Direct non-target analysis of dissolved organic matter and disinfection by-products in drinking water with nano-LC-FT-ICR-MS†
Received
14th February 2023
, Accepted 24th April 2023
First published on 15th May 2023
Abstract
Non-target analysis of drinking water often requires solid-phase extraction (SPE) as a sample pretreatment to enrich the dissolved organic matter (DOM) and remove salts. However, highly polar and mobile DOM fractions are extracted with only low yield. This limits the deeper understanding of drinking water treatment processes, such as disinfection, resulting in an incomplete picture of the formation of disinfection by-products (DBPs). In this study, we present a nano-LC-FT-ICR-MS method to directly analyze DOM and DBPs in drinking water, shedding light on the previously inaccessible polar fraction of DOM and DBPs. With only 1 μL injection volume, 19
856 and 21
095 DOM compounds were detected across nano-LC elution profiles in samples with and without SPE pretreatment, respectively. Three times as many highly polar DOM compounds were detected in the non-extracted (2269 compounds) as compared to the SPE processed sample (817 compounds). Likewise, 15% more nitrogenous DOM compounds were detected without extraction (8716 compounds) compared to the SPE processed sample (7556 compounds). After disinfection, 2136 and 2225 non-halogenated nitrogenous DBPs were detected in samples from two drinking water treatment plants. The successful direct analysis of dissolved organics in drinking water using nano-LC-FT-ICR-MS enables a more complete picture of DOM and DBPs in drinking water treatment processes.
Water impact
Non-target analysis of drinking water DOM and DBPs usually requires enrichment and desalination of samples, discriminating the highly polar fractions. The novel direct analysis method for drinking water with low carbon content – employing nano-LC and ultrahigh resolution mass spectrometry – presented here enables a more inclusive perspective of DBP formation and their chemical properties.
|
Introduction
Disinfection has been applied in drinking water treatment plants (DWTPs) for decades to prevent water-borne health problems. However, disinfection by-products (DBPs) form due to the unintended reaction of dissolved organic matter (DOM) with disinfectants (such as chlorine, monochloramine, ozone, chlorine dioxide, and UV) leading to increasing concerns about drinking water quality.1–5 Even though the concentration of DOM in drinking water can be 1 mg L−1 or lower,6,7 its reaction with disinfectants still produces numerous DBPs.6,8–10 Until now, over 700 DBPs have been identified,11 whereas many still remain unidentified. Many of the identified and meanwhile regulated DBPs, such as trihalomethane (THMs), haloacetic acid (HAAs), and N-nitrosodimethylamine (NDMA) have been proven to be genotoxic and cytotoxic.12,13 In addition, unregulated DBPs were also reported to cause severe health issues, such as harming the reproductive system and central nervous system, e.g. causing leukemia.14–16 Moreover, DBP toxicity is additive, and whether the known DBPs are more toxic than unknown DBPs is unclear.3
Non-target analysis of DBPs with high resolution mass spectrometry (HRMS), such as Q-TOF,17,18 Orbitrap,7,19 and FT-ICR-MS,20–22 has greatly improved the identification of molecular formulas of DBPs. Solid phase extraction (SPE) is commonly used as a pretreatment step before direct infusion (DI) into HRMS as it can enrich a broad range of organic compounds and efficiently remove inorganic constituents.6,7,22–26 In general, reversed-phase (RP) type SPE sorbents facilitate the recovery of nonpolar and polar compounds,21,27–29 whereas the most polar compounds can also be overlooked due to their incomplete recovery during the SPE process.20 In addition, the traditional SPE-DI analysis with HRMS cannot be used to obtain isomeric information of DOM and DBPs due to the large structural diversity of both classes of compounds.21,28,30
Recently, the development of online high performance liquid chromatography (HPLC) coupled with FT-ICR-MS or Orbitrap was reported to be beneficial for the separation of isomeric compounds in complex DOM mixtures, as well as for the removal of salts and the reduction of matrix effects.31–34 However, a large volume (20–100 μl) of sample and high concentration of dissolved organic carbon (DOC, ∼200 ppm) is often required for sensitive detection of DOM on LC columns in HPLC systems.26,31 In comparison, lower concentrations and injection volumes can be used with nano-liquid chromatography (nano-LC) coupled to FT-ICR-MS for the measurement of soil extracts35 and soil solutions,36 which shows the potential of nano-LC also for water samples with low DOC concentrations. Compared to (U)HPLC, due to the smaller column size and small particles used with high pressure, nano-LC achieves better chromatographic separation and thus also higher sensitivity.
The objective of this work was to study (polar) DOM and DBP fractions in drinking water samples, which are overlooked by traditional SPE pretreatment approaches for non-targeted analysis. To close this analytical gap, a novel online nano-LC-FT-ICR-MS method was developed and applied for the direct analysis of drinking water samples. Both, original and SPE processed samples were analyzed with nano-LC-FT-ICR-MS and compared with respect to detected DOM compounds. Further, the disinfected drinking water samples from two DWTPs (with chlorine gas (Cl2) and chlorine dioxide (ClO2) disinfection) were used to demonstrate the applicability of the method for non-targeted DBP detection.
Materials and methods
Chemicals and water samples
To establish and demonstrate the direct analysis of drinking water samples, disinfected drinking water samples from two different drinking water treatment plants (DWTPs) were used. Drinking water samples with and without disinfection with Cl2 (DWTP-A) and ClO2 (DWTP-B) were collected on March 22 and February 11, 2021, respectively. All samples were collected in glass bottles (baked at 400 °C for 4 hours) and stored at 4 °C in the dark. Collected chlorinated water samples were not quenched since 1) the quenching agent itself may react with the DOM and DBPs,37 and 2) the residual chlorine concentration was lower than 0.2 mg L−1 in both samples. More information about both DWTPs, water samples, and chemicals are presented in the ESI† Text S1 and Table S1.
Analytical grade D-glucuronic acid, fraxin, isoferulic acid, 3-O-β-D-glucuronide and 2-(4-(2,2-dicarboxy-ethyl)-2,5-dimethoxy-benzyl)-malonic acid were used as model compounds for the nano-LC column quality control (peak intensity and retention time monitoring). Detailed information of the model compounds are shown in Table S2.†
Solid phase extraction
For solid phase extraction (SPE), an automated extraction system (FreeStyle, LCTech, Obertaufkirchen, Germany) was used with 50 mg Bond Elut Priority PolLutant (PPL) cartridges (Agilent Technologies, Santa Clara, CA, USA) and 150 mL sample at pH 2 (adjusted with HCl: ultrapure, Merck, Germany). After extraction, the samples were eluted with 1 mL of methanol (MeOH: Biosolve, Valkenswaard, Netherlands). At a nominal enrichment factor of 150, the carbon recovery rate was (50 ± 3)%, comparable to previous studies using PPL for drinking water.6,21 Prior to analysis by nano-LC, MeOH in the SPE eluate was completely dried with nitrogen gas, then re-dissolved with ultrapure water (Milli-Q, Merck, Darmstadt, Germany) water to adjust to the same DOC concentration as the corresponding non-extracted water sample (i.e., 2–2.5 mg L−1, cf. Table S2†).
Nano-LC-FT-ICR-MS
Samples with and without disinfection were directly analyzed with nano-LC-FT-ICR-MS. The chromatographic separation was done on a nano-LC system (Ultimate 3000 RSLC nano, Thermo Fisher Scientific, Waltham, MA), which was equipped with an NCS-3500RS binary pump and a WPS-3000TPL RS autosampler. The separation was performed on a C18 column (Acclaim PepMap, 75 μm × 150 mm, 3 μm, 100 Å, Thermo Fisher Scientific, USA), equipped with a guard column (Acclaim PepMap, 2 cm × 75 μm, 3 μm, Thermo Fischer Scientific). A 1 μL sample loop was used for the 1 μL injection volume.
The nano-LC gradient started with 99% ultrapure water as eluent A and 1% MeOH (Biosolve, Valkenswaard, Netherlands) as eluent B at 1 min. The pH of the eluent A was adjusted to 3 with 0.05% formic acid (FA: Sigma-Aldrich, USA) and ammonium hydroxide (NH4OH: Sigma-Aldrich, USA), the same amount of FA and NH4OH was added to eluent B as well. In the following 10 min, the MeOH ratio linearly increased to 99%, and stabilized for 20 min at this ratio for complete flushing of the column with organic eluent, then decreased to 1% in 20 min, followed with another 15 min column equilibration. The flow rate was constant at 500 nL min−1. More details regarding the gradient are provided in Table S3.†
A Fourier-transform ion cyclotron resonance mass spectrometer (FT-ICR-MS) equipped with a dynamically harmonized cell (solariX XR, Bruker Daltonics Inc., Billerica, MA, USA) and a 12 T refrigerated actively shielded superconducting magnet (Bruker Biospin, Wissembourg, France) was used. The nano-LC system was directly coupled to the FT-ICR-MS by a nanoESI source (CaptiveSpray, Bruker Daltonics) operated in negative ionization mode. A capillary voltage of 1500 V, a nebulizer gas pressure of 0.2 bar, and a drying gas at 150 °C with flow rate of 3.0 L min−1 was used. A data size of 2 Megaword (147 start m/z, ∼0.84 s transients, full profile mode) was used for all online analyses. A mass resolving power (m/Δm50%) of 270
000 was achieved at m/z 400 (Table S5†), which is sufficient to resolve all major DOM species in the mass range of 150 to 1000 Da.38,39 A commonly existing DOM peak at m/z 313.0929 (C14H18O8) was selected to tune the ion accumulation time (IAT) in order to achieve comparable absolute intensity of the base peak in corresponding segments for both, the non-extracted (DW-nLC-A) and the extracted (DW-SPE-nLC-A) sample. This resulted in an IAT of 340 ms and 110 ms for direct and SPE processed DWTP-A sample analysis, respectively, while 260 ms was used for the direct analysis of DWTP-B.
Data analysis
The nano-LC chromatogram from 11 min to 21 min was split into 20 segments with 0.5 min width based on the elution profile of a set of model compounds (Fig. S1†). Using model compounds, the chromatographic performance (i.e., resolution) of our nano-LC method was assessed. Since individual organic compounds can fully elute within 0.5 min we estimate the lowest number of isomers of unresolved DOM elution profiles similar to previous studies.31 Each segment was treated as an individual spectrum and internally calibrated (DataAnalysis 5.0, Bruker Daltonics) with masses commonly found in DOM (m/z 150–1000, 54 < number of calibrants < 186). Assuming singly charged ions (based on 12C–13C1m/z spacing), molecular formulas (MFs) were assigned to each m/z value in the mass range (150–1000 m/z) using an in-house software.31
Formula assignment and filtering rules were applied as follows: C1–60, 13C0–1, H1–122, O0–40, N0–2, S0–1, 34S0–1, 35Cl0–3, 37Cl0–3, 0.3 < H/C < 2.5, 0 < O/C < 1, 0 < N/C < 1.5, 0 < DBE < 25 (double bond equivalent, DBE = 1 + 1/2 (2C–H + N)), −10 < DBE-O < 10.40–42 Isotopes 13C, 34S, and 37Cl were used for quality control but removed from the final data set. Cl-containing molecular formulas (MFs) were validated with 35Cl/37Cl isotopologue intensity ratio patterns (for 1 Cl: (35Cl137Cl0)/(35Cl037Cl1) = 3.2 ± 0.15; 2 Cl: (35Cl237Cl0)/(35Cl137Cl1) = 1.6 ± 0.15; 3Cl: (35Cl337Cl0)/(35Cl237Cl1) = 1 ± 0.15).22 The Cl-containing MFs were further verified by matching the theoretical isotopologue peak intensity distribution to the measured isotopologue peaks in raw LC-MS spectra. Although the structure/exact chemical identity is unknown (confidence level 4),43 MFs in segments are referred to as compounds based on unequivocal formulas and polarity (retention time) information.
All compounds found in blanks (ultrapure water measured with nano-LC-FT-ICR-MS) were removed from the corresponding segments in the samples. DBPs were identified in this study after subtracting all compounds found in non-disinfected samples from the disinfected water samples in the corresponding segments. MF data are available from https://doi.org/10.48758/ufz.13076.
Segments between 11 to 13 min are defined as early segments, the middle segments as eluting between 13 to 17.5 min, and the late segments between 17.5 to 21 min. According to the separation on a reversed-phase column, compounds eluting at earlier retention times are typically associated with a higher polarity as compared to compounds eluting later.44
Results and discussion
Detection of DOM in drinking water samples with and without SPE pretreatment
Both extracted (DW-SPE-nLC-A) and non-extracted (DW-nLC-A) drinking water (DW) samples were injected with a volume of 1 μL (total amount of carbon: 1.95 ng C) into the nano-LC-FT-ICR-MS system. The summed peak intensity of the quality control peak m/z 313.0929 for the 20 segments between 11 to 21 min retention time (RT) was comparable at 1.3 × 107 and 1.6 × 107 in DW-nLC-A and DW-SPE-nLC-A, respectively. Comparing the chromatographic separation of the three isomeric model compounds (molecular formula: C16H18O10) on the nano-LC system with a similar solvent gradient on a UHPLC system previously developed in our group31 shows that the retention factors on the nano-LC column are smaller (Table S4†), achieving a better chromatographic separation of isomers (Fig. S1†).
In total, 21
095 compounds (4874 unique MFs) and 19
856 compounds (4474 unique MFs) were detected across all segments in DW-nLC-A and DW-SPE-nLC-A, respectively. Despite similar total number of compounds, 7374 and 6135 compounds were uniquely detected in DW-nLC-A and DW-SPE-nLC-A, respectively (Fig. S2 and S3†). The number of detected unique MFs in both samples was comparable to previous results obtained with SPE processed surface water samples analyzed by SPE and DI-FT-ICR-MS.28
The largest differences between the SPE processed sample and the non-extracted sample regarding the number of detected compounds were observed in both early (11–13 min) and late (17.5–21 min) segments. For the early eluting compounds, which represents the most polar fraction of DOM in drinking water, over 2.8 times more compounds were detected in DW-nLC-A (2269 compounds) as compared to DW-SPE-nLC-A (817 compounds). In addition, the intensity weighted average (wa) oxygen-to-carbon ratio (O/Cwa) of the early eluting segments was higher in DW-nLC-A than in DW-SPE-nLC-A (Fig. 1). In the late eluting segments, a higher weighted average hydrogen-to-carbon (H/Cwa) ratio (+0.1 ± 0.05; Fig. 1) alongside with a lower weighted average modified aromaticity index (AImod,wa) and double bond equivalent minus oxygen (DBE-Owa) was observed in DW-nLC-A as compared to DW-SPE-nLC-A (Fig. S4a and b†). This indicates that both, more saturated (i.e., less aromatic) and more polar compounds were detected in the non-extracted sample. The loss of highly polar fractions45 and unsaturated compounds29 during solid phase extraction is a known phenomenon and was previously also observed for effluent organic matter (EfOM).27 Notably, in the late segments, corresponding to less polar DOM compounds, 20% more uniquely detected compounds were found in the non-extracted (DW-nLC-A) as compared to the SPE processed sample (DW-SPE-nLC-A), which was different from previous observations of EfOM using a HPLC system.27 This may be due to the fact that 1) the bank filtration (water passage through soils) has already removed most of the non-polar, aromatic compounds of the DW source water by microbial biofilms and physical retention,46 or 2) the nanoLC-nanoESI combination achieves a higher sensitivity with improved ionization of non-polar compounds during direct analysis, counteracting the preferential extraction of such compounds by SPE.
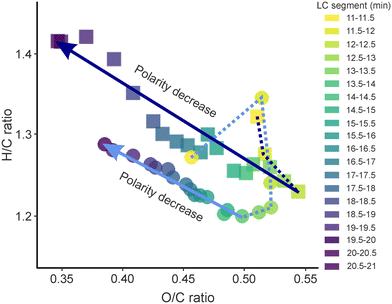 |
| Fig. 1 Intensity weighted average hydrogen-to-carbon (H/C) vs. oxygen-to-carbon (O/C) ratios of all MFs detected in segments of non-extracted (DW-nLC-A: squares) and SPE processed (DW-SPE-nLC-A: circles) samples. Color code according to retention time range of segments. Note that in the earliest segments of DW-SPE-nLC-A only few MF were detected (cf. Fig. S2†). Lines connecting dots are used as visual aid. | |
On the contrary, in the middle segments (13–17.5 min), 9% more DOM compounds were detected in DW-SPE-nLC-A (10
142 compounds) than in DW-nLC-A (9291 compounds), and 26% more MFs were uniquely detected in DW-SPE-nLC-A (3295 MFs) as compared to DW-nLC-A (2445 MFs). This indicates that the SPE process enriched nonpolar compounds, which is consistent with previous observations that reversed-phase type SPE (like the PPL sorbents) preferentially enriches non-polar DOM.27,45
Regarding DOM isomer separation (i.e., MFs occurring in multiple segments), over 81% of the MFs in DW-nLC-A eluted across multiple segments whereas this number was only 52% for the SPE processed (DW-SPE-nLC-A) sample. A loss of isomers during extraction was also observed on the individual compound (i.e., m/z) level. The extracted ion chromatogram (EIC) of one ubiquitously existing MF in DOM (m/z 313.0929: C14H18O8) reveals a loss of intensity at early retention times (corresponding to the most polar isomers) and an increase of intensity at the middle retention times (corresponding to non-polar isomers) as result of the SPE process (Fig. S5†). In addition, based on the lower number of uniquely detected compounds in both the early and late eluting segments in DW-SPE-nLC-A we conclude that not only the highly polar isomeric compounds may be lost during SPE process, but also the most non-polar compounds in DW were inefficiently eluted from the SPE cartridges, consistent with findings from Raeke et al. (2016).45
Nitrogenous DOM in drinking water samples with and without SPE pretreatment
The DOM containing nitrogen (N-DOM) is particularly concerning in drinking water as it comprises possible precursors of the nitrogenous DBPs (N-DBPs),47 many of which have been shown to be more toxic than other, non-nitrogenous DBPs.12,48,49 As compared to the SPE processed sample, 15% more N-DOM compounds were detected in the samples without SPE pretreatment (DW-nLC-A: 8716 compounds, DW-SPE-nLC-A: 7556 compounds). In addition, 44% and 39% of all MFs were N-DOM MFs in DW-nLC-A (2142 MFs) and DW-SPE-nLC-A (1736 MFs), respectively. In typical SPE-DI analysis of drinking water samples, this number can be as low as 22%.6,28 The higher percentage of detected N-DOM compounds in the directly analyzed DW samples in this study can be explained by less bias from the SPE preprocessing (usually done at pH 2), knowing to inefficiently retain N-DOM, and less suppression of N-DOM from acidic CHO-DOM compounds due to the separation of compounds on the nano-LC column.31
Similar to the entirety of DOM compounds (Fig. S2†), the largest difference for N-DOM compounds between non-extracted and SPE processed drinking water was found in both the early and late eluting segments, with over 148% (996 vs. 401 compounds) and 54% (3375 vs. 2190 compounds) more compounds detected in DW-nLC-A versus DW-SPE-nLC-A, respectively (Fig. 2). However, for the N-DOM compounds, the difference between non-extracted and SPE processed sample was more pronounced than for the bulk DOM. Likewise, in the middle eluting segments, 14% more N-DOM compounds were detected in DW-SPE-nLC-A as compared to DW-nLC-A. The high percentage of highly polar and very non-polar compounds detected in non-extracted samples confirms the overall lower extraction efficiencies of N-DOM on reversed-phase SPE material, if extraction is performed under acidic conditions (typically at pH = 2).27 The higher percentage of non-polar N-DOM found in the non-extracted DW sample further confirms that the extraction process is biased towards preferential extraction of non-polar CHO compounds while losing nitrogen containing, polar and non-polar compounds, which may be important DBP precursors, as also observed for EfOM.27,45
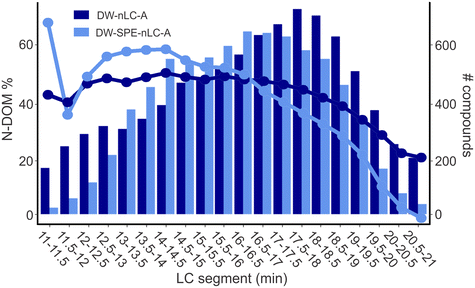 |
| Fig. 2 Percentage (left y-axis, circles and lines) and number (right y-axis, bars) of N-DOM compounds in each segment of samples DW-nLC-A (dark blue) and DW-SPE-nLC-A (light blue). | |
Direct analysis of DBPs in drinking water samples with different disinfectants
The low concentration of DOC and the naturally existing salts in drinking water samples are challenging for a robust and reliable non-target analysis of DBPs.26 To demonstrate the applicability of a direct analysis of DBPs in drinking water with nano-LC-FT-ICR-MS, drinking water samples disinfected with Cl2 (DWTP-A) and ClO2 (DWTP-B) were analyzed. Both samples had moderately low DOC (1.95 mg L−1 and 2.69 mg L−1) and residual disinfectant concentrations (∼0.2 mg Cl2/L; Table S1†).
A similar number of non-halogenated DBPs were detected in disinfected samples of DWTP-A (4989) and DWTP-B (4826). Of these, over 43% (2136 compounds) and 46% (2225 compounds) were non-halogenated N-DBPs in DW-nLC-A and DW-nLC-B, respectively (Fig. 3 and S6†).
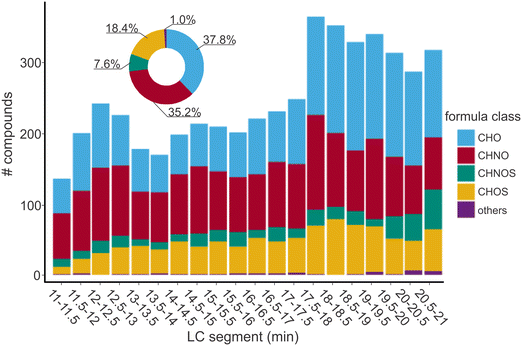 |
| Fig. 3 Formula class distribution of non-halogenated DBP compounds in sample DW-nLC-A. The bars indicate the total number of non-halogenated DBPs detected in each segment distinguished by formula classes (CHO, CHNO, CHOS, CHNOS, and others). The inlay summarizes the percentage of formula classes of all non-halogenated DBPs (all segments combined). | |
Overall, more N-DBPs were detected in the late eluting segments (on average 130 compounds) than in the early and middle eluting segments (∼100 compounds) in DW-nLC-A and DW-nLC-B. In the early and middle eluting segments, a relatively high fraction of non-halogenated N-DBPs (∼48%) was detected in DW-nLC-A and DW-nLC-B. However, in late eluting segments, a lower percentage of non-halogenated N-DBPs was detected (∼37%) in both DW-nLC-A and DW-nLC-B (Fig. 3 and S6†). This may be due to 1) more highly non-polar N-DBPs being formed during disinfection, which accordingly elute in the late segments, 2) the suppression of non-halogenated N-DBPs caused by CHO compounds (i.e., non-heteroatom compounds, likely to be of acidic character) is less pronounced in the early eluting segments.
Also, in both samples, lower H/Cwa and higher O/Cwa ratios were observed for non-halogenated DBPs as compared to the DOM in corresponding middle and late eluting segments (Fig. S7†). This is because the non-halogenated DBPs are produced by oxidation during disinfection, which would form byproducts with higher polarity (higher O/C ratio) than the corresponding precursors.50
Cl-DBPs were detected in both DWTPs despite the low concentration of DOC and the very low concentration of disinfectants (∼0.5 mg L−1) used. In total, 16 Cl-DBPs (Table S6†) were validated according to isotopologue intensity pattern (Fig. 4 and S8†) in DW-nLC-A and DW-nLC-B. Of these, four had distinct EICs (Fig. 4 and S9†), including one of the previously reported CHO-Cl MF26,50 (C15H13Cl1O6, Fig. 4). More than 30 structures for the molecular formula C15H13Cl1O6 are listed in ChemSpider, and further validation of its chemical structure could be accomplished in future studies by MS/MS experiments and utilization of standard compounds.
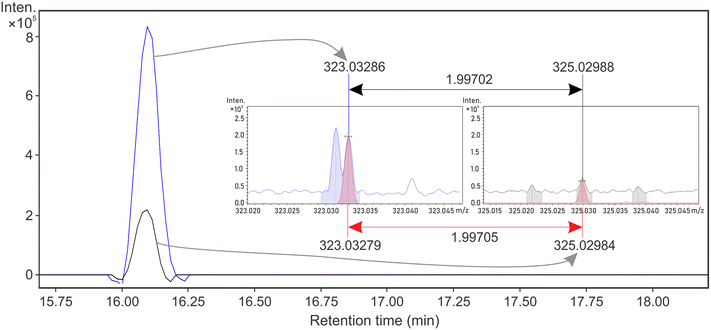 |
| Fig. 4 EICs of molecular formula C15H13Cl1O6 isotopologue peaks: m/z 323.0238 (blue) and 325.0298 (black). Inside figures: the theoretical isotopologue peaks (red) and measured peaks (blue and black) in raw spectra at the same retention time. The mass differences of isotopologue peaks were indicated with black (raw spectra) and red (theoretical isotopologue) arrows. | |
The relatively low number of detected Cl-DBPs in this study can be attributed to: 1) low disinfectant dose: drinking water disinfection in both DWTP-A and DWTP-B uses a much smaller chlorine and ClO2 concentrations as compared to other DWTP and lab studies, which typically use more than 5 mg Cl2/L,20,28,51 and 2) the concentration of DOC (determining parameter for Cl-DBP formation)8 in both DWTPs was also lower than in previous studies,26,50 leading to lower concentrations of DBPs. Despite the low DOC and disinfectant concentration, the nano-LC-MS method still revealed Cl-DBPs proving its applicability for sensitive detection of N-DBPs and Cl-DBPs.
Conclusions
The direct analysis of drinking water with nano-LC-FT-ICR-MS revealed previously overlooked highly polar and highly saturated DOM and DBPs in drinking water. In contrast to the current state of research on DBPs, which usually uses 1 L of drinking water, desalination/enrichment with SPE, and measurement with DI-HRMS at higher DOC concentrations, detailed molecular analysis from only 1 μL of sample directly injected into nano-LC-FT-ICR MS is possible. The presented nano-LC-FT-ICR-MS method offers advantages through less sample preparation with accordingly less potential bias and contamination and is also more environmentally friendly, saving solvents for SPE and LC. Although the number of Cl-DBPs detected in this study is low compared to published studies,6,8,21 the direct analysis of drinking water at high sensitivity is a very promising approach as it can bypass the SPE process and detect more non-halogenated N-DBPs. As some non-halogenated DBPs are not only inherently toxic (especially N-DBPs),4,52 but also serve as intermediates for the formation of halogenated DBPs,53 a sensitive and bias-free analysis of DBPs in drinking water is necessary to allow for a better assessment of the disinfection process. Additionally, we suggest including real drinking water samples in addition to lab-disinfected samples to foster a realistic perspective of the amount of DBPs detectable with non-targeted analysis. Overall, the direct analysis of drinking water may lead to a broader and better understanding of unknown DBPs and is recommended for non-targeted analysis of DOM from drinking water and disinfected samples.
Conflicts of interest
The authors declare no competing financial interest.
Acknowledgements
We are very grateful to Jan Kaesler for his help with method development, Johann Wurz for software development, and the Chinese Scholarship Council for providing scholarship to L. H. We acknowledge the ProVIS Centre for Chemical Microscopy within the Helmholtz Centre for Environmental Research Leipzig, which is supported by European regional development funds (EFRE—Europe Funds Saxony) and the Helmholtz Association. The NTA Study Reporting Tool (SRT) was used during peer review of this study (https://doi.org/10.1021/acs.analchem.1c02621).
References
- S. D. Richardson, M. J. Plewa, E. D. Wagner, R. Schoeny and D. M. Demarini, Occurrence, genotoxicity, and carcinogenicity of regulated and emerging disinfection by-products in drinking water: a review and roadmap for research, Mutat. Res., 2007, 636, 178–242, DOI:10.1016/j.mrrev.2007.09.001.
- S. E. Hrudey and J. Fawell, 40 years on: what do we know about drinking water disinfection by-products (DBPs) and human health?, Water Sci. Technol.: Water Supply, 2015, 15, 667–674, DOI:10.2166/ws.2015.036.
- S. S. Lau, X. Wei, K. Bokenkamp, E. D. Wagner, M. J. Plewa and W. A. Mitch, Assessing Additivity of Cytotoxicity Associated with Disinfection Byproducts in Potable Reuse and Conventional Drinking Waters, Environ. Sci. Technol., 2020, 54, 5729–5736, DOI:10.1021/acs.est.0c00958.
- S. D. Richardson, Disinfection by-products and other emerging contaminants in drinking water, TrAC, Trends Anal. Chem., 2003, 22, 666–684, DOI:10.1016/S0165-9936(03)01003-3.
- R. K. Padhi, S. Subramanian and K. K. Satpathy, Formation, distribution, and speciation of DBPs (THMs, HAAs, ClO2−, and ClO3−) during treatment of different source water with chlorine and chlorine dioxide, Chemosphere, 2019, 218, 540–550, DOI:10.1016/j.chemosphere.2018.11.100.
- P. Phungsai, F. Kurisu, I. Kasuga and H. Furumai, Changes in Dissolved Organic Matter Composition and Disinfection Byproduct Precursors in Advanced Drinking Water Treatment Processes, Environ. Sci. Technol., 2018, 52, 3392–3401, DOI:10.1021/acs.est.7b04765.
- I. Kasuga, M. Suzuki, F. Kurisu and H. Furumai, Molecular-level characterization of biodegradable organic matter causing microbial regrowth in drinking water by non-target screening using Orbitrap mass spectrometry, Water Res., 2020, 184, 116130, DOI:10.1016/j.watres.2020.116130.
- A. Andersson, M. Harir, M. Gonsior, N. Hertkorn, P. Schmitt-Kopplin, H. Kylin, S. Karlsson, M. J. Ashiq, E. Lavonen, K. Nilsson, Ä. Pettersson, H. Stavklint and D. Bastviken, Waterworks-specific composition of drinking water disinfection by-products, Environ. Sci.: Water Res. Technol., 2019, 5, 861–872, 10.1039/C9EW00034H.
-
T. Karanfil, S. W. Krasner, P. Westerhoff and Y. Xie, in Disinfection By-Products in Drinking Water, American Chemical Society, 2008, ch. 1, vol. 995, pp. 2–19 Search PubMed.
- G. Huang, T. W. Ng, H. Chen, A. T. Chow, S. Liu and P. K. Wong, Formation of assimilable organic carbon (AOC) during drinking water disinfection: A microbiological prospect of disinfection byproducts, Environ. Int., 2020, 135, 105389, DOI:10.1016/j.envint.2019.105389.
- S. D. Richardson and S. Y. Kimura, Water Analysis: Emerging Contaminants and Current Issues, Anal. Chem., 2020, 92, 473–505, DOI:10.1021/acs.analchem.9b05269.
- A. D. Shah and W. A. Mitch, Halonitroalkanes, Halonitriles, Haloamides, and N-Nitrosamines: A Critical Review of Nitrogenous Disinfection Byproduct Formation Pathways, Environ. Sci. Technol., 2012, 46, 119–131, DOI:10.1021/es203312s.
- N. J. P. Wawryk, C. B. Craven, L. K. J. Blackstock and X.-F. Li, New methods for identification of disinfection byproducts of toxicological relevance: Progress and future directions, J. Environ. Sci., 2021, 99, 151–159, DOI:10.1016/j.jes.2020.06.020.
- A. F. Gilca, C. Teodosiu, S. Fiore and C. P. Musteret, Emerging disinfection byproducts: A review on their occurrence and control in drinking water treatment processes, Chemosphere, 2020, 259, 127476, DOI:10.1016/j.chemosphere.2020.127476.
- A. Hebert, D. Forestier, D. Lenes, D. Benanou, S. Jacob, C. Arfi, L. Lambolez and Y. Levi, Innovative method for prioritizing emerging disinfection by-products (DBPs) in drinking water on the basis of their potential impact on public health, Water Res., 2010, 44, 3147–3165, DOI:10.1016/j.watres.2010.02.004.
- S. Chowdhury, M. J. Rodriguez and R. Sadiq, Disinfection byproducts in Canadian provinces: Associated cancer risks and medical expenses, J. Hazard. Mater., 2011, 187, 574–584, DOI:10.1016/j.jhazmat.2011.01.085.
- H. K. Liberatore, D. C. Westerman, J. M. Allen, M. J. Plewa, E. D. Wagner, A. M. McKenna, C. R. Weisbrod, J. P. McCord, R. J. Liberatore, D. B. Burnett, L. H. Cizmas and S. D. Richardson, High-Resolution Mass Spectrometry Identification of Novel Surfactant-Derived Sulfur-Containing Disinfection Byproducts from Gas Extraction Wastewater, Environ. Sci. Technol., 2020, 54, 9374–9386, DOI:10.1021/acs.est.0c01997.
- H. Tang, H. Zhong, Y. Pan, Q. Zhou, Z. Huo, W. Chu and B. Xu, A New Group of Heterocyclic Nitrogenous Disinfection Byproducts (DBPs) in Drinking Water: Role of Extraction pH in Unknown DBP Exploration, Environ. Sci. Technol., 2021, 55, 6764–6772, DOI:10.1021/acs.est.1c00078.
- J. Yang, W. Li, Q. Zhu, M. Yang, J. Li, J. Zhang, B. Yang and X. Zhao, Identification, Formation, and Predicted Toxicity of Halogenated DBPs Derived from Tannic Acid and Its Biodegradation Products, Environ. Sci. Technol., 2019, 53, 13019–13030, DOI:10.1021/acs.est.9b03073.
- H. Zhang, Y. Zhang, Q. Shi, J. Hu, M. Chu, J. Yu and M. Yang, Study on Transformation of Natural Organic Matter in Source Water during Chlorination and Its Chlorinated Products using Ultrahigh Resolution Mass Spectrometry, Environ. Sci. Technol., 2012, 46, 4396–4402, DOI:10.1021/es203587q.
- M. Gonsior, P. Schmitt-Kopplin, H. Stavklint, S. D. Richardson, N. Hertkorn and D. Bastviken, Changes in Dissolved Organic Matter during the Treatment Processes of a Drinking Water Plant in Sweden and Formation of Previously Unknown Disinfection Byproducts, Environ. Sci. Technol., 2014, 48, 12714–12722, DOI:10.1021/es504349p.
- X. Liu, R. Liu, B. Zhu, T. Ruan and G. Jiang, Characterization of Carbonyl Disinfection By-Products During Ozonation, Chlorination, and Chloramination of Dissolved Organic Matters, Environ. Sci. Technol., 2020, 54, 2218–2227, DOI:10.1021/acs.est.9b04875.
- X. Wang, J. Wang, Y. Zhang, Q. Shi, H. Zhang, Y. Zhang and M. Yang, Characterization of unknown iodinated disinfection byproducts during chlorination/chloramination using ultrahigh resolution mass spectrometry, Sci. Total Environ., 2016, 554–555, 83–88, DOI:10.1016/j.scitotenv.2016.02.157.
- C. H. Jeong, C. Postigo, S. D. Richardson, J. E. Simmons, S. Y. Kimura, B. J. Mariñas, D. Barcelo, P. Liang, E. D. Wagner and M. J. Plewa, Occurrence and Comparative Toxicity of Haloacetaldehyde Disinfection Byproducts in Drinking Water, Environ. Sci. Technol., 2015, 49, 13749–13759, DOI:10.1021/es506358x.
- A. Andersson, M. Gonsior, M. Harir, N. Hertkorn, P. Schmitt-Kopplin, L. Powers, H. Kylin, D. Hellström, K. Nilsson, Ä. Pettersson, H. Stavklint and D. Bastviken, Molecular changes among non-volatile disinfection by-products between drinking water treatment and consumer taps, Environ. Sci.: Water Res. Technol., 2021, 7, 2335–2345, 10.1039/D1EW00389E.
- C. Postigo, A. Andersson, M. Harir, D. Bastviken, M. Gonsior, P. Schmitt-Kopplin, P. Gago-Ferrero, L. Ahrens, L. Ahrens and K. Wiberg, Unraveling the chemodiversity of halogenated disinfection by-products formed during drinking water treatment using target and non-target screening tools, J. Hazard. Mater., 2021, 401, 123681, DOI:10.1016/j.jhazmat.2020.123681.
- E. Jennings, A. Kremser, L. Han, T. Reemtsma and O. J. Lechtenfeld, Discovery of Polar Ozonation Byproducts via Direct Injection of Effluent Organic Matter with Online LC-FT-ICR-MS, Environ. Sci. Technol., 2022, 56, 1894–1904, DOI:10.1021/acs.est.1c04310.
- R. P. Milstead and C. K. Remucal, Molecular-Level Insights into the Formation of Traditional and Novel Halogenated Disinfection Byproducts, ACS ES&T Water, 2021, 1, 1966–1974, DOI:10.1021/acsestwater.1c00161.
- X. Wang, Y. Ji, Q. Shi, Y. Zhang, C. He, Q. Wang, S. Guo and C. Chen, Characterization of wastewater effluent organic matter with different solid phase extraction sorbents, Chemosphere, 2020, 257, 127235, DOI:10.1016/j.chemosphere.2020.127235.
- D. F. Smith, D. C. Podgorski, R. P. Rodgers, G. T. Blakney and C. L. Hendrickson, 21 Tesla FT-ICR Mass Spectrometer for Ultrahigh-Resolution Analysis of Complex Organic Mixtures, Anal. Chem., 2018, 90, 2041–2047, DOI:10.1021/acs.analchem.7b04159.
- L. Han, J. Kaesler, C. Peng, T. Reemtsma and O. J. Lechtenfeld, Online Counter Gradient LC-FT-ICR-MS Enables Detection of Highly Polar Natural Organic Matter Fractions, Anal. Chem., 2021, 93, 1740–1748, DOI:10.1021/acs.analchem.0c04426.
- D. Kim, S. Kim, S. Son, M.-J. Jung and S. Kim, Application of Online Liquid Chromatography 7 T FT-ICR Mass Spectrometer Equipped with Quadrupolar Detection for Analysis of Natural Organic Matter, Anal. Chem., 2019, 91, 7690–7697, DOI:10.1021/acs.analchem.9b00689.
- C. Patriarca, J. Bergquist, P. J. R. Sjoberg, L. Tranvik and J. A. Hawkes, Online HPLC-ESI-HRMS Method for the Analysis and Comparison of Different Dissolved Organic Matter Samples, Environ. Sci. Technol., 2018, 52, 2091–2099, DOI:10.1021/acs.est.7b04508.
- J. A. Hawkes, C. Patriarca, P. J. R. Sjöberg, L. J. Tranvik and J. Bergquist, Extreme isomeric complexity of dissolved organic matter found across aquatic environments, Limnol. Oceanogr. Lett., 2018, 3, 21–30, DOI:10.1002/lol2.10064.
- M. P. Ladd, R. J. Giannone, P. E. Abraham, S. D. Wullschleger and R. L. Hettich, Evaluation of an untargeted nano-liquid chromatography-mass spectrometry approach to expand coverage of low molecular weight dissolved organic matter in Arctic soil, Sci. Rep., 2019, 9, 5810, DOI:10.1038/s41598-019-42118-9.
- M. Lohse, S. Blaser, D. Vetterlein, S. Schlűter, E. Oburger, T. Reemtsma and O. J. Lechtenfeld, On-line nano-solid phase extraction Fourier-transform ion cyclotron resonance mass spectrometry workflow to analyze small scale gradients of soil solution organic matter in the rhizosphere, Anal. Chem., 2020, 92, 10442–10449, DOI:10.1021/acs.analchem.0c00946.
- I. Kristiana, A. Lethorn, C. Joll and A. Heitz, To add or not to add: The use of quenching agents for the analysis of disinfection by-products in water samples, Water Res., 2014, 59, 90–98, DOI:10.1016/j.watres.2014.04.006.
- B. P. Koch, K. U. Ludwichowski, G. Kattner, T. Dittmar and M. Witt, Advanced characterization of marine dissolved organic matter by combining reversed-phase liquid chromatography and FT-ICR-MS, Mar. Chem., 2008, 111, 233–241, DOI:10.1016/j.marchem.2008.05.008.
- J. A. Hawkes, P. J. R. Sjöberg, J. Bergquist and L. J. Tranvik, Complexity of dissolved organic matter in the molecular size dimension: insights from coupled size exclusion chromatography electrospray ionisation mass spectrometry, Faraday Discuss., 2019, 218, 52–71, 10.1039/C8FD00222C.
- B. P. Koch, G. Kattner, M. Witt and U. Passow, Molecular insights into the microbial formation of marine dissolved organic matter: recalcitrant or labile?, Biogeosciences, 2014, 11, 4173–4190, DOI:10.5194/bg-11-4173-2014.
- P. Herzsprung, N. Hertkorn, W. von Tümpling, M. Harir, K. Friese and P. Schmitt-Kopplin, Understanding molecular formula assignment of Fourier transform ion cyclotron resonance mass spectrometry data of natural organic matter from a chemical point of view, Anal. Bioanal. Chem., 2014, 406, 7977–7987, DOI:10.1007/s00216-014-8249-y.
- T. Kind and O. Fiehn, Seven Golden Rules for heuristic filtering of molecular formulas
obtained by accurate mass spectrometry, BMC Bioinf., 2007, 8, 105, DOI:10.1186/1471-2105-8-105.
- E. L. Schymanski, J. Jeon, R. Gulde, K. Fenner, M. Ruff, H. P. Singer and J. Hollender, Identifying Small Molecules via High Resolution Mass Spectrometry: Communicating Confidence, Environ. Sci. Technol., 2014, 48, 2097–2098, DOI:10.1021/es5002105.
- S. Sandron, A. Rojas, R. Wilson, N. W. Davies, P. R. Haddad, R. A. Shellie, P. N. Nesterenko, B. P. Kelleher and B. Paull, Chromatographic methods for the isolation, separation and characterisation of dissolved organic matter, Environ. Sci.: Processes Impacts, 2015, 17, 1531–1567, 10.1039/C5EM00223K.
- J. Raeke, O. J. Lechtenfeld, M. Wagner, P. Herzsprung and T. Reemtsma, Selectivity of solid phase extraction of freshwater dissolved organic matter and its effect on ultrahigh resolution mass spectra, Environ. Sci.: Processes Impacts, 2016, 18, 918–927, 10.1039/C6EM00200E.
- E. E. Lavonen, D. N. Kothawala, L. J. Tranvik, M. Gonsior, P. Schmitt-Kopplin and S. J. Köhler, Tracking changes in the optical properties and molecular composition of dissolved organic matter during drinking water production, Water Res., 2015, 85, 286–294, DOI:10.1016/j.watres.2015.08.024.
- X. Liu, Y. Lin, T. Ruan and G. Jiang, Identification of N-Nitrosamines and Nitrogenous Heterocyclic Byproducts during Chloramination of Aromatic Secondary Amine Precursors, Environ. Sci. Technol., 2020, 54, 12949–12958, DOI:10.1021/acs.est.0c02142.
- X.-F. Li and W. A. Mitch, Drinking Water Disinfection Byproducts (DBPs) and Human Health Effects: Multidisciplinary Challenges and Opportunities, Environ. Sci. Technol., 2018, 52, 1681–1689, DOI:10.1021/acs.est.7b05440.
- E. D. Wagner and M. J. Plewa, CHO cell cytotoxicity and genotoxicity analyses of disinfection by-products: An updated review, J. Environ. Sci., 2017, 58, 64–76, DOI:10.1016/j.jes.2017.04.021.
- E. E. Lavonen, M. Gonsior, L. J. Tranvik, P. Schmitt-Kopplin and S. J. Köhler, Selective Chlorination of Natural Organic Matter: Identification of Previously Unknown Disinfection Byproducts, Environ. Sci. Technol., 2013, 47, 2264–2271, DOI:10.1021/es304669p.
- J. Sanchís, A. Jaén-Gil, P. Gago-Ferrero, E. Munthali and M. J. Farré, Characterization of organic matter by HRMS in surface waters: Effects of chlorination on molecular fingerprints and correlation with DBP formation potential, Water Res., 2020, 176, 115743, DOI:10.1016/j.watres.2020.115743.
- X. Liu, L. Chen, M. Yang, C. Tan and W. Chu, The occurrence, characteristics, transformation and control of aromatic disinfection by-products: A review, Water Res., 2020, 184, 116076, DOI:10.1016/j.watres.2020.116076.
- J. Jiang, J. Han and X. Zhang, Nonhalogenated Aromatic DBPs in Drinking Water Chlorination: A Gap between NOM and Halogenated Aromatic DBPs, Environ. Sci. Technol., 2020, 54, 1646–1656, DOI:10.1021/acs.est.9b06403.
|
This journal is © The Royal Society of Chemistry 2023 |