Next generation decentralized water systems: a water-energy-infrastructure-human nexus (WEIHN) approach
Received
10th July 2023
, Accepted 28th August 2023
First published on 28th August 2023
Abstract
The escalating challenge of water scarcity, intensified by water-energy interdependency, demands an urgent shift towards sustainable solutions. As this concern heightens, the focus on water-energy-infrastructure-human nexus (WEIHN) becomes pivotal to achieving sustainable and resilient water management strategies. In the context of rapid urbanization and population growth, decentralized water systems (DWS) have emerged as a promising alternative to centralized water systems (CWS), offering cost-effective and environmentally sustainable solutions tailored to local water needs and demands. However, a comprehensive and integrative analysis of the economic, social, and environmental impacts needs to be considered before the implementation of DWS in WEIHN. Therefore, analyzing WEIHN at a cross-sectoral level and exploring the roles of infrastructure and humans can provide valuable insights into the challenges and opportunities associated with DWS development. This review provides a forward-looking roadmap that highlights the critical roles of the infrastructure and human perspectives in WEIHN. First, we identify roadblocks in the CWS and then point out the targeted areas supporting DWS. Second, we investigate the current capability and limitation of infrastructure spatial configuration, safety-related metrics, and interdependency in WEIHN. Third, we emphasize the significance of various human roles, including end-users, operators, and decision-makers in WEIHN. Finally, based on the review findings in water, energy, infrastructure, and human dimensions, we conduct a comprehensive in-depth analysis of innovative technologies (e.g., sensor fusion, digital water infrastructure) and effective management policies.
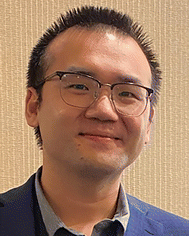 Yuankai Huang | Dr. Huang earned his B.S. in Environmental Science and M.S. in Environmental Engineering at the South China University of Technology (Guangzhou, China), and his Ph.D. in Environmental Engineering at the University of Connecticut in 2022. He is currently a Postdoctoral Fellow at the Georgia Institute of Technology. His research focuses on pioneering real-time water quality sensor design, renewable energy technologies development, and data-driven methods to revolutionize digital water-energy infrastructure. |
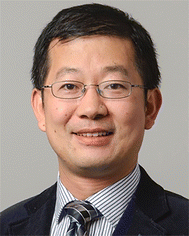 Wei Zhang | Dr. Zhang earned his B.S. and M.S. in Civil Engineering at the Tongji University (Shanghai, China) and his Ph.D. in Civil Engineering at Louisiana State University. He is currently an Associate Professor and Castleman Term Professor of Engineering Innovation in the Department of Civil and Environmental Engineering at the University of Connecticut. Before joining UConn, was a senior specialist in Technip USA Inc. Dr. Zhang's research focused on the resilience of civil infrastructure and systems, wind engineering, natural hazards, and community resilience. |
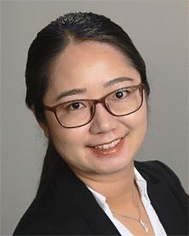 Jin Zhu | Dr. Zhu earned her B.S. in Construction Management at the Southeast University (Nanjing, China), and her M.S. in Public Administration and Ph.D. in Civil Engineering at the Florida International University. She worked as a Postdoctoral Research Associate at the Texas A&M University. She is currently an Assistant Professor at the University of Connecticut. Her research focuses on addressing resilience challenges in complex socio-technical systems, such as complex construction engineering projects, infrastructure systems, and communities. |
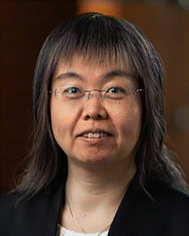 Baikun Li | Dr. Li earned her B.S. and M.S. in Environmental Engineering at Harbin Institute of Technology (Harbin, China) and her Ph.D. in Environmental Engineering at the University of Cincinnati. After her post-doctorate research at the Pennsylvania State University (University Park), she started her career as an Assistant Professor at the Penn State Harrisburg. She then moved to the University of Connecticut in 2006. Dr. Li is currently a Centennial Professor of Environmental Engineering in the Department of Civil and Environmental Engineering at the University of Connecticut. Her research focuses on water quality monitoring, energy-saving water treatment technologies, water-energy nexus, and resource recovery from wastewater. |
Water impact
This review critically examines the evolving roles of decentralized water systems (DWS) within the water-energy-infrastructure-human nexus (WEIHN) framework. We focus on infrastructure and human perspectives to identify opportunities and challenges, propose innovative technologies for water management, and suggest potential policies. Our insights are expected to guide future research and practice in designing and implementing DWS with significant impacts on water sustainability and resilience.
|
1. Introduction of the water-energy-infrastructure-human nexus (WEIHN)
As the world population is expected to increase by 2 billion to around 9.7 billion in 2050,1 pursuing sustainable solutions for water scarcity and energy crisis, producing renewable energy, and protecting humans and the ecosystem are increasingly highlighted for future water-energy nexus (WEN). The WEN refers to the interdependency of the two most significant resources, water and energy, in which they are produced, treated, distributed, and(or) recycled with different scales varying from community to global field.2–5 As the demand for water resources to satisfy the requirements of the water-energy interdependency continues to grow (e.g., water demand for power generation is expected to increase 74% between 2005 and 2030 in the Rocky Mountain region6), a comprehensive analysis that considers the infrastructure and human dimensions is critical to ensure the efficient and sustainable water supply and management in the next generation water-energy-infrastructure-human nexus (WEIHN).
Traditional water supply and treatment systems within the WEIHN are primarily centralized, encompassing water collection, treatment, and energy generation managed from a single core unit.7,8 These centralized water systems (CWS) have typically been favored, particularly in densely populated areas, due to their economies of scale and the capacity to handle large-scale water treatment and distribution.9,10 For instance, a life cycle assessment (LCA) for the City of Atlanta's CWS revealed that the CWS contributed 81% of the average impacts of a single family home resident per year in terms of the annual water use.11 However, the burgeoning pace of urbanization and population growth escalates the distances required for water and energy collection and conveyance. This expansion leads to increased inefficiencies within CWS as sprawling urban areas result in greater energy loss during transmission in the WEIHN, making the system uneconomical and unsustainable.12,13 In response to these challenges of CWS, decentralized water systems (DWS) have begun to emerge as a promising alternative, which offers cost-effective, environmentally sustainable solutions tailored to local water needs and demands.14 Despite facing historical impediments, such as a lack of technological advancements, financial constraints, and inadequate policy support,14–16 DWS implementation has become increasingly feasible due to advancements in water treatment, recycling, and monitoring technologies,17 robust policy support,18 and a heightened awareness of community participation.19 However, a comprehensive and integrative analysis of the economic, social, and environmental impacts should be considered before the implementation of DWS in a WEIHN.20 In this context, analyzing WEIHN from a cross-sectoral perspective and understanding the roles of infrastructure and human engagement can provide valuable insights into the challenges and opportunities associated with DWS development, including the need for reliable and resilient infrastructure, as well as the importance of engaging with stakeholders and communities to promote sustainable water and energy use.
Our systematic literature review reveals that research on WEIHN has grown rapidly in the last decade, as demonstrated by 3470 papers published between 2012 and 2022 (Fig. 1). However, limited attention has been given to the infrastructure (278 papers) and human (389 papers) dimensions of WEIHN, which are critical to achieving sustainable and resilient water and energy systems. Most existing studies and reviews on WEIHN have only focused on the flow of resources (water and energy) and thus fail at elucidating their interactions with infrastructure and human factors in a systematic framework. Such deficiency is concerning as infrastructures play a crucial role in generating and delivering resources, and their performance is dependent on the reliability of their components as well as human behaviors.21,22
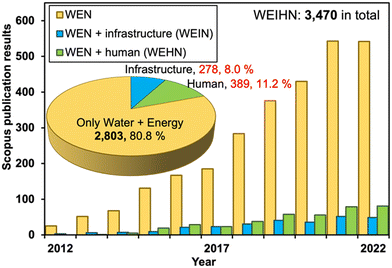 |
| Fig. 1 The number of articles on WEIHN since 2012. The yellow block indicates WEIHN-related research, the blue block denotes articles considering an infrastructure perspective (water-energy-infrastructure nexus, WEIN) and the green block stands for articles considering human perspective (water-energy-human nexus, WEHN). The data was obtained from a comprehensive literature search conducted on Scopus by searching with the article title, abstract, and keywords. The following keywords and their combinations were used: WEN: “Water-Energy Nexus”; WEIN: “Water-energy Nexus” AND “Infrastructure”; WEHN: “Water-Energy Nexus” AND “Human”; WEIHN: WEN+WEIN+WEHN. | |
Although there has been some exploration of infrastructure-based interdependency in WEIHN, these studies predominantly focused on physical interdependency and cost optimization but overlooked the critical safety-based performance metrics to understand the vulnerability of interdependent infrastructures.23,24 In addition, the various roles of human behaviors, including water and energy consumption and decision-making among end-users, operators, and policy makers, immensely influence WEIHN. Capturing human behavior data, both qualitatively and quantitatively, however, poses a significant challenge, thus underscoring the need for more methodological advancements in this area of the WEIHN.25
This review focuses on bridging the knowledge gap between the DWS and the current understanding of resources, infrastructure, and humans in WEIHN. We first identify the roadblocks in the CWS and then point out targeted areas supporting innovations in the DWS. Secondly, we delve into the current capabilities and limitations of infrastructures within WEIHN, concentrating specifically on spatial configuration, safety-related metrics, and interdependencies, with reference to both centralized and decentralized systems. Thirdly, we emphasize the significance of various human roles, including end-users, operators, and decision-makers in centralized/decentralized WEIHN. Finally, we propose a forward-looking roadmap for the next-generation WEIHN. Based on the review findings in water, energy, infrastructure, and human dimensions, we present a comprehensive and insightful analysis of innovative technologies (e.g., sensor fusion, digital water infrastructure), and suggest the potential effective policies on water sustainability and water management.
2. Critical analysis of water systems in WEIHN
This section delivers an inclusive overview of water systems within WEIHN, particularly emphasizing the challenges embedded in the existing CWS that leans heavily on traditional technologies for extensive water treatment and conveyance and is unsustainable in the face of escalating water and energy demands (Fig. 2a). We delve into water sustainability within the existing WEIHN framework, spotlighting the current state and challenges tied to water-energy interdependency, distribution networks, monitoring methods, and process control models of CWS. Furthermore, we offer insights into the prospective evolution of DWS within the future-generation decentralized WEIHN, and illuminate the pathways towards more sustainable and efficient water management strategies.
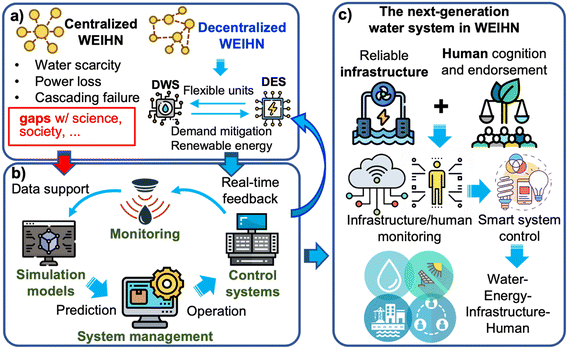 |
| Fig. 2 Comprehensive scheme demonstrating the centralized and decentralized WEIHN. a) Current status of centralized and decentralized WEIHN. b) Future perspective of monitoring, models, and control strategies in WEIHN. c) The next-generation water systems in WEIHN by combining infrastructure and human perspectives. | |
2.1 Current status of centralized water systems (CWS)
The symbiotic relationships between water and energy within the WEIHN are pivotal to ensuring energy generation, resource recovery, and preservation of water safety and quality.26 The CWS inherently offers advantages due to its economies of scale. Operating on a large scale allows for more efficient resource utilization, with the costs of major infrastructural investments distributed over a larger consumer base.27 The centralized water-energy models enable standardized processes and large-scale treatment facilities, which typically result in lower per-unit treatment costs.28 The cost dynamics of water reclamation and distribution in a centralized plant can be influenced by population density. For example, when considering a hilly topography, the total cost is estimated to be around $33.24 per m3 for a sparser population density of 96 people per km2, while this cost dramatically decreases to $4.94 per m3 when the population density increases to 3860 people per km2.29 This comparison illustrates the profound impact that population density can have on the economic efficiency of CWS, highlighting an advantage in denser areas. Furthermore, the centralized networks allow for the strategic placement of treatment facilities, minimizing transport costs and enhancing operational efficiency.30 These economies of scale contribute to the potential for greater affordability and consistency in water quality and supply within CWS.
However, in the face of steadily growing water demands and stringent water quality regulations, water extraction and distribution within the existing CWS becomes energy-intensive. Regrettably, the corresponding energy demand is predominantly met by the centralized energy system (CES), which is heavily dependent on traditional fossil fuels and centralized water-energy infrastructure.31 This structure results in extensive water consumption during energy production, specifically for cooling, while processes such as coal mining have caused severe water pollution.32 For instance, in the United States, thermal power plants accounted for ∼41% (∼200 trillion liters) of the total annual water withdrawals in 2017.33 Although efforts to improve wastewater treatment and water transport/reuse efficiency present a path towards enhanced energy efficiency and accompanying environmental and economic benefits, current centralized infrastructures present huge challenges, including substantial energy losses along extensive transmission and distribution lines, and the potential for cascading failures within interconnected networks (Fig. 2a). Addressing these challenges to ensure sustainable water management is critical, particularly in the context of shifting towards a more decentralized and sustainable WEIHN.
In the pursuit of addressing the various challenges in CWS, substantial strides have been made in developing innovative technologies and strategies to reduce energy consumption and improve treatment efficiency. One notable example is the development of capacitive deionization (CDI) technology, which has gained popularity in recent years as a means of recovering nutrients and metals from water and wastewater streams by electrosorption.34–36 The estimated energy consumption of the CDI process has been proved to be more than an order of magnitude lower than traditional recovery techniques such as reverse osmosis.37 Despite this promise, CDI suffers from poor scalability (limit to cm3 size38), deficient conductivity, and low specific surface area of electrodes.39 Although high conductivity and large surface area electrodes have been developed, their economic feasibility for widespread use remains a barrier, limiting the applicability of CDI in CWS.40,41 An alternative approach for enhancing energy efficiency in CWS is the implementation of integrated basin management strategies for freshwater management (Fig. 2b). However, striking a balance between energy efficiency and water contaminants removal - particularly for specific pollutants like heavy metals,42 microplastics,43 or perfluoroalkyl substances (PFAS)44 – is challenging due to existing technological constraints and knowledge gaps (e.g., studies often overlook the system-level context and the manufacture scalability of new membrane materials at reduced energy expenditure45) (Fig. 2a and b). Hence, there is an imperative need to develop alternative water systems capable of circumventing these limitations and enhancing water treatment efficiency.
2.2 Next-generation decentralized water systems (DWS)
To address the challenge of balancing contaminant removal efficiency and energy consumption in the CWS, decision-makers are turning to a decentralized resource management strategy – decentralized WEIHN that involves supplying energy and treating water through a series of on-site processes rather than gathering all sources into a hub.46–48 In a decentralized WEIHN, DWS combines small-scale advanced water treatment technologies, such as granular activated carbon (GAC)-electrochemical systems49 and constructed wetlands,50 to meet water quality requirements at lower energy consumption than a centralized WEIHN. The cut-off point at which DWS becomes more economically feasible is context-specific and influenced by various factors such as population density, technological advancement, availability of local resources, and regulatory environment. In areas with sparse populations or regions that require adaptive responses to fluctuating demands, the economics of DWS may outweigh those of CWS.51 For instance, the decentralized rural wastewater treatment systems generally produce effluents with chemical oxygen demands, 5 day biological oxygen demands, and NH4+–N concentrations below 50 mg L−1, 10 mg L−1, and 5 mg L−1, respectively, which allows the water to be reused on site for irrigation in some of the rural areas in China.52 Localized systems might enable the leverage of specific renewable resources, reduction in transmission losses, and the provision of more resilient services in the face of systemic failures, contributing to a potential economic advantage in certain circumstances.53 Identifying this cut-off point requires a detailed cost-benefit analysis, taking into account both immediate operational expenses and long-term impacts on sustainability, resilience, and adaptability. In order to reduce energy usage, a decentralized energy system (DES) can be incorporated into the DWS, thus enhancing efficiency and flexibility in the decentralized WEIHN, especially during periods of power loss propagation caused by component failures (Fig. 2a).54 Furthermore, DWS can independently maintain resource supplies under critical system failures in traditional water and energy supply systems.14 A multi-site DES incorporating renewable energy sources such as biofuels and hydroelectric power can alleviate the demand for CWS built in densely populated areas with diminishing natural resources.55,56
However, the implementation of decentralized WEIHN still faces hurdles, such as scaling up early-stage novel treatment technologies like CDI, microbial fuel cells (MFCs), and solar-driven membrane desalination due to high material cost and energy consumption.57,58 In addition, limited political legislation and governance attention remain a challenge, as the importance of scale in space and time has been largely ignored.59 One major concern for these challenges is the poor availability of human resources, specifically trained personnel, to operate and manage decentralized systems.60 The need for specialized skills and local expertise poses an obstacle in many regions, particularly in remote or underserved areas. Determining a balance between reliable and sustainable resource supply and reasonable capital costs remains a major problem.61 Despite DWS markedly improving contaminant removal efficiency and lowering energy consumption via the integration of small-scale advanced water treatment technologies, its implementation can be difficult in areas with scarce water resources and limited water collection options, often resulting in CWS as the sole solution.62 Considering that DWS is frequently managed by a diverse array of stakeholders, such as decision-makers, operators, and end-users, it becomes critical to prioritize investments in infrastructure and policy frameworks that support the deployment and expansion of decentralized WEIHN to ensure a reliable and sustainable supply of resources for future generations (Fig. 2c).63
2.3 Current state and future perspective of monitoring, models, and control strategies in the water systems of WEIHN
Understanding the water systems in DWS is critical for ensuring efficient and sustainable resource management. The challenges of managing DWS arise from the diverse range of consumers from different cultural backgrounds, income/expenditure levels, education levels, and individual habits, which result in perceived risks to human health and inconsistent knowledge cognition.64 To address these challenges, the legal and regulation institutes set for DWS and DES have been established and developed worldwide in the past decades.65–67 However, the distribution and treatment systems of DWS/DES cannot be categorized or supervised according to one legal department, leading to ambiguous attribution of responsibility and liability towards the institutes involved.68 Furthermore, with the development of advanced technologies that can improve the removal and purification efficiencies in DWS, various influencing factors, such as weather,69 geometry,70 shocks,71 land types,72 and water contaminants (e.g., metals,73 nutrients,74 bacteria,75 microplastics,76 pharmaceuticals,77 and pesticides78) must be analyzed precisely and comprehensively. Therefore, it is crucial to understand the current status of monitoring and control technologies in DWS in order to ensure efficient and sustainable resource management (Fig. 2b).
Traditional sensor technologies used in the water systems of WEIHN have primarily focused on water-based applications, geared towards enhancing wastewater treatment efficiency and producing clean drinking water.79,80 However, for the next-generation WEIHN, the application of sensors must extend beyond the water dimension and incorporate the perspectives of infrastructure and humans to meet the divergent demands of centralized and decentralized systems (Fig. 2c). Although sensors and sensing technologies have made headway in water-energy infrastructures, particularly in the precise measurement of resource flow,81 several key challenges persist in the monitoring processes, such as poor accuracy in long-term data collection and processing capacity due to the limitations of sensor materials and data processing capabilities. For instance, the lack of effective materials to prevent biofouling, and the absence of robust data processing algorithms to correct sensor data drifting, have been identified as the major barriers.82–84 The data sparsity problem impedes the assessment of WEIHN performance and hinders stakeholders and decision-makers from foreseeing emerging problems and executing efficient control methodologies.85 In addition, the optimization of sensor networks within DWS is a key consideration for economic viability. By focusing on a minimum viable product, unnecessary sensors can be removed, reducing both capital investment and maintenance costs.86,87 For instance, to optimize resource use and minimize cost, a system utilizes data fusion and reconstruction techniques has been developed to enable the sensor network, enhance its utility (>95%), and reduce energy consumption (by >20%).88 This approach promotes a more streamlined and cost-effective deployment of decentralized WEIHN, making them more accessible and attractive to various stakeholders. Therefore, there is a pressing need to develop more advanced sensor technologies, algorithms, and sensor networks for the next generation of WEIHN.
Various models can be established to optimize the WEIHN performance based on the monitoring results obtained from different sensor technologies (Table 1). For instance, the system-wide economic-water-energy model (SEWEM) has been used to explore the correlation between energy requirements and supply constraints and determine its impacts on water and land allocation decisions.89 Because most of the current models mainly focus on operation, economic, and management principles to obtain information and understand interactions and interdependencies of WEIHN,7,90–93 it is important to consider human factors in the model development for future WEIIHN as human behaviors can enormously affect the WEIHN performance (Fig. 2c). For instance, operators can properly operate water and energy infrastructures to ensure the stability of resource supply at the community level.94 Similarly, decision-makers can formulate policy initiatives (e.g., non-potable water storage and reuse in irrigation and the installation of mini power generator sets) to effectively deal with uncertainties in the availability of water and energy fluctuations.95 However, political and practical aspects are often not considered in the existing WEIHN models. To address this challenge, innovative methods have been developed to incorporate political and social perspectives into the WEIHN simulation. For example, an agent-based spatiotemporal model has been used to simulate water-energy consumption under different scenarios of human activities,96 and a system dynamics model has been applied to investigate water and energy use under different scenarios using household resource consumption data.97 The inclusion of infrastructure and human parts in the model development can render a more comprehensive understanding of WEIHN, enabling the stakeholders and decision-makers to identify emerging problems and execute efficient smart control methodologies (Fig. 2c).
Table 1 Current models applications in WEIHN
Methods |
Model type |
Purpose |
Limitation |
Ref. |
System-wide economic-water-energy model (SEWEM) |
Qualitative |
Explore the (un)importance of energy requirements |
Do not consider long-term dynamics |
89
|
Water–energy–food (WEF) Nexus tool 2.0 |
Quantitative |
Identify sustainable national resource allocation |
Empirically based, only assumes linear relationships |
98
|
The climate, land, energy, and water systems (CLEWs) framework |
Qualitative |
Discuss two or more resource interactions under different political contexts |
Not stable at the different levels of the analysis |
99
|
Multi-scale integrated analysis of societal and ecosystem metabolism (MuSIASEM) |
Quantitative |
Generate a robust quantitative assessment of the energy, water and food nexus |
Extensive data required |
100
|
Water evaluation and planning (WEAP) |
Quantitative |
Calculate water and carbon footprints |
Did not model resources and transformation sections |
101
|
Computable general equilibrium – system dynamics and water environmental model (CGE-SyDWEM) |
Quantitative |
Predict future energy use, CO2 emissions, economic growth, water resource stress, and water quality changes |
Extensive data required |
102
|
Multi-regional input–output (MRIO) model |
Quantitative |
Quantify the energy for water and water for energy in the city- and regional-level scales |
Unstable at different population density areas |
103
|
Urban water optioneering tool (UWOT) |
Qualitative/quantitative |
Facilitate the selection of combinations of water-saving strategies and technologies |
Inability to handle complex decision-making algorithms |
104
|
Integrated computable general equilibrium (CGE) mode |
Qualitative/quantitative |
Study the impacts of energy tax policy on water withdrawal |
Complex with too many inputs |
105
|
After acquiring and processing data, water and energy models can be developed and applied to implement efficient control strategies in the water systems to formulate effective solutions for energy-intensive water treatment and distribution by achieving system visualization and virtualization towards enhanced resilience (Fig. 2b).82 Control systems enable multi-variable systems management by applying physical constraints and maintaining the balance between generation and demand in WEIHN, as well as facilitating data acquisition efficiency by improving serviceability.106 Traditional optimization of water system control models primarily aims to minimize system costs. For example, a multi-objective mixed-integer optimization model has been developed to optimize the energy and water networks for a neighborhood of residential units, with the goal of maximizing sustainability.107 Evaluative control models (e.g., life-cycle assessment,108 total economic assessment,109 and scenario analysis110) and adoption methods (e.g., individual DWS and hybrid water system by integrating DWS with CWS111) have been proposed to testify the possibility and feasibility of implementing DWS into WEIHN. Control models such as Advanced Process Control systems were utilized for data collection and system management, reliant on programmable logic controllers.112 Addressing the limited availability of human resources may involve automating key processes to minimize the need for human intervention, building capacity to develop training programs for local operators, and integrating remote monitoring and support to provide remote assistance and expertise that can overcome localized human resource constraints. These controllers play a pivotal role in overseeing the system and executing process commands.
Currently, the data collection on infrastructure and human aspects is still limited due to the lack of consensus on defining data for system failure and quantifying concerns related to security.113 To solve this problem, crowd sensing/sourcing has been developed to supply enormous datasets and engage infrastructure and human aspects in the WEIHN analysis.114 However, crowd sensing hasn't demonstrated consistent success across all the relevant domains (e.g., detection of microplastics115) due to scarce incentives for public participation. As for infrastructure, flow-based and graph-based models have been widely used to investigate the interactions between water and energy resources.116–118 The optimal design and control of infrastructures meet the resource demand and prevent inept infrastructure investment.119 Nonetheless, the strategic planning and management of infrastructure operations at different levels – from individual units like solar panels120 to larger systems like local power grids121 – remains an unmet research need. This complexity arises from the intricate nature of policy-making and resource allocation necessary for effective operation. Consequently, the development of WEIHN models should consider not only operational, economic, and management principles but also infrastructure and human factors to advance performance and resilience (Fig. 2c).
3. Consideration of multifactorial infrastructure for WEIHN
The performance of infrastructure plays a critical role in linking water and energy resources in WEIHN. Existing WEIHN studies have largely focused on large-scale infrastructure such as water treatment and power plants,122–124 while the decentralized nature of next-generation WEIHN requires a reexamination of the link between water-energy and infrastructure since it involves more diverse and dispersed infrastructure elements, including DWS (e.g., rainwater harvesting,125 on-site wastewater treatment126), DES (e.g., solar energy,127 micro-hydro power,128 biogas,129 and biomass130) and integrated water-energy systems (e.g., water-energy microgrids131). The unique characteristics of infrastructure within a WEIHN imply that component failures caused by extreme weather events or aging can result in large-scale system breakdowns, as seen in the 2021 Texas power crisis.132 Although the State of Texas narrowly escaped a total grid failure, power outages still affected 4.5 million customer premises. These blackouts not only caused severe inconvenience but also resulted in a minimum economic loss of $130 billion, in addition to the tragic loss of numerous lives.133 Consequently, inclusive consideration of infrastructures is vital in serving customers and constructing resilient systems with advanced capabilities of withstanding natural disasters. This section focuses on a multifactorial assessment of WEIHN performance from the infrastructure perspective, highlighting three critical aspects to bridge the knowledge gap in this area.
3.1 Evaluation of infrastructure performance in WEIHN
The evaluation of infrastructure performance in WEIHN can be categorized into three essential aspects: spatial configuration, performance metrics, and interdependency (Fig. 3). Specifically, infrastructure spatial configuration pertains to the planning of utility infrastructure in WEIHN,134,135 infrastructure performance metrics refer to resource consumption or infrastructure safety indicators in WEIHN, and infrastructure interdependency highlights the associations between water and energy infrastructure systems.136,137
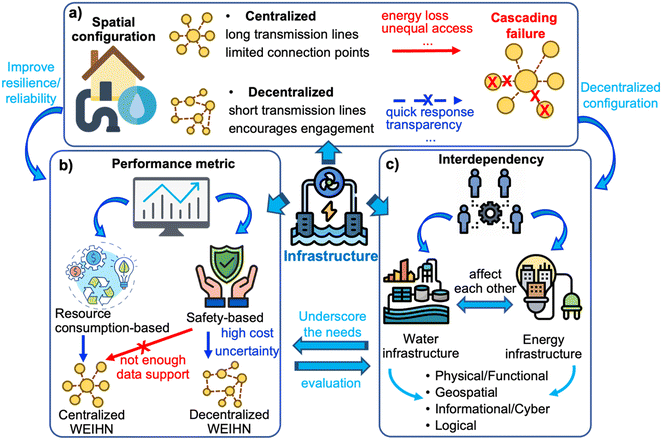 |
| Fig. 3 The comprehensive scheme of the roles of infrastructure in WEIHN. a) The spatial configuration performance in the centralized and decentralized WEIHN. b) The performance metric in the centralized and decentralized WEIHN. c) The interdependency between water and energy infrastructures. | |
Infrastructure spatial configuration.
Infrastructure spatial configuration plays a vital role in the deployment of WEIHN since the spatial pattern of patches makes up the infrastructure networks and determines the efficiency and resilience of WEIHN.138 In a traditional centralized WEIHN, infrastructure networks are typified by a small number of large-scale production facilities, such as drinking water plants serving a vast range of isolated consumers. Although this configuration efficiently minimizes the number of routes required to reach various endpoints, it remains vulnerable to cascading failures incurred by natural disasters or targeted attacks (Fig. 3a).139 Especially, centralized infrastructure networks necessitate extensive transmission lines, increasing the risk of energy loss and failure during transmission under targeted attacks triggered by hurricanes or earthquakes.140 Furthermore, centralized WEIHN usually has a limited number of connection points often located in urban areas, which creates an urban–rural divide and leads to unequal access to water and energy resources.141 To address these challenges incurred by the complexity of infrastructure systems, adaptation strategies should be vigorously examined in the context of infrastructure distribution, connection, and disaster response.
Transitioning from a centralized WEIHN to a decentralized WEIHN involves substantial changes in terms of infrastructure spatial configuration and disaster handling approaches. The decentralized WEIHN configurations feature relatively smaller producers than centralized systems, with generation and potential storage capacities co-located with end-users (Fig. 3a). This configuration allows infrastructures to serve as a generator and distributor of resources and as storage to reduce cascading failures by shortening the length from generators to end-users and responding quickly to failure requests.47 For example, the integration of renewable energy sources with electrolyzers can allow for the local production and storage of hydrogen, alleviating reliance on large-scale infrastructure and enhancing the system's responsiveness to failure requests.142 Furthermore, compared to a centralized WEIHN that often relies on a limited number of stakeholders (e.g., government agencies) to make decisions related to infrastructure spatial configuration, a decentralized WEIHN integrates emerging technologies (e.g., smart meters25 and internet of things (IoT) devices143) (Fig. 3a), and bolsters community engagement and participation throughout the decision-making processes related to water and energy infrastructures. When exposed to cascading failure and natural disasters, high transparency and accountability in the decentralized WEIHN can improve system performance and provide real-time data for resource use. However, DWS in rural settings may face challenges in ensuring the reliability of drinking water from wells and drainage to septic systems during disasters, since wells can become contaminated due to flooding or other disruptions, while septic systems may fail if drainage is compromised.144 Consequently, households in these areas may need to rely on generators or other DES to ensure the continuous operation of DWS during disasters.145
Infrastructure performance metric.
Infrastructure performance metrics are crucial for evaluating the effectiveness and efficiency of WEIHN, and can be classified into two categories: resource consumption-based and safety-based (Fig. 3b). Resource consumption-based performance metrics provide information on the WEIHN status and are directly related to end-users and decision-makers.146–148 For example, it was estimated that a middle-sized reverse osmosis (RO) plant with a capacity of ∼25
000 m3 per day and energy demand of ∼5 kW h m−3 could provide water to 48
000 households. This is comparable to providing electricity to 10
300 households of the same size, underlining the significant energy demand in the current WEIHN.149 The excessive dependence on resource-based metrics may obscure the impact of damages or failures on the overall behavior of WEIHN, underscoring the importance of safety-based metrics. Different from the resource consumption-based metrics, safety-based metrics explore the infrastructure components' behavior concerning the failure and dysfunctionality in infrastructure.150 Unfortunately, most existing studies tend to assess the infrastructure performance from a resource perspective, and neglect safety considerations due to the sparse availability of data on resource consumption and generation (Fig. 3b). Consequently, the current WEIHN infrastructure is characterized by high resource consumption and low system safety.151
On the matter of performance metrics, the key advantage of decentralized WEIHN lies in its potential for rapidly enhancing the resilience (the capacity to recover from damage) and reliability (the ability to resist internal or external disruptions) of crucial infrastructures. Decentralized WEIHN facilitates resource transportation and reduces losses compared with centralized WEIHN.152,153 For example, the cost of energy in Sub-Saharan Africa was saved by approximately 90% when the majority of the region's energy was provided by decentralized solar energy systems.23 However, decentralized storage infrastructures pose certain challenges (Fig. 3b). For instance, the elevated initial costs associated with installation and construction may obstruct their broad deployment, particularly in settings with limited resources.154 Furthermore, water resource generators in decentralized WEIHN are typically subject to considerable uncertainty and low reliability due to the environmental variations encountered over temporal and spatial scales.155 Thereby, it is crucial to balance the benefits and challenges of the infrastructure performance metrics in the decentralized WEIHN. Decisions about water and energy systems design and planning should take into account the specific context in which these systems are implemented.
Infrastructure interdependency.
Interdependency in the WEIHN refers to the interactions between different water and energy infrastructures within a system. In a centralized WEIHN, water and energy infrastructures are tightly interdependent, with the functionality of one system directly affecting the other (Fig. 3c). For example, power plants often require large amounts of water for cooling, and the failure of water distribution pipelines could cause the loss of functionality of the power generators.156,157 While standard data collection, such as supervisory control and data acquisition (SCADA), can identify physical interdependency in terms of resource consumption,150 other forms of interdependencies may remain undetected until a disruptive event triggers a cascade of failures within the WEIHN. Future studies could explore the role of physical-cyber interdependencies in the WEIHN, including the identification and detection of possible data errors that may affect resource flow.158 Digital twin (DT) is a promising technology to address this need by receiving input from infrastructures and simulating failure scenarios.159,160 However, comprehensively integrating all forms of interdependencies – physical, cyber, geographical, and logical – necessitates further exploration through complex and costly mathematical modeling techniques. Therefore, the intricate interdependence of water and energy infrastructures must be considered to ensure the resilience and sustainability of the WEIHN (Fig. 3c).
Decentralized WEIHN introduces a more intricate web of interdependencies between water and energy infrastructures. Integration of multiple decentralized resource generators reduces the physical interdependency between the central plant and end-user, while the physical interdependence between these decentralized resource generators and the main plant is strengthened, as the stored resources are dispatched to the central plant for backup.91,161 In addition, decision-makers within a decentralized WEIHN need to determine the trade-offs concerning nexus-related issues, since the choice of power generators can substantially influence the physical interdependency between water and energy infrastructures. For example, escalating the use of renewable resource generators (e.g., distributed solar panels) in decentralized WEIHN could diminish reliance on traditional resources (e.g., coal and fuel). For example, with a conservative estimate of 1 kWp solar photovoltaic (PV) capacity per 10 m2 of building roof surface in key sectors, the technical potential for solar power in Malaysia is approximately 11
000 MWp or 11 GWp. This potential could be translated into more than 12
000 GW h of solar-generated electricity, which would account for 20% of the nation's energy demand.162 This transition will assuage the physical interdependency between water and power infrastructures compared to the centralized WEIHN.
3.2 Challenges and future perspectives in next-generation decentralized WEIHN
Infrastructure spatial configuration.
Compared to centralized WEIHN, the decentralized WEIHN typically feature a more distributed and flexible infrastructure spatial configuration, allowing for easier integration of renewable energy sources and improving the system's energy efficiency and reliability.163 However, variations in consumers' access to resources within the decentralized WEIHN could exacerbate inequality of supply across marginalized areas and make the system susceptible to random attacks.164 Consequently, developing efficient methods to detect and/or diagnose failures before the occurrence of potential hazards has been a key area of innovation. Existing approaches (e.g., failure mode and effect analysis (FMEA) to determine the main failure mechanisms and data-driven strategies) require detailed and sufficient failure/maintenance logs,165 posing difficulties for sensor deployment and data processing algorithms. In a decentralized WEIHN, there are numerous small-scale producers and end-users, creating complexity in collecting data related to the status of infrastructure components and resources.166 To address this challenge, wireless sensor network technology presents a solution by enhancing failure diagnosis with real-time high-fidelity data and complementing the sensors for resource flow.167 Moreover, emerging technologies such as geographic information systems (GIS) and optimization algorithms can improve the design of infrastructure spatial configurations by optimizing network connectivity, minimizing costs, and improving system performance.168 However, even if the incorporation of advanced tools such as FMEA and GIS can elevate the capabilities of the infrastructure in decentralized WEIHN, the potential trade-offs in complexity and cost should be recognized. For instance, exploring the integration of machine learning algorithms (MLA) for optimizing energy consumption in the DES requires specialized expertise of personnel and might increase operational costs.169 Implementing these advanced tools should be aligned with the specific needs and budgetary constraints of the WEIHN, ensuring that technology adoption does not inadvertently hamper economic feasibility.
Infrastructure performance metrics.
Given the increased complexity and heterogeneity of decentralized WEIHN infrastructure, new performance metrics that consider the technical aspects of the system, as well as the social and economic aspects (e.g., social equity, environmental sustainability, and economic efficiency), should be developed to better capture the multidimensional nature of the decentralized WEIHN infrastructure.170 Moreover, deploying advanced sensors and data processing algorithms becomes vital to furnish precise and timely information about the performance of infrastructure components.83,171 Real-time monitoring and control can augment the capabilities of failure detection and diagnosis and enable swift response and reorganization of individual components without disrupting the overall system.80 Furthermore, the incorporation of emerging technologies such as artificial intelligence (AI) and MLA can foster the development of predictive maintenance strategies.172 These strategies will enhance the overall infrastructure performance and mitigate the risk of cascading failures, thereby fostering more robust and efficient decentralized WEIHNs.173,174
Infrastructure interdependency.
Interdependence between the water and energy systems requires meticulous consideration to ensure the resilience and sustainability of decentralized WEIHN, in which the increasing use of renewable resource generators can reduce the reliance on traditional resources, and diminish the physical interdependency between water and power compared to centralized WEIHN.175 However, this transition towards renewable resources could inadvertently escalate water demand, potentially straining water resources, instigating water scarcity, and thereby compromising the sustainability of the entire system.176 Additionally, the remote or rural location of decentralized infrastructure presents a unique set of challenges in maintaining and operating the interdependent water and energy infrastructure. These areas typically rely on wells for water and septic systems for drainage, thereby raising the risk of failures and outages without reliable energy support.14 Addressing these challenges calls for the implementation of strategies such as leveraging advanced monitoring and simulation technologies to detect and quantify the interdependencies between water and energy infrastructures.
4. Consideration of the human dimension in decentralized WEIHN
As indispensable resources for human survival, water and energy necessitate sustainable management to ensure societal welfare. A wide variety of human behaviors become significant within the context of WEIHN, including resource production, consumption, recycling, and the operation and maintenance of water and energy infrastructures (Fig. 4a).177 Characterizing human behaviors in WEIHN can elevate our understanding of the spatially and temporally disaggregated water-energy demands resulting from individual consumption patterns. Furthermore, studying human interventions and their impact on WEIHN can enable efficient solutions to improve system performance. This section explores various human behaviors and interactions in WEIHN, and analyzes interventions to change these behaviors in the next-generation decentralized WEIHN. Critical challenges regarding the human dimension in WEIHN are also identified. A comprehensive human-centered framework is proposed as a potential opportunity to improve WEIHN performance from the human perspective.
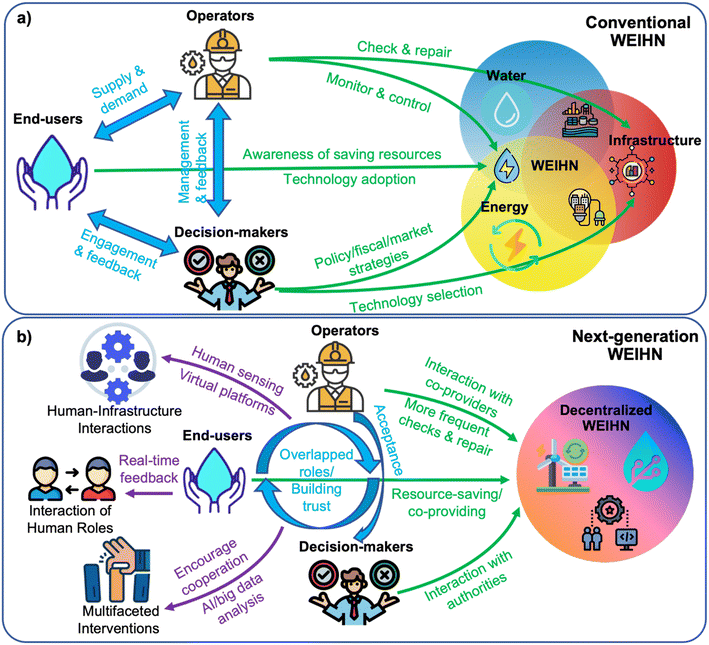 |
| Fig. 4 The roles of human behaviors in WEIHN. a) The human behaviors and the interactions of the three human roles (end-users, operators, and decision-makers) in a conventional centralized WEIHN. b) The human behaviors and the interactions between the three human roles in a decentralized WEIHN, and the possible solutions to address the potential challenges of the human behaviors in the next-generation WEIHN. | |
4.1 Human behaviors in decentralized WEIHN
The optimal operation of WEIHN hinges not only on technological systems and policies but also pivots on the behaviors of three human constituents: end-users, operators, and decision-makers (Fig. 4a). The roles these individuals wield an extensive influence on the sustainable management of existing WEIHN resources. Strategic interventions of these roles can notably influence resource supply and demand, operating conditions of infrastructures, and emissions within the WEIHN framework. Table 2 offers a thorough exploration of human behaviors within WEIHN, accompanied by interventions that may inspire behavioral alterations. It is crucial to understand and address the complex dynamics of these human behaviors in order to achieve effective and sustainable resource management in WEIHN.
Table 2 The analysis of human behaviors in current WEIHN
Human type |
Behaviors |
Analysis methods |
Analytical results |
Ref. |
End-users |
Awareness of resource-saving |
Literature review, a survey of academic experts and two interdisciplinary expert workshops |
Identifies four attributes that need to capture to reflect the uncertainty associated with the paradigm shift |
178
|
Participant observation, survey, participatory action research |
Brings a more holistic understanding of human and nature relationships at the household level |
179
|
Resource-saving adoptions |
Hierarchical and K-means cluster analysis |
Provides water conservation information and engagement opportunities that effectively engage and resonate with complex consumers |
180
|
An agent-based spatiotemporal integrated approach |
People living in the central districts tended to consume more water and related energy |
181
|
Operators |
Monitor and control |
Operational scenario |
Increases resource efficiency and environmental performance of water distribution networks |
182
|
Open loop optimal and model predictive control (MPC) controllers |
A grey water recycling system has a huge potential to conserve water while ensuring efficient use of energy |
183
|
Check and repair |
Conceptual framework |
Provides a robust capacity for future development of energy and water resources |
184
|
Decision-makers |
Policy initiatives |
A multi-criteria decision-making (MCDM)-based social network analysis |
The current WEIHN do not exploit maximum capacities to establish relationships with one another |
185
|
A fuzzy-cognitive mapping approach |
Reveals the interdependencies among nexus sectors and the existence of unanticipated effects |
186
|
Fiscal measurements |
A macro-management theory |
Districts with a high welfare mass (WM) do not suffer when a defund is applied, but districts that have a low WM gain from subsidies |
187
|
Technology selection |
Strategic decision-support modeling |
Presents a quantified dependence between the target reliability level and the penalty for exceeding water use |
188
|
Interactions of different roles |
Sustainable development goals |
The SDG synergies approach based on available information |
Raises important issues for understanding and managing cross-scale interactions |
189
|
Marketing strategies |
Agent-based models (ABMs) |
The need to integrate richer and more realistic human behavior into WEIHN research has been recognized |
190
|
Resource-saving adoptions |
Sensitivity analysis of an agent-based model |
Provides an innovative framework that simulates human interactions and evaluates how these interactions can affect WEIHN |
191
|
End-users.
The consumption of water and energy within WEIHN is shaped by the behaviors, attitudes, and habits of end-users, particularly their proclivity towards resource conservation and the adoption of resource-saving technologies (Fig. 4a). To accurately capture and analyze consumption patterns at a regional level, it is crucial to elucidate end-users' behaviors on an individual basis. End-users' awareness of saving water and energy and their adoption of resource-saving appliances differ depending on their socio-demographic backgrounds (e.g., age, income, education level, and social connections).192,193 In order to effectuate changes in end-users behaviors within WEIHN, interventions can be instituted via policy measures, fiscal incentives, marketing techniques, and social networks.194 For example, policies related to promoting the use of reclaimed water for irrigation have the potential for potable water savings (ranging from 1.7% to 50.5%) and reduction in sewage generation (between 2.1% and 52.1%) among end-users in Joinville, Southern Brazil, according to city-scale analysis.195 Fiscal measures such as penalties for excessive resource use can motivate end-users to conserve water and energy resources.196 These interventions require collaboration among various stakeholders, including government policy-makers, water and energy resource providers, and sustainability campaign initiators. Implementing such interventions would have a long-term impact on reducing total resource usage in a region, as end-users are the primary consumers of water and energy resources.
Operators.
Operators are crucial in maintaining the stability and safety of the water and energy infrastructures within WEIHN. Their daily monitoring and control activities, combined with periodic inspections and repair work, contribute to both resource supply stability and infrastructure safety (Fig. 4a). Operators can improve the efficiency and reduce waste of WEIHN by detecting and repairing infrastructure damage and checking and repairing water leaks in a water distribution system.197 Changing operator behaviors can be achieved through cross-sector coordination efforts, such as team-building activities,198 and educational programs.199 These changes can ensure optimal infrastructure conditions in the long-term, contributing to consistent resource production, supply, and recycling.200 However, a lack of resources, technical capacity, and motivation can hinder operators' ability to detect and address infrastructure damage, reducing the overall efficiency of the WEIHN.201,202
Decision-makers.
Decision-makers are vital to WEIHN as they orchestrate the implementation of policies, fiscal measures, and marketing strategies that influence water and energy production, use, and recycling (Fig. 4a).203,204 A sufficient consideration of decision-makers' behaviors in WEIHN could improve resource availability and reduce resource consumption and thus facilitate regional sustainable development.205 For example, mandates that require the use of solar photovoltaic technology for supplemental energy production can mitigate water demand in traditional power plants, thereby enhancing the system's efficiency.206 Changing decision-makers' behaviors in WEIHN can be achieved through communication with operators and end-users, such as conducting interviews to obtain feedback on critical decisions (Fig. 4a).207 Additionally, cooperation among different sectors (e.g., integrated and coordinated policy-making between water and energy sectors) could lead to more effective decision-making.208 Therefore, engaging stakeholders and ensuring their cooperation in decision-making processes is crucial to achieving sustainable development in a more effective manner.209
Human behaviors in WEIHN have substantial impacts on resource availability, consumption, and sustainability. One key aspect of this is the impact of population increases, particularly due to in-migration. Projections to 2100 estimate that water demand could rise by 28.9% more than in scenarios without labor in migration based on a case study in Tieling City, China.210 End-users, operators, and decision-makers together hold crucial positions, and their behaviors must be acknowledged to augment the precision and fidelity of WEIHN consumption patterns, improve infrastructure safety and stability, and boost regional sustainable development. In most studies, these three human roles are usually considered independently.211,212 A more effective approach towards sustainable development in WEIHN is to engage and cooperate with all stakeholders in decision-making processes, and improve WEIHN efficiency and resource sustainability.
4.2 Human behaviors in the next-generation decentralized WEIHN
Compared to the conventional centralized WEIHN, the next-generation decentralized WEIHN provides end-users with enhanced control of resource consumption, which can be achieved by offering end-users real-time data on their consumption patterns, thereby raising awareness of resource conservation (Fig. 4b).213 For example, smart water metering systems provide end-users with real-time data on their water consumption patterns, enabling them to identify opportunities to conserve water and reduce costs.214 These systems can also detect leaks caused by pipeline aging problems in water supply systems, alerting end-users to potential problems and enabling them to take action to prevent further damage.215 It is important to note that the roles of end-users, operators, and decision-makers in decentralized WEIHN can overlap and evolve over time based on the needs and preferences of the community (Fig. 4b). For instance, in a community-managed rainwater harvesting system, the end-users could also be decision-makers and operators who make collective decisions on the allocation of harvested rainwater, the maintenance and repair of the infrastructure, and the adoption of new technologies or practices.216 DWS brings immense resource-saving opportunities from the human perspective since end-users are expected to play a more active role. To maximize the benefits of decentralized WEIHN, it is essential to develop strategies that engage end-users in the development and operation of DWS.
Within the decentralized WEIHN, operators are required to carry out more frequent monitoring and inspections due to the inherent vulnerability of the decentralized configuration's water and energy infrastructure (Fig. 4b).217,218 This susceptibility originates from the intermittency of renewable resources, such as rainwater and solar radiation, attributable to weather fluctuations.219 Moreover, extreme weather conditions could potentially cause infrastructure damage – for example, heavy rainfall could lead to leaks, while intense solar radiation could result in damage to solar panels.220 In some cases, operators may also be end-users, such as individual property owners who may operate and maintain their own water supply and treatment systems in rural areas.221 Furthermore, industrial parks or estates might operate their decentralized water systems that are designed to cater to their specific water requirements. These systems could encompass treatment facilities, distribution networks, and storage tanks, all under the management of the park or estate.222 Thereby, the roles of operators, end-users, and decision-makers in decentralized WEIHN are highly interrelated and overlapped, highlighting the need for a comprehensive and integrated approach to WEIHN management, which takes into account the diverse perspectives and interests of all stakeholders (Fig. 4b).
In decentralized WEIHN, decision-makers are critical for selecting appropriate technologies and formulating standards to ensure safe and reliable service delivery. Because the decentralized rainwater harvesting and greywater reuse systems require hybrid water treatment technologies to remove numerous types of contaminants (e.g., heavy metal, pathogens, microplastics), decision-makers are responsible for selecting and adopting hybrid water treatment technologies suitable for DWS (e.g., combining human intervened-based and nature-based technologies).223,224 In addition, decision-makers may also be operators and end-users when the agricultural cooperatives operate and manage their own water supply and distribution systems (e.g., wells, pumps, irrigation systems, and storage tanks) owned and operated by the cooperative. In this case, the agricultural cooperative is responsible for maintaining the system and ensuring the water quality and availability for their use.225 Furthermore, decision-makers' choices within decentralized water and energy systems profoundly affect end-user benefits such as public health and electricity costs. Prompt decisions on infrastructure investments, water treatment technologies, and pricing policies can directly influence end-users' access to clean and affordable water (Fig. 4b).226 Hence, it is of paramount importance for decision-makers to engage in open dialogue with end-users and incorporate their feedback into the decision-making process, thus enhancing the effectiveness and responsiveness of management strategies in the decentralized WEIHN.
4.3 Challenges and potential opportunities of the human dimension in WEIHN
Effective management of the next-generation decentralized WEIHN requires an insightful understanding of human behaviors within the systems, as well as the development of multifaceted interventions that target different human agents. Herein, we identify three major human-centered challenges and propose a framework for better understanding and management of human dimensions in decentralized WEIHN.
Challenge for human-infrastructure interactions.
Although many studies have assessed and improved the performance of infrastructures in WEIHN,227,228 few have investigated the human behaviors related to these infrastructures.229 Because the complexity of infrastructures requires extensive technical knowledge and expertise to operate and maintain the decentralized WEIHN, a major challenge of human-infrastructure interactions is to ensure that the infrastructures and technologies are designed to meet the needs of local communities in a broader social and environmental context.230 For example, the water and energy policy decisions are progressively influenced by the social dimensions of WEIHN, a fact underscored by the ongoing large-scale energy transitions worldwide. These transitions encompass the adoption of hydraulic fracturing technologies for oil and gas extraction, the deployment of renewable energy generation to combat climate change, and the development of alternative fuels, as well as hybrid and electric vehicles.231 Another challenge is to develop effective governance structures and policies that can manage the different human roles in the planning, design, and operation of WEIHN.232 Coordination and cooperation between these human roles are critical for ensuring the efficient and sustainable management of DWS. In the future WEIHN, establishing transparent communication channels and delineating roles and responsibilities will be critical, which can be facilitated through collaborative tools (e.g., digital water infrastructure and virtual platforms), real-time communication, and data sharing.233,234 Furthermore, advanced sensors and evaluation tools can enable the continuous monitoring of infrastructure performance, fostering prompt and effective responses to anomalies. The long-term continuous monitoring provided by reliable water sensors is expected to save 44.9% NH4+ discharge, 12.8% energy consumption, and 26.7% greenhouse emission under normal operational conditions.84 The use of these advanced technologies enhances predictive maintenance, reducing the likelihood of unexpected failures and improving the overall performance of infrastructures (Fig. 4b). It should be noted that these advanced technologies require specialized knowledge and skills for operation, maintenance, and troubleshooting. For instance, in a decentralized water treatment facility utilizing advanced sensor networks for real-time monitoring, the complexity of these sensors demands trained technicians and more intricate maintenance procedures.235 Therefore, meticulous consideration of the corresponding human challenges and potential cost implications is essential to achieving a harmonious and economically viable integration within decentralized WEIHN.
Challenge for interaction of human roles.
In the decentralized WEIHN, a major challenge in understanding the interaction and feedback mechanism between end-users, operators, and decision-makers is the difficulty in collecting accurate and real-time feedback data (Fig. 4b). For instance, end-users may exhibit different levels of compliance with specific policies due to their diverse socio-economic backgrounds and transient psychological status,236,237 while operators' comprehension of decisions and level of acceptance may vary over time and affect their operation behaviors.238 The scarcity and varied skill levels of human resources can create discrepancies in implementation, understanding, and compliance with policies across different regions and communities. Hence, gathering precise, real-time feedback from end-users and operators is essential for decision-makers to fine-tune their policy initiatives and management behaviors, enhancing policy acceptance among these key stakeholders. In the decentralized WEIHN, aggregating end-users' feedback regarding a specific policy (e.g., water quality standards) is necessary to estimate potential effects before implementing the policy.239,240 Thus, achieving alignment in infrastructure planning, design, and operation with regulatory needs and public interest hinges upon effective communication and collaboration among diverse human agents within WEIHN.241,242 As an example, frameworks focusing on sustainable environments and circular economies often garner more public acceptance, as they resonate with values deemed increasingly vital to personal and societal identities.243 Future research could strategically encourage bottom-up acceptance of emerging technologies and concepts like climate change mitigation and energy transition, through carefully framed messaging that addresses public concerns and underscores how these innovations can foster a more sustainable society.244 In addition, leveraging automated systems, digital tools, and innovations in remote support can minimize the dependency on extensive human intervention, thereby enhancing efficiency and precision.245
Challenge for multifaceted interventions.
The third major challenge faced in WEIHN management is the lack of effective multifaceted interventions combining various interventions that act on end-users, operators, and decision-makers to achieve optimal management.242 Interventions on any type of human agent alone usually cannot lead to the most desirable outcomes. For instance, in the context of water sustainability, targeting decision-makers solely may not be sufficient to achieve optimal management when implementing a greywater recycling system.246 Decision-makers must interact with end-users and operators in communities and obtain acceptance and support, potentially through a co-design approach.247 However, implementing multifaceted interventions is challenging as it requires cooperation and collaboration among various stakeholders, including academic and research institutions, water and energy equipment manufacturers, media and communications organizations.248 Designing multifaceted intervention strategies depends on a thorough understanding of large-scale human behavior data that can be obtained through data-driven approaches. Potential future strategies encompass the development of incentive mechanisms to foster stakeholder cooperation, enhancing public awareness of integrated WEIHN management, and exploiting emerging technologies like AI and big data analytics for designing more effective interventions (Fig. 4b). Incentives such as tax credits or financial subsidies can encourage the adoption of sustainable practices, such as water conservation and the use of renewable energy sources, which can reinforce the overall sustainability of the WEIHN.249 Leveraging big data analytics can track infrastructure performance, pinpoint areas necessitating improvements, and provide crucial insights that shape policy and investment decisions.250
5. Conclusion, future perspective, and outlook of next-generation WEIHN
5.1 Conclusion
In this critical review, we elaborate on a forward-looking roadmap of WEIHN from a systemic framework. We identify the roadblocks to the centralized WEIHN systems and point out the targeted areas supporting innovations in the decentralized WEIHN systems. We highlight the limitations of traditional CWS and analyze the potential of DWS to provide cost-effective and environmentally water-sustainable solutions tailored to local water needs and demands. Although the studies on WEIHN have grown rapidly in the last decade, limited attention has been given to the infrastructure and human dimensions of WEIHN. Therefore, we emphasize the need for a comprehensive analysis of infrastructure and human dimensions to ensure the efficient and sustainable supply and management of water and energy in the next generation of WEIHN. We then pinpoint the current capabilities, limitations, and future perspectives of infrastructure and human dimensions in WEIHN. In this section, we lay out future opportunities for next-generation WEIHN and cover emerging topics ranging from fundamental scientific exploration to engineering implementations.
5.2 Future perspective and outlook
Water safety and resource recovery.
The advent of next-generation decentralized WEIHN is set to enhance water safety and resource recovery via innovative water transport/supply approaches and treatment technologies. These innovations will enable the prudent production and utilization of water close to the point of use in an energy-efficient manner, with wastewater smoothly reintroduced into the water cycle without excessive energy consumption.251,252 Specifically, solar-powered low-energy water purification/harvesting technologies may obviate the need for complicated device configurations and additional electricity input.253 This offers the possibility of creating highly integrated, portable devices for use in off-grid, remote, decentralized WEIHN settings. For example, solar-driven membrane distillation (MD) with an active volume of ≈ 1 m2 can produce ≈4 L per day under 8 h of sunlight in the summer (700 W m−2) without any form of heat recovery to meet the basic drinking water requirements.184 Moreover, the next-generation WEIHN enables integrated onsite energy generation, nutrient recycling, and water reuse in wastewater treatment facilities to achieve maximal resource recovery. Zero liquid discharge (ZLD), an advanced multi-step water treatment process that involves ultrafiltration,254 reverse osmosis,255 and electrodialysis,256 can be a future solution to both maximizing water recovery in decentralized WEIHN while minimizing the liquid waste from a power generation cycle, resulting in a closed cycle of water treatment and reuse. For instance, ZLD involves multiple mineral precipitation and crystallization steps capable of producing useful salts such as calcium carbonate ($ 350 per ton), which partially compensates for the operation costs of ZLD.257 To further improve the water treatment and energy generation in next-generation WEIHN, reliable sensors with materials innovations (e.g., anti-fouling coating layer) and accurate data processing algorithms can generate data-driven models to predict pollution events and guide contaminant removal processes. For example, multi-sensor fusion techniques based on extended machine learning algorithms (e.g., neural network) can be applied to integrate multiple water parameters (e.g., flow rate, pH, and inlet/outlet contaminant concentrations) at different spatiotemporal resolutions for fault detection and process control in the next generation WEINN.258
Environmental footprints.
Next-generation WEIHN targets assessing water and energy footprint refers to the total freshwater and energy directly and indirectly required to produce a commodity or service.259 Decentralized WEIHN helps reduce CO2 emissions by promoting the use of renewable energy sources such as solar, wind, and hydropower instead of fossil fuels which are the major contributors to carbon emissions.260 Various methods can be employed to measure the carbon footprints of WEIHN. For instance, LCA helps identify areas where carbon emissions and energy consumption can be reduced in different unit processes (e.g., water treatment, pumping, distribution) in WEIHN.261 The close interaction between water and energy in WEIHN also makes it possible to assess virtual water consumption and energy usage by quantitative models. For instance, a fuzzy stochastic multi-objective mixed-integer non-linear optimization model has been used to calculate the water, energy, and carbon footprint to optimize the irrigation rate with an error <5%, possessing the potential to maximum net economic benefit, maximum renewable energy production, minimum water footprint, and minimum carbon footprint in WEIHN.262 In addition, failure detection or diagnosis with efficient methods can be a future solution in next-generation WEIHN. Innovative automatic failure detection techniques such as support vector machine (SVM) can be deployed to assess the condition of infrastructure components.263 With the development of advanced data-driven and physics-driven techniques, failure detection could be realized more accurately and immediately.
Data sharing, human behaviors, and workforce development.
To overcome the long-standing problem of data scarcity in WEIHN, open-source databases should be developed to collect and share data in a standard specification and facilitate calibration and validation of system models. Technologically, advanced methods such as physiological sensors and models can be leveraged to decipher real-world human behaviors and interactions.264 These efforts can underscore the principles of diversity, equity, inclusion, and accessibility (DEIA) in water systems, maximizing data access among all groups involved in WEIHN. For example, physiological sensors and models could be used to explore an individual-level understanding of operators' acceptance of new technologies, or an individual-level understanding of end-users' acceptance of non-potable water reuse.265 Consequently, low-cost user-friendly water quality sensors (e.g., nutrients sensors, heavy metal sensors) can be deployed for real-time water quality monitoring across WEIHN in underdeveloped areas to promptly identify water quality problems and present first-hand data for decision-making.82 In addition, the insights gained from these real-time water-energy data can guide the design of multifaceted interventions such as training workshops to enhance operators' skills or policy initiatives in target households.266 Implementation of renewable energy technologies (e.g., microbial electrochemical systems for hydrogen generation) ensures that the sensor-data-resource in future WEIHN can be reflected in the transition to a sustainable water future.267
From the human perspective, solutions centered on human understanding, development of social norms, and trust-building can be implemented in conjunction with human-sensing techniques. By enhancing individual understanding of resource crisis and inequality of resource availability, end-users are encouraged to save water and energy.268 Developing social norms of appropriate resource use can also reduce waste generation under peer pressure and change human behaviors through social connections.269 In conclusion, the future of WEIHN hinges on the advancement of data science techniques and the adoption of human-centric solutions, fostering a more sustainable and efficient water-energy system, and involving the cultivation of the necessary workforce skills to support the DEIA in water sustainability.
Conflicts of interest
There are no conflicts to declare.
Acknowledgements
This study is supported by the National Science Foundation (NSF) Smart and Connected Communities (SCC) Project (Award No.: ECCS-2018492), NSF Environmental Engineering Program GOALI Project (Grant No.: ENVE-1706343), and the Mini-Grant Initiative at the Department of Civil and Environmental Engineering (CEE) at University of Connecticut.
References
- R. Lal, Feeding 11 billion on 0.5 billion hectare of area under cereal crops, Food Energy Secur., 2016, 5, 239–251 CrossRef.
- N. Vakilifard, M. Anda, P. A. Bahri and G. Ho, The role of water-energy nexus in optimising water supply systems – Review of techniques and approaches, Renewable Sustainable Energy Rev., 2018, 82, 1424–1432 CrossRef.
- J. Dai, S. Wu, G. Han, J. Weinberg, X. Xie, X. Wu, X. Song, B. Jia, W. Xue and Q. Yang, Water-energy nexus: A review of methods and tools for macro-assessment, Appl. Energy, 2018, 210, 393–408 CrossRef.
- S.-Y. Pan, S. W. Snyder, Y. J. Lin and P.-C. Chiang, Electrokinetic desalination of brackish water and associated challenges in the water and energy nexus, Environ. Sci.: Water Res. Technol., 2018, 4, 613–638 RSC.
- J. Machell, K. Prior, R. Allan and J. M. Andresen, The water energy food nexus – challenges and emerging solutions, Environ. Sci.: Water Res. Technol., 2015, 1, 15–16 RSC.
- M. D. Bartos and M. V. Chester, The Conservation Nexus: Valuing Interdependent Water and Energy Savings in Arizona, Environ. Sci. Technol., 2014, 48, 2139–2149 CrossRef CAS PubMed.
- S. Nair, B. George, H. M. Malano, M. Arora and B. Nawarathna, Water–energy–greenhouse gas nexus of urban water systems: Review of concepts, state-of-art and methods, Resour., Conserv. Recycl., 2014, 89, 1–10 CrossRef.
- M. Bartos, B. Wong and B. Kerkez, Open storm: a complete framework for sensing and control of urban watersheds, Environ. Sci.: Water Res. Technol., 2018, 4, 346–358 RSC.
- P. A. Wilderer and D. Schreff, Decentralized and centralized wastewater management: a challenge for technology developers, Water Sci. Technol., 2000, 41, 1–8 CrossRef.
- H. Jeong, O. A. Broesicke, B. Drew, D. Li and J. C. Crittenden, Life cycle assessment of low impact development technologies combined with conventional centralized water systems for the City of Atlanta, Georgia, Front. Environ. Sci. Eng., 2016, 10, 1 CrossRef CAS.
- H. Jeong, E. Minne and J. C. Crittenden, Life cycle assessment of the City of Atlanta, Georgia's centralized water system, Int. J. Life Cycle Assess., 2015, 20, 880–891 CrossRef CAS.
- E. M. Biggs, E. Bruce, B. Boruff, J. M. A. Duncan, J. Horsley, N. Pauli, K. McNeill, A. Neef, F. Van Ogtrop, J. Curnow, B. Haworth, S. Duce and Y. Imanari, Sustainable development and the water–energy–food nexus: A perspective on livelihoods, Environ. Sci. Policy, 2015, 54, 389–397 CrossRef.
- H. Jeong, O. A. Broesicke, B. Drew and J. C. Crittenden, Life cycle assessment of small-scale greywater reclamation systems combined with conventional centralized water systems for the City of Atlanta, Georgia, J. Cleaner Prod., 2018, 174, 333–342 CrossRef.
- M. Peter-Varbanets, C. Zurbrügg, C. Swartz and W. Pronk, Decentralized systems for potable water and the potential of membrane technology, Water Res., 2009, 43, 245–265 CrossRef CAS PubMed.
- M. A. Massoud, A. Tarhini and J. A. Nasr, Decentralized approaches to wastewater treatment and management: Applicability in developing countries, J. Environ. Manage., 2009, 90, 652–659 CrossRef PubMed.
- J. A. C. Castellar, A. Torrens, G. Buttiglieri, H. Monclús, C. A. Arias, P. N. Carvalho, A. Galvao and J. Comas, Nature-based solutions coupled with advanced technologies: An opportunity for decentralized water reuse in cities, J. Cleaner Prod., 2022, 340, 130660 CrossRef.
- Y. Fan, F. Qian, Y. Huang, I. Sifat, C. Zhang, A. Depasquale, L. Wang and B. Li, Miniature microbial fuel cells integrated with triggered power management systems to power wastewater sensors in an uninterrupted mode, Appl. Energy, 2021, 302, 117556 CrossRef CAS.
- S. Eggimann, L. Mutzner, O. Wani, M. Y. Schneider, D. Spuhler, M. Moy De Vitry, P. Beutler and M. Maurer, The Potential of Knowing More: A Review of Data-Driven Urban Water Management, Environ. Sci. Technol., 2017, 51, 2538–2553 CrossRef CAS PubMed.
- A. Hope, T. Roberts and I. Walker, Consumer engagement in low-carbon home energy in the United Kingdom: Implications for future energy system decentralization, Energy Res. Soc. Sci., 2018, 44, 362–370 CrossRef.
- D. M. Byrne, H. A. C. Lohman, S. M. Cook, G. M. Peters and J. S. Guest, Life cycle assessment (LCA) of urban water infrastructure: emerging approaches to balance objectives and inform comprehensive decision-making, Environ. Sci.: Water Res. Technol., 2017, 3, 1002–1014 RSC.
- A. Goldthau, Rethinking the governance of energy infrastructure: Scale, decentralization and polycentrism, Energy Res. Soc. Sci., 2014, 1, 134–140 CrossRef.
- A. Sharifi and Y. Yamagata, Principles and criteria for assessing urban energy resilience: A literature review, Renewable Sustainable Energy Rev., 2016, 60, 1654–1677 CrossRef.
- J. T. Lee and D. S. Callaway, The cost of reliability in decentralized solar power systems in sub-Saharan Africa, Nat. Energy, 2018, 3, 960–968 CrossRef.
- S. Zhou, Y. Yang, S. T. Ng, J. F. Xu and D. Li, Integrating data-driven and physics-based approaches to characterize failures of interdependent infrastructures, Int. J. Crit. Infrastruct. Prot., 2020, 31, 100391 CrossRef.
- G. M. Bethke, A. R. Cohen and A. S. Stillwell, Emerging investigator series: disaggregating residential sector high-resolution smart water meter data into appliance end-uses with unsupervised machine learning, Environ. Sci.: Water Res. Technol., 2021, 7, 487–503 RSC.
- M. M. Mekonnen, P. W. Gerbens-Leenes and A. Y. Hoekstra, The consumptive water footprint of electricity and heat: a global assessment, Environ. Sci.: Water Res. Technol., 2015, 1, 285–297 RSC.
- I. Roefs, B. Meulman, J. H. G. Vreeburg and M. Spiller, Centralised, decentralised or hybrid sanitation systems? Economic evaluation under urban development uncertainty and phased expansion, Water Res., 2017, 109, 274–286 CrossRef CAS PubMed.
- J. M. Clomburg, A. M. Crumbley and R. Gonzalez, Industrial biomanufacturing: The future of chemical production, Science, 2017, 355, aag0804 CrossRef PubMed.
- N. Diaz-Elsayed, N. Rezaei, A. Ndiaye and Q. Zhang, Trends in the environmental and economic sustainability of wastewater-based resource recovery: A review, J. Cleaner Prod., 2020, 265, 121598 CrossRef CAS.
- D. Yue, F. You and S. W. Snyder, Biomass-to-bioenergy and biofuel supply chain optimization: Overview, key issues and challenges, Comput. Chem. Eng., 2014, 66, 36–56 CrossRef CAS.
- B. P. Koirala, E. van Oost and H. van der Windt, Community energy storage: A responsible innovation towards a sustainable energy system?, Appl. Energy, 2018, 231, 570–585 CrossRef.
- A. Pandit, E. A. Minné, F. Li, H. Brown, H. Jeong, J.-A. C. James, J. P. Newell, M. Weissburg, M. E. Chang, M. Xu, P. Yang, R. Wang, V. M. Thomas, X. Yu, Z. Lu and J. C. Crittenden, Infrastructure ecology: an evolving paradigm for sustainable urban development, J. Cleaner Prod., 2017, 163, S19–S27 CrossRef.
- A. Aili, D. Zhao, G. Tan, X. Yin and R. Yang, Reduction of water consumption in thermal power plants with radiative sky cooling, Appl. Energy, 2021, 302, 117515 CrossRef.
- C. Zhang, D. He, J. Ma, W. Tang and T. D. Waite, Faradaic reactions in capacitive deionization (CDI) - problems and possibilities: A review, Water Res., 2018, 128, 314–330 CrossRef CAS PubMed.
- X. Zhang, K. Zuo, X. Zhang, C. Zhang and P. Liang, Selective ion separation by capacitive deionization (CDI) based technologies: a state-of-the-art review, Environ. Sci.: Water Res. Technol., 2020, 6, 243–257 RSC.
- J. Chang, F. Duan, C. Su, Y. Li and H. Cao, Removal of chloride ions using a bismuth electrode in capacitive deionization (CDI), Environ. Sci.: Water Res. Technol., 2020, 6, 373–382 RSC.
- J. Brame, Q. Li and P. J. J. Alvarez, Nanotechnology-enabled water treatment and reuse: emerging opportunities and challenges for developing countries, Trends Food Sci. Technol., 2011, 22, 618–624 CrossRef CAS.
- C. Kim, P. Srimuk, J. Lee, M. Aslan and V. Presser, Semi-continuous capacitive deionization using multi-channel flow stream and ion exchange membranes, Desalination, 2018, 425, 104–110 CrossRef CAS.
- Z. Li, B. Song, Z. Wu, Z. Lin, Y. Yao, K.-S. Moon and C. P. Wong, 3D porous graphene with ultrahigh surface area for microscale capacitive deionization, Nano Energy, 2015, 11, 711–718 CrossRef CAS.
- Y. Liu, C. Nie, X. Liu, X. Xu, Z. Sun and L. Pan, Review on carbon-based composite materials for capacitive deionization, RSC Adv., 2015, 5, 15205–15225 RSC.
- H. Li, L. Zou, L. Pan and Z. Sun, Novel Graphene-Like Electrodes for Capacitive Deionization, Environ. Sci. Technol., 2010, 44, 8692–8697 CrossRef CAS PubMed.
- R. Chen, T. Sheehan, J. Lian Ng, M. Brucks and X. Su, Capacitive deionization and electrosorption for heavy metal removal, Environ. Sci.: Water Res. Technol., 2020, 6, 258–282 RSC.
- P. U. Iyare, S. K. Ouki and T. Bond, Microplastics removal in wastewater treatment plants: a critical review, Environ. Sci.: Water Res. Technol., 2020, 6, 2664–2675 RSC.
- F. Dixit, B. Barbeau, S. Ghavam Mostafavi and M. Mohseni, PFOA and PFOS removal by ion exchange for water reuse and drinking applications: role of organic matter characteristics, Environ. Sci.: Water Res. Technol., 2019, 5, 1782–1795 RSC.
- J. R. McCutcheon and M. S. Mauter, Fixing the desalination membrane pipeline, Science, 2023, 380, 242–244 CrossRef CAS PubMed.
- M. Khalkhali, B. Dilkina and W. Mo, The role of climate change and decentralization in urban water services: A dynamic energy-water nexus analysis, Water Res., 2021, 207, 117830 CrossRef CAS PubMed.
- D. D. Guta, J. Jara, N. P. Adhikari, Q. Chen, V. Gaur and A. Mirzabaev, Assessment of the Successes and Failures of Decentralized Energy Solutions and Implications for the Water–Energy–Food Security Nexus: Case Studies from Developing Countries, Resources, 2017, 6, 24 CrossRef.
- Y. B. Meir, K. Opfer and E. Hernandez, Decentralized renewable energies and the water-energy-food nexus in rural Morocco, Environ. Challenges, 2022, 6, 100432 CrossRef CAS.
- E. Andrés García, M. Agulló-Barceló, P. Bond, J. Keller, W. Gernjak and J. Radjenovic, Hybrid electrochemical-granular activated carbon system for the treatment of greywater, Chem. Eng. J., 2018, 352, 405–411 CrossRef.
- F. H. Lakho, H. Q. Le, F. Mattheeuws, W. Igodt, V. Depuydt, J. Desloover, D. P. L. Rousseau and S. W. H. Van Hulle, Decentralized grey and black water reuse by combining a vertical flow constructed wetland and membrane based potable water system: Full scale demonstration, J. Environ. Chem. Eng., 2021, 9, 104688 CrossRef CAS.
- K. Rabaey, T. Vandekerckhove, A. V. de Walle and D. L. Sedlak, The third route: Using extreme decentralization to create resilient urban water systems, Water Res., 2020, 185, 116276 CrossRef CAS PubMed.
- X. Guo, Z. Liu, M. Chen, J. Liu and M. Yang, Decentralized wastewater treatment technologies and management in Chinese villages, Front. Environ. Sci. Eng., 2014, 8, 929–936 CrossRef.
- J. Keller, Why are decentralised urban water solutions still rare given all the claimed benefits, and how could that be changed?, Water Res.: X, 2023, 19, 100180 CAS.
- K. Orehounig, R. Evins and V. Dorer, Integration of decentralized energy systems in neighbourhoods using the energy hub approach, Appl. Energy, 2015, 154, 277–289 CrossRef.
- M. H. M. Ashnani, A. Johari, H. Hashim and E. Hasani, A source of renewable energy in Malaysia, why biodiesel?, Renewable Sustainable Energy Rev., 2014, 35, 244–257 CrossRef.
- Z. Ge and Z. He, Long-term performance of a 200 liter modularized microbial fuel cell system treating municipal wastewater: treatment, energy, and cost, Environ. Sci.: Water Res. Technol., 2016, 2, 274–281 RSC.
- M. Elimelech and W. A. Phillip, The Future of Seawater Desalination: Energy, Technology, and the Environment, Science, 2011, 333, 712–717 CrossRef CAS PubMed.
- A. Ali, R. A. Tufa, F. Macedonio, E. Curcio and E. Drioli, Membrane technology in renewable-energy-driven desalination, Renewable Sustainable Energy Rev., 2018, 81, 1–21 CrossRef CAS.
- C. Pahl-Wostl, P. Gorris, N. Jager, L. Koch, L. Lebel, C. Stein, S. Venghaus and S. Withanachchi, Scale-related governance challenges in the water–energy–food nexus: toward a diagnostic approach, Sustain. Sci., 2021, 16, 615–629 CrossRef.
- H. D. Teklehaimanot and A. Teklehaimanot, Human resource development for a community-based health extension program: a case study from Ethiopia, Hum. Resour. Health, 2013, 11, 39 CrossRef PubMed.
- M. Garrido-Baserba, I. Barnosell, M. Molinos-Senante, D. L. Sedlak, K. Rabaey, O. Schraa, M. Verdaguer, D. Rosso and M. Poch, The third route: A techno-economic evaluation of extreme water and wastewater decentralization, Water Res., 2022, 218, 118408 CrossRef CAS PubMed.
- X. Wen, J. Lu, J. Wu, Y. Lin and Y. Luo, Influence of coastal groundwater salinization on the distribution and risks of heavy metals, Sci. Total Environ., 2019, 652, 267–277 CrossRef PubMed.
- M. Wilder and P. Romero Lankao, Paradoxes of Decentralization: Water Reform and Social Implications in Mexico, World Dev., 2006, 34, 1977–1995 CrossRef.
- M. Kumar, Non-universal nature of energy poverty: Energy services, assessment of needs and consumption evidences from rural Himachal Pradesh, Energy Policy, 2020, 138, 111235 CrossRef.
- M. Yaqoot, P. Diwan and T. C. Kandpal, Review of barriers to the dissemination of decentralized renewable energy systems, Renewable Sustainable Energy Rev., 2016, 58, 477–490 CrossRef.
- P. Alstone, D. Gershenson and D. M. Kammen, Decentralized energy systems for clean electricity access, Nat. Clim. Change, 2015, 5, 305–314 CrossRef.
- C. A. de Melo, G. de M. Jannuzzi and S. V. Bajay, Nonconventional renewable energy governance in Brazil: Lessons to learn from the German experience, Renewable Sustainable Energy Rev., 2016, 61, 222–234 CrossRef.
- Q. Liu, L. Yang and M. Yang, Digitalisation for Water Sustainability: Barriers to Implementing Circular Economy in Smart Water Management, Sustainability, 2021, 13, 11868 CrossRef.
- M. Haji, R. Govindan and T. Al-Ansari, A computational modelling approach based on the ‘Energy - Water - Food nexus node’ to support decision-making for sustainable and resilient food security, Comput. Chem. Eng., 2022, 163, 107846 CrossRef CAS.
- D. Zheng, Z. An, C. Yan and R. Wu, Spatial-temporal characteristics and influencing factors of food production efficiency based on WEF nexus in China, J. Cleaner Prod., 2022, 330, 129921 CrossRef.
- S. M. Mahdavian, M. Ahmadpour Borazjani, H. Mohammadi, M. R. Asgharipour and H. Najafi Alamdarlo, Assessment of food-energy-environmental pollution nexus in Iran: the nonlinear approach, Environ. Sci. Pollut. Res., 2022, 29, 52457–52472 CrossRef PubMed.
- A. R. McFarland, L. Larsen, K. Yeshitela, A. Nigussie Engida and N. G. Love, Guide for using green infrastructure in urban environments for stormwater management, Environ. Sci.: Water Res. Technol., 2019, 5, 643–659 RSC.
- Y. Fan, Z. Xu, Y. Huang, T. Wang, S. Zheng, A. DePasquale, C. Brüeckner, Y. Lei and B. Li, Long-term continuous and real-time in situ monitoring of Pb(II) toxic contaminants in wastewater using solid-state ion selective membrane (S-ISM) Pb and pH auto-correction assembly, J. Hazard. Mater., 2020, 400, 109891 CrossRef PubMed.
- Y. Huang, F. MahmoodPoor Dehkordy, Y. Li, S. Emadi, A. Bagtzoglou and B. Li, Enhancing anaerobic fermentation performance through eccentrically stirred mixing: Experimental and modeling methodology, Chem. Eng. J., 2018, 334, 1383–1391 CrossRef CAS.
- B. Højris, S. C. B. Christensen, H.-J. Albrechtsen, C. Smith and M. Dahlqvist, A novel, optical, on-line bacteria sensor for monitoring drinking water quality, Sci. Rep., 2016, 6, 23935 CrossRef PubMed.
- V. Hidalgo-Ruz, L. Gutow, R. C. Thompson and M. Thiel, Microplastics in the Marine Environment: A Review of the Methods Used for Identification and Quantification, Environ. Sci. Technol., 2012, 46, 3060–3075 CrossRef CAS PubMed.
- Y. Yang, Y. S. Ok, K.-H. Kim, E. E. Kwon and Y. F. Tsang, Occurrences and removal of pharmaceuticals and personal care products (PPCPs) in drinking water and water/sewage treatment plants: A review, Sci. Total Environ., 2017, 596–597, 303–320 CrossRef CAS PubMed.
- W. Zhu, C. Duan and B. Chen, Energy-pollutant nexus for wastewater treatment in China based on multi-regional input-output analysis, J. Cleaner Prod., 2022, 363, 132490 CrossRef CAS.
- K. B. Newhart, R. W. Holloway, A. S. Hering and T. Y. Cath, Data-driven performance analyses of wastewater treatment plants: A review, Water Res., 2019, 157, 498–513 CrossRef CAS PubMed.
- W. Zhang, N. B. Tooker and A. V. Mueller, Enabling wastewater treatment process automation: Leveraging innovations in real-time sensing, data analysis, and online controls, Environ. Sci.: Water Res. Technol., 2020, 6, 2973–2992 RSC.
- P.-C. Chen, V. Alvarado and S.-C. Hsu, Water energy nexus in city and hinterlands: Multi-regional physical input-output analysis for Hong Kong and South China, Appl. Energy, 2018, 225, 986–997 CrossRef.
- Y. Huang, X. Wang, W. Xiang, T. Wang, C. Otis, L. Sarge, Y. Lei and B. Li, Forward-Looking Roadmaps for Long-Term Continuous Water Quality Monitoring: Bottlenecks, Innovations, and Prospects in a Critical Review, Environ. Sci. Technol., 2022, 56, 5334–5354 CrossRef CAS PubMed.
- X. Wang, Y. Fan, Y. Huang, J. Ling, A. Klimowicz, G. Pagano and B. Li, Solving Sensor Reading Drifting Using Denoising Data Processing Algorithm (DDPA) for Long-Term Continuous and Accurate Monitoring of Ammonium in Wastewater, ACS EST Water, 2021, 1, 530–541 CrossRef CAS.
- Y. Huang, X. Qian, X. Wang, T. Wang, S. J. Lounder, T. Ravindran, Z. Demitrack, J. McCutcheon, A. Asatekin and B. Li, Electrospraying Zwitterionic Copolymers as an Effective Biofouling Control for Accurate and Continuous Monitoring of Wastewater Dynamics in a Real-Time and Long-Term Manner, Environ. Sci. Technol., 2022, 56, 8176–8186 CrossRef CAS PubMed.
- H. Ryu, D. Thompson, Y. Huang, B. Li and Y. Lei, Electrochemical sensors for nitrogen species: A review, Sens. Actuators Rep., 2020, 2, 100022 CrossRef.
- V. C. Gungor and F. C. Lambert, A survey on communication networks for electric system automation, Comput. Netw., 2006, 50, 877–897 CrossRef.
- T. Rault, A. Bouabdallah and Y. Challal, Energy efficiency in wireless sensor networks: A top-down survey, Comput. Netw., 2014, 67, 104–122 CrossRef.
- D. Chen, Z. Liu, L. Wang, M. Dou, J. Chen and H. Li, Natural Disaster Monitoring with Wireless Sensor Networks: A Case Study of Data-intensive Applications upon Low-Cost Scalable Systems, Mob. Netw. Appl., 2013, 18, 651–663 CrossRef.
- M. Bekchanov and J. P. A. Lamers, The effect of energy constraints on water allocation decisions: The elaboration and application of a system-wide economic-water-energy model (SEWEM), Water, 2019, 8(6), 253 CrossRef.
- M.-H. Yuan and S.-L. Lo, Principles of food-energy-water nexus governance, Renewable Sustainable Energy Rev., 2022, 155, 111937 CrossRef.
- S. Radini, E. Marinelli, Ç. Akyol, A. L. Eusebi, V. Vasilaki, A. Mancini, E. Frontoni, G. B. Bischetti, C. Gandolfi, E. Katsou and F. Fatone, Urban water-energy-food-climate nexus in integrated wastewater and reuse systems: Cyber-physical framework and innovations, Appl. Energy, 2021, 298, 117268 CrossRef.
- W. J. Cosgrove and D. P. Loucks, Water management: Current and future challenges and research directions, Water Resour. Res., 2015, 51, 4823–4839 CrossRef.
- X.-C. Wang, P. Jiang, L. Yang, Y. V. Fan, J. J. Klemeš and Y. Wang, Extended water-energy nexus contribution to environmentally-related sustainable development goals, Renewable Sustainable Energy Rev., 2021, 150, 111485 CrossRef.
- A. T. Hoang, V. V. Pham and X. P. Nguyen, Integrating renewable sources into energy system for smart city as a sagacious strategy towards clean and sustainable process, J. Cleaner Prod., 2021, 305, 127161 CrossRef.
- G. C. de Oliveira, E. Bertone and R. A. Stewart, Challenges, opportunities, and strategies for undertaking integrated precinct-scale energy–water system planning, Renewable Sustainable Energy Rev., 2022, 161, 112297 CrossRef.
- K. J. Ding, J. M. Gilligan, Y. C. E. Yang, P. Wolski and G. M. Hornberger, Assessing food–energy–water resources management strategies at city scale: An agent-based modeling approach for Cape Town, South Africa, Resour., Conserv. Recycl., 2021, 170, 105573 CrossRef.
- E. Bertone, O. Sahin, R. A. Stewart, P. X. W. Zou, M. Alam, K. Hampson and E. Blair, Role of financial mechanisms for accelerating the rate of water and energy efficiency retrofits in Australian public buildings: Hybrid Bayesian Network and System Dynamics modelling approach, Appl. Energy, 2018, 210, 409–419 CrossRef.
- B. T. Daher and R. H. Mohtar, Water–energy–food (WEF) Nexus Tool 2.0: guiding integrative resource planning and decision-making, Water Int., 2015, 40, 748–771 CrossRef.
- E. P. Ramos, M. Howells, V. Sridharan, R. E. Engström, C. Taliotis, D. Mentis, F. Gardumi, L. de Strasser, I. Pappis, G. P. Balderrama, Y. Almulla, A. Beltramo, C. Ramirez, C. Sundin, T. Alfstad, A. Lipponen, E. Zepeda, T. Niet, J. Quirós-Tortós, J. Angulo-Paniagua, A. Shivakumar, S. Ulloa and H. Rogner, The climate, land, energy, and water systems (CLEWs) framework: A retrospective of activities and advances to 2019, Environ. Res. Lett., 2021, 16, 033003 Search PubMed.
- T. Serrano-Tovar, B. Peñate Suárez, A. Musicki, J. A. de la Fuente Bencomo, V. Cabello and M. Giampietro, Structuring an integrated water-energy-food nexus assessment of a local wind energy desalination system for irrigation, Sci. Total Environ., 2019, 689, 945–957 CrossRef CAS PubMed.
- N. H. Malik, F. Shaikh, L. Kumar and M. S. Hossain, Quantification of the water-energy-carbon nexus of the coal fired powerplant in water stressed area of Pakistan, Energy Sources, Part A, 2020, 00, 1–23 Search PubMed.
- Q. Su, H. Dai, Y. Lin, H. Chen and R. Karthikeyan, Modeling the carbon-energy-water nexus in a rapidly urbanizing catchment: A general equilibrium assessment, J. Environ. Manage., 2018, 225, 93–103 CrossRef CAS PubMed.
- S. Wang and B. Chen, Energy–water nexus of urban agglomeration based on multiregional input–output tables and ecological network analysis: A case study of the Beijing–Tianjin–Hebei region, Appl. Energy, 2016, 178, 773–783 CrossRef.
- C. K. Makropoulos, K. Natsis, S. Liu, K. Mittas and D. Butler, Decision support for sustainable option selection in integrated urban water management, Environ. Model. Softw., 2008, 23, 1448–1460 CrossRef.
- Y. Zhou, H. Li, K. Wang and J. Bi, China's energy-water nexus: Spillover effects of energy and water policy, Glob. Environ. Change, 2016, 40, 92–100 CrossRef.
- A. Wang, Y. Pei, Z. Qian, H. Zareipour, B. Jing and J. An, A two-stage anomaly decomposition scheme based on multi-variable correlation extraction for wind turbine fault detection and identification, Appl. Energy, 2022, 321, 119373 CrossRef.
- H. G. Shakouri and A. Kazemi, Multi-objective cost-load optimization for demand side management of a residential area in smart grids, Sustain. Cities Soc., 2017, 32, 171–180 CrossRef.
- T. Pacetti, L. Lombardi and G. Federici, Water-energy Nexus: A case of biogas production from energy crops evaluated by Water Footprint and Life Cycle Assessment (LCA) methods, J. Cleaner Prod., 2015, 101, 278–291 CrossRef.
- S. O. Muhanji and A. M. Farid, An enterprise control methodology for the techno-economic assessment of the energy water nexus, Appl. Energy, 2020, 260, 114274 CrossRef.
- Y. Li, J. T. Trimmer, S. Hand, X. Zhang, K. G. Chambers, H. A. C. Lohman, R. Shi, D. M. Byrne, S. M. Cook and J. S. Guest, Quantitative sustainable design (QSD) for the prioritization of research, development, and deployment of technologies: a tutorial and review, Environ. Sci.: Water Res. Technol., 2022, 8, 2439–2465 RSC.
- M. Sapkota, M. Arora, H. Malano, M. Moglia, A. Sharma, B. George and F. Pamminger, An overview of hybrid water supply systems in the context of urban water management: Challenges and opportunities, Water, 2015, 7, 153–174 CrossRef CAS.
- C. M. Chew, M. K. Aroua and M. A. Hussain, Advanced process control for ultrafiltration membrane water treatment system, J. Cleaner Prod., 2018, 179, 63–80 CrossRef CAS.
- P. Radanliev, D. C. De Roure, R. Nicolescu, M. Huth, R. M. Montalvo, S. Cannady and P. Burnap, Future developments in cyber risk assessment for the internet of things, Comput. Ind., 2018, 102, 14–22 CrossRef.
- M. Kadadha, H. Otrok, R. Mizouni, S. Singh and A. Ouali, SenseChain: A blockchain-based crowdsensing framework for multiple requesters and multiple workers, Future Gener. Comput. Syst., 2020, 105, 650–664 CrossRef.
- A. Faltynkova and M. Wagner, Developing and testing a workflow to identify microplastics using near infrared hyperspectral imaging, Chemosphere, 2023, 336, 139186 CrossRef CAS PubMed.
- G. Stergiopoulos, P. Kotzanikolaou, M. Theocharidou, G. Lykou and D. Gritzalis, Time-based critical infrastructure dependency analysis for large-scale and cross-sectoral failures, Int. J. Crit. Infrastruct. Prot., 2016, 12, 46–60 CrossRef.
- W. Liu and Z. Song, Review of studies on the resilience of urban critical infrastructure networks, Reliab. Eng. Syst. Saf., 2020, 193, 106617 CrossRef.
- A. Abedi, L. Gaudard and F. Romerio, Review of major approaches to analyze vulnerability in power system, Reliab. Eng. Syst. Saf., 2019, 183, 153–172 CrossRef.
-
B. M. Frischmann, Infrastructure: The Social Value of Shared Resources, Oxford University Press, 2012 Search PubMed.
- B. McKuin, A. Zumkehr, J. Ta, R. Bales, J. H. Viers, T. Pathak and J. E. Campbell, Energy and water co-benefits from covering canals with solar panels, Nat. Sustain., 2021, 4, 609–617 CrossRef.
- N. S. Grigg, Global water infrastructure: state of the art review, Int. J. Water Resour. Dev., 2019, 35, 181–205 CrossRef.
- W. Mo and Q. Zhang, Energy–nutrients–water nexus: Integrated resource recovery in municipal wastewater treatment plants, J. Environ. Manage., 2013, 127, 255–267 CrossRef CAS PubMed.
- M. Z. Jacobson and M. A. Delucchi, Providing all global energy with wind, water, and solar power, Part I: Technologies, energy resources, quantities and areas of infrastructure, and materials, Energy Policy, 2011, 39, 1154–1169 CrossRef CAS.
- M. Wolsink, The research agenda on social acceptance of distributed generation in smart grids: Renewable as common pool resources, Renewable Sustainable Energy Rev., 2012, 16, 822–835 CrossRef.
- J.-A. James, S. Sung, H. Jeong, O. A. Broesicke, S. P. French, D. Li and J. C. Crittenden, Impacts of Combined Cooling, Heating and Power Systems, and Rainwater Harvesting on Water Demand, Carbon Dioxide, and NOx Emissions for Atlanta, Environ. Sci. Technol., 2018, 52, 3–10 CrossRef CAS PubMed.
- S. Eggimann, B. Truffer and M. Maurer, Economies of density for on-site waste water treatment, Water Res., 2016, 101, 476–489 CrossRef CAS PubMed.
- S.-L. Wu, H. Chen, H.-L. Wang, X. Chen, H.-C. Yang and S. B. Darling, Solar-driven evaporators for water treatment: challenges and opportunities, Environ. Sci.: Water Res. Technol., 2021, 7, 24–39 RSC.
- W. Apichonnabutr and A. Tiwary, Trade-offs between economic and environmental performance of an autonomous hybrid energy system using micro hydro, Appl. Energy, 2018, 226, 891–904 CrossRef.
- K. Solon, E. I. Volcke, M. Spérandio and M. C. van Loosdrecht, Resource recovery and wastewater treatment modelling, Environ. Sci.: Water Res. Technol., 2019, 5, 631–642 RSC.
- H. Wang, C. R. Proctor, M. A. Edwards, M. Pryor, J. W. Santo Domingo, H. Ryu, A. K. Camper, A. Olson and A. Pruden, Microbial Community Response to Chlorine Conversion in a Chloraminated Drinking Water Distribution System, Environ. Sci. Technol., 2014, 48, 10624–10633 CrossRef CAS PubMed.
- F. Moazeni and J. Khazaei, Optimal operation of water-energy microgrids; a mixed integer linear programming formulation, J. Cleaner Prod., 2020, 275, 122776 CrossRef.
- G. Zhang, H. Zhong, Z. Tan, T. Cheng, Q. Xia and C. Kang, Texas electric power crisis of 2021 warns of a new blackout mechanism, CSEE J. Power Energy Syst., 2022, 8, 1–9 Search PubMed.
- S. Shrestha, V. Panchalogaranjan and P. Moses, The February 2021 U.S. Southwest power crisis, Electr. Power Syst. Res., 2023, 217, 109124 CrossRef.
- G. Fu, S. Wilkinson, R. J. Dawson, H. J. Fowler, C. Kilsby, M. Panteli and P. Mancarella, Integrated Approach to Assess the Resilience of Future Electricity Infrastructure Networks to Climate Hazards, IEEE Syst. J., 2018, 12, 3169–3180 Search PubMed.
- W. Sun, P. Bocchini and B. D. Davison, Overview of Interdependency Models of Critical Infrastructure for Resilience Assessment, Nat. Hazards Rev., 2022, 23(1), 04021058 CrossRef.
- S. M. Rinaldi, J. P. Peerenboom and T. K. Kelly, Identifying, understanding, and analyzing critical infrastructure interdependencies, IEEE Control Syst. Mag., 2001, 21, 11–25 Search PubMed.
- A. F. M. K. Chowdhury, T. D. Dang, A. Bagchi and S. Galelli, Expected Benefits of Laos' Hydropower Development Curbed by Hydroclimatic Variability and Limited Transmission Capacity: Opportunities to Reform, J. Water Resour. Plan. Manag., 2020, 146, 05020019 CrossRef.
- W. Zhang, A. Valencia, L. Gu, Q. P. Zheng and N.-B. Chang, Integrating emerging and existing renewable energy technologies into a community-scale microgrid in an energy-water nexus for resilience improvement, Appl. Energy, 2020, 279, 115716 CrossRef.
- E. Hossain, S. Roy, N. Mohammad, N. Nawar and D. R. Dipta, Metrics and enhancement strategies for grid resilience and reliability during natural disasters, Appl. Energy, 2021, 290, 116709 CrossRef.
- A. Alassi, S. Bañales, O. Ellabban, G. Adam and C. MacIver, HVDC Transmission: Technology Review, Market Trends and Future Outlook, Renewable Sustainable Energy Rev., 2019, 112, 530–554 CrossRef.
- S. Khennas, Understanding the political economy and key drivers of energy access in addressing national energy access priorities and policies: African Perspective, Energy Policy, 2012, 47, 21–26 CrossRef.
- M. Yue, H. Lambert, E. Pahon, R. Roche, S. Jemei and D. Hissel, Hydrogen energy systems: A critical review of technologies, applications, trends and challenges, Renewable Sustainable Energy Rev., 2021, 146, 111180 CrossRef.
- S. Geetha and S. Gouthami, Internet of things enabled real time water quality monitoring system, Smart Water, 2017, 2, 1 CrossRef.
- S. Mohebbi, Q. Zhang, E. Christian Wells, T. Zhao, H. Nguyen, M. Li, N. Abdel-Mottaleb, S. Uddin, Q. Lu, M. J. Wakhungu, Z. Wu, Y. Zhang, A. Tuladhar and X. Ou, Cyber-physical-social interdependencies and organizational resilience: A review of water, transportation, and cyber infrastructure systems and processes, Sustain. Cities Soc., 2020, 62, 102327 CrossRef.
- C. Tavera-Ruiz, J. Martí-Herrero, O. Mendieta, J. Jaimes-Estévez, P. Gauthier-Maradei, U. Azimov, H. Escalante and L. Castro, Current understanding and perspectives on anaerobic digestion in developing countries: Colombia case study, Renewable Sustainable Energy Rev., 2023, 173, 113097 CrossRef CAS.
- S. Ahmad, H. Jia, Z. Chen, Q. Li and C. Xu, Water-energy nexus and energy efficiency: A systematic analysis of urban water systems, Renewable Sustainable Energy Rev., 2020, 134, 110381 CrossRef.
- X. Huang, X. Luo, J. Chen, Z. Yang, Y. Chen, J. María Ponce-Ortega and M. M. El-Halwagi, Synthesis and dual-objective optimization of industrial combined heat and power plants compromising the water-energy nexus, Appl. Energy, 2018, 224, 448–468 CrossRef.
- E. S. Spang, W. R. Moomaw, K. S. Gallagher, P. H. Kirshen and D. H. Marks, The water consumption of energy production: An international comparison, Environ. Res. Lett., 2014, 9, 105002 CrossRef.
- S. Lattemann and T. Höpner, Environmental impact and impact assessment of seawater desalination, Desalination, 2008, 220, 1–15 CrossRef CAS.
- S. Kriaa, L. Pietre-Cambacedes, M. Bouissou and Y. Halgand, A survey of approaches combining safety and security for industrial control systems, Reliab. Eng. Syst. Saf., 2015, 139, 156–178 CrossRef.
- O. Shumilova, K. Tockner, A. Sukhodolov, V. Khilchevskyi, L. De Meester, S. Stepanenko, G. Trokhymenko, J. A. Hernández-Agüero and P. Gleick, Impact of the Russia–Ukraine armed conflict on water resources and water infrastructure, Nat. Sustain., 2023, 6, 578–586 CrossRef.
- S. M. H. Ali, M. Lenzen, F. Sack and M. Yousefzadeh, Electricity generation and demand flexibility in wastewater treatment plants: Benefits for 100% renewable electricity grids, Appl. Energy, 2020, 268, 114960 CrossRef.
- M. Khalkhali, B. Dilkina and W. Mo, The role of climate change and decentralization in urban water services: A dynamic energy-water nexus analysis, Water Res., 2021, 207, 117830 CrossRef CAS PubMed.
- Y. T. Jung, N. C. Narayanan and Y. L. Cheng, Cost comparison of centralized and decentralized wastewater management systems using optimization model, J. Environ. Manage., 2018, 213, 90–97 CrossRef PubMed.
- T. D. Fletcher, H. Andrieu and P. Hamel, Understanding, management and modelling of urban hydrology and its consequences for receiving waters: A state of the art, Adv. Water Resour., 2013, 51, 261–279 CrossRef.
- H. Ibrahim, A. Ilinca and J. Perron, Energy storage systems—Characteristics and comparisons, Renewable Sustainable Energy Rev., 2008, 12, 1221–1250 CrossRef CAS.
- D. T. Ingersoll, Z. J. Houghton, R. Bromm and C. Desportes, NuScale small modular reactor for Co-generation of electricity and water, Desalination, 2014, 340, 84–93 CrossRef CAS.
- Z. M. Yaseen, S. O. Sulaiman, R. C. Deo and K.-W. Chau, An enhanced extreme learning machine model for river flow forecasting: State-of-the-art, practical applications in water resource engineering area and future research direction, J. Hydrol., 2019, 569, 387–408 CrossRef.
- J. E. Pesantez, F. Alghamdi, S. Sabu, G. Mahinthakumar and E. Z. Berglund, Using a digital twin to explore water infrastructure impacts during the COVID-19 pandemic, Sustain. Cities Soc., 2022, 77, 103520 CrossRef PubMed.
- Z. Xu, F. MahmoodPoor Dehkordy, Y. Li, Y. Fan, T. Wang, Y. Huang, W. Zhou, Q. Dong, Y. Lei, M. D. Stuber, A. Bagtzoglou and B. Li, High-fidelity profiling and modeling of heterogeneity in wastewater systems using milli-electrode array (MEA): Toward high-efficiency and energy-saving operation, Water Res., 2019, 165, 114971 CrossRef CAS PubMed.
- C. Mu, T. Ding, M. Qu, Q. Zhou, F. Li and M. Shahidehpour, Decentralized optimization operation for the multiple integrated energy systems with energy cascade utilization, Appl. Energy, 2020, 280, 115989 CrossRef.
- R. Ali, I. Daut and S. Taib, A review on existing and future energy sources for electrical power generation in Malaysia, Renewable Sustainable Energy Rev., 2012, 16, 4047–4055 CrossRef.
- S. Gawusu, X. Zhang, A. Ahmed, S. A. Jamatutu, E. D. Miensah, A. A. Amadu and F. A. J. Osei, Renewable energy sources from the perspective of blockchain integration: From theory to application, Sustain. Energy Technol. Assess., 2022, 52, 102108 Search PubMed.
- D. C. Matisoff, R. Beppler, G. Chan and S. Carley, A review of barriers in implementing dynamic electricity pricing to achieve cost-causality, Environ. Res. Lett., 2020, 15, 093006 CrossRef.
- M. Gopalakrishnan, M. Subramaniyan and A. Skoogh, Data-driven machine criticality assessment – maintenance decision support for increased productivity, Prod. Plan. Control, 2022, 33, 1–19 CrossRef.
- S. Noor, W. Yang, M. Guo, K. H. van Dam and X. Wang, Energy Demand Side Management within micro-grid networks enhanced by blockchain, Appl. Energy, 2018, 228, 1385–1398 CrossRef.
- D. Jones, C. Snider, A. Nassehi, J. Yon and B. Hicks, Characterising the Digital Twin: A systematic literature review, CIRP J. Manuf. Sci. Technol., 2020, 29, 36–52 CrossRef.
- S. B. Henderson, B. Beckerman, M. Jerrett and M. Brauer, Application of Land Use Regression to Estimate Long-Term Concentrations of Traffic-Related Nitrogen Oxides and Fine Particulate Matter, Environ. Sci. Technol., 2007, 41, 2422–2428 CrossRef CAS PubMed.
- A. Diez-Olivan, J. Del Ser, D. Galar and B. Sierra, Data fusion and machine learning for industrial prognosis: Trends and perspectives towards Industry 4.0, Inf. Fusion, 2019, 50, 92–111 CrossRef.
- C. Crosson, A. Achilli, A. A. Zuniga-Teran, E. A. Mack, T. Albrecht, P. Shrestha, D. L. Boccelli, T. Y. Cath, G. T. Daigger, J. Duan, K. E. Lansey, T. Meixner, S. Pincetl and C. A. Scott, Net Zero Urban Water from Concept to Applications: Integrating Natural, Built, and Social Systems for Responsive and Adaptive Solutions, ACS EST Water, 2021, 1, 518–529 CrossRef CAS.
- C. Cascone, K. R. Murphy, H. Markensten, J. S. Kern, C. Schleich, A. Keucken and S. J. Köhler, AbspectroscoPY, a Python toolbox for absorbance-based sensor data in water quality monitoring, Environ. Sci.: Water Res. Technol., 2022, 8, 836–848 RSC.
- S. Zhong, K. Zhang, M. Bagheri, J. G. Burken, A. Gu, B. Li, X. Ma, B. L. Marrone, Z. J. Ren, J. Schrier, W. Shi, H. Tan, T. Wang, X. Wang, B. M. Wong, X. Xiao, X. Yu, J.-J. Zhu and H. Zhang, Machine Learning: New Ideas and Tools in Environmental Science and Engineering, Environ. Sci. Technol., 2021, 55, 12741–12754 CAS.
- S. Ayvaz and K. Alpay, Predictive maintenance system for production lines in manufacturing: A machine learning approach using IoT data in real-time, Expert Syst. Appl., 2021, 173, 114598 CrossRef.
- J. C. P. Cheng, W. Chen, K. Chen and Q. Wang, Data-driven predictive maintenance planning framework for MEP components based on BIM and IoT using machine learning algorithms, Autom. Constr., 2020, 112, 103087 CrossRef.
- A. Bhide and C. R. Monroy, Energy poverty: A special focus on energy poverty in India and renewable energy technologies, Renewable Sustainable Energy Rev., 2011, 15, 1057–1066 CrossRef.
- A. Alvez, D. Aitken, D. Rivera, M. Vergara, N. McIntyre and F. Concha, At the crossroads: can desalination be a suitable public policy solution to address water scarcity in Chile's mining zones?, J. Environ. Manage., 2020, 258, 110039 CrossRef PubMed.
- N. R. Magliocca, Agent-based modeling for integrating human behavior into the food–energy–water nexus, Land, 2020, 9, 1–25 CrossRef.
- M. Sharmina, D. Abi Ghanem, A. L. Browne, S. M. Hall, J. Mylan, S. Petrova and R. Wood, Envisioning surprises: How social sciences could help models represent ‘deep uncertainty’ in future energy and water demand, Energy Res. Soc. Sci., 2019, 50, 18–28 CrossRef.
- H. Yoon and D. Sauri, ‘No more thirst, cold, or darkness!’–Social movements, households, and the coproduction of knowledge on water and energy vulnerability in Barcelona, Spain, Energy Res. Soc. Sci., 2019, 58, 101276 CrossRef.
- K. E. Gibson, A. R. Fortner, A. J. Lamm and L. A. Warner, Managing Demand-Side Water Conservation in the United States: An Audience Segmentation Approach, Water, 2021, 13, 2992 CrossRef CAS.
- C. Zhuge, M. Yu, C. Wang, Y. Cui and Y. Liu, An agent-based spatiotemporal integrated approach to simulating in-home water and related energy use behaviour: A test case of Beijing, China, Sci. Total Environ., 2020, 708, 135086 CrossRef CAS PubMed.
- J. Helmbrecht, J. Pastor and C. Moya, Smart solution to improve water-energy nexus for water supply systems, Procedia Eng., 2017, 186, 101–109 CrossRef.
- E. Wanjiru and X. Xia, Optimal energy-water management in urban residential buildings through grey water recycling, Sustain. Cities Soc., 2017, 32, 654–668 CrossRef.
- W. Mo, Z. Lu, B. Dilkina, K. H. Gardner, J. C. Huang and M. C. Foreman, Sustainable and resilient design of interdependent water and energy systems: A conceptual modeling framework for tackling complexities at the infrastructure-human-resource nexus, Sustainability, 2018, 10, 1–10 CrossRef.
- S. G. Kharanagh, M. E. Banihabib and S. Javadi, An MCDM-based social network analysis of water governance to determine actors' power in water-food-energy nexus, J. Hydrol., 2020, 581, 124382 CrossRef.
- P. Martinez, M. Blanco and B. Castro-Campos, The Water–Energy–Food Nexus: A Fuzzy-Cognitive Mapping Approach to Support Nexus-Compliant Policies in Andalusia (Spain), Water, 2018, 10, 664 CrossRef.
- Y. Teitelbaum, A. Yakirevich, A. Gross and S. Sorek, Simulations of the Water Food Energy Nexus for policy driven intervention, Heliyon, 2020, 6, e04767 CrossRef CAS PubMed.
- J. Gao, X. Xu, G. Cao, Y. M. Ermoliev, T. Y. Ermolieva and E. A. Rovenskaya, Strategic decision-support modeling for robust management of the food–energy–water nexus under uncertainty, J. Cleaner Prod., 2021, 292, 125995 CrossRef.
- L. Guijun, W. Yongsheng, H. Daohan and Y. Hongtao, A multi-agent model for urban water-energy-food sustainable development simulation, ACM Int. Conf. Proceeding Ser., 2017, F1306, 105–110 Search PubMed.
- N. R. Magliocca, Agent-based modeling for integrating human behavior into the food–energy–water nexus, Land, 2020, 9, 1–25 CrossRef.
- A. Tourigny and Y. Filion, Sensitivity Analysis of an Agent-Based Model Used to Simulate the Spread of Low-Flow Fixtures for Residential Water Conservation and Evaluate Energy Savings in a Canadian Water Distribution System, J. Water Resour. Plan. Manag., 2019, 145, 04018086 CrossRef.
- M. C. Aprile and D. Fiorillo, Water conservation behavior and environmental concerns: Evidence from a representative sample of Italian individuals, J. Cleaner Prod., 2017, 159, 119–129 CrossRef.
- A. Tourigny and Y. Filion, Sensitivity Analysis of an Agent-Based Model Used to Simulate the Spread of Low-Flow Fixtures for Residential Water Conservation and Evaluate Energy Savings in a Canadian Water Distribution System, J. Water Resour. Plan. Manag., 2019, 145, 04018086 CrossRef.
- V. Piñeiro, J. Arias, J. Dürr, P. Elverdin, A. M. Ibáñez, A. Kinengyere, C. M. Opazo, N. Owoo, J. R. Page, S. D. Prager and M. Torero, A scoping review on incentives for adoption of sustainable agricultural practices and their outcomes, Nat. Sustain., 2020, 3, 809–820 CrossRef.
- R. J. Cureau and E. Ghisi, Reduction of potable water consumption and sewage generation on a city scale: A Case study in Brazil, Water, 2019, 11, 2351 CrossRef.
- R. K. Jain, J. E. Taylor and G. Peschiera, Assessing eco-feedback interface usage and design to drive energy efficiency in buildings, Energy Build., 2012, 48, 8–17 CrossRef.
- P. Hammond, M. Suttie, V. T. Lewis, A. P. Smith and A. C. Singer, Detection of untreated sewage discharges to watercourses using machine learning, npj Clean Water, 2021, 4, 1–10 CrossRef.
- E. Kelly, K. F. Shields, R. Cronk, K. Lee, N. Behnke, T. Klug and J. Bartram, Seasonality, water use and community management of water systems in rural settings: Qualitative evidence from Ghana, Kenya, and Zambia, Sci. Total Environ., 2018, 628–629, 715–721 CrossRef CAS PubMed.
- J. Helmbrecht, J. Pastor and C. Moya, Smart solution to improve water-energy nexus for water supply systems, Procedia Eng., 2017, 186, 101–109 CrossRef.
- H. U. Sverdrup, A. H. Olafsdottir and K. V. Ragnarsdottir, On the long-term sustainability of copper, zinc and lead supply, using a system dynamics model, Resour. Conserv. Recycl.: X, 2019, 4, 100007 Search PubMed.
- Y. Yan, Y. Qian, H. Sharif and D. Tipper, A Survey on Smart Grid Communication Infrastructures: Motivations, Requirements and Challenges, IEEE Commun. Surv. Tutor., 2013, 15, 5–20 Search PubMed.
- J. F. M. Koppenjan and B. Enserink, Public–Private Partnerships in Urban Infrastructures: Reconciling Private Sector Participation and Sustainability, Public Adm. Rev., 2009, 69, 284–296 CrossRef.
- X. Zhang and V. V. Vesselinov, Energy-water nexus: Balancing the tradeoffs between two-level decision makers, Appl. Energy, 2016, 183, 77–87 CrossRef.
- L. Yu, Y. Xiao, X. T. Zeng, Y. P. Li and Y. R. Fan, Planning water-energy-food nexus system management under multi-level and uncertainty, J. Cleaner Prod., 2020, 251, 119658 CrossRef.
- B. Cansino-Loeza, X. G. Sánchez-Zarco, E. G. Mora-Jacobo, F. E. Saggiante-Mauro, R. González-Bravo, J. Mahlknecht and J. M. Ponce-Ortega, Systematic Approach for Assessing the Water–Energy–Food Nexus for Sustainable Development in Regions with Resource Scarcities, ACS Sustainable Chem. Eng., 2020, 8, 13734–13748 CrossRef CAS.
- C. A. Scott, S. A. Pierce, M. J. Pasqualetti, A. L. Jones, B. E. Montz and J. H. Hoover, Policy and institutional dimensions of the water-energy nexus, Energy Policy, 2011, 39, 6622–6630 CrossRef.
- A. M. Valek, J. Sušnik and S. Grafakos, Quantification of the urban water-energy nexus in México City, México, with an assessment of water-system related carbon emissions, Sci. Total Environ., 2017, 590, 258–268 CrossRef PubMed.
- C. Howarth and I. Monasterolo, Understanding barriers to decision making in the UK energy-food-water nexus: The added value of interdisciplinary approaches, Environ. Sci. Policy, 2016, 61, 53–60 CrossRef.
- J. D. Sachs, G. Schmidt-Traub, M. Mazzucato, D. Messner, N. Nakicenovic and J. Rockström, Six Transformations to achieve the Sustainable Development Goals, Nat. Sustain., 2019, 2, 805–814 CrossRef.
- J. Yang, K. Lei, S. Khu and W. Meng, Assessment of Water Resources Carrying Capacity for Sustainable Development Based on a System Dynamics Model: A Case Study of Tieling City, China, Water Resour. Manag., 2015, 29, 885–899 CrossRef.
- C. Samberger, The role of water circularity in the food-water-energy nexus and climate change mitigation, Energy Nexus, 2022, 6, 100061 CrossRef.
- A. Karabulut, B. N. Egoh, D. Lanzanova, B. Grizzetti, G. Bidoglio, L. Pagliero, F. Bouraoui, A. Aloe, A. Reynaud, J. Maes, I. Vandecasteele and S. Mubareka, Mapping water provisioning services to support the ecosystem–water–food–energy nexus in the Danube river basin, Ecosyst. Serv., 2016, 17, 278–292 CrossRef.
- G. Quezada, A. Walton and A. Sharma, Risks and tensions in water industry innovation: Understanding adoption of decentralised water systems from a socio-technical transitions perspective, J. Cleaner Prod., 2016, 113, 263–273 CrossRef.
- S.-M. Cheong, G.-W. Choi and H.-S. Lee, Barriers and Solutions to Smart Water Grid Development, Environ. Manage., 2016, 57, 509–515 CrossRef PubMed.
- T. C. Britton, R. A. Stewart and K. R. O'Halloran, Smart metering: enabler for rapid and effective post meter leakage identification and water loss management, J. Cleaner Prod., 2013, 54, 166–176 CrossRef.
- L. Moretto and M. Ranzato, A socio-natural standpoint to understand coproduction of water, energy and waste services, Urban Res. Pract., 2017, 10, 1–21 CrossRef.
- A. Helmrich, S. Markolf, R. Li, T. Carvalhaes, Y. Kim, E. Bondank, M. Natarajan, N. Ahmad and M. Chester, Centralization and decentralization for resilient infrastructure and complexity, Environ. Res.: Infrastruct. Sustain., 2021, 1, 021001 Search PubMed.
- A. Vosughi, A. Tamimi, A. B. King, S. Majumder and A. K. Srivastava, Cyber–physical vulnerability and resiliency analysis for DER integration: A review, challenges and research needs, Renewable Sustainable Energy Rev., 2022, 168, 112794 CrossRef.
- K. Siala, A. K. Chowdhury, T. D. Dang and S. Galelli, Solar energy and regional coordination as a feasible alternative to large hydropower in Southeast Asia, Nat. Commun., 2021, 12, 4159 CrossRef CAS PubMed.
- H. A. Kazem, M. T. Chaichan, A. H. A. Al-Waeli and K. Sopian, A review of dust accumulation and cleaning methods for solar photovoltaic systems, J. Cleaner Prod., 2020, 276, 123187 CrossRef.
- N. Ghaffour, T. M. Missimer and G. L. Amy, Technical review and evaluation of the economics of water desalination: Current and future challenges for better water supply sustainability, Desalination, 2013, 309, 197–207 CrossRef CAS.
- L. Willuweit and J. J. O'Sullivan, A decision support tool for sustainable planning of urban water systems: Presenting the Dynamic Urban Water Simulation Model, Water Res., 2013, 47, 7206–7220 CrossRef CAS PubMed.
- M. Oteng-Peprah, M. A. Acheampong and N. K. DeVries, Greywater characteristics, treatment systems, reuse strategies and user perception—a review, Water, Air, Soil Pollut., 2018, 229, 1–16 CrossRef CAS PubMed.
- Y. Kobayashi, N. J. Ashbolt, E. G. R. Davies and Y. Liu, Life cycle assessment of decentralized greywater treatment systems with reuse at different scales in cold regions, Environ. Int., 2020, 134, 105215 CrossRef PubMed.
- F. M. Enescu, N. Bizon, A. Onu, M. S. Răboacă, P. Thounthong, A. G. Mazare and G. Şerban, Implementing Blockchain Technology in Irrigation Systems That Integrate Photovoltaic Energy Generation Systems, Sustainability, 2020, 12, 1540 CrossRef.
- R. Kakodkar, G. He, C. D. Demirhan, M. Arbabzadeh, S. G. Baratsas, S. Avraamidou, D. Mallapragada, I. Miller, R. C. Allen, E. Gençer and E. N. Pistikopoulos, A review of analytical and optimization methodologies for transitions in multi-scale energy systems, Renewable Sustainable Energy Rev., 2022, 160, 112277 CrossRef CAS.
- M. Ouyang, L. Dueñas-Osorio and X. Min, A three-stage resilience analysis framework for urban infrastructure systems, Struct. Saf., 2012, 36–37, 23–31 CrossRef.
- M. Ghosn, L. Dueñas-Osorio, D. M. Frangopol, T. P. McAllister, P. Bocchini, L. Manuel, B. R. Ellingwood, S. Arangio, F. Bontempi, M. Shah, M. Akiyama, F. Biondini, S. Hernandez and G. Tsiatas, Performance Indicators for Structural Systems and Infrastructure Networks, J. Struct. Eng., 2016, 142, F4016003 CrossRef.
- M. Fayiah, S. Dong, S. Singh and E. A. Kwaku, A review of water–energy nexus trend, methods, challenges and future prospects, Int. J. Energy Water Resour., 2020, 4, 91–107 CrossRef.
- A. Bakchan, M. E. Hacker and K. M. Faust, Resilient Water and Wastewater Infrastructure Systems through Integrated Humanitarian-Development Processes: The Case of Lebanon's Protracted Refugee Crisis, Environ. Sci. Technol., 2021, 55, 6407–6420 CrossRef CAS PubMed.
- C. A. Miller, J. Richter and J. O'Leary, Socio-energy systems design: A policy framework for energy transitions, Energy Res. Soc. Sci., 2015, 6, 29–40 CrossRef.
- J. Liu, V. Hull, H. C. J. Godfray, D. Tilman, P. Gleick, H. Hoff, C. Pahl-Wostl, Z. Xu, M. G. Chung, J. Sun and S. Li, Nexus approaches to global sustainable development, Nat. Sustain., 2018, 1, 466–476 CrossRef.
- C. Alcaraz and S. Zeadally, Critical infrastructure protection: Requirements and challenges for the 21st century, Int. J. Crit. Infrastruct. Prot., 2015, 8, 53–66 CrossRef.
- S. Morrison-Smith and J. Ruiz, Challenges and barriers in virtual teams: a literature review, SN Appl. Sci., 2020, 2, 1096 CrossRef.
- T. P. Ruggaber, J. W. Talley and L. A. Montestruque, Using Embedded Sensor Networks to Monitor, Control, and Reduce CSO Events: A Pilot Study, Environ. Eng. Sci., 2007, 24, 172–182 CrossRef CAS.
- E. Kriegler, B. C. O'Neill, S. Hallegatte, T. Kram, R. J. Lempert, R. H. Moss and T. Wilbanks, The need for and use of socio-economic scenarios for climate change analysis: A new approach based on shared socio-economic pathways, Glob. Environ. Change, 2012, 22, 807–822 CrossRef.
- B. Li, Y. Fan, Y. Huang, W. Linthicum, F. Liu, A. OReilly Beringhs, Y. Dang, Z. Xu, S. Y. Chang, J. Ling, B. D. Huey, S. L. Suib, A. W. K. Ma, P. X. Gao, X. Lu, Y. Lei and M. T. Shaw, Toward long-term accurate and continuous monitoring of nitrate in wastewater using poly (tetrafluoroethylene) (PTFE)−Solid-state ion-selective electrodes (S-ISEs), ACS Sens., 2020, 5, 3182–3193 CrossRef PubMed.
- J. Rasmussen, Risk management in a dynamic society: a modelling problem, Saf. Sci., 1997, 27, 183–213 CrossRef.
- Z. Khan, P. Linares and J. García-González, Integrating water and energy models for policy driven applications. A review of contemporary work and recommendations for future developments, Renewable Sustainable Energy Rev., 2017, 67, 1123–1138 CrossRef.
- S. Behmel, M. Damour, R. Ludwig and M. J. Rodriguez, Water quality monitoring strategies — A review and future perspectives, Sci. Total Environ., 2016, 571, 1312–1329 CrossRef CAS PubMed.
- C. Howarth and I. Monasterolo, Opportunities for knowledge co-production across the energy-food-water nexus: Making interdisciplinary approaches work for better climate decision making, Environ. Sci. Policy, 2017, 75, 103–110 CrossRef.
- S. Namany, T. Al-Ansari and R. Govindan, Sustainable energy, water and food nexus systems: A focused review of decision-making tools for efficient resource management and governance, J. Cleaner Prod., 2019, 225, 610–626 CrossRef.
- C. Gómez-Román, L. Lima, S. Vila-Tojo, A. Correa-Chica, J. Lema and J.-M. Sabucedo, “Who Cares?”: The Acceptance of Decentralized Wastewater Systems in Regions without Water Problems, Int. J. Environ. Res. Public Health, 2020, 17, 9060 CrossRef PubMed.
- E. Trutnevyte, Does cost optimization approximate the real-world energy transition?, Energy, 2016, 106, 182–193 CrossRef.
- M. Javaid, A. Haleem, R. P. Singh and R. Suman, Substantial capabilities of robotics in enhancing industry 4.0 implementation, Cognitive Robotics, 2021, 1, 58–75 CrossRef.
- Y. Deng, M.-A. Cardin, V. Babovic, D. Santhanakrishnan, P. Schmitter and A. Meshgi, Valuing flexibilities in the design of urban water management systems, Water Res., 2013, 47, 7162–7174 CrossRef CAS PubMed.
- A. Bhaduri, C. Ringler, I. Dombrowski, R. Mohtar and W. Scheumann, Sustainability in the water–energy–food nexus, Water Int., 2015, 40, 723–732 CrossRef.
- F. Othman, M. Bahrin and N. Azli, Industry 4.0: A review on industrial automation and robotic, J. Teknol., 2016, 78, 137–143 Search PubMed.
- Z. Abdmouleh, R. A. M. Alammari and A. Gastli, Review of policies encouraging renewable energy integration & best practices, Renewable Sustainable Energy Rev., 2015, 45, 249–262 CrossRef.
- I. A. T. Hashem, V. Chang, N. B. Anuar, K. Adewole, I. Yaqoob, A. Gani, E. Ahmed and H. Chiroma, The role of big data in smart city, Int. J. Inf. Manage, 2016, 36, 748–758 CrossRef.
- A. K. Plappally and J. H. Lienhard V, Energy requirements for water production, treatment, end use, reclamation, and disposal, Renewable Sustainable Energy Rev., 2012, 16, 4818–4848 CrossRef.
- A. Johns, J. Qian, M. E. Carolan, N. Shaikh, A. Peroutka, A. Seeger, J. M. Cerrato, T. Z. Forbes and D. M. Cwiertny, Functionalized electrospun polymer nanofibers for treatment of water contaminated with uranium, Environ. Sci.: Water Res. Technol., 2020, 6, 622–634 RSC.
- X. Zhao, Y. Chen, Y. Yin, L. Zou, Q. Chen, K. Liu, P. Lin, H. Su and Y. Chen, Janus Polypyrrole Nanobelt@Polyvinyl Alcohol Hydrogel Evaporator for Robust Solar-Thermal Seawater Desalination and Sewage Purification, ACS Appl. Mater. Interfaces, 2021, 13, 46717–46726 CrossRef CAS PubMed.
- M. Schwarze, Micellar-enhanced ultrafiltration (MEUF) – state of the art, Environ. Sci.: Water Res. Technol., 2017, 3, 598–624 RSC.
- A. Benjamin Schantz, B. Xiong, E. Dees, D. R. Moore, X. Yang and M. Kumar, Emerging investigators series: prospects and challenges for high-pressure reverse osmosis in minimizing concentrated waste streams, Environ. Sci.: Water Res. Technol., 2018, 4, 894–908 RSC.
- F. Gao, L. Wang, J. Wang, H. Zhang and S. Lin, Nutrient recovery from treated wastewater by a hybrid electrochemical sequence integrating bipolar membrane electrodialysis and membrane capacitive deionization, Environ. Sci.: Water Res. Technol., 2020, 6, 383–391 RSC.
- E. Sahinkaya, A. Sahin, A. Yurtsever and M. Kitis, Concentrate minimization and water recovery enhancement using pellet precipitator in a reverse osmosis process treating textile wastewater, J. Environ. Manage., 2018, 222, 420–427 CrossRef CAS PubMed.
- S. Qiu, H. Zhao, N. Jiang, Z. Wang, L. Liu, Y. An, H. Zhao, X. Miao, R. Liu and G. Fortino, Multi-sensor information fusion based on machine learning for real applications in human activity recognition: State-of-the-art and research challenges, Inf. Fusion, 2022, 80, 241–265 CrossRef.
- D. Vanham, Does the water footprint concept provide relevant information to address the water–food–energy–ecosystem nexus?, Ecosyst. Serv., 2016, 17, 298–307 CrossRef.
- S. D. Musa, T. Zhonghua, A. O. Ibrahim and M. Habib, China's energy status: A critical look at fossils and renewable options, Renewable Sustainable Energy Rev., 2018, 81, 2281–2290 CrossRef.
- X. Liao, Y. Tian, Y. Gan and J. Ji, Quantifying urban wastewater treatment sector's greenhouse gas emissions using a hybrid life cycle analysis method – An application on Shenzhen city in China, Sci. Total Environ., 2020, 745, 141176 CrossRef CAS PubMed.
- Q. Yue, Y. Wang, L. Liu, J. Niu, P. Guo and P. Li, Type-2 fuzzy mixed-integer bi-level programming approach for multi-source multi-user water allocation under future climate change, J. Hydrol., 2020, 591, 125332 CrossRef.
- S. Zheng, C. Li and G. Wei, QSAR modeling for reaction rate constants of e aq − with diverse organic compounds in water, Environ. Sci.: Water Res. Technol., 2020, 6, 1931–1938 RSC.
- E. Shmueli, V. K. Singh, B. Lepri and A. Pentland, Sensing, understanding, and shaping social behavior, IEEE Trans. Comput. Soc. Syst., 2014, 1, 22–34 Search PubMed.
- S. R. Harris-Lovett, C. Binz, D. L. Sedlak, M. Kiparsky and B. Truffer, Beyond User Acceptance: A Legitimacy Framework for Potable Water Reuse in California, Environ. Sci. Technol., 2015, 49, 7552–7561 CrossRef CAS PubMed.
- J. Dargin, B. Daher and R. H. Mohtar, Complexity versus simplicity in water energy food nexus (WEF) assessment tools, Sci. Total Environ., 2019, 650, 1566–1575 CrossRef CAS PubMed.
- Y. Li, J. Styczynski, Y. Huang, Z. Xu, J. McCutcheon and B. Li, Energy-positive wastewater treatment and desalination in an integrated microbial desalination cell (MDC)-microbial electrolysis cell (MEC), J. Power Sources, 2017, 356, 529–538 CrossRef CAS.
- S. L. Harlan, S. T. Yabiku, L. Larsen and A. J. Brazel, Household Water Consumption in an Arid City: Affluence, Affordance, and Attitudes, Soc. Nat. Resour., 2009, 22, 691–709 CrossRef.
- J. Walzberg, A. Carpenter and G. A. Heath, Role of the social factors in success of solar photovoltaic reuse and recycle programmes, Nat. Energy, 2021, 6, 913–924 CrossRef.
|
This journal is © The Royal Society of Chemistry 2023 |