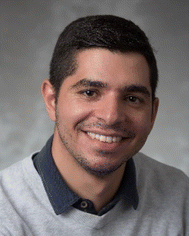 Abdallah Elsayed | Dr Abdallah Elsayed is an Associate Professor at the University of Guelph with a research focus on materials for the automotive, biomedical, and environmental sectors. Abdallah has more than 40 journal publications, two patents, and one book chapter. Abdallah is a professional engineer and chair of the ASM Ontario Chapter as well as member of TMS and CIM. Abdallah was the 2022 recipient of the ASM Bronze Medal, 2020 recipient of the ASM Bradley Stoughton Award for Young Teachers, 2020 TMS Light Metals Division Young Leaders Professional Development Award winner and selected as a CIM Emerging Professional in 2020. |
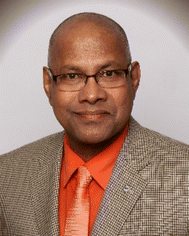 Amar Mohanty | Prof. Amar Mohanty is a Distinguished Research Chair in Sustainable Biomaterials at the Ontario Agriculture College and the Director of the Bioproducts Discovery and Development Centre. Dr Mohanty is the Editor-in-Chief of Sustainable Composites @ Composites Part C – Open Access. He is one of the most cited researchers worldwide with more than 800 publications to his credit, including 470 peer-reviewed journal papers, 70 patents (awarded/applied), 6 edited books, 30 book chapters, 300+ conference papers and over 160 plenary and keynote presentations. His Google-Scholar citations count is 53 274 and h-index 104 (October 14, 2023). |
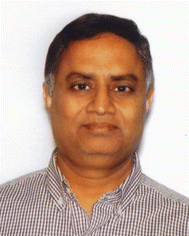 B. V. Ramarao | Prof. Bandaru V. Ramarao is a professor and chair of the Department of Chemical Engineering at the State University of New York College of Environmental Science and Forestry in Syracuse New York. He was an editor of the Elsevier Journal Separation and Purification Technology and is co-author of Granular Filtration of Aerosols and Hydrosols. He has co-authored more than 90 journal publications and has chaired several conferences and meetings. Dr Ramarao is an elected Fellow of the American Institute of Chemical Engineers and received the Institute’s Andrew Chase Award from the Forest Bioproducts Division. |
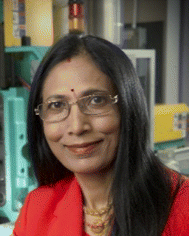 Manjusri Misra | Dr Manjusri Misra is a professor and Tier 1 Canada Research Chair (CRC) in Sustainable Biocomposites in the School of Engineering and holds a joint appointment in the Department of Plant Agriculture at the University of Guelph. She is also the Research Program Director of the Bioeconomy Panel for the Ontario Agri-Food Innovation Alliance, a program between the Ontario Ministry of Agriculture, Food, and Rural Affairs (OMAFRA) and the University of Guelph She is a Fellow of the Royal Society of Chemistry (UK), the American Institute of Chemical Engineers (AIChE), and the Society of Plastic Engineers (SPE). |
Sustainability spotlight
Biodegradable polymers have the potential to alleviate a significant strain on the environment inflicted by synthetic, non-biodegradable options. The polyhydroxyalkanoate (PHA) family of polymers potentially have a key role to play in this advancement due to their universal biodegradability but are hindered by their high cost of production and poor mechanical properties. This review examines the current state of research being conducted on producing PHAs in a cost-effective manner through utilizing lignocellulosic biomass. By pre-treating biomass such as farm residue and food processing waste, these abundant resources can dramatically reduce the cost of PHA production as a low-cost feedstock. This work aligns with the UN sustainability goal SDG 12 “Responsible consumption and production”.
|
1 Introduction
Since their widespread adoption in the 1950s, plastics have revolutionized materials used in everyday life.1,2 While the effective use of plastic has lowered the cost of many products, there is a growing concern regarding the negative impacts that increased plastic production and improper disposal are having, and will continue to have, on the environment. Immediate environmental problems and those looming in the near future have caused industry and academia alike to innovate and develop solutions.
Since the 1950s, global plastic production has grown at an average rate of approximately 9% per year, with expected global production reaching 540 million metric tonnes by 2040.3,4 Despite this significant production growth, it is believed that a meagre 14% of plastic produced annually is effectively recycled.5 Because synthetic polymers do not biodegrade, these non-recycled plastics will remain in landfills or the environment for several hundred years.6 With the continuation of current trends, it is expected that by 2050, about 12 billion metric tons of plastic waste would accumulate in landfills or the natural environment, and GHG emissions from plastics would account for 15% of the global carbon budget.2,7
Once in the natural environment, plastics either do not break down at all or will break down extremely slowly, releasing toxic chemicals and microplastics into the environment.8 This can cause immediate injury to wildlife in the form of a choking hazard or a more passive manner by allowing these by-products to permeate into their bodies. This has widespread impacts on the food chain, as these compounds will not break down within the body but continuously travel up the food chain instead.9 Recent studies by Ragusa et al. have detected microplastics in both human placenta and breast milk, highlighting the urgent nature of this issue.10,11 These accumulating hazards have encouraged the development of biopolymers, capable of biodegrading in various environments. Bio-based polymers are polymers that are produced by a naturally renewable resource. These are a subcategory of sustainable polymers and can be produced through the utilization of plants, vegetable oils, microbial metabolism, and more.12,13
Polyhydroxyalkanoates (PHAs) are among the most popular bio-based polymers as they can be produced by a variety of microbes under growth-limited conditions and are fully degradable in both soil and marine environments. This biodegradability makes them an attractive option for replacing common petroleum-based polymers such as polyethylene (PE), polyethylene terephthalate (PET) and polypropylene (PP). This is particularly the case in many single-use applications such as cutlery and packaging. However, the major limitations of the currently produced PHAs, especially short-chain PHAs, are their poor mechanical properties and their high production costs.14 While the mechanical properties can be improved via producing medium chain PHAs and polymer blending, the high production cost is of greater concern.15–19 Currently, PHAs are produced from the fermentation of sugars and fatty acids extracted from food crops, such as corn starch, sugarcane, and vegetable oil. The feedstock cost itself accounts for more than 50% of the production cost. To combat this, abundant and low or zero-cost lignocellulosic biomass, such as agricultural residues, forest residues, agro-food processing waste, and dedicated energy crops, can be used as a carbon source for PHA production.
There is a vast abundance of these lignocellulosic feedstocks available for use and this has led to the development of various technologies to use these feedstocks in a variety of applications such as energy, biorefinery, and value-added material.20 Lignocellulosic feedstocks are mainly composed of carbohydrates (cellulose and hemicellulose) and lignin. Cellulose is the most abundant biopolymer on earth while lignin is ranked second.21 In addition to conventional lignocellulosic feedstocks, food waste generated in processing facilities, farms, restaurants, and households, is another high potential feedstock for PHA production.22 In fact, it is estimated that approximately one-third of food produced worldwide is wasted.23 This would allow for the sustainable production of biodegradable polymer products with no impact on the global food demand. Therefore, the use of food waste for PHA production would not impact the human food supply chain in any capacity.24 Sustainable production of consumer products must be a key focus for the polymer industry moving forward to prevent the depletion of critical natural resources.
Although lignocellulosic feedstocks are promising alternatives to currently used food crops for PHA production, several challenges exist in terms of low PHA yields, need of pre-treatment, inhibition during fermentation, and downstream recovery of PHAs. This review presents a detailed discussion on several important topics, such as reactor configurations, microbial metabolic pathways, extraction methods, and lignocellulosic biomass pre-treatment strategies. Studies that have used various types of lignocellulosic biomass are discussed and the results are compared, with an emphasis on studies producing poly(3-hydroxybutyrate) PHB and poly(hydroxybutyrate-co-3-hydroxyvalerate) PHBV. A brief discussion on the techno-economic feasibility is also provided. Finally, some challenges and prospects are discussed.
2 Polyhydroxyalkanoates
Polyhydroxyalkanoates (PHAs) are bacterially produced aliphatic polyesters well known for safely biodegrading in any environment. They are made as energy storage molecules by microbes that accumulate within bacteria cytoplasm. The ability of PHAs to biodegrade in soil, compost, or water in a non-toxic manner has made them highly appealing for use as a replacement for synthetic polymers throughout various industries, particularly in packaging and other single-use products.25
2.1 Classification of PHAs
Defined by the carbon chain length of the 3-hydroxyalkanoate monomer, over 100 types of PHA monomers have been identified to date, allowing for several thousand potential co-polymer combinations.26 PHAs are classified as either short chain length (scl; 4–5 carbon atoms), medium chain length (mcl; 6–14 carbon atoms), or long chain length (lcl; 15+ carbon atoms). PHAs can also exist as copolymers, consisting of both mcl- and scl-PHAs, and having superior properties compared to homopolymers. The most widely used are those of the scl-variety, poly(3-hydroxybutyrate) (PHB) and poly(3-hydroxyvalerate) (PHV), as well as their co-polymer poly(hydroxybutyrate-co-3-hydroxyvalerate) (PHBV). Table 1 lists common PHAs and their respective C chain length. Fig. 1 shows the general monomer structure for PHAs. This review will focus on scl-PHAs as they are the most prevalent throughout industrial production and are more widely available.
Table 1 List of most common PHAs and their constituent monomer
Classification |
Polymer |
Functional group |
Acronym |
# of C |
Short chain |
Poly(3-hydoxypropionate) |
3HP |
PHP |
3 |
Poly(3-hydroxybutyrate) |
3HB |
PHB |
4 |
Poly(3-hydroxyvalerate) |
3HV |
PHV |
5 |
Poly(3-hydroxybutyrate-co-3-hydroxyvalerate) |
3HB/3HV |
PHBV |
4 and 5 |
Medium chain |
Poly(3-hydroxyhexanoate) |
3HHx |
PHHx |
6 |
Poly(3-hydroxyoctanoate) |
3HO |
PHO |
8 |
Poly(3-hydroxynonanoate) |
3HN |
PHN |
9 |
Poly(3-hydroxydecanoate) |
3HD |
PHD |
10 |
Poly(3-hydroxydodecanoate) |
3HDD |
PHDD |
12 |
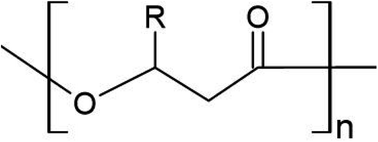 |
| Fig. 1 General structure of PHA monomer. R represents where a functional group extends the polymer depending on the total chain length. | |
2.2 Synthesis of PHAs
It is essential first to understand the fundamentals of PHA synthesis. PHAs can be produced in three ways: fermentation of sugars and fatty acids by microbes, synthesis within genetically modified plants, and enzymatic catalysis. Of these options, fermentation has been determined to be the most cost-effective and reliable approach for PHA synthesis mainly because of relatively high PHA yields. Common substrates for the synthesis of PHAs include simple sugars and oils, such as glucose and glycerol. Table 2 contains several studies showing the potential yields using these high purity substrates. A staggering 300 bacterial species have been reported to be capable of producing PHAs, in both aerobic and anaerobic environments and extreme locales.27 PHAs have been formed by both Gram-positive and Gram-negative species. The most common varieties researched include Bacillus megaterium, Ralstonia eutropha, Cupriavidus necator, and Pseudomonas putida, along with others from the genera Azotobacter, Syntrophomonas, Aeromonas, and Clostridium.28–31 Bacterial strains are typically sorted into two categories, those which require a nutrient-deficient environment during the stationary phase of fermentation or those that produce PHAs during the growth phase with a surplus of nutrients available.32 Bacteria that require a nutrient-deficient environment include R. eutropha, P. putida, and P. oleovorans.33 Those which do not require such an environment include E. coli and A. latus.34 A growing body of work exists that utilizes recombinant or genetically engineered bacteria for fermentation.35,36 A popular choice for these genetic modification studies is E. coli due to its ease of being modified, which is a front-runner for widespread industry adoption.37 Bacterial strains can be modified to suit a variety of different purposes, such as improved substrate utilization, altered metabolic pathways, and gene expression modification.34,38–40 These various modifications can transform E. coli into potent and productive producers for the PHA industry. The proposed lifecycle for the sustainable production of PHAs can be found in Fig. 2. Within this lifecycle, waste lignocellulosic biomass from a variety of sources can be utilized and subsequently pretreated, fermented, and processed into PHA products. Upon disposal, ideally to municipal industrial composting facilities, the PHAs can then degrade and be integrated with the created compost and be used to enrich soil which assists in growing further lignocellulosic biomass. In the event the PHAs are improperly disposed of into the natural environment, the universal biodegradability of the products will help to minimize the environmental impact of that waste.
Table 2 Studies utilizing common PHA feedstocks and their potential yields
Substrate |
Microorganism |
PHA g L−1 |
PHA yield (%) |
Ref |
Glucose |
Bacillus sp |
3.09 |
— |
41
|
Glucose |
C. necator DSM 545 |
112 |
76 |
42
|
Glucose |
H. venusta KT832796 |
33 |
88 |
43
|
Crude glycerol |
Alphaproteobacteria |
0.24 |
80 |
44
|
Betaprotobacteria |
Glycerol |
Z. denitrificans MW1 |
55 |
67 |
45
|
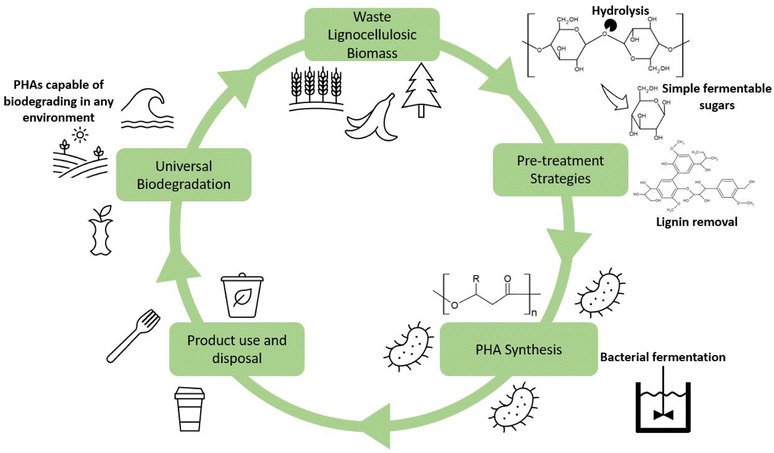 |
| Fig. 2 Potential lifecycle of PHAs through utilization of lignocellulosic waste as feedstock. | |
2.2.1 Metabolic processes.
PHA accumulates in small granules within the cytoplasm of bacteria, in particles ranging from 0.2–0.5 μm. Three metabolic pathways exist by which bacteria can synthesize PHAs. The path the metabolites take can branch depending on the feedstock or bacterial strain used in the process. A significantly more detailed exploration of the metabolic pathways used for PHA production can be found in a recent review conducted by Choi et al.46
2.2.1.1 Pathway I.
The first pathway is for the synthesis of scl-PHAs. Sugar, fatty acid, or amino acid molecules are converted to two acetyl-CoA molecules, followed by two hydroxybutyryl-CoA molecules by β-ketoacyl-CoA thiolase (PhaA) and acetoacetyl-CoA reductase (PhaB), which are subsequently polymerized into PHB by P(3HB) polymerase (PhaCscl) via a condensation reaction.47,48 Common scl-PHAs created in this fashion are PHB, PH4B, PHP, PHBP.49 The addition of propionic acid can be used to facilitate the production of co-polymer PHBV, as the propionyl-CoA formed via this same pathway is the precursor to 3-hydroxyvalerate.50–52
2.2.1.2 Pathway II.
The second pathway relies on the degradation of fatty acid molecules via β-oxidation to create mcl-PHAs.50 During this process, fatty acids are converted to enoyl-CoA, then to R-3-hydroxyacyl-CoA by R-3-hydroxyacyl-CoA hydratase, which is finally formed into an mcl-PHA by mcl-PHA synthase (PhaCmcl). Mcl-PHAs formed in this manner generally include PHHx, PHO, and PHN.49
2.2.1.3 Pathway III.
The third pathway for PHA synthesis can utilize either R-3-hydroxyacyl-ACP from in situ fatty acid synthesis or R-3-hydroxyacyl-CoA from β-oxidation. R-3-hydroxyacyl-ACP is converted to R-3-hydroxyacyl-CoA via enzyme PhaG and made available to PhaC for polymerization into various mcl-PHAs depending on the substrate provided to the culture.53
2.2.2 Nutrient limitation.
As previously stated, most PHA-producing bacteria require a nutrient-deficient environment. Various nutrients have vital roles in cellular growth, metabolism, and PHA production. PHAs are synthesized by bacteria as an energy storage molecule, which will only be done during a state of excess energy (carbon source) while being deficient in the means for cellular growth (nitrogen, phosphorous). To do this, nutrient-deficient conditions are often created in a two-step fermentation process; the growth phase and the production or PHA accumulation phase. The growth phase allows for maximal biomass production, followed by a reduction in nitrogen and/or phosphorus depending on the strain of bacteria used. Once enough biomass has been accumulated, the nitrogen and phosphorus feed rates are brought down to a minimum. Key molecules and structures such as ATP, DNA, and cell membranes require a steady supply of P and N nutrients, without them biomass cannot continue to grow.54 This state of nutrient deficiency ensures that all excess carbon is utilized for PHA synthesis instead of biomass growth and has been observed in various species to improve PHA productivity significantly, with many studies reporting a nearly 2–3 fold increase in PHA production once in a nutrient deficient state.55–57
2.2.3 Fermentation configurations.
The productivity of any fermentation process is highly dependent on the reactor configuration and strategy. The two main categories of fermentation are continuous and non-continuous. Non-continuous methods include batch and fed-batch configurations, while continuous can have either one or several steady-state reactors in series. Most lab scale studies are conducted with batch or fed-batch configurations, and many industrial-size applications are conducted with fed-batch or continuous setups.
2.2.3.1 Batch.
Batch cultivations are so named as they occur in discrete volumes and must be reset following each fermentation. All nutrients are added at the beginning of the operation, and all products are collected at the end. These reactor configuration styles tend to result in a low PHA yield as it is challenging to maintain optimal nutrient concentration for optimal polymer synthesis throughout fermentation. Additionally, it has been observed that the PHAs produced often suffer from degradation and are metabolized by the microbes towards the end of the fermentation as other carbon sources are depleted.58 It is unlikely that future production of PHAs on an industrial scale will utilize a batch-style configuration.
2.2.3.2 Fed-batch.
Fed-batch fermentations significantly improve upon traditional batch fermentations in several ways. The ability to modify the feed and nutrient rates during the process grants a significant amount of flexibility not afforded by the batch style. In a fed-batch system, PHA production occurs in two phases. The first is the growth phase, in which the biomass is grown to a desired level by providing all the required nutrients, such as carbohydrates, nitrogen, and phosphorus. The C source dramatically increases in the second phase while the N and P levels are reduced.59 It is possible to generate high biomass yields and promising carbon conversion efficiency using this method, giving fed-batch fermentations the possibility of being utilized at a greater scale. The major drawback of this reactor configuration is that they eventually need to be stopped, as repeatedly adding volume to a reactor of fixed size will eventually result in a full vessel. Stopping, resetting, and resuming a study increases the risk of contamination within the reactor.
2.2.3.3 Continuous.
Continuous reactor configurations are so named as nutrients are continuously pumped in, and products are removed throughout fermentation. This is done at an equal rate, keeping the working volume of the reactor at a steady state the entire time. Furthermore, reactors can be placed in series such that the products of one reactor become the feedstock of the next in the system. These are known as multi-stage fermentations and have the potential to create very high PHA yields. In a typical two-stage setup, biomass accumulation occurs in the first reactor under normal conditions. The biomass is then moved at a specific rate to the second reactor, in which there are nutrient limitations. This drives the production of PHAs with great effectiveness. This concept can be used with a series of reactors, sometimes even as many as 5, as demonstrated by Atlić et al.60 In this situation, the first reactor is used for biomass growth, and the subsequent 4 are for polymer synthesis. These continuous systems likely hold the most potential for industrial-scale production of PHAs due to their advantages by nature of being continuously operating. As the process continually runs, the requirement to entirely shut the system down for cleaning is much less frequent than in a batch or fed-batch system. This cuts the downtime of the system significantly. This also has the benefit of reducing the likelihood of microbial contamination.
2.2.4 Extraction methods.
While the goal of PHA production is to accumulate as much polymer as possible, this is of no value unless most or all of it can be extracted efficiently and at high purity. This critical step of the process also has the ability to have the harshest impact on the environment, as the use of chemicals is widespread in the polymer manufacturing industries. There are two main methods to harvest PHAs from cells. The first is the use of solvents to dissolve the PHA directly and then precipitate them out at a later stage. The second is dissolving the biomass through the use of various chemicals and/or enzymes, leaving just the PHA granules behind. Factors requiring consideration when selecting an extraction method include their economic feasibility, safety, energy cost, product yield, environmental impact, and ability to be scaled up.61 Additional factors to be considered include the method's impact on the polymer itself, such as the molecular weight.62 The following sections will compare various extraction methods used for PHA synthesis. For a much more in-depth review of these extraction methods and more, please see the recent review by Kurian et al.63
2.2.4.1 Solvent recovery.
This is an effective and common method used for PHA recovery. A solvent is used to rupture the cell membrane, making it easier for chemicals to gain access to the PHA granules inside the cell. Acetone and chloroform are the most popular choices of solvent as they effectively dissolve the PHA without degrading it. Following dissolution, a second reagent is added to precipitate the polymer. Cold ethanol or methanol are the primary choices for this step due to low cost and effectiveness. While very effective, this method of extraction is unlikely to be very favorable in the future. Chloroform is extremely toxic, and many countries have banned the use of it for processing consumer goods altogether. Further drawbacks include the often high energy input, as many of these dissolution processes require a solvent temperature exceeding 100 °C.64
2.2.4.2 Green solvent recovery.
Unlike traditional solvent recovery, green solvents have the benefit of requiring significantly less energy input, less toxic chemicals, and mild processing conditions.65 There are 6 principles of green extraction as defined by Chemat et al., the most significant being that this mode of extraction should produce useful coproducts instead of waste, and that the solvent itself should be biodegradable once extraction is complete.65 Yabueng et al. put these principles into practice in their study of extracting PHB with the green solvent 1,3-dioxolane.66 Adding water to the 1,3-dioxolane suspension was able to drop the PHB out of the solution, resulting in a 93% recovery yield of PHB from C. necator. Green solvents have the potential for widespread use as a PHA extraction method, however, more work is required to fill in the knowledge gaps that exist. Studying more green solvents at a larger scale will give insight into whether this will be a viable strategy moving forward.
2.2.4.3 Enzymatic recovery.
The goal of this method is to digest the biomass, leaving only the PHA granules behind. Alkali chemicals have also been used in the past for this purpose although with a significantly greater environmental impact. As reported by Kapritchkoff et al., proteolytic enzymes could digest the biomass of R. eutropha, effectively purifying and extracting the PHB produced.67 Interestingly, they determined that using two different enzymes in the sequence was able to further improve the yields. Using only one enzyme (bromelain) resulted in 61% PHB yield while using a second enzyme (pancreatin) sequentially resulted in a yield of 90% while being one-third of the cost of using only bromelain.67 While this work used very specific enzymes, another study used a very different approach by utilizing a vast cocktail of enzymes produced by A. orzyzae to purify PHBV.68 Kachrimanidou et al. were able to garner impressive yields with this setup, with a recovery yield of 98% and purity of 97%.68 While enzymes have been shown to have the potential to extract PHAs with high yield and great purity, the barrier to their widespread adoption is the high price, similar to PHAs themselves. Enzymes can add upwards of $10 per kg of PHA processed if purchased directly from a supplier, while the price of cultivating your own in-house such as Kachrimanidou et al. is unknown. Further work will need to be done with enzymes to understand their potential in PHA extraction and methods to bring down their production costs.
2.2.4.4 Animal recovery methods.
New work has challenged the concept of needing chemicals in the extraction process, and instead looks to the animal kingdom for help by recruiting various mammals and insects. The main theory behind this method is that animals will be able to digest the cellular biomass produced during fermentation but not the PHA component stored within the cell. Therefore, when the cells are consumed, the PHA is later excreted in the feces of the studied animal. The polymer then simply needs to be washed and heated to be ready for use. This has been done with both mealworm larvae and lab rats, with impressive results. Mealworm larvae feces, when simply rinsed with water, yielded a PHA purity of 89%.69 Lab rats showed similar positive results, with a purity of 89%.70 While this is an extremely interesting approach to PHA extraction, the biggest drawback of this method is the cost and scalability of sustaining the space needed for the animals used in this process. It is recommended that future work continues on this subject to further examine its potential and scalability.
2.3 Barriers to PHA adoption
Despite the attractive biodegradable properties of PHAs, they remain uncompetitive within wider industries. This can be attributed to poor mechanical performance and significantly higher costs than their synthetic counterparts. The mechanical downfalls can be compensated through strategies such as polymer blending and processing.16–19,71 While a proven effective method, this is beyond the scope of this review. Therefore, the most significant hurdle preventing the widespread adoption of PHAs throughout the industry is their higher cost. With synthetic plastic production costing approximately $1250 USD/Mt, PHA production falls between $4000 and $15000 USD/Mt.72 This wide gap in costs is prohibitive in the eyes of businesses that require significant volumes of polymers each year. Of the production cost, the carbon substrate used as feedstock for the microbial cultures accounts for approximately 50% of the total cost.73 These high feedstock costs can be attributed to the use of pure glucose or sucrose as the energy source for bacterial cultures. In addition to the use of a pure substrate, sterile conditions are used mainly featuring only one species of bacteria. Additional efforts to reduce the cost of production include the use of mixed microbial cultures (MMCs) in non-sterile conditions. This maximizes yields and consistency, as the substrate used will often determine the specific PHA produced. A significant focus has been placed on using low or no-cost waste streams from various industries to serve as the carbon source for the production process while still producing a solid yield of high purity.74–76 Waste feedstocks can be generated from various sources within the agrifood industry, such as food processing facilities, farms, and animal by-products.
3 Lignocellulosic biomass
The 200 billion tonnes of lignocellulosic biomass produced each year globally makes it the most abundantly produced raw material on Earth and provides an incredible opportunity for use in value-added processes such as biofuels or sustainable materials.77 The primary makeup of lignocellulosic biomass has three main constituents being cellulose, hemicellulose, and lignin with a small fraction of inorganic ash.78 The chemical structure of the main components of concern, cellulose and lignin, are depicted in Fig. 3. The exact ratio of these components is highly dependent on the plant species, as woody species will have a higher lignin content. In contrast, leafy plants will be considerably lower in lignin content. However, the typical range falls within 40–50% cellulose, 20–40% hemicellulose, and 10–40% lignin.79Table 3 presents the cellulose, hemicellulose, and lignin content of a variety of lignocellulosic biomasses.
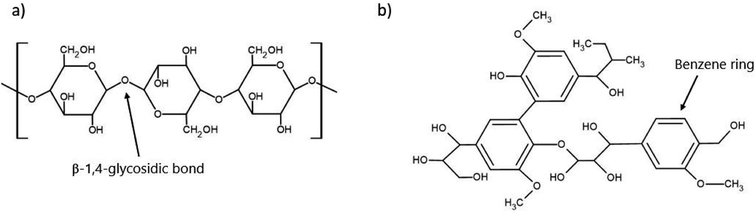 |
| Fig. 3 Chemical structure of two of the critical components of lignocellulosic biomass (a) cellulose and (b) lignin. | |
Table 3 Cellulose, hemicellulose, and lignin content of various lignocellulosic biomass types
Material |
Cellulose (%) |
Hemicellulose (%) |
Lignin (%) |
Ref |
Rice husk |
34.7 |
17.4 |
25.5 |
80
|
Apple |
47.49 |
27.77 |
24.72 |
81
|
Grape |
17.5 |
6.9 |
51.7 |
82
|
Coconut husk |
34 |
21 |
27 |
83
|
Barley hull |
34.0 |
36.0 |
16.0 |
84
|
Mango peel |
9.19 |
14.51 |
4.25 |
85
|
Corn stover |
37.6 |
21.5 |
19.1 |
86
|
Corn stalk |
34.5 |
27.6 |
21.8 |
87
|
Oak |
43.2 |
21.9 |
35.4 |
88
|
Banana peel |
13.2 |
14.8 |
14 |
89
|
Walnut shells |
23.3 |
20.4 |
53.5 |
90
|
Despite their abundance, lignocellulosic feedstocks provide a key challenge in their processing as they are naturally recalcitrant to microbial degradation, mainly due to the lignin barrier. In addition to the physical barrier, lignin (Fig. 3b) contains an abundance of aromatic rings, which can result in the formation of various phenolic compounds. These compounds can often prevent PHA-producing microbes from successfully metabolizing other compounds, effectively preventing their growth and productivity.91 To counteract this, pre-treatments such as alkaline, enzymatic, or physicochemical are used to reduce the biomass recalcitrance and enhance the subsequent sugar recovery.92 The purpose of these pre-treatments is to partially remove lignin and hemicellulose, reduce the crystallinity of the remaining cellulose, and improve the material's porosity so that it is more available for enzymatic hydrolysis by the microorganisms.91
3.1 Pre-treatment methods
In the process of PHA production from lignocellulosic biomass, the carbohydrates are converted to sugar monomers using enzymes, which are subsequently fermented to PHAs using bacteria. However, due to a complex matrix of cellulose, hemicellulose and lignin (Fig. 4), lignocellulosic biomass is highly recalcitrant and need to undergo a pre-treatment process to achieve high sugar yields during the enzymatic hydrolysis process. Several pre-treatment technologies have been developed to break the recalcitrance, and make cellulose accessible to enzymes to produce sugars.
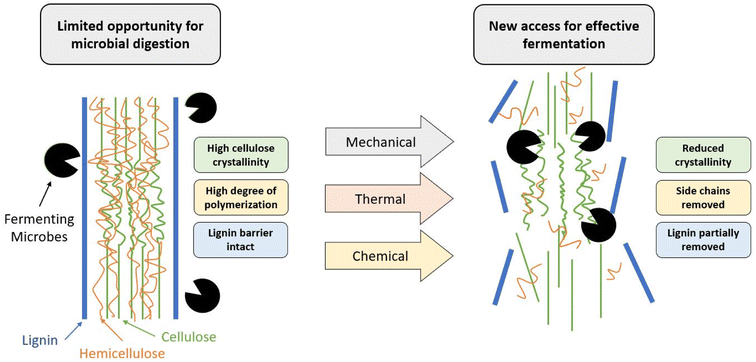 |
| Fig. 4 Depiction of the role that pre-treatment methods have in improving fermentation efficiency. | |
3.1.1 Mechanical.
Mechanical or physical pre-treatment involves size reduction of the biomass to alter the physical structure, increase porosity, decrease the degree of polymerization, and increase the specific surface area of biomass to facilitate enzyme action during hydrolysis.93 While it depends on the type and duration of milling along with the type of biomass, increased hydrolysis yields of 5–25% have been observed following a size reduction operation.94 There is a point of diminishing returns, however, as a study by Chang and Holtzapple suggests there is little benefit to hydrolysis yield in a size reduction beyond 425 microns.95
3.1.2 Hydrothermal pre-treatment.
In the hydrothermal pre-treatment approach, biomass is heated to a point at which the hemicellulose and lignin components begin to solubilize, typically 160–220 °C.96 This is generally done with either hot liquid water or pressurized steam. During this process, a portion of the hemicellulose is hydrolyzed which then form acids, which subsequently assisting in the hydrolysis of further hemicellulose.97 As the hemicellulose solubilizes, so does the lignin. The by-products which can be made by this process include phenolic compounds which are toxic to many species of bacteria and has an inhibitory effect on their production. A study by Laser et al. found that exceeding 220 °C at 3% solid concentration completely halted fermentation as a result of the formation of these toxic lignin compounds.98 Therefore, thermal pre-treatments must be conducted with care to prevent the formation of these inhibitory compounds.
3.1.3 Acid pre-treatment.
Acid pre-treatment is the most commonly used pre-treatment approach and has been found effective on a large number of feedstocks for enhancing hydrolysis efficiency. Although various acids, such as sulfuric acid, nitric acid, phosphoric acid, acetic acid, and hydrochloric acids, have been investigated, the dilute acid pre-treatment process is the most studied. During this process, lignocellulosic biomass is treated with dilute acid (0.05–5%) under high temperatures (160–220 °C) for a period of time (a few seconds to several hours). Acid treatments aim to solubilize most of the hemicellulose fraction of the biomass and breaking lignin–polysaccharide linkages, making the cellulose more available for microbial digestion. In an alternative approach, concentrated acids (strong acid pre-treatment) can be used at mild operating conditions. However, the use of strong acids suffers from the limitation of high maintenance cost of equipment due to corrosion. Also, in the presence of a strong acid, lignin will rapidly solubilize and subsequently precipitate, creating inhibitory compounds which prevent an effective fermentation.99 Another factor which must be considered is the effect of the pre-treatment chemical on the physical properties of the PHA produced. Kucera et al. observed a 6.2 °C higher crystallization temperature for PHB than usual in a pre-treated feedstock.100
3.1.4 Alkali pre-treatment.
Alkaline pre-treatments of biomass have two major benefits. First, the addition of an alkali compound results in the biomass swelling up, providing more surface area available for enzymatic attack. Secondly, alkaline hydrolysis can remove end groups from large polysaccharide chains at high enough alkali concentrations, creating low molecular weight compounds for easy fermentation.101 Like strong acid solutions, strong alkali solutions can result in the creation of solubilized lignin compounds, hindering the microbes' ability to conduct fermentation. In addition to this, it is possible for the biomass to absorb alkali salts, necessitating additional pre-treatments prior to fermentation and subsequently adding to the total processing costs.102
3.1.5 Oxidative pre-treatment.
Another pre-treatment strategy is the use of oxidative compounds such as hydrogen peroxide. This is done with the goal of removing the lignin and hemicellulose biomass fractions while improving the accessibility of the cellulose fraction to the microbes. As the various biomass components cannot be selectively targeted, this process oxidizes lignin, hemicellulose, and cellulose alike.103 This creates a high risk for the formation of inhibitory compounds, as the oxidized lignin results in the creation of soluble aromatic compounds.104 While effective at the removal of hemicellulose and lignin, oxidative reactions are not an effective treatment on their own as significant cellulose losses occur due to non-selective oxidation in addition to the formation of inhibitory compounds.104
3.1.6 Combination treatments.
It is extremely common for researchers to make use of a combination of pre-treatment methods to maximize the simple sugars created via hydrolysis while at the same time minimizing the creation of inhibitory compounds. Typically, these combination treatments will be a combination of a thermal treatment along with the addition of an acid, alkali, or enzymatic compound. The exact order in which this is conducted as well as the type and strength of the compound added is highly dependent on the exact biomass being treated.
3.2 Waste sources
Lignocellulosic waste is generated all over the world in vast quantities. The most common sources of these waste streams include farms and food processors, which generate both field waste as well as fruit residue. Examples of studies utilizing lignocellulosic waste can be found in Table 4.
Table 4 Examples of studies on PHA production using lignocellulosic biomass
Reactor configuration |
Microorganism |
Substrate |
Pre-treatment method |
PHA |
CDW (g L−1) |
PHA (g L−1) |
PHA yield (%) |
Ref. |
Batch |
P. chlororaphis DSM 19603 |
Apple pulp |
Thermal treatment |
mcl-PHAs |
8.74 |
4.30 |
49.25 |
112
|
E. coli LSBJ |
Brewer's spent grain |
Hydrothermal |
PHB |
7.49 |
2.39 |
32 |
113
|
P. cepacia ATCC 17759 |
Aspen wood hydrolysate |
Enzymatic treatment |
PHB |
2.59 |
1.55 |
60 |
114
|
R. eutropha ATCC 17697 |
Pineapple peel |
Acid hydrolysis |
PHB/PHV |
— |
∼1.8 |
— |
109
|
C. necator A-04 |
Pineapple core |
Acid hydrolysis |
PHB |
5.88 |
2.09 |
35.6 |
115
|
H. halophila CCM 3662 |
Saw dust (pine wood) |
Acid treatment |
PHB |
2.14 |
1.00 |
46.85 |
100
|
H. campisalis MCM B-1027 |
Banana peel |
Drying + milling, aqueous extract |
PHBV |
0.70 |
0.15 |
21.5 |
116
|
Orange peel |
0.78 |
0.33 |
42.5 |
Bagasse |
0.77 |
0.36 |
47.5 |
B. subtilis MTCC 144 |
Watermelon rind |
Drying + milling, aqueous extract |
PHB |
16.5 |
12.97 |
78.61 |
111
|
Papaya peel |
15.0 |
11.65 |
77.67 |
Orange peel |
19.39 |
9.68 |
49.93 |
B. cepecia (CCM 2656) |
Brewer's spent grain |
Thermal treatment |
PHB |
— |
1.13 |
— |
105
|
Bacillus MG12 |
Sugarcane waste |
Acid treatment |
PHBV |
2.89 |
0.24 |
8 |
106
|
B. cereus
|
Water hyacinth |
Mechanical treatment |
PHB |
0.55 |
9 |
16 |
117
|
H. halophila CCM 3662 |
Grape pomace sugar extract |
Enzymatic hydrolysis |
PHB |
3.1 |
1.8 |
57.0 |
110
|
H. organivorans CCM 7142 |
3.9 |
2.1 |
55.4 |
C. necator H16 CCM 3726 |
4.1 |
1.9 |
47.2 |
C. necator and P. citronellolis |
Apple pulp |
Autoclave |
PHB/mcl-PHA |
5.51 |
1.85 |
33.6 |
118
|
C. necator DSM 545 |
Corn stover liquor |
Alkaline treatment |
PHB |
— |
3.3 |
— |
119
|
Fed-batch |
C. necator DSM 545 |
Corn stover liquor |
Alkaline treatment |
PHB |
— |
4.5 |
— |
119
|
B. sacchari DSM17165 |
Wheat straw hydrolysate |
Enzymatic treatment |
PHB |
100–140 |
— |
72 |
15
|
H. mediterranei
|
Date waste |
None |
PHBV |
18.0 |
4.5 |
25 |
107
|
P. sacchari IPT 101 LMG 19450 |
Hardwood hydrolysate |
Enzymatic treatment |
PHB |
59.5 |
34.5 |
58 |
120
|
P. putida KT2440 |
Corn stover |
Acid + alkali + enzymatic treatment |
mcl-PHA |
5.68 |
1.00 |
17.6 |
121
|
3-Stage fed batch |
Mixed culture |
Unspecified fruit waste |
Acidogenic fermentation |
PHBV |
7.83 |
6.30 |
80.5 |
122
|
Mixed culture |
Apple pulp |
Acidogenic fermentation |
PHBV |
11 |
9.79 |
89 |
123
|
3.2.1 Crop and field waste.
Field waste is created from both cash crops and food crops, including matter such as stems, stalks, leaves, vines, and seeds left behind in a field following a harvest. Similarly, waste produced by a food processor will have a similar composition as these items are discarded prior to the food being prepared for human consumption. This can include both food crops and cash crops. While generally low in fermentable sugars initially, they can be pre-treated using a variety of methods to hydrolyze them into reducing sugars.
A recent study by Kucera et al. noted the effective use of acid hydrolysis on pine wood sawdust for the production of PHB.100 The resulting hydrolysates were comprised of mainly arabinose, xylose, and glucose, which were effectively metabolized by H. halophila. While creating a lower yield of PHB compared to a sugar-rich substrate such as molasses (1.00 g PHB/L and 2.57 g PHB/L, respectively), saw dust is an extremely cheap material which can be found abundantly in many industries nearly anywhere in the world.
However, these pre-treatment methods can come with an assortment of downfalls. Depending on the substrate used, various inhibitory compounds can be created as a result. This was demonstrated by Corchado-Lopo et al., where a wide assortment of pre-treatments were tested (acid, acid-thermal, acid-thermal + various washes) on brewers' spent grain (BSG).105 While these treatments were able to increase the availability of sugars, they resulted in the creation of an assortment of phenolic inhibitory compounds from the solubilization of lignin such as vanillin, coumaric acid, and syringic acid. The highest PHB productivity (1.13 g L−1) therefore came from the non-treated BSG by the bacteria B. cepacia. Similar results were observed by Thomas et al. (2021) where the PHB productivity from raw BSG hydrolysate (3.53 g L−1) was found to be higher compared to hydrothermal pre-treated BSG hydrolysate (2.39 g L−1) using E. coli LSBJ as the fermentative microbe.113
For the efficient production of PHBV, genetic modification is often conducted on the microbes to control the relevant metabolic pathways, adding complexity and cost to the operation. Moorkoth and Nampoothiri were able to produce an extremely high HV content (42%) PHBV co-polymer through the fermentation of sugarcane waste with the wild culture Bacillus MG12.106 Despite the low yield of PHBV (0.236 g L−1) due to the inhibitory compounds such as furfural and formic acid found in the sugarcane waste, the ability to produce high HV content PHBV is noteworthy. The HV content can then be controlled via altering the concentration of propionic acid within the fermentation medium.
Cesário et al. used wheat straw hydrolysates for the production of PHB using B. sacchari in a fed-batch configuration, reaching up to 105 g PHB/L, overcoming carbon catabolite repression (CCR).15 The CCR is in essence when a single carbon source is extremely dominant that the microbes no longer consume the other sugars present. By providing a rich blend of other C5 sugars, the wheat hydrolysate is able to avoid this phenomena.
3.2.2 Fruit residue.
Fruit residue takes many shapes and forms, including seeds, pulp, peels, and shells as a result of food processing. Compared to other types of agricultural waste, fruit waste has the benefit of more readily accessible sugars for microbes to immediately ferment. This lessens the need for extreme pre-treatment methods, such as in a study by Alsafadi et al.107 Date fruit waste was used with no hydrolysis pre-treatment used prior to fermentation, and was able to yield 4.5 g PHBV/L.107 No trace element supplement solution was provided in this trial as it was found that the date waste provided enough for the H. mediterranei, which can help further reduce the cost of production.
A variety of other fruit wastes are popular for PHA synthesis, namely apple pomace, pineapple residues, and grape pomace. This is highly geographically dependent and varies based on local production volumes. Common forms of waste from these fruits include peels and pomace as a result of pressing processes.108 The microbial species R. eutropha ATCC 17697 has been demonstrablly capable of producing PHV from pineapple peel residue hydrolysate, with over 60% of PHAs produced being of the PHV variety.109 Similarly, grape pomace has shown potential which could prove extremely beneficial for wine producing regions. Kovalcik et al. utilized grape pomace (comprised of skins, seeds, and stalks) and extracted the glucose via enzymatic hydrolysis.110 Using three species of bacteria H. halophila, H. organivorans, and C. necator they were able to average 1.9 g PHB/L, compared to an average of 4.4 g PHB/L when using pure glucose.110
Other promising substrates include an extract from various fruit peels such as watermelon, papaya, and orange. Rao et al. was able to achieve a PHA% yield of 79, 78, and 50 from each of these substrates, respectively.111 In this study, the authors noted that a compound commonly found within the peels of citrus fruits, ligand CID ID 10621, binds with the protein citrate synthase, effectively blocking the tricarboxylic acid (TCA) cycle, a metabolic pathway. This results in a greater abundance of acetyl-CoA, a precursor required for PHA synthesis. This demonstrates the need for consideration to be given to the various compounds and by-products created by these substrates, as they can have either a beneficial or hindering effect on PHA production.
4 Critical review and opportunities
The vast quantity of lignocellulosic biomass available worldwide makes it a promising substrate for low-cost PHA production, however, several challenges remain to address before the process could be a commercial success. Similar to the case of lignocellulosic biofuel biorefineries, biomass heterogeneity and compositional variation could be a challenge. As a naturally grown material, the quality and composition of the biomass can dramatically change throughout different stages of ripeness, seasons, or processing conditions. This inconsistency can create challenges for PHAs producers who have historically desired a 100% pure substrate to improve the consistency of the PHA produced. Methods to maintain the year-round viability of agricultural waste as a substrate for PHA synthesis will need to be determined to encourage investment in such a process.
As discussed in the previous sections, pre-treatment is a critical step in the lignocellulosic biorefineries to achieve high sugar yields during enzymatic hydrolysis. The high cost of reactors and energy required during biomass pre-treatment could contribute significantly to overall process sustainability. Although no study has compared the economics of various biomass pre-treatment technologies during lignocellulosic biomass to PHA production, based on lignocellulosic ethanol refineries studies it can be predicted that the choice of pre-treatment technology should play a critical role in the economic feasibility of the process. The choice of pretreatment is also critical from the process efficiency point of view as the high-severity pretreatments could lead to higher sugar yields during the enzymatic hydrolysis, however, might result in lower fermentation yields due to the formation of inhibitory compounds. Extensive work will need to be conducted to determine the inhibitory compounds produced during pre-treatment processes on the specific substrate being used. This should coincide with future genetic modification work, where microbes can be tailored to either be unaffected by these compounds or find a way to productively utilize them instead. This will turn one of the greatest drawbacks of lignocellulosic biomass pre-treatment into a benefit and allow for greater scalability to the industrial level.
Another major area of research focus is the downstream recovery of the PHAs from fermentation broth. The PHA yield from a waste substrate is oftentimes lower than that from a pure source. This makes the subsequent extraction process more difficult and resource intensive. This challenge could be addressed by either increasing the fermentation titers or using advanced separation technologies. Most of the microbial strains have lower PHA yields from the fermentation of C5 sugars (from hemicellulose) compared to glucose fermentation. The development of genetically engineered strains that can efficiently ferment both C5 and C6 sugars could improve the PHB titers which would reduce the downstream recovery cost and energy, and ultimately reduce the overall PHA production cost. Furthermore, there is a need for the further development of green methods for both pre-treatment of biomass and extraction of PHAs. The biodegradable, environmentally friendly nature of PHAs is quickly undone if the means of their production require a vast array of harsh chemicals.
Other areas in which the cost of production can be reduced is through the use of open, mixed cultures, as fewer resources will be needed to guarantee a sterile environment. This will have the dual benefit of lowering the cost while also making production more accessible.
5 Commercial-scale economic feasibility
As the lignocellulosic biomass-based PHA production processes are at an early stage of development and mainly investigated at the lab scale only, techno-economic analysis (TEA) using process simulation models can provide an insight into the commercial-scale technical and economic feasibility of the process and help in identifying the hotspots (cost and/or energy-intensive unit operations).
In the conventional PHB production from pure sugars, the cost of feedstock (pure sugars) accounts for a major fraction. Manikandan et al. (2021) conducted a TEA of PHB production from carob pods using a closed-loop biorefinery approach.124 In the PHA production process, biomass was treated with methanol to recover lignin and the treated biomass was further processed to extract glucose and fructose, which were subsequently fermented using Ralstonia eutropha. A process model was developed in Aspen Plus to simulate 5000 m3-scale bioreactor with 96 batches per year. Due to the high cost of pure sugars, the feedstock cost accounted for 67% of the operational costs, whereas, in the case of carob pods biorefinery, the feedstock cost accounted only for 14–16%. Due to these lower feedstock costs, the use of biomass hydrolysate as a replacement for pure sugars for PHA production reduced the pay-out period from 12.6 to 6.8 years. The capital investment of the refinery was estimated $418 million. Rajendran and Han (2022) conducted a TEA of co-production of PHA and biofuel (ethanol, biohydrogen, and 2,3-butanediol) from food waste at a processing scale of 50 MT per day. The process was found profitable, with a capital investment of $42.8 million and a minimum selling price of PHA to be $4.83 per kg.125 From the process simulations under various operating conditions, it was observed that the minimum selling price of PHA could further be reduced to $2.41 per kg by performing hydrolysis at high-solids (30%).
Biomass dewatering (separation of cells from fermentation broth) during the PHB recovery process is always considered an energy and cost-intensive step. Manikandan et al. (2021) compared the economics of conventional centrifugation and novel ceramic membranes for biomass dewatering.124 The capital cost of the ceramic membranes was only $0.15 million compared to $26 million for the centrifuge, and the power requirement was also found to be 75% lower, resulting in cost-efficient PHB production. Pavan et al. (2019) performed a comprehensive techno-economic analysis for a PHB production facility (2000 tonnes per year) from citric molasses using C. necator DSM 545.126 Among various process options (mainly differed in the downstream recovery of PHA), the minimum capital investment required was an estimated $33.12 million. Correspondingly, the PHB production was estimated to be $4.28 per kg. It was observed that even with the similar PHB extraction efficiencies among various downstream recovery options, the PHB production cost was different due to the difference in the associated equipment purchase cost and utilities used. The study also reported that the production cost could be further reduced by increasing the production scale. At 10
000 tonnes per year production scale, the PHB production cost was found $2.37 per kg.
As fermentation is the heart of the overall PHB production process, the selection of a bioreactor/fermenter can affect the process economics. Manikandan et al. (2021) compared the economics for two types of bioreactors for fermentation: stirred tank bioreactor (STBR) and annular bioreactor (ABR). Although the capital cost ($49.69 vs. $44.96 million) and operating cost ($458.33 vs. $229.17 million) was relatively higher compared to the STBR, the fermentation titers were also significantly higher (70.8 vs. 44.2 g L−1) that led to high yields of PHB production (30
267 vs. 18
895 tonnes per year) and lower downstream recovery costs.
Although, TEA studies on lignocellulosic biomass-based bioplastics are scarce at this point, process models and TEA could play a significant role in understanding the commercial feasibility and guiding the research to improve process sustainability. From the studies discussed above, it can be concluded that the downstream recovery process, type of bioreactor, and production scale are critical components in deciding the economic viability of PHA production from lignocellulosic biomass.
6 Conclusions
This manuscript has described the means by which PHAs are synthesized in both commercial and laboratory environments and the various synthesis pathways used by microbes for the creation of PHAs. Common pre-treatment strategies for lignocellulosic biomass along with their benefits and drawbacks were discussed, with a review of the current literature regarding the use of this biomass for PHA synthesis. Based on this study, several areas of focus for future works have been put forward.
Despite challenges, the efficient creation of PHAs from lignocellulosic biomass waste streams shows significant potential to help dramatically reduce the cost of PHA production. This sustainable production strategy for biodegradable polymers will help to reduce both the cost and environmental impact of polymer products, such as the forecasted 15% of global greenhouse gas emissions being attributed to polymers by 2050. It is the opinion of the authors that investment into discovering additional cost-effective methods of PHA production is a worthy endeavor to tackle the ever-growing issue of plastic pollution. The use of low-cost lignocellulosic waste will help progress towards the UN sustainability goal of responsible consumption and production (SDG 12) by encouraging the development of a circular economy by providing a greater value to the producers of these wastes such as farmers and food processors.
Author contributions
P. Z.: methodology, investigation, data curation, formal analysis, visualization, writing – original draft. D. K.: investigation, validation, writing – review & editing. A. E.: validation, writing – review & editing. A. K. M.: conceptualization, investigation, methodology, validation, supervision, resources, writing – review & editing. B. V. R.: writing – review & editing. M. M.: conceptualization, investigation, methodology, validation, supervision, resources, supervision, funding acquisition and administration, writing – review & editing. All authors contributed to discussion, reviews, edit and approval of the manuscript for publication.
Conflicts of interest
The authors declare no conflict of interests.
Acknowledgements
The authors would like to acknowledge the financial support of (i) the Ontario Research Fund, Research Excellence Program; Round 9 (ORF-RE09) Ontario Ministry of Economic Development, Job Creation and Trade, Canada (project no. 053970 and 054345); (ii) the Ontario Ministry of Agriculture, Food and Rural Affairs (OMAFRA), Canada/University of Guelph – HQP program; (iii) OMAFRA – Bioeconomy for Industrial Uses Research Program (project no. 030486, 030671 and 030578); (iv) the Agriculture and Agri-Food Canada (AAFC), Maple Leaf Food, Canada and Bank of Montreal (BMO), Canada through Bioindustrial Innovation Canada (BIC) Bioproducts AgSci Cluster Program (project no. 054015, 054449 and 800148); and (v) the Natural Sciences and Engineering Research Council of Canada (NSERC), Canada Research Chair (CRC) program project no. 460788 to carry out this study.
References
- Y. Chen, A. K. Awasthi, F. Wei, Q. Tan and J. Li, Sci. Total Environ., 2021, 752, 141772 CrossRef CAS PubMed.
- R. Geyer, J. R. Jambeck and K. L. Law, Sci. Adv., 2017, 3, 3–8 Search PubMed.
- C. Symeonides, M. Brunner, Y. Mulders, P. Toshniwal, M. Cantrell, L. Mofflin and S. Dunlop, J. Paediatr. Child Health, 2021, 57, 1795–1804 CrossRef PubMed.
- S. Sharma, V. Sharma and S. Chatterjee, Sci. Total Environ., 2023, 875, 162627 CrossRef CAS PubMed.
- J. M. Garcia and M. L. Robertson, Science, 2017, 358, 870–872 CrossRef CAS PubMed.
- M. Mierzwa-Hersztek, K. Gondek and M. Kopeć, J. Polym. Environ., 2019, 27, 600–611 CrossRef CAS.
- J. Zheng and S. Suh, Nat. Clim. Change, 2019, 9, 374–378 CrossRef.
- O. I. Ungureanu, D. Bulgariu, A. M. Mocanu and L. Bulgariu, Water, 2020, 12, 1–12 CrossRef.
- D. Lithner, Å. Larsson and G. Dave, Sci. Total Environ., 2011, 409, 3309–3324 CrossRef CAS PubMed.
- A. Ragusa, A. Svelato, C. Santacroce, P. Catalano, V. Notarstefano, O. Carnevali, F. Papa, M. C. A. Rongioletti, F. Baiocco, S. Draghi, E. D'Amore, D. Rinaldo, M. Matta and E. Giorgini, Environ. Int., 2021, 146, 106274 CrossRef CAS PubMed.
- A. Ragusa, V. Notarstefano, A. Svelato, A. Belloni, G. Gioacchini, C. Blondeel, E. Zucchelli, C. De Luca, S. D’avino, A. Gulotta, O. Carnevali and E. Giorgini, Polymers, 2022, 14, 1–14 CrossRef PubMed.
- B. Imre, L. García, D. Puglia and F. Vilaplana, Carbohydr. Polym., 2019, 209, 20–37 CrossRef CAS PubMed.
- A. K. Mohanty, F. Wu, R. Mincheva, M. Hakkarainen, J. M. Raquez, D. F. Mielewski, R. Narayan, A. N. Netravali and M. Misra, Nat. Rev. Methods Primers, 2022, 2, 1–27 CrossRef.
- D. Tan, Y. Wang, Y. Tong and G. Q. Chen, Trends Biotechnol., 2021, 39, 953–963 CrossRef CAS PubMed.
- M. T. Cesário, R. S. Raposo, M. C. M. D. de Almeida, F. van Keulen, B. S. Ferreira and M. M. R. da Fonseca, New Biotechnol., 2014, 31, 104–113 CrossRef PubMed.
- P. Zytner, F. Wu, M. Misra and A. K. Mohanty, ACS Omega, 2020, 5, 14900–14910 CrossRef CAS PubMed.
- P. Zytner, A. K. Pal, F. Wu, A. Rodriguez-uribe, A. K. Mohanty and M. Misra, ACS Omega, 2022, 8, 1946–1956 CrossRef PubMed.
- A. K. Pal, F. Wu, M. Misra and A. K. Mohanty, Composites, Part B, 2020, 198, 108141 CrossRef CAS.
- P. Feijoo, A. K. Mohanty, A. Rodriguez-Uribe, J. Gámez-Pérez, L. Cabedo and M. Misra, Int. J. Biol. Macromol., 2023, 225, 1291–1305 CrossRef CAS PubMed.
- S. Qin, B. Shekher Giri, A. Kumar Patel, T. Sar, H. Liu, H. Chen, A. Juneja, D. Kumar, Z. Zhang, M. Kumar Awasthi and M. J. Taherzadeh, Bioresour. Technol., 2021, 321, 124496 CrossRef CAS PubMed.
- J. G. Linger, D. R. Vardon, M. T. Guarnieri, E. M. Karp, G. B. Hunsinger, M. A. Franden, C. W. Johnson, G. Chupka, T. J. Strathmann, P. T. Pienkos and G. T. Beckham, Proc. Natl. Acad. Sci. U. S. A., 2014, 111, 12013–12018 CrossRef CAS PubMed.
- R. Santagata, M. Ripa, A. Genovese and S. Ulgiati, J. Cleaner Prod., 2021, 286, 125490 CrossRef.
-
M. Blakeney, Food Loss and Food Waste: Causes and Solutions, Rome, 2011 Search PubMed.
- V. Dornburg, I. Lewandowski and M. Patel, J. Ind. Ecol., 2003, 7, 93–116 CrossRef CAS.
- K. W. Meereboer, M. Misra and A. K. Mohanty, Green Chem., 2020, 22, 5519–5558 RSC.
- A. Steinbüchel and H. E. Valentin, FEMS Microbiol. Lett., 1995, 128, 219–228 CrossRef.
- R. H. Findlay and D. C. White, Appl. Environ. Microbiol., 1983, 45, 71–78 CrossRef CAS PubMed.
- Z. Sun, J. A. Ramsay, M. Guay and B. A. Ramsay, Appl. Microbiol. Biotechnol., 2007, 75, 475–485 CrossRef CAS PubMed.
- K. Budwill, P. M. Fedorak and W. J. Page, Appl. Environ. Microbiol., 1992, 58, 1398–1401 CrossRef CAS PubMed.
- F. Ben Rebah, D. Prévost, R. D. Tyagi and L. Belbahri, Appl. Biochem. Biotechnol., 2009, 158, 155–163 CrossRef CAS PubMed.
- S. Chanprateep, K. Buasri, A. Muangwong and P. Utiswannakul, Polym. Degrad. Stab., 2010, 95, 2003–2012 CrossRef CAS.
- S. Muhammadi, M. Afzal and S. Hameed, Green Chem. Lett. Rev., 2015, 8, 56–77 CrossRef.
- S. Y. Lee, H. H. Wong, J. Il Choi, S. H. Lee, S. C. Lee and C. S. Han, Biotechnol. Bioeng., 2000, 68, 466–470 CrossRef CAS PubMed.
- L. L. Madison and G. W. Huisman, Microbiol. Mol. Biol. Rev., 1999, 63, 21–53 CrossRef CAS PubMed.
- S. Shanmugam, A. Hari, D. Kumar, K. Rajendran, T. Mathimani, A. E. Atabani, K. Brindhadevi and A. Pugazhendhi, Fuel, 2021, 285, 119052 CrossRef CAS.
- W. Hohenschuh, D. Kumar and G. S. Murthy, J. Renewable Sustainable Energy, 2014, 6, 63113 CrossRef.
- W. S. Ahn, S. J. Park and S. Y. Lee, Biotechnol. Lett., 2005, 23, 119–122 Search PubMed.
- S. K. Hahn, Y. K. Chang and S. Y. Lee, Appl. Environ. Microbiol., 1995, 61, 34–39 CrossRef CAS PubMed.
- S. Fidler and D. Dennis, FEMS Microbiol. Lett., 1992, 103, 231–235 CrossRef CAS.
- G. Q. Chen and X. R. Jiang, Synth. Syst. Biotechnol., 2017, 2, 192–197 CrossRef PubMed.
- S. Mohapatra, P. R. Mohanta, B. Sarkar, A. Daware, C. Kumar and D. P. Samantaray, Proc. Natl. Acad. Sci., India, Sect. B, 2017, 87, 459–466 CrossRef CAS.
- C. Haas, T. El-Najjar, N. Virgolini, M. Smerilli and M. Neureiter, New Biotechnol., 2017, 37, 117–122 CrossRef CAS PubMed.
- A. Stanley, H. N. Punil Kumar, S. Mutturi and S. V. N. Vijayendra, Appl. Biochem. Biotechnol., 2018, 184, 935–952 CrossRef CAS PubMed.
- A. H. Mohamad Fauzi, A. S. M. Chua, L. W. Yoon, T. Nittami and H. K. Yeoh, Process Saf. Environ. Prot., 2019, 122, 200–208 CrossRef CAS.
- M. H. A. Ibrahim and A. Steinbüchel, Appl. Environ. Microbiol., 2009, 75, 6222–6231 CrossRef CAS PubMed.
- S. Y. Choi, I. J. Cho, Y. Lee, Y. J. Kim, K. J. Kim and S. Y. Lee, Adv. Mater., 2020, 32, 1–37 Search PubMed.
- S. Y. Lee, Y. K. Lee and H. N. Chang, J. Ferment. Bioeng., 1995, 79, 177–180 CrossRef CAS.
- G. W. Haywood, A. J. Anderson and E. A. Dawes, FEMS Microbiol. Lett., 1989, 60, 245 CrossRef.
- D. C. Meng, R. Shen, H. Yao, J. C. Chen, Q. Wu and G. Q. Chen, Curr. Opin. Biotechnol., 2014, 29, 24–33 CrossRef CAS PubMed.
- Q. Liu, G. Luo, X. R. Zhou and G. Q. Chen, Metab. Eng., 2011, 13, 11–17 CrossRef CAS PubMed.
- S. Slater, T. Gallaher and D. Dennis, Appl. Environ. Microbiol., 1992, 58, 1089–1094 CrossRef CAS PubMed.
- D. Byrom, Trends Biotechnol., 1987, 5, 246–250 CrossRef CAS.
- M. Li, X. Chen, X. Che, H. Zhang, L. P. Wu, H. Du and G. Q. Chen, Metab. Eng., 2019, 52, 253–262 CrossRef CAS PubMed.
- J. Wang, K. Chon, X. Ren, Y. Kou, K. J. Chae and Y. Piao, Bioresour. Technol., 2019, 281, 90–98 CrossRef CAS PubMed.
- W. Tu, D. Zhang and H. Wang, Waste Manage., 2019, 96, 149–157 CrossRef CAS PubMed.
- D. J. Portugal-Nunes, S. S. Pawar, G. Lidén and M. F. Gorwa-Grauslund, AMB Express, 2017, 7, 1–12 CrossRef CAS PubMed.
- E. Grousseau, E. Blanchet, S. Déléris, M. G. E. Albuquerque, E. Paul and J. L. Uribelarrea, Bioresour. Technol., 2014, 153, 206–215 CrossRef CAS PubMed.
- M. Zinn, B. Witholt and T. Egli, Adv. Drug Delivery Rev., 2001, 53, 5–21 CrossRef CAS PubMed.
- A. C. Blaga, C. Ciobanu, D. Caşcaval and A. I. Galaction, Biochem. Eng. J., 2018, 131, 70–76 CrossRef CAS.
- A. Atlić, M. Koller, D. Scherzer, C. Kutschera, E. Grillo-Fernandes, P. Horvat, E. Chiellini and G. Braunegg, Appl. Microbiol. Biotechnol., 2011, 91, 295–304 CrossRef PubMed.
- K. Dietrich, M. J. Dumont, L. F. Del Rio and V. Orsat, Sustain. Prod. Consum., 2017, 9, 58–70 CrossRef.
- M. Koller, H. Niebelschütz and G. Braunegg, Eng. Life Sci., 2013, 13, 549–562 CrossRef CAS.
- N. S. Kurian and B. Das, Int. J. Biol. Macromol., 2021, 183, 1881–1890 CrossRef CAS PubMed.
- C. Pérez-Rivero, J. P. López-Gómez and I. Roy, Biochem. Eng. J., 2019, 150, 107283 CrossRef.
- F. Chemat, M. A. Vian and G. Cravotto, Int. J. Mol. Sci., 2012, 13, 8615–8627 CrossRef CAS PubMed.
- N. Yabueng and S. C. Napathorn, Process Biochem., 2018, 69, 197–207 CrossRef CAS.
- F. M. Kapritchkoff, A. P. Viotti, R. C. P. Alli, M. Zuccolo, J. G. C. Pradella, A. E. Maiorano, E. A. Miranda and A. Bonomi, J. Biotechnol., 2006, 122, 453–462 CrossRef CAS PubMed.
- V. Kachrimanidou, N. Kopsahelis, A. Vlysidis, S. Papanikolaou, I. K. Kookos, B. Monje Martínez, M. C. Escrig Rondán and A. A. Koutinas, Food Bioprod. Process., 2016, 100, 323–334 CrossRef CAS.
- P. Murugan, L. Han, C. Y. Gan, F. H. J. Maurer and K. Sudesh, J. Biotechnol., 2016, 239, 98–105 CrossRef CAS PubMed.
- B. Kunasundari, C. R. Arza, F. H. J. Maurer, V. Murugaiyah, G. Kaur and K. Sudesh, Sep. Purif. Technol., 2017, 172, 1–6 CrossRef CAS.
-
A. Hodzic, Natural Fibers, Biopolymers, and Biocomposites, CRC Press, Boca Raton, FL, 2005 Search PubMed.
- M. R. Kosseva and E. Rusbandi, Int. J. Biol. Macromol., 2018, 107, 762–778 CrossRef CAS PubMed.
- B. Kim, Enzyme Microb. Technol., 2000, 27, 774–777 CrossRef CAS PubMed.
- W. H. Lee, C. Y. Loo, C. T. Nomura and K. Sudesh, Bioresour. Technol., 2008, 99, 6844–6851 CrossRef CAS PubMed.
- S. Povolo, P. Toffano, M. Basaglia and S. Casella, Bioresour. Technol., 2010, 101, 7902–7907 CrossRef CAS PubMed.
- Q. Wen, S. Liu, Y. Liu and Z. Chen, Int. J. Biol. Macromol., 2021, 182, 1785–1792 CrossRef CAS PubMed.
- J. Sharma, V. Kumar, R. Prasad and N. A. Gaur, Biotechnol. Adv., 2022, 56, 107925 CrossRef CAS PubMed.
- S. D. Stefanidis, K. G. Kalogiannis, E. F. Iliopoulou, C. M. Michailof, P. A. Pilavachi and A. A. Lappas, J. Anal. Appl. Pyrolysis, 2014, 105, 143–150 CrossRef CAS.
- P. McKendry, Bioresour. Technol., 2002, 83, 37–46 CrossRef CAS PubMed.
- K. S. Heng, R. Hatti-Kaul, F. Adam, T. Fukui and K. Sudesh, J. Chem. Technol. Biotechnol., 2017, 92, 100–108 CrossRef CAS.
- M. R. B. Guerrero, M. Marques Da Silva Paula, M. M. Zaragoza, J. S. Gutiérrez, V. G. Velderrain, A. L. Ortiz and V. Collins-Martínez, Int. J. Hydrogen Energy, 2014, 39, 16619–16627 CrossRef CAS.
- M. Gómez-Brandón, C. Lazcano, M. Lores and J. Domínguez, J. Hazard. Mater., 2011, 187, 291–295 CrossRef PubMed.
- A. K. Bledzki, A. A. Mamun and J. Volk, Compos. Sci. Technol., 2010, 70, 840–846 CrossRef CAS.
- R. K. Prasad, S. Chatterjee, P. B. Mazumder, S. K. Gupta, S. Sharma, M. G. Vairale, S. Datta, S. K. Dwivedi and D. K. Gupta, Chemosphere, 2019, 231, 588–606 CrossRef CAS PubMed.
- R. S. Orozco, P. B. Hernández, G. R. Morales, F. U. Núñez, J. O. Villafuerte, V. L. Lugo, N. F. Ramírez, C. E. B. Díaz and P. C. Vázquez, BioResources, 2014, 9, 1873–1885 Search PubMed.
- N. Q. Ren, G. L. Cao, W. Q. Guo, A. J. Wang, Y. H. Zhu, B. feng Liu and J. F. Xu, Int. J. Hydrogen Energy, 2010, 35, 2708–2712 CrossRef CAS.
- Y. Wang, H. Wang, X. Feng, X. Wang and J. Huang, Int. J. Hydrogen Energy, 2010, 35, 3092–3099 CrossRef CAS.
- B. Kumar, N. Bhardwaj, K. Agrawal, V. Chaturvedi and P. Verma, Fuel Process. Technol., 2020, 199, 106244 CrossRef CAS.
- A. K. Kumar and S. Sharma, Bioresour. Bioprocess., 2017, 4, 1–19 CrossRef PubMed.
- B. de Caprariis, P. De Filippis, A. Petrullo and M. Scarsella, Fuel, 2017, 208, 618–625 CrossRef CAS.
- S. Obruca, P. Benesova, L. Marsalek and I. Marova, Chem. Biochem. Eng. Q., 2015, 29, 135–144 CrossRef CAS.
- M. Li and M. R. Wilkins, Int. J. Biol. Macromol., 2020, 156, 691–703 CrossRef CAS PubMed.
- D. Kumar, A. Juneja and V. Singh, Fuel Process. Technol., 2018, 173, 66–74 CrossRef CAS.
- H. Hartmann, I. Angelidaki and B. K. Ahring, Water Sci. Technol., 2000, 41, 145–153 CrossRef CAS PubMed.
- V. S. Chang and M. T. Holtzapple, Appl. Biochem. Biotechnol., Part A, 2000, 84–86, 5–37 CrossRef CAS PubMed.
- J. Wang, J. Liu, J. Li and J. Y. Zhu, Materials, 2021, 14, 1–12 Search PubMed.
- D. Gregg and J. N. Saddler, Appl. Biochem. Biotechnol., 1996, 57, 711–727 CrossRef.
- M. Laser, D. Schulman, S. G. Allen, J. Lichwa, M. J. Antal and L. R. Lynd, Bioresour. Technol., 2002, 81, 33–44 CrossRef CAS PubMed.
- C. Liu and C. E. Wyman, Ind. Eng. Chem. Res., 2004, 43, 2781–2788 CrossRef CAS.
- D. Kucera, I. Pernicová, A. Kovalcik, M. Koller, L. Mullerova, P. Sedlacek, F. Mravec, J. Nebesarova, M. Kalina, I. Marova, V. Krzyzanek and S. Obruca, Bioresour. Technol., 2018, 256, 552–556 CrossRef CAS PubMed.
- Y. L. Loow, T. Y. Wu, J. M. Jahim, A. W. Mohammad and W. H. Teoh, Cellulose, 2016, 23, 1491–1520 CrossRef CAS.
- Y. Zheng, Z. Pan and R. Zhang, Int. J. Agric. Biol. Eng., 2009, 2, 51–68 CAS.
- P. Kaparaju and C. Felby, Bioresour. Technol., 2010, 101, 3175–3181 CrossRef CAS PubMed.
- A. T. W. M. Hendriks and G. Zeeman, Bioresour. Technol., 2009, 100, 10–18 CrossRef CAS PubMed.
- C. Corchado-Lopo, O. Martínez-Avila, E. Marti, J. Llimós, A. M. Busquets, D. Kucera, S. Obruca, L. Llenas and S. Ponsá, New Biotechnol., 2021, 62, 60–67 CrossRef CAS PubMed.
- D. Moorkoth and K. M. Nampoothiri, Bioresour. Technol., 2016, 201, 253–260 CrossRef CAS PubMed.
- D. Alsafadi, M. I. Ibrahim, K. A. Alamry, M. A. Hussein and A. Mansour, Sci. Total Environ., 2020, 737, 139716 CrossRef CAS PubMed.
- M. K. Awasthi, J. A. Ferreira, R. Sirohi, S. Sarsaiya, B. Khoshnevisan, S. Baladi, R. Sindhu, P. Binod, A. Pandey, A. Juneja, D. Kumar, Z. Zhang and M. J. Taherzadeh, Renewable Sustainable Energy Rev., 2021, 143, 110972 CrossRef CAS.
- O. Vega-Castro, J. Contreras-Calderon, E. León, A. Segura, M. Arias, L. Pérez and P. J. A. Sobral, J. Biotechnol., 2016, 231, 232–238 CrossRef CAS PubMed.
- A. Kovalcik, I. Pernicova, S. Obruca, M. Szotkowski, V. Enev, M. Kalina and I. Marova, Food Bioprod. Process., 2020, 124, 1–10 CrossRef CAS.
- A. Rao, S. Haque, H. A. El-Enshasy, V. Singh and B. N. Mishra, Biomolecules, 2019, 9, 1–16 CrossRef PubMed.
- J. R. Pereira, D. Araújo, P. Freitas, A. C. Marques, V. D. Alves, C. Sevrin, C. Grandfils, E. Fortunato, M. A. M. Reis and F. Freitas, Int. J. Biol. Macromol., 2021, 167, 85–92 CrossRef CAS PubMed.
- C. M. Thomas, R. A. Scheel, C. T. Nomura, B. Ramarao and D. Kumar, Biomass Convers. Biorefin., 2021 DOI:10.1007/s13399-021-01738-w.
- B. Yang, Z. Dai, S. Y. Ding and C. E. Wyman, Biofuels, 2011, 2, 421–449 CrossRef CAS.
- V. Sukruansuwan and S. C. Napathorn, Biotechnol. Biofuels, 2018, 11, 1–15 CrossRef PubMed.
- S. O. Kulkarni, P. P. Kanekar, J. P. Jog, S. S. Sarnaik and S. S. Nilegaonkar, Int. J. Biol. Macromol., 2015, 72, 784–789 CrossRef CAS PubMed.
- R. Mehrabi, G. Bagheri and F. Alipour, J. Entomol. Zool. Stud., 2015, 3, 33–37 Search PubMed.
- A. T. Rebocho, J. R. Pereira, L. A. Neves, V. D. Alves, C. Sevrin, C. Grandfils, F. Freitas and M. A. M. Reis, Bioengineering, 2020, 7, 1–18 CrossRef PubMed.
- M. Li and M. Wilkins, Bioresour. Technol., 2020, 299, 122676 CrossRef CAS PubMed.
- K. Dietrich, E. R. Oliveira-Filho, M. J. Dumont, J. G. C. Gomez, M. K. Taciro, L. F. da Silva, V. Orsat and L. F. D. Rio, Ind. Crops Prod., 2020, 154, 112703 CrossRef CAS.
- Z. H. Liu, M. L. Olson, S. Shinde, X. Wang, N. Hao, C. G. Yoo, S. Bhagia, J. R. Dunlap, Y. Pu, K. C. Kao, A. J. Ragauskas, M. Jin and J. S. Yuan, Green Chem., 2017, 19, 4939–4955 RSC.
- M. Matos, R. A. P. Cruz, P. Cardoso, F. Silva, E. B. Freitas, G. Carvalho and M. A. M. Reis, ACS Sustain. Chem. Eng., 2021, 9, 8270–8279 CrossRef CAS.
- B. V. Sousa, F. Silva, M. A. M. Reis and N. D. Lourenço, Biochem. Eng. J., 2021, 176, 108210 CrossRef CAS.
- N. A. Manikandan, K. Pakshirajan and G. Pugazhenthi, Chemosphere, 2021, 284, 131371–131382 CrossRef CAS PubMed.
- N. Rajendran and J. Han, Bioresour. Technol., 2022, 348, 126796 CrossRef CAS.
- F. A. Pavan, T. L. Junqueira, M. D. B. Watanabe, A. Bonomi, L. K. Quines, W. Schmidell and G. M. F. de Aragao, Biochem. Eng. J., 2019, 146, 97–104 CrossRef CAS.
|
This journal is © The Royal Society of Chemistry 2023 |