DOI:
10.1039/D1VA00039J
(Critical Review)
Environ. Sci.: Adv., 2022,
1, 620-661
Fluoride contamination, consequences and removal techniques in water: a review†
Received
6th December 2021
, Accepted 5th September 2022
First published on 28th September 2022
Abstract
Fluoride contamination has created a drinking water crisis globally. At low concentrations, its presence is essential; however, it becomes toxic to human beings upon consumption of more than 1.5 mg L−1 in mainly contaminated drinking water due to geochemical reactions and geological or anthropogenic factors. To better understand the toxicity of fluoride, in this study, we examine the recent research on the possible negative consequences of excess fluoride on diverse species. A high fluoride concentration in drinking water cause skeletal fluorosis and long-term kidney, brain, thyroid, and liver damages. This review also focuses on the different techniques for the defluoridation of water, such as electro-coagulation, adsorption, membrane processes, etc., and compares their adsorption capabilities under various situations, while their changes in the literature are reviewed. Furthermore, we present the advantages and disadvantages of different methods and conclude that each technique has shortcomings, with no single approach fitting all aspects. The condition of water pollution with fluoride and recently created technology to remove fluoride from water is evaluated, although research on fluoride contamination of water resources has been reviewed in the literature. Alternatively, this study also examines fluorosis mitigation strategies in the global and Indian settings and existing physicochemical and biological mitigation approaches. Also, the research and development results in fluoride clean-up are reviewed. Specifically, the following topics will be covered in this review: (1) fluoride contamination status, (2) consequences of fluoride contamination in drinking water on human health, and (3) current defluoridation technology.
Environmental significance
Fluoride may be beneficial or harmful to human health, depending on its concentration and the amount of fluoride ingested over time. According to the WHO and BIS, the permissible limit of fluoride in drinking water ranges between 1.0 and 1.5 ppm. To date, many defluoridation techniques have been developed, but no one technique has been found to be competent, unassailable, and competitive enough to be widely implemented. An extensive analysis through the archives of defluoridation research indicates that significantly few endorsed sustainable solutions have been developed thus far. On a large scale, fluoride treatment is costly, and thus local governments and communities should focus on being aware and educated to provide satisfactory and reasonable remedial technology.
|
1. Introduction
Drinking water scarcity is now a global challenge in urban and rural areas, where more than 1.5 billion people directly depend on groundwater for drinking purposes worldwide.1 In the last few years, groundwater has been considered to meet domestic needs and agricultural and industrial requirements, resulting in a drastic change in the chemical composition of underground water.2 Pollutants originating from various sources can be classed as organic, inorganic, bacteriological, etc., which enter aquifers, creating a drinking water crisis. The World Health Organization (WHO) considers that fluoride is one water contaminant, adversely affecting human health.3 Drinking water is measured as the critical fluoride carrier in the human body, where the digestive system absorbs 90% of the fluoride intake through water.4
Some rocks such as fluorite, biotite, topaz, fluorapatite, cryolite hornblende, and muscovite contain fluoride-rich minerals, which release fluoride present in them after interacting with water.5 The fluoride ion concentration available in groundwater is dependent on various factors, which include the physio-chemical condition of the aquifer from where the groundwater is extracted,6 intensity of rock weathering, depth of water in the aquifer,7 acidity and porosity of soil and rocks, interaction between different chemical elements, temperature in the surrounding region,8 mineral composition of rocks, and the geochemistry of the groundwater.9 In arid and semi-arid regions, the concentration of fluoride in the groundwater is maximum. However, most of the world's groundwater is observed to cross the fluoride concentration standard limit, as suggested by the WHO.10 According to the WHO guideline, the concentration of fluoride in drinking water should be in the range of 0.5 to 1.5 mg L−1, which is generally safe.11 However, the amount may vary between 0.5 to 1.0 mg L−1 depending on climatic variables such as temperature.12 Regarding the two climatic conditions, hot and cold climates, the maximum fluoride concentration suggested by the WHO is 1 and 1.5 mg L−1, respectively,13 which accounts for the higher water consumption in the hotter climate than in the colder weather.14 The national standard limit that meets the maximum fluoride value in drinking water is reported to be 1.0 mg L−1. If the fluoride level crosses the limit of 1.0 mg L−1 by any means, either anthropogenic or geogenic sources, the groundwater will be classified as fluoride-polluted groundwater. In our country, the practical limit for fluoride in drinking water is recognized to be 1 mg L−1. However, the concentration range of fluoride in drinking water varies according to different organizations such as the WHO, Bureau of Indian Standards (BIS), Indian Standard Institution (ISI), Indian Council of Medical Research (ICMR), and others, as listed in Table S1.† It has been observed that a large portion of the population globally (mainly >200 million) depends on drinking water with a concentration of fluoride greater than the safe limit described by the WHO, which is 1.5 mg L−1. Fish and tea contain a high fluoride content, and hence members of the population eating this food are more prone to fluoride exposure.15
Consequently, studying the sustainability of excessive fluoride in groundwater and its hazardous impact on people is critical worldwide. Herein, we aim focus on the severity and seriousness of this issue by analyzing the current studies and presenting information on the exclusive and extraordinary Indian research on the effect of a higher natural concentration of fluoride in groundwater and the public scientifically. Furthermore, we aim to dissect the origins, bioavailability, and geochemistry of fluoride and its worldwide rank, focusing on human health consequences.
2. Fluorine: chemical profile
Fluorine (F2) is a halogen group member in the periodic table. It is a gas with a corrosive nature and has a characteristic pale, yellow-green colour. It has high reactivity and electronegativity. Fluoride is obtained as a reduced form of fluorine, which is a monovalent ion having a charge of −1, like other halides. It possesses distinct properties in compound form compared to other halides. It resembles hydroxide ions both structurally and chemically. Both the fluoride ion (F−) and bi-fluoride ion (HF2−) are obtained as a result of a solution of inorganic fluoride in water.16 Some inorganic fluorides without insignificant hydrolysis are water-soluble. Due to the smaller radius/charge ratio of fluoride, it has a high solvation effect, and also in comparison to chloride and other halides, fluoride appreciable differs in terms of reactivity. Naked fluoride refers to fluoride ions that are almost relatively unsolved and behave as a solid Lewis base.17 For the detection of the fluoride and its compound fluorine-19, nuclear magnetic resonance (19F NMR) spectroscopy is used.
3. Sources of fluoride
Natural resources and manufactured-generated industrial pollutants are the primary sources of excessive fluoride in the environment. Fluoride is naturally added to ground and surface water by fluoride-rich natural rocks and soil that water interacts with them. Because of the deterioration or leaching of fluoride-bearing rocks during the percolation of water into the earth, groundwater becomes more contaminated than surface water, as shown in Fig. S1.† The rate of evaporation, the length of time water spends in aquifer zones, and intense and long-term irrigation are all variables that influence how much fluoride is added to groundwater. The fluorine concentration in some mineral rocks is shown in Fig. S2.† Fluorine (F2) is a greenish diatomic gas, which owing to its highly reactive nature, seldom occurs in its pure gaseous form except in a few industrial operations.18 The chemical, glass, and ceramic industries, coal power stations, semiconductor manufacturing units, electroplating, brick and iron industries, aluminum smelting facilities, and beryllium extraction operations are some of the sectors that contribute the most fluoride-rich effluent. Fluoride is also introduced into the human body via everyday items such as toothpaste, mouthwash, medicines, cosmetics, and chewing gum.
3.1 Paths of fluoride uptake
Water, medicines, food, air, and cosmetics products are vulnerable sources of fluoride absorption. Water and other food sources are the most common sources of ingested fluoride.
3.1.1 Water.
Fluoride is found in water as a fluoride ion and has a concentration of about 625 mg kg−1 in the crust of Earth. Fluorosis in humans is mainly induced by consuming fluoridated water. Fluoride may be found in various concentrations in all-natural water sources. The geological, chemical and physical properties of aquifers, soil and rock acidity and porosity, temperature of the corresponding areas, the depth of wells, and the impact of other chemicals influence the fluoride concentration in groundwater. The fluoride concentration present in drinking water is minimal, while in seawater, it is approximately 1.3 parts per million (ppm). The range of fluoride in the freshwater supply generally varies between 0.01–0.3 ppm, whereas in the ocean it is in the range of 1.2–1.5 ppm.19 The solubility of fluoride in water limits its concentration to 3.1 mg L−1 in the presence of calcium, which has a concentration of 40 ppm. If calcium is not present in the solution, then its higher concentration is also stable. Hence, the groundwater in regions having fluoride-bearing minerals and calcium-poor aquifers is expected to have a higher fluoride concentration. The fluoride concentration is also expected to increase in groundwater where cation exchange of sodium for calcium occurs. On the ground, fluoride-rich bands extend from Syria to Libya, Egypt, Jordan, and Kenya, and from Turkey to China, Northern Thailand, and India.20 The fluoride concentration in freshwater sources is shown in Fig. S3† (ppm in percent),21 and the fluoride concentration in minerals and soils (mg L−1 in percent) is shown in Fig. S4.†22
3.1.2 Foods and beverages.
Fluoride is found in almost all foods in tiny amounts. The amount of fluoride in food is determined by the soil, water, and fertilizers used for irrigation and agriculture. Compared to water and soil, the effect of fluoride entering the body via food and drinks is smaller. Various food items such as wheat, cabbage, tea, carrots, spinach, and some drinks contain fluoride, which enters the human body.23 The fluoride in these products is most likely due to the use of fluoridated water from the soil or fertilizer for food and beverage production. Tea plants absorb a high content of fluoride, with 97% of it accumulating in their leaves.24 Tea leaves have a fluoride concentration of about 97% that of the soluble fluoride in the soil, and it also has 2 to 7 times the full amount of fluoride in the soil.25 The fluoride content in regular strength instant tea in distilled water is 3.3 ppm,26 which causes dental fluorosis.25 Tibetan children exhibit dental fluorosis as a result of drinking brick tea, which has a high fluoride content of 493.2–1000 mg kg−1 compared to black tea (23.6–52.1 mg kg−1) and green tea (232–240 mg kg−1).27 The fluoride concentration in tea beverages from India, Tibet, and China range from 1.55–3.21 ppm,28 2.59 ± 1.73 ppm,29 and 1.60–7.34 ppm, respectively.25 Fig. S5(a) and (b)† show the specifics of fluoride-rich foods.
3.1.3 Drugs.
Long-term usage of some medications, such as aspirin, has been linked to fluoride toxicity. Sodium fluoride is used to cure osteoporosis, niflumic acid is used to treat rheumatoid arthritis, and a fluoride-based mouth rinse is used to prevent cavities. Fluoride supplements containing inorganic fluoride in drinking water, pills, and other medications prevent tooth decay. The anti-inflammatory drug niflumic acid is used to treat rheumatoid arthritis. Sodium fluoride is a fluoride salt that is used in mouthwash to prevent cavities in the mouth.
3.1.4 Air.
Fluorides are extensively distributed in the atmosphere due to the industrial manufacture of phosphate fertilizers, manufacture of aluminum, coal ash from coal combustion, and volcanic activity. However, air exposure only accounts for a tiny portion of the overall fluoride exposure.30 In comparison to non-industrial regions, fluoride exposure in the air is higher in industrial zones. In parts of Morocco and China, there is a large amount of fluoride in the air.31 In particular Chinese regions, the indoor burning of high-fluoride coal for cooking produces 16 to 46 g cm−3 of fluorides in the air.32 Fluorosis affects ten million individuals in China due to the burning of high-fluoride coal.33
3.1.5 Cosmetic.
Fluorides are present in daily use goods such as mouthwash, toothpaste, and cosmetics. Raw materials such as calcium carbonate, talc, and chalk are used for manufacturing these goods, increasing the fluoride levels between 800 and 1000 ppm. In fluorinated brands, fluoride is added in the range of 1000 to 4000 ppm. Various products that contain fluorides are employed to minimize dental decay in children. They include toothpaste with 1.0 to 1.5 g kg−1 fluoride, fluoride solution, and fluoride gels, corresponding to the topical treatment between 0.25 and 24.0 g kg−1 of fluoride, and fluoride tablets with around 0.25, 0.50, and 1.0 mg fluoride per tablet, which are responsible for direct fluoride contact in the children. It was estimated that toothpaste swallowed by a few children had nearly 0.50 or 0.75 mg of fluoride per day in one child.34
3.1.6 Soil.
The fluoride levels in soil typically range from 200 to 300 parts per million.35 Fluoride is not easily leached from soils because it is held in the soil via solid through interactions with the various components present in the soil.36 The amount of fluoride in the soil increases with the depth, with just 5% to 10% of the complete fluoride inside soil being water-soluble.37 The chemical form, deposition rate, soil chemistry, and temperature influence the destiny of inorganic fluorides discharged into the soil, where most fluorides in acidic soils with pH < 6 are present in complexes related to aluminium or iron or on the displacement of hydroxide with the surface of the clay, fluoride bonds to it. The pH and fluoride concentration have a significant impact on the adsorption of fluoride, which is most noticeable between pH 3 and 4 and diminishes at pH 6.5.36 The presence of Cl−, SO24−, F− and NO3− in groundwater is caused by fertilizer application under heavy irrigation. Groundwater from irrigated fields and soils has a higher fluoride concentration owing to alkalinization.
3.1.7 Humans.
According to the research by Watanabe et al.,38 fluoride and hydrofluoric acid are absorbed via the skin in human beings and animals. Fluoride is absorbed and quickly transported throughout the body through blood, with approximately 99% of it ending up in bones and teeth.39 Fluoride is absorbed from the stomach through an inert diffusion procedure, which is inversely proportional to pH,40 but quickly absorbed from the small intestine following gastric emptying.41 Fluoride absorption is reduced with the availability of a calcium-rich diet and contact with CaCO3.42 In soft tissues, there is no fluoride accumulation, although hydrogen fluoride may penetrate the intracellular fluid of soft tissues.43 The fluoride concentration in soft tissues reflects the fluoride concentration in the blood. It is highly concentrated in the renal tubules, at a concentration higher than that in plasma.44 Due to its higher contact with fluoride, the kidney may be a possible location and target of chronic fluoride damage.45 The placenta may regulate fluoride transfer from maternal blood to fetal blood in humans,46 while fluoride is only weakly transferred from plasma to milk.47 Human milk has been shown to have fluoride levels between 5 and 10 g L−1.48 Saliva excretes around 1% of ingested fluoride or less,49 where the saliva fluoride concentrations seem to reflect the plasma fluoride levels.50 Low amounts of fluoride were found in sweat, according to Henschler et al.,51 which were around 20% that of the plasma levels. Fluoride excretion by the kidneys accounts for 35% to 70% of the fluoride consumed in adults.52 Consequently, indicators of acute fluoride exposure may be seen in the urine, plasma, and saliva. The fluoride concentrations in drinking water and the level of fluoride in the clippings of a fingernail are directly related, as shown by studies in Hungarian53 and Brazilian children,54 which suggested that the levels of fluoride in the fingernails are a reliable biomarker of direct contact with it, and thus they are not well consistent for plasma or bone. However, the level of fluoride in the nails reflects its consumption three to six months before.55 Adults living in regions with 1.0 mg L−1 fluoride in the water have an average dietary intake of 0.02–0.048 mg per kg per day, whereas those living in places with less than 0.3 ppm fluoride in the water have an average dietary intake between 0.004 and 0.014 mg per kg per day. In regions with fluoridated water, that from food consumption by children varies from 0.03 to 0.06 mg per kg per day, whereas in areas without fluoridated water, it is 0.01 to 0.04 mg per kg per day.56 Infants bottle-fed with fluoride water reconstituted milk formula consume between 0.12 and 0.18 mg per kg per day. The total fluoride intake for babies with an average weight of 8.1 kg at six months is between 1.0 and 1.5 mg per day, which is an adult dose.57 In humans, Li et al.58 proposed the lowest observed-adverse-effect level (LOAEL) of 0.25 mg fluoride per kg per day and no-observed-adverse-effect limit (NOAEL) of 0.15 mg fluoride per kg per day, but these values are presently under dispute.
3.1.8 Other sources.
The sources of fluoride in the environment include industrial plants, aluminum smelters producing glass brick, hydrofluoric acid, tile works and phosphate fertilizer plastic factories, textile dyeing, and industries that consume high sulfur non-coking coal for thermal power. Currently, high-tech companies developing semiconductors and integrated circuits produce significant fluoride-containing industrial wastewater.59 Cigarettes, which contain an average of 236 ppm fluoride, play a significant role in human fluoride consumption.60 Teflon-coated cookware may potentially increase the fluoride absorption in humans. The fluoride concentrations in Teflon-coated cookware is as high as almost 3 ppm, whereas it is lower in aluminum cookware. Also, the fluoride concentrations are high in stainless steel and Pyrex ware, although to a lower extent.61 In normal and high quantities, fluoride in water may cause aluminum to leach from cooking utensils and copper from pipework.55
4. Contamination in ground water
The contamination of groundwater with fluoride is now one of the most significant global issues, given that this contamination is natural and inevitable. Fluorosis is an endemic disease in tropical climates but only to a certain extent. A high fluoride concentration in water in extensive geographical belts is associated with (1) volcanic rocks, (2) marine origin sediments in mountainous regions, and (3) granitic and gneissic rocks. This contamination occurs due to two main factors, which are reported to be geogenic62 and anthropogenic sources.63
4.1 Geogenic source
Fluoride is present in various rocks in the Earth's crust, posing an average value of 625 mg kg−1.64 The increase in the fluoride content in groundwater is attributed to several geological processes. Geothermal springs, volcanic activities, tectonic processes, weathering, and other geological processes involving rock and water contact are the main routes for fluoride contamination.64 The fluoride concentration in water is positively associated with ions such as chloride and phosphate, whereas that with the calcium ion is negative.65 The generation of water with the loss of water from the system via evaporation has been studied using isotopic data such as carbon (C), oxygen (O), and hydrogen (H) isotopes. Also, it was determined how various aquifers are connected and how climate influences fluoride concentration.66 After much research, it has been found that the geogenic sources of fluoride contamination are the main reason for population exposure due to the intake of groundwater polluted with fluoride.67 Several minerals such as fluorite, topaz, and their parent rocks including granite, basalt, and shale release fluoride in the groundwater.68 The mobilization of fluoride through the leaching of rocks and over-utilization of groundwater is the root cause of fluoride contamination.69 Fig. S6† depicts the different rocks and their fluoride content (mg kg−1).70 Soil and rocks are natural sources of fluoride. Moreover, natural leaching, which occurs during the percolation of water through the soil and rocks, plays an essential role in groundwater contamination. There are also some indirect ways by which groundwater contamination occurs, including storage of water between soil and rocks for a long time, evaporation of water, and use of irrigation systems for an extended period. The fluoride ion can occupy the hydroxide ion site by replacing it in the mineral structure given that these two ions are negatively charged and are almost similar in size.71 The modification of rock minerals and discharge of the dissolved compound occur via geochemical processes due to the exposure of rocks to weather agents such as water. The dissolution of minerals containing fluoride in rocks is accelerated by temperature and residence time.72 When the alkalinity of groundwater is high, hydroxyl ions quickly replace the fluoride ions in minerals such as mica, elite, and amphiboles.73 Moreover, many bicarbonate and sodium minerals enhance the concentration of fluoride in the groundwater.74 The possibility of fluoride dissolution increases when calcium-rich groundwater changes to sodium-rich groundwater.75
Under average temperature and pressure conditions, hydrogen fluoride and sodium fluoride are sufficiently soluble, whereas calcium and magnesium fluoride are poorly soluble. The hydraulic conductivity of aquifers, groundwater circulation, and various other geochemical processes regulate the advancement of fluoride concentration in groundwater76 and F− bearing minerals.77 After a detailed study, it was found that the fluoride concentration also changes with a change in the groundwater level. The presence of clay also impacts the dissolution of fluoride in the groundwater because it can reduce the hydraulic conductivity and enhance the time of residence of water in aquifers.78 Fluoride with a content of almost 295 ppm is also present in coal.79 In humid areas, the fluoride level is less prominent because they receive massive rainfall, which finally dilutes the fluoride level to a great extent. Volcanic eruption also contributes fluoride to groundwater. Volcanic ash containing fluoride is released during volcanic eruptions, which occur during rainfall. Moreover, due to the high solubility of volcanic ash, it readily comes in contact with groundwater. Even after the completion of volcanic activity, the fluoride concentration remains high in the surrounding water and grass for many years.80
4.2 Anthropogenic sources
Anthropogenic factors may potentially raise the fluoride level in groundwater such as the use of fluoride-containing phosphate fertilizers, rodenticides, fumigants, herbicides, and insecticides.81 Fluoride pollution may also be caused by coal combustion, fly ash, and fluoride particle emissions from the steel, aluminium, glass, and tile industries.82 Anthropogenic activities such as coal combustion, the extensive use of fertilizers containing phosphate in agriculture, and cement manufacture generally produce fluoride to a large extent.83 Industries such as semiconductor manufacturing, coal-fired power stations, and aluminium smelters release wastewater enriched with fluoride.84 Compared to natural water, the fluoride concentrations in these industrial effluents are very high, ranging between 10 and 1000 ppm.85 It has been observed that the use of fertilizers containing phosphate in an unscientific manner is one of the primary anthropogenic sources of groundwater contamination with fluoride.86 The superphosphate fertilizers used to increase agricultural productivity contribute almost 0.34 ppm of fluoride.59 The region near brick kiln industries has an elevated fluoride concentration in groundwater.81
5. Health effects
Fluoride may be helpful or harmful for humans depending on the overall concentration of fluoride consumed over a period. A deficiency in fluoride causes dental caries and weakening of the bones when its concentration is less than 0.5 mg L−1, whereas if there is an intake of more than 1.5 mg L−1, it causes fluorosis. The accumulation of fluoride more than the permissible limit leads to hazardous health issues in infants, children, and adults. The different effects of fluoride on the human body are shown in Fig. 1. Fluoride will not affect a person briefly, but it gets stored in the brain and slowly deteriorates the body with time, as shown in Fig. 2.
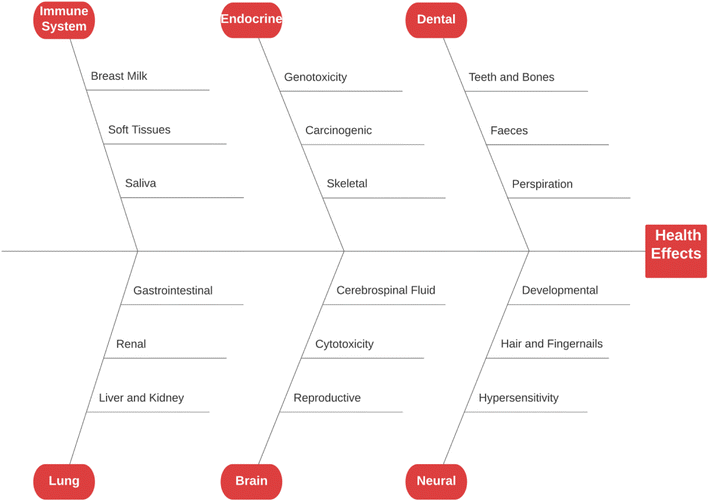 |
| Fig. 1 Schematic representation of the general health effect of fluoride. | |
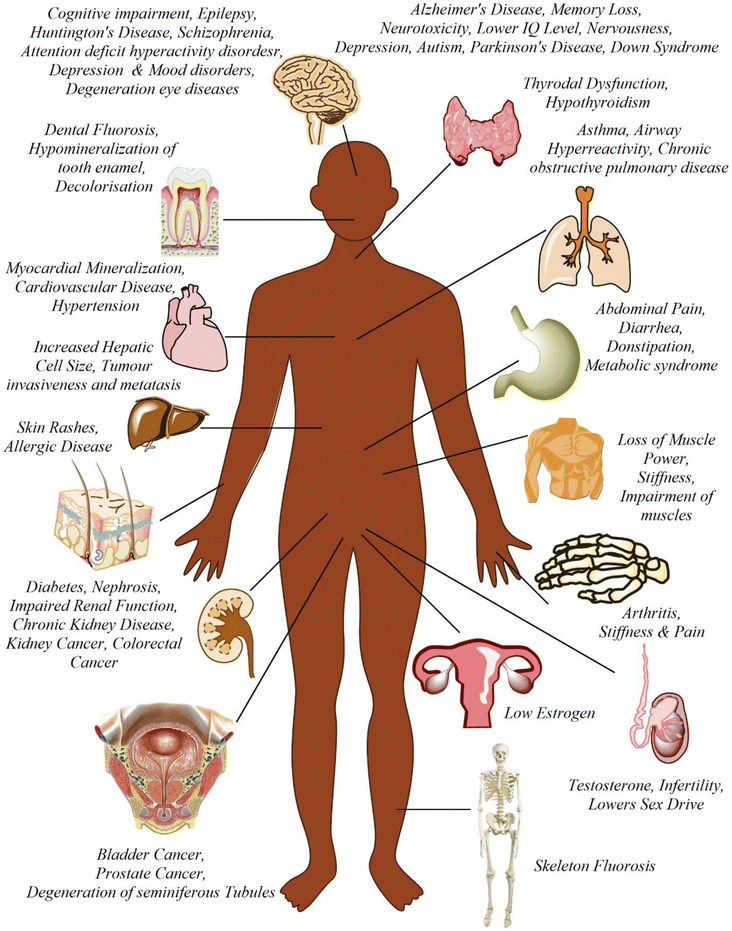 |
| Fig. 2 Effect of fluoride on human body. | |
The primary basis of fresh water is groundwater, which is the most consumed form for irrigation purposes. According to the report presented by the WHO, more than 25 countries have a concentration of fluoride more than the permissible limit, and nearly 200 million people depend on the contaminated water, which is a severe threat causing health issues to consumers. Many African countries have a higher fluoride concentration than the guideline set by the WHO of 1.5 mg L−1.87 The Asian countries with a high fluoride concentration in their groundwater include Bangladesh, China, India, Indonesia, Iran, Iraq, Jordan, Korea, Pakistan, Palestine, Saudi Arabia, Sri Lanka, Syria, Thailand, Turkey, and Yemen.88 The regions of Canada, Mexico, and the United States especially need defluoridation due to the high fluoride concentration in their groundwater.89 Even countries of Latin America, namely Argentina, Ecuador, and Peru, have high fluoride concentrations in their groundwater.90 A excessive concentration of fluoride in groundwater is a significant problem in European countries. Some regions of Germany also face this problem.91Fig. 3 displays a world map with all the countries having drinking water with fluoride.92
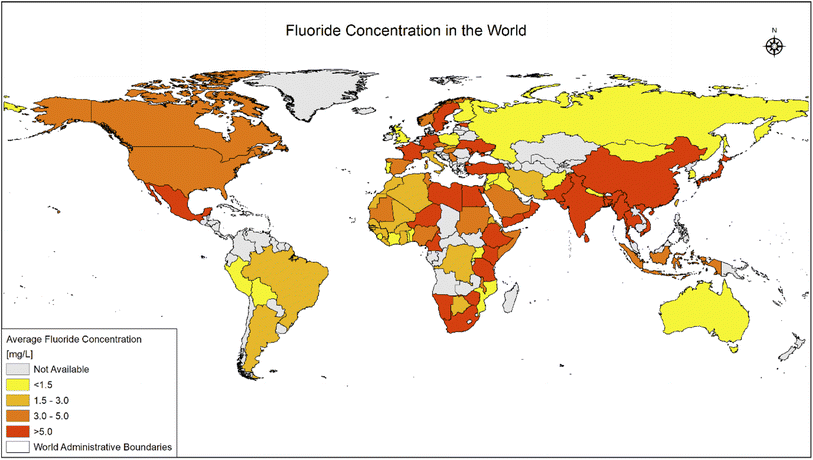 |
| Fig. 3 Map of world showing fluoride (in ppm) distribution in all countries. This map was modified or prepared based on published scientific reports.91 | |
In 1937, the Prakasam area of Andhra Pradesh reported the first incidence of fluorosis endemic in humans.93 Only four states of India, namely Andhra Pradesh, Uttar Pradesh, Punjab, and Tamil Nadu, were recognized with fluorosis patients in 1950. Currently, fluoride is present in 20 of India's 29 states, which is projected to rise,94 as shown in Fig. 4. Fig. 4 displays a map of India with fluoride in the drinking water in all districts.95 In India, just a few families with fluorosis were found in 1937. However, fluorosis was projected to affect 25 million individuals in India by the United Nations International Children's Emergency Fund (UNICEF) in 1995. Currently, fluorosis affects 66 million people in India, with 6 million children less than 14. Around 411 million individuals in 201 districts across 20 states are affected by fluoride in India, and thus are possibly in danger of fluoride poisoning.
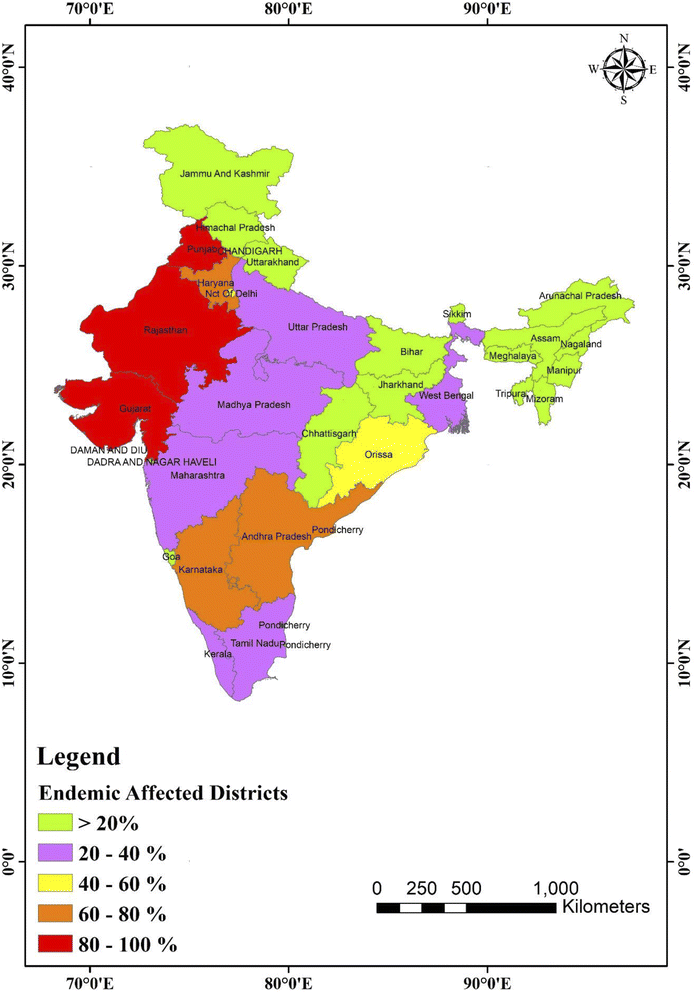 |
| Fig. 4 Map of India showing fluoride (in ppm) distribution in the different states and union territories. This map was modified or prepared based on published scientific reports.95 | |
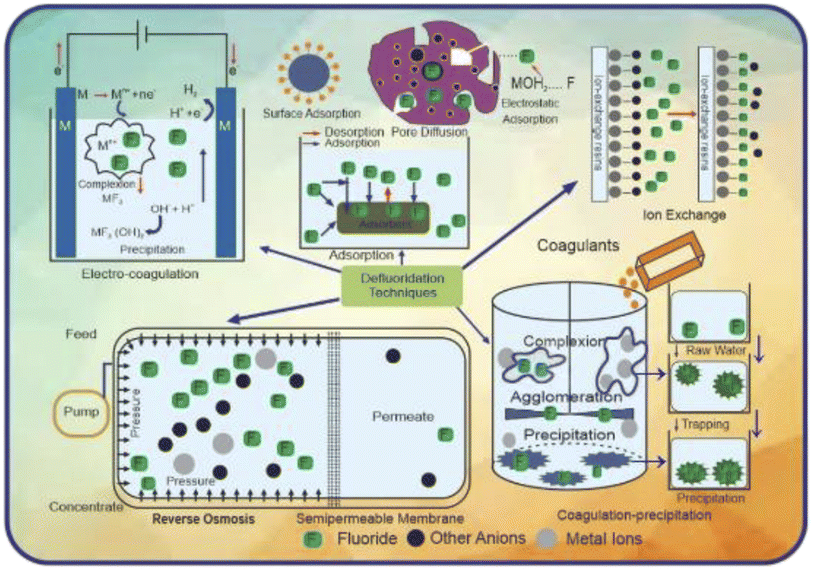 |
| Fig. 5 Various defluoridation techniques. | |
5.1 Dental
Dental caries is the world's most common issue in children. Fluoride plays a significant role in minimizing the chance of enamel fluorosis and preventing dental caries. During the developmental period of teeth, continuous exposure to fluoride helps develop resistance against dental decay and fluorosis of the enamel. The enamel is the most mineralized hydroxyapatite crystal, which is rich in carbonate, but deficient in calcium.96 To maintain the state of stable equilibrium with the fluid in the surrounding region of the crystal, a sufficient amount of ions such as Ca2+, PO43−, OH−, and F− are present. During cariogenic acid attack, the pH value in the vicinity of the tooth decreases due to the release of H+ ions from organic acids, which are formed by plaque bacteria from carbohydrates. These H+ ions are released to interact with the phosphate ions (PO43−) available in plaque fluid and reduces them to HPO42−, and finally H2PO4−. This reduction process helps to release calcium from potent tooth substances and also balances the neutrality.97 Compared to the incorporated fluoride, the smaller quantity of fluoride present in the solution in the vicinity of the tooth more effectively prevents the process of demineralization. Moreover, it also can inhibit dental caries more than the large amount of fluoro-hydroxyapatite in the enamel. This protection is ascribed to the fact that the fluoride ions present in solution are more effective to prevent caries than the fluorides present in the enamel crystals. Now, a small amount of fluoride ions is adsorbed on the crystalline surface, and a state of dynamic equilibrium is achieved between the fluoride ions in solution in the vicinity region and the adsorbed fluoride ions. Finally, this adsorption inhibits the process of demineralization.97 The presence of fluoride in the correct amount (≥0.7 mg L−1) is significant for developing dental enamel and normal mineralization of bones.98 Up to 40% of tooth decay can be prevented by fluoride.99
5.2 Teeth and bones
An essential constituent of the tooth enamel and skeleton is hydroxyapatite. The hydroxide ions replaced by fluoride form a significantly harder compound called fluorapatite. If present in a small amount, fluorapatite prevents the tooth from decaying and reinforces the tooth's enamel. However, if fluoride at elevated doses is taken for a long time, then the hydroxyapatites get converted to fluorapatite in a more significant amount, making the bone and teeth stiffer and brittle and promoting the disease called dental fluorosis. Dental fluorosis gets converted to skeletal fluorosis as the fluoride concentration increases to 3.0 mg L−1. About 65% of the endemic fluorosis, which occurs due to fluoride-contaminated drinking water, is reported in arid and semi-arid regions.100 Table S2† shows the effect of prolonged use of drinking water on human health, which is related to its fluoride content.101 Crippling skeletal fluorosis is considered one of the leading causes of morbidity in a large area of the globe. If the fluoride present in drinking water is less than 0.5 mg L−1, then the possibility of dental caries increases in children.102 If the amount of fluoride present is up to 1.5 mg L−1, it helps strengthen the skeleton. The threat of skeletal and dental fluorosis increases with an increase in the fluoride concentration in water. The most common problem encountered is the mottling of the teeth when the fluoride concentration is in the range of 1.5 to 4.0 mg L−1. When the fluoride intake crosses 6.0 mg L−1, it opens the path for multidimensional health effects, mainly dental and skeletal fluorosis.103 Drinking water containing excess fluoride is responsible for almost 65% of the total endemic fluorosis.104 Out of the total fluoride consumed by the body, its maximum amount is retained by the bones or teeth, with almost 80–90% in new-born babies and 60% in adults, and the remaining fluoride gets excreted via the urine.105 The early symptom of dental fluorosis is enamel discoloration,106 which may convert to discrete or aggregated pitting.107
In the three districts of Rajasthan, India, namely Dungarpur, Banswara, and Udaipur, a survey was conducted and it was reported that more than 21% of teenagers and 36% of adults of the area having fluoride concentration of 1.5 ppm in the drinking water were affected by dental fluorosis. Also, the fastest spreading rate (77.1%) of dental fluorosis was found in the age group of 17–22 years.108 The other effects of fluorosis are restricted joint movement, joint pain, and limb motor dysfunction. In India and some other countries, these symptoms make life very complex for people suffering from severe health issues such as paralysis.108
5.3 Neural
When present in a concentration of more than 1 mg L−1, fluoride increases the chance of neurotoxicity, which may disturb the learning and memorizing capacity. Compared to the mature brain, the developing brain is more prone to damage by toxicants, which may lead to permanent damage.109 Current research has established that the mental ability of children in high fluoride areas is relatively lower than those living in low fluoride areas.110 A comparative study was carried out between 1988 and 2008 in China to relate the intelligence quotient (IQ) level with fluoride concentration and it was found that the children living in fluoride-enriched areas have a chance of low IQ by at least five times more than the children from the region having a lower fluoride concentration.111 The excess fluoride concentration increases the lipid peroxidation level and hinders various chief neuronal enzymes.112 This study suggested that fluoride directly affects neurons, myelin, and neurotransmitters, suggesting that fluoride may directly damage the functioning of the brain.113 A characteristic study was performed to understand the correlation between neonatal neurodevelopment and fluoride intake by mothers during pregnancy. It was found that the neonatal neurodevelopment of babies was very slow when the fluoride intake by their mothers was large during pregnancy compared to the babies not exposed to an elevated fluoride concentration.114 After chronic fluoride administration, various changes occur in the blood, brain, and liver of animals, including metabolic lesions, abnormal behavior designs, and changed neuronal cerebrovascular integrity.115 When the groundwater contains fluoride of more than 10 mg L−1, it accelerates more diseases such as neurological problems, hypertension, and cancer, which become challenging for human health.116
5.4 Reproductive
Currently, the world is struggling with the growing issue of infertility, where fluoride is considered to be it is one of the main factors. Increased fluoride exposure is related to an increased luteinizing hormone (LH) level and follicle-stimulating hormone (FSH),117 decreased thyroid hormone (TH),118 reduced estrogen level (EL),119 and disturbed ratio of estrogen receptor to androgen receptor (ER/AR).120 The morphology, solidity, and metabolism of spermatozoa change in rats affected by fluorosis and their fertility was reduced by 33%.121 In a male patient affected by skeletal fluorosis, a reduced circulating testosterone concentration was observed.122 The level of potassium and sodium in spermatozoa is reduced, as fluoride enables the leakage of potassium ions.123 Due to the genotoxic effect of sodium fluoride (NaF) on mouse germ cells, the sperm abnormality increases, and the chromosomal aberrations increase in the primary testicular cells.124 When an excess amount of NaF was applied to the ovaries of albino rats, stomal congestion, ovarian follicles, and dilated blood vessels were observed.125
5.5 Liver and kidney
When the three crucial parts of the body, namely the kidney, liver, and heart, are exposed to excess fluoride concentrations for a long time, they start showing histopathological and functional changes.126 A study showed that the continuous ingestion of fluoride through the diet accelerates chronic kidney disease (CKD).127 An experiment on mice by exposing them to high fluoride showed a higher fluoride concentration in their urine, renal cell apoptosis, reduced serum glutathione peroxidase (GPx3), and increased malondialdehyde (MDA).128 Also, NaF induces hypertension by increasing the production of reactive oxygen species (ROS) and elevating the NF-kB expression in the kidney and heart.129 A person suffering from kidney problems is more susceptible to fluorosis even if fluoride is consumed in the permissible limit due to a reduction in their ability to excrete fluoride via urine.
5.6 Lung
Fluorosis is associated with asthma in laborers working in the aluminum industry.130 In most factories, the key source of fluoride pollution is the production of aluminum and phosphate.131 Studies showed that respiratory ROS production may be linked with many respiratory diseases.132 The levels of pro-inflammatory cytokines and ROS were elevated in the lungs of fluoride-treated mice, whereas there was a decrease in anti-inflammatory factors. The NrF2/keap-1 signaling pathway supports cell survival by maintaining anti-oxidant function and activity during oxidative stress. It was observed that elevated endogenous anti-oxidants, decreased ROS and number of lung lesions, and up-regulation of NrF2 occurred after mice were pre-treated with epigallocatechin gallate (EGCG).133
5.7 Immune system
Fluoride produces an adverse effect on the immune system of the body. A study showed that the immunity of silk worms is reduced upon exposure to NaF, which hinders their cellular immunity.134 This study concluded that at lower fluoride concentration (5 mg L−1), the level of cellular expression of anti-inflammatory factor IL-10 decreases in mouse macrophages. Alternatively, a greater fluoride concentration (50–75 mg L−1) helped enhance the macrophage inflammatory protein 2 (Mip 2) and pro-inflammatory cytokine IL-6 and increased ROS and reactive nitrogen species. This resulted in elevated lipid peroxidation and redox imbalance, decreasing the macrophage population.135 Moreover, fluoride ions also form a complex with magnesium and other metals, inhibiting many enzymes.136
5.8 Skeletal
An increase in the mass and density of bones and a variety of joint and skeletal complaints define skeletal fluorosis. The mechanism(s) causing skeletal fluorosis are unknown. Nevertheless, the phases of growth and development have been thoroughly characterized.137 Pain and stiffness in the backbone and hip area, joints, and increased bone density are common early signs (osteosclerosis). “Poker back” is the situation where the entire spine stiffens until it forms one continuous column of bone. Different ligaments of the spine may tend to be calcified and ossified as this illness worsens. Fluorosis causes neurological problems, muscular atrophy, paralysis, debilitating deformities in the spine and major joints, and spinal cord compression in its later stages. Water consumption, water quality, and other dietary variables influence the content of fluoride required to develop skeletal fluorosis.138 It has been indicated that skeletal fluorosis has not developed among the population in the United States (US) where the drinking water concentration is less than 4 mg L; however, those with renal impairments consuming substantial amounts of drinking water at 2–8 mg L−1 may be at risk.139
5.9 Developmental
There is a good association between the concentration of fluoride estimated in the umbilical and maternal cord with the blood plasma, as discovered by many studies, suggesting that the placenta facilitates inactive fluoride transmission from the mother to fetus.140 Studies on laboratory animals show that even at extremely high ingestion rates, detrimental developmental effects are feasible; nonetheless, the assessment of developmental problems in the human population has been inconsistent, owing to the poor study quality.141 The probable relationship between fluoride intake and the occurrence of Down's syndrome,142 particularly in children born through women below the age of 30,143 is an avenue of inquiry that merits additional exploration.
5.10 Renal
The renal system excretes most of the excessive fluoride from the body, and thus it is vulnerable when in contact with a high concentration of fluoride compared to the other organs.144 It has also been shown that it is more susceptible to fluoride poisoning than soft tissues. Moreover, only two published studies demonstrate that chronic fluoride consumption has non-carcinogenic consequences on the kidney, both of which are related to kidney stones.141 Residents in high fluoride regions in Finland, where the fluoride concentrations in groundwater surpass 1.5 mg L−1, had higher hospital acceptance rates for urolithiasis, which is commonly known as kidney stones, than residents in the other regions, according to Juuti and Heinonen;145 however, the difference was only 16%. Singh et al.146 examined over 18
700 persons in an Indian location where the fluoride levels varied from 3.5 to 4.9 mg L−1 and discovered that patients with evidence of skeletal fluorosis were 4.6 times more likely to face the issue of kidney stones. However, it was difficult to make clear conclusions given that the individuals in this study were presumably at higher risk of suffering from kidney stone development due to malnutrition.
5.11 Endocrine
Fluoride seemed to impact normal endocrine function and response in laboratory animals and human populations, although there is substantial difficulty in interpreting the findings of this study. Reduced thyroid function, increase in calcitonin activity, increase in parathyroid activity, secondary hyperparathyroidism, and poor glucose tolerance are the principal effects of fluoride on the endocrine system (type II diabetes).141 However, these impacts differ in intensity among various people, and most of them may be characterized as subclinical, given that they are not deemed harmful to human health. The link discovered by various studies, such as endemic goiter and fluoride exposure in human beings, is perhaps the most acceptable illustration of the difficulty required in considering the properties of fluoride on the endocrine scheme.147 Although these geographical correlations state the reason and resulting connection, the actual causative approaches for the thyroid action of fluoride have yet to be determined.141
5.12 Gastrointestinal
In acute fluoride poisoning, gastrointestinal symptoms such as diarrhea, vomiting, nausea, and abdominal discomfort have already been recorded.148 In animal tests, fluoride has been shown to accelerate the stomach acid output, restrict the flow of blood away from the stomach lining, and kill gastrointestinal tract epithelial cells.149 The amount of chronic fluoride consumption necessary to elicit these effects in people has not been determined, where it is possible that this threshold changes depending on other circumstances. For example, in regions with endemic fluorosis where nutrition is often poor, unpleasant gastrointestinal symptoms are prevalent,143 although equivalent amounts of fluoride exposure in the United Kingdom (UK) and the United States may not always induce the same reaction.150 Another significant aspect seems to be peak concentration rather than the time of exposure given that slow-release fluorides and calcium supplements have been shown to reduce adverse stomach effects in clinical studies when fluoride is supplied through tablets.151
5.13 Carcinogenic
An epidemiological survey aimed at determining the carcinogenic risk of chronic fluoride disclosure encountered a variety of obstacles. The most severe issues are that cancer is diagnosed every year, if not in decades, after exposure to the relevant variables when people move in and out of the research region. This often results in the miscalculation of the research group's orientation. Another challenge is the wide variety of malignancies and their probable causes, which involve independently evaluating each form of cancer. Consequently, it is not unexpected that attempts to link fluoridated water and general cancer rates have failed.152 Researchers have paid close attention to the potential that fluoride contributes to bone cancer because it is accumulated in the skeleton. Although there is controversy in applying these results to people, specific laboratory investigations of animals have found indications of increased osteosarcoma and osteoma.141 Fluoride use may also raise the risk of kidney and bladder cancer due to the propensity for hydrogen fluoride, a caustic and possibly poisonous chemical, to develop in acidic environment of urine. Grandjean et al.153 performed investigations that provide strong support for this.
5.14 Hair and fingernails
Measuring fluoride in the hair and fingernails is a typical way of assessing the overall fluoride load in the body.154 Although the fingernails reflect exposure in the last 3 to 6 months, namely short-term biomarkers, crippled fingernails definitively designate high fluoride concentrations.155 Several reports in the literature discuss the shifting decorations of current biomarkers, namely hair and nail, and sensitivity to high fluoride concentrations. The use of pins as a biomarker was initially suggested.156 According to the research, fingernails show a higher daily fluoride (3 to 6 mg L−1) intake 3.5 months after the initial exposure. Because the reference values for fluoride correlation have yet to be established, researchers are actively investigating the use of nails to predict the fluorosis levels. Although toenails, particularly the big toe, are less susceptible to exogenous fluoride, other affecting variables such as dietary habits, age, sex, and environmental factors should be addressed.157
5.15 Soft tissues
In soft tissues, the fluoride concentration is relatively low, which does not change with age. The intracellular fluoride in soft tissue and the extracellular fluoride in the fluid are easily interchangeable. During the increased uptake of fluoride, the fluoride content in soft tissue changes within a limited range and demonstrates slight fluctuation.158 The rate of fluoride deposition in soft tissue is determined by the alkalinity and blood flow velocity in the tissue.159 Thus, advanced epidemiologic research is needed to corroborate the findings of deposited fluoride possessions for soft tissue.
5.16 Cerebrospinal fluid
The blood–brain obstacle actively defends against excessive flooding of fluoride into the brain by transporting fluoride to the cerebrospinal fluid. The fluoride concentrations in brain fluid are less than half that of complete plasma fluoride.160 The fluoride concentration in the cerebral fluid increases with excess fluoride intoxication in patients with compromised blood–brain barriers.161
5.17 Urine
Fluoride reduction via urination is mainly a pH-controlled mechanism, where renal excretion rises as the urine becomes more alkaline. Reduced renal clearance of fluoride is caused by increased hydrofluoric acid (HF) reabsorption at acidic pH.162 Jarnberg et al.163 accurately detailed the renal excretion process of fluoride. Urine is formed by available blood filtration in tubular and glomeruli reabsorption, according to the mechanism. HF is reabsorbed throughout the nephrons through an ionic diffusion process, and the grade of reabsorption is proportional to the fluid pH.
5.18 Faeces
The unabsorbed portion of ingested fluoride in the gastrointestinal tunnel is about 10% of the total ingested fluoride, which is excreted in the stool. The number of other food components such as fat, protein, and fiber; the availability of divalent cations, namely Ca and Mg; age, and physiological conditions, all influence faecal excretion.164 The solubility of ingested fluoride is also an essential element that controls the F− outflow in the urine and faeces.165
5.19 Saliva
In humans, the typical fluoride concentration in the saliva is around 75% of the plasma fluoride content, suggesting that saliva can be used as a fast biomarker. Without being exposed to a high concentration of fluoride, humans exhibit salivary fluoride values in the range of 0.01 to 0.06 mg L−1. Within 15 minutes of intake, the level of fluoride in the saliva tends to rise by 15 times than its typical value, and then reverts to its average amount between 20 and 60 minutes.166
5.20 Perspiration
In people and animals with adequate sweat glands and high sweating rates, perspiration is an essential path of fluoride excretion. The fluoride concentration in human sweat is roughly 20% of the plasma fluid concentration, whereas perspiration may eliminate 13–38% of the total absorbed fluoride at high temperatures and humidity.167
5.21 Breast milk
Milk fluoride concentrations are highly correlated with the total fluoride intake.168 A good association among plasma, breast milk, and fluoride content in water was documented in several investigations.169 The fluoride level of milk in women with dental fluorosis was 0.550 mg L−1 in a current survey of 62 mothers, compared to 0.006 for mothers without dental fluorosis.170
5.22 Cytotoxicity
In mammalian cells, the excessive consumption of fluoride may cause chromosomal abnormalities and genetic mutations.171 Fluoride has a significant impact on deoxyribonucleic acid (DNA) and protein production, even at low concentrations. Excess fluoride has also been linked to changes in cell shape and DNA breakage in nucleosomes.172 The cytotoxic impact is mainly determined by the exposure intensity, frequency, and duration.
5.23 Hypersensitivity
Existing investigations in this sector provide insufficient evidence on the logical association between hypersensitivity and fluoride ion concentration. Thus, to link hypersensitivity and fluoride exposure in experimental animals, researchers often choose a high dosage, an ineffective mode of administration, or both.173 However, the results obtained may not be extended to fluoride exposure in people given that the experimental dose is disputed. Human hypersensitive response research is similarly inconclusive and contentious. Eczema, roseola, stomatitis, and gastrointestinal issues are the most frequent responses.174
5.24 Brain
Excessive fluoride consumption has been shown in several studies to impair the structure organization and function of the central and peripheral neurological systems.175 The build-up of excess fluoride in the hippocampus has also been linked to neuron degeneration, decreased aerobic metabolism, and alterations of free radical metabolism.176 Several other investigations on experimental animals have shown that traces of metal concentration in the brain and the antioxidant defense system are altered.
5.25 Genotoxicity
The word genotoxicity refers to the presence of a toxin that damages the integrity of cells by destroying their genetic contents.177 The technique and judgments used in vivo and in vitro research linked to fluoride toxicity significantly impact genotoxicity studies.178 Fluoride does not generate mutagenic alterations in the Ames assay, which is a highly reliable way to detect genotoxicity,179 despite inconsistent findings in the literature.
5.26 Other toxic effects
Fluoride may produce a toxic effect on the human body in several ways. Initially, fluoride acts on the intestinal mucosa, but later, due to hydrofluoric acid formation in the abdomen, it causes gastro-intestinal irritation or corrosive effects.180 Fluoride can also disturb lipid functioning and adipogenesis.104 Fluoride exposure is also related to the problem of obesity in children. However, the result remains inconclusive given that some researchers show a positive correlation between obesity and fluoride,181 while others reported either no effect or negative correlation between them.182
6. Fluoride mitigation knowledge
Fluoride has a variety of negative impacts on humans and plants; thus, it must be monitored and mitigated. Consequently, it is essential to take appropriate steps to minimize the concentration of fluoride in potable water from affected zones within the permissible limit. Different strategies are used to reduce the risk of fluorosis. They include using some alternate water resources, improving the nutritional status by using some specific foods in the diet, and water defluoridation.183 One attempt tried to supply treated surface water, not groundwater, at the community level as an alternative water resource. However, this method is not possible for developing countries due to the considerable expense.184 Sometimes we can go through two water systems in which the water with a less amount of fluoride can be used for household purposes such as cooking and drinking, while water containing a higher amount of fluoride can be used for other chores.185 Rainwater harvesting can also be considered another alternative.186 However, the main limitation of this method is the requirement of ample space to store rainwater and the uneven distribution of rainfall in different areas.187 Consuming foods rich in vitamin C and calcium (nutritious food) also reduces the fluoride effect to some extent.183 However, if no alternative source of water is available, then the only option left is the process of defluoridation.188 Although several defluoridation processes exist, no one solution is prosperous, safe, and cost-effective enough to be extensively used. An examination of the history of defluoridation research indicates that just a few reliable, long-term treatments have been discovered.189
Furthermore, in endemic parts of the developing world, adsorption/ion exchange and coagulation procedures are extensively utilized as fluoride removal strategies. Many nations, including India and Tanzania, utilize defluoridation treatments that are both residential and community-based at various levels. People in India and Sri Lanka have recently had a paradigm change in their attitudes about community-based water treatment schemes that use activated alumina as an electro-coagulation and sorbent. Moreover, most defluoridation methods are out of reach for people living in fluoride-affected rural regions. Other processes such as nanofiltration, electrodialysis, and reverse osmosis, can provide superior quality water, but they are costly and require a high level of technical expertise, limiting their application in fluoride removal. Fig. 5 presents a schematic diagram explaining the fundamental principles of several defluoridation procedures.
Each approach has its own set of benefits, drawbacks, and influencing variables, and it only works well under ideal circumstances. Various physical and chemical methods may accomplish defluoridation of drinkable water. Biological approaches for phytoremediation and defluoridation of the air, water, and soil employing bio-sorbents generated from plant materials and bioremediation via bacteria have also been documented.
6.1 Chemical and physical process of defluoridation
6.1.1 Ion exchange.
In this process, a material called an ion exchanger is used, where water is allowed to pass through its bed to eliminate the undesirable ions according to the following reaction: | Matrix-NR3 + Cl− + F− ⇌ matrix-NR3 + F− + Cl− | (1) |
The fluoride ion replaces the chloride ion in the resin, and the substitution process continues until every site of the resin is occupied. To regenerate the resin, it is back-washed with water containing dissolved sodium chloride salt, enabling fluoride to be replaced by chloride and start acting as an ion exchanger.190 The fluoride ion possesses higher electronegativity, which is the specific driving force to replace chloride ions in the resin. By using lanthanum, Chikuma et al.191 modified the method of fluoride removal by anion exchange. Chikuma and Nishimura192 used Amberlite IRA-400 in an aqueous solution for fluoride removal and found that the fluoride ion replaces the chloride ion present in the resin. Ho et al.193 increased the capacity of the ion exchange method through titanium oxyhydroxide. Zirconia and silica having tiny particle sizes are doped on iron oxyhydroxide, a mesoporous material, to increase its ion exchange capacity, but this process is expensive and the issue of membrane arises. Meenakshi et al.194 investigated the fluoride removal capacity of chelating Ceralite IRA 400 (CER) and Resin FR 10 (IND) and concluded that in comparison to the anion exchange resin, the chelating resin is highly selective for the removal of fluoride. The ion exchange technique has a high potential for eliminating fluoride from aqueous solutions (up to 95%). However, the resins are costly, making the treatment uneconomical although the resins may be readily renewed. Furthermore, the regeneration process generates a lot of fluoride-loaded waste, which is a downside of the technique.195
6.1.2 Precipitation/coagulation.
In the early 1930s, researchers tried to develop a technique for fluoride removal from water, which will be economical and sustainable. This process involves the removal of contaminants from water, thereby reducing turbidity. In the coagulation process, a particular chemical called a coagulant is used to destabilize the tiny particles present in water. The different materials used as coagulants to remove fluoride from water include aluminum, iron, alum, lime, zeolites, silica gel, sodium aluminate, ferric chloride, and silica gel.196 Among them, alum and lime are the most commonly used. The best example of fluoride removal from the coagulation/precipitation method is the Nalgonda technique. This method involves the addition of aluminum salts, bleaching powder, and lime to water contaminated with fluoride, in six consecutive steps, as follows: coagulation/flocculation, disinfection, filtration, rapid mixing, sedimentation, and sludge concentration.196 This whole process is carried out in the following sequence. (a) Formation of insoluble flocs of aluminum hydroxide, (b) sinking of sediments at the bottom, and (c) co-precipitation of fluoride and bleaching powder.197 However, considering that the efficiency of the Nalgonda technique for water de-fluoridation is approximately 70%, it is not suitable for the treatment of water with elevated fluoride concentrations. Also, due to the high cost of alum, this method is expensive.196 In the precipitation method, the fluoride in calcium fluoride is precipitated from water.196 To precipitate the fluoride, phosphate, and calcium are used, followed by the process of filtration by employing bone char pre-saturated with fluoride ions. A medium containing saturated bone charcoal behaves like a catalyst for fluoride precipitation in the form of fluorapatite and CaF2.198
6.1.3 Electrocoagulation.
Electro-coagulation is a simple and effective method for the removal of flocculating agents obtained by the electro-oxidation of a sacrificial anode, in general, consisting of aluminum and iron. This process does not involve any chemical flocculants or coagulant and help in reducing the amount of sludge to be disposed. Three fundamental processes are involved in this method, namely, electrochemistry, hydrodynamics, and coagulation. The electrocoagulation reactor consists of an electrolytic cell having a cathode and anode.199 Ghosh et al.200 proposed the removal of fluoride through an electrocoagulation process from drinking water with a fluoride concentration ranging between 2 to 10 mg L−1, employing both mono and bipolar connections. They noticed that in comparison to mono-polar connection, bipolar connection prefers fluoride removal. Under the bipolar connection, the final suggested breaking point of fluoride (1 mg L−1) was achieved at 625 A m−2 within an interval of 30 minutes. Moreover, for mono and bipolar connection, the operational costs are 0.38 and 0.62 US $ m−3, respectively. Vasudevan et al.201 observed the effect of a direct current and alternating current on the removal of fluoride from water, taking an aluminum alloy as the cathode and anode. Due to the direct current, an impermeable oxide layer was developed on the cathode surface, where anode corrosion takes place due to oxidation. This reduced the efficiency of the method given that the current between the cathode and anode could not be controlled. However, this issue could be removed using an alternating current. It was observed that at pH 7.0, the current density of 1.0 A dm−2, by employing aluminum alloy electrodes, the removal efficiencies of the alternating and direct current were 93% and 91.5%, respectively. The energy consumption was 1.883 and 2.541 kW h kL−1, respectively. Moreover, a temperature study revealed that the process of electrocoagulation was spontaneous and exothermic.
6.1.4 Reverse osmosis.
In the reverse osmosis process, a tank is separated into two parts through a semi-permeable membrane. With the aid of hydraulic pressure, the contaminated water can move from one side to the other through a semi-permeable membrane. Water and small impurities can pass through the membrane, but salts and many other contaminants cannot pass through the semi-permeable membrane.202 The speed of the reverse osmosis process can be increased by increasing the osmotic pressure on the contaminated side, enabling the water to move through the semi-permeable membrane from the impure side to the fresh side.203 This removal efficiency by this process reaches 90% or more in the absence of hindrance due to other ions.204 The two critical parameters that affect the membrane performance are pH and temperature, affecting the fluoride removal efficiency. For water purification, the membrane is selected based on cost, temperature, pressure, recovery, salt rejection, and nature of water to be tested.205 In the reverse osmosis process, all the ions present in the water are removed, which is its major drawback. For the average growth of the body and other metabolic processes, minerals are necessary. However, for the fulfilment of minerals, the treated water has to undergo re-mineralization, making it expensive.
6.1.5 Nanofiltration.
Among the various membrane processes, because of the high specific membrane selectivity of nanofiltration, it is regarded as the perfect membrane technique for removing fluoride.206 The pore size of nanofiltration membranes is slightly more significant than that of reverse osmosis membranes, enabling the easier movement of both the solvent and solute. Consequently, low pressure is required and the flow is fast in nanofiltration.207 It was observed that in fluoride removal and desalination of some brackish water, nanofiltration gave a superior result compared to other membrane techniques.208 It was observed that between two commercial nanofiltration membranes, namely NF-270 and NF-90, NF-270 decreased the fluoride level from 10 to 1.5 mg L−1, while NF-90 decreased the level from 20 mg L−1 to 0.5 mg L−1.209 Also, it was reported that the fluoride level could be reduced from 417.9 mg L−1 to below 1.5 mg L−1 by using BW30 and NF-90 membranes.210
6.1.6 Adsorption process.
The adsorption method involves particle accumulation from the bulk segment to the solid or liquid segment and is treated as a boundary phenomenon.211 On the surface of adsorbents, a substance layer is formed, which is known as adsorption. Thus, the method of adsorption can be explained in the following steps. Firstly, the accumulation of a fluoride layer across the surface of adsorbent particles from the heterogeneous solution. The second step involves the adsorption of fluoride ions on the surface of the adsorbent particles. The last step involves intra-particle diffusion, where the adsorbed fluoride shifts to the inner surfaces of the porous adsorbent materials.212 Specifically, the quantity of fluoride adsorbed on the adsorbent surface per unit mass of the adsorbent reflects the water purification extent from fluoride contamination.213 The various types of adsorbents are shown in Table S3.† The extent to which an absorbent is efficient in removing fluoride depends on factors such as the initial concentration of fluoride, the type of adsorbent used, pH of the water, the existence of interfering ions, and the time of contact.214 Among the different methods for defluoridation, adsorption is considered the most effective for a small population due to its more straightforward operational process, simple design, presence of a variety of adsorbents, and comparatively low setup cost.215 However, some adsorbents are very expensive, while some of them for defluoridation are not fit technically in rural areas.216 Also, the use of some adsorbents is limited due to their inadequate removal capacity. Active alumina is used on a large scale as an adsorbent at the domestic and community levels to remove fluoride from drinking water. It was noted that the use of active alumina as an adsorbent involves both the adsorption process and ion exchange method.217 The use of active alumina effectively removes fluoride from water; however, it can also cause adverse health effects. The complex compound formed between aluminum and fluoride is recognized as the reason for Alzheimer's disease and is also responsible for some health complexes.218 Ferric hydroxides, [Fe(OH)3], as granules, are a mixture of poorly crystallized iron oxide (FeOOH), showing a positive result in removing fluoride from drinking water.219
The adsorption capacity, fluoride removal effectiveness under ideal experimental circumstances, and regeneration capacity of important adsorbents are summarised below.
6.1.6.1 Aluminium-based adsorbents.
Alumina has been extensively investigated and recognized as the most efficient adsorbent for water defluoridation.220 It must be activated to be an efficient adsorbent. Steady or quick gibbsite pyrolysis or gibbsite-comprising materials such as flash calcination produces activate alumina (AA).221 Because highly porous aluminum oxide possesses a high surface area, activated alumina is an appealing adsorbent with higher fluoride removal capability than other adsorption media. The cationic networks across the alumina crystal generate a positive charge, which attracts anionic species. The adsorption capacity of activated alumina is affected by its crystalline form and pH of the water. The development of fluoride complexes is considered a significant factor in fluoride adsorption through activated alumina from solution, as represented below:
The activated alumina may not be soluble in the aqueous solution comprised of fluoride ions, resulting in the formation of a variety of aluminum and fluoride and hydroxyl-aluminum complexes. The steps of the equilibrium equation to represent the Al–F complexes are as follows:
The formation of complexes of the specific aluminum ions together with the hydroxyl ions in the solution is represented as follows:
| Al3+ + 2OH ⇌ Al(OH)2+ | (11) |
| Al3+ + mOH ⇌ Al(OH)+3−m | (13) |
The solution pH is responsible for the adsorption of fluoride on activated alumina due to the electrostatic interactions between the alumina surface and dominant species with fluoride in the solution.
Goswami and Purkait222 used acidic alumina to defluoridate water and found that pH 4.4 resulted in the removal of the most fluoride. The adsorption process fitted well with the Langmuir model, showing an adsorbent capacity value of 8.4 mg g−1, while the kinetics adopted a pseudo-second-order model. Farrah et al.223 investigated the association between fluoride and amorphous Al(OH)3, alumina, or gibbsite in the pH range of 3 to 8 and fluoride concentration of 0.1–1.0 mg L−1. A high amount of the amorphous AI(OH)3 gel was dissolved by forming AlF complexes at a pH value of 6 and an overall F−
:
Al ratio of > 2.5, with the distribution of fluoro ions governed through the equilibrium F− value. Some solid remained in the pH 4–7 range at lower F
:
Al ratios, and F− was severely sorbed from solution. According to the authors, the highest absorption of F− was seen in the pH range of 5.5 to 6.5, which was approximately 9 mol kg−1. The fluoride absorption was reduced at a lower pH owing to the preferred production of AlFx soluble entities, where at the maximum pH, OH− displaced the fluoride ion from the solid, and the quantity of fluoride ion adsorbed on the complexes quickly decrease to around zero in the pH range of 6 to 8. The fluoride uptake fluctuated according to the Langmuir isotherm at a constant pH (5 and 7.5). In acid conditions, the quantity of substrate transformed into AlFx complexes increased as the pH decreased and the initial fluoride concentration increased. Gibbsite exhibited the same dissolving patterns as feldspar but was considerably slower. This substrate absorbed less F− (10–20 mmol kg−1). Many researchers have altered activated alumina, chemically or thermally, to improve its adsorption effectiveness. Tripathy et al.224 synthesized alum-impregnated activated alumina (AIAA) and discovered that it was more effective for water defluoridation. Its surface area was enhanced by impregnating alum from 113 m2 g−1 to 176 m2 g−1. At pH 6.5, with an adsorbent dosage of 8 g L−1, a contact time of 180 min, and an initial concentration of 20 mg L−1 in 50 mL water, AIAA could remove 99% of fluoride. The Bradley equation revealed that the adsorption capacity reduced as the pH increased, linking the isotherm and fluctuation in the adsorbent dosage data. Fluoride removal was shown to be attributed to simple surface precipitation rather than adsorption, according to the energy-dispersive X-ray analysis (EDAX). The modest adsorption rate of activated alumina is readily available for usage in large-scale water treatment. Magnesia showed some promising features in removing fluoride, although its application as an adsorbent is limited owing to its powder form. Maliyekkal et al.225 created magnesia-amended activated alumina (MAAA) to combine the benefits of these two materials and examined the capability of the generated adsorbent to remove fluoride. MAAA, which was fabricated by calcining magnesium hydroxide-impregnated alumina at 450 °C, has a much higher capability for the adsorption of fluoride than AA. More than 95% fluoride removal occurred within a contact time of 180 min and neutral pH. The production of Mg(OH)2 with the help of interacting magnesia with water was proposed for adsorption of fluoride. The fluoride ions in the polluted water substituted the hydroxyl ions in the brucite crystal lattice during the production of Mg(OH)2 without disrupting the crystal structure of the molecule. According to the Sips equation, the maximum adsorption capacity of MAAA for fluoride removal was 10.12 mg g−1. The pH range of 5.0–7.5 was optimal for fluoride elimination. Higher bicarbonate and sulfate concentrations lowered the fluoride adsorption capability. As an eluent, a 2% sodium hydroxide solution was used to regenerate the fluoride-bearing MAAA.
Table 1 shows a comparison of several aluminum-based adsorbents for the removal of fluoride under ideal experimental circumstances. Based on physical factors that impact the complicated chemistry of fluoride in water, the performance of different adsorbents may vary.
Table 1 Optimum experimental conditions of various aluminium-based adsorbents with their maximum adsorption capacities and fluoride removal efficiency
S. no. |
Adsorbent |
Adsorption capacity [mg g−1] |
pH |
Dose [g L−1] |
Contact time (h) |
Initial fluoride conc. [mg L−1] |
Langmuir adsorption |
Freundlich adsorption |
1st Order model |
2nd Order model |
Characterization |
Removal [%] |
Regeneration |
Reference |
1 |
Acidic alumina |
8.4 |
4.4 |
4.5 |
1.5 |
15 |
Yes |
No |
No |
Yes |
XRD, SEM, BET, FTIR, EDAX |
94 |
85 |
222
|
2 |
Alumina impregnated alginate beads |
17 |
7.4–8.2 |
2 |
5 |
1–100 |
Yes |
No |
No |
Yes |
SEM, EDX, ATR-FTIR, XRD |
99.9 |
— |
226
|
3 |
Calcium aluminate material |
4.37 |
3–11 |
3 |
24 |
8.9 |
Yes |
No |
No |
Yes |
XRD, SEM |
85 |
84 |
227
|
4 |
Chitosan based mesoporous alumina-450 |
8.26 |
3–9 |
4 |
24 |
5 |
Yes |
No |
No |
Yes |
XRD, SEM, FTIR, EDAX |
78 |
— |
228
|
5 |
Copper oxide coated alumina |
7.22 |
4–9 |
0.4 |
24 |
10 |
Yes |
No |
No |
Yes |
XRD, SEM |
98 |
97 |
229
|
6 |
Calcium oxide loaded mesoporous alumina |
137 |
6.8 |
3 |
8 |
10 |
Yes |
No |
No |
Yes |
XRD, HRTEM |
90 |
— |
230
|
7 |
Gamma alumina with magnesium oxide nanocrystals |
5.6 |
6.3–7.3 |
0.5 |
2.33 |
5 |
Yes |
No |
No |
Yes |
XRD, BET, SEM, EDAX, FTIR |
85 |
— |
231
|
8 |
Hydroxyapatite modified activated alumina |
14.4 |
8 |
7 |
8 |
9 |
Yes |
Yes |
— |
— |
SEM, EDX, FTIR |
— |
— |
232
|
9 |
Lanthanum-impregnated activated alumina |
16.9 |
7.6–8.6 |
1 |
— |
10 |
Yes |
No |
— |
— |
SEM, TEM, XRD, TGA EXAFS |
77.2 |
98 |
233
|
10 |
La3+-modified activated alumina |
6.7 |
4–8 |
2 |
2 |
10 |
Yes |
No |
No |
Yes |
SEM-EDS, EXAFS |
— |
— |
234
|
11 |
Magnesium dioxide-coated alumina |
0.16 |
7 |
8 |
3 |
10 |
Yes |
No |
No |
Yes |
SEM, EDAX, MMS |
98 |
— |
235
|
12 |
Modified immobilized activated alumina |
0.76 |
7 |
10 |
1 |
12 |
Yes |
Yes |
— |
— |
— |
95 |
85 |
236
|
13 |
Magnesia-amended activated alumina |
10.12 |
7 |
4 |
3 |
10 |
— |
— |
No |
Yes |
XRD, SEM, EDAX, GAPA |
95 |
90 |
225
|
14 |
Manganese oxide-coated alumina |
2.85 |
4–7 |
5 |
3 |
— |
Yes |
No |
No |
Yes |
XRD, BET |
91 |
94.6 |
237
|
15 |
Hydrous manganese oxide-coated alumina |
7.09 |
5.2 |
— |
— |
10–70 |
Yes |
No |
No |
Yes |
SEM, BET, XRD, FTIR, XPS, pHpzc |
95 |
85 |
238
|
16 |
Aluminum-impregnated coconut fiber |
3.192 |
5.0 |
0.5 |
1 |
1 |
Yes |
Yes |
Yes |
Yes |
XRD, FTIR, SEM |
88 |
98 |
239
|
6.1.6.2 Calcium-based adsorbent.
Owing to the high affinity of fluoride together with its biocompatibility in the human body, several researchers have looked at calcium-based adsorbents to remove fluoride.240 Gandhi et al.241 investigated chalk powder as a fluoride adsorbent. Due to its high porosity, chalk powder was selected as an adsorbent. The adsorption of fluoride on the chalk powder showed linear behavior for the concentration of fluoride, adsorbent dosage, and contact duration in batch adsorption investigations. The kinetic isotherms showed the pseudo-first-order and second-order models, and the adsorption data fitted well with the Langmuir and Freundlich models. In nature, the adsorption process is exothermic and spontaneous. The efficacy of stimulated and conventional quick lime in the forms of adsorbents to remove fluoride was also examined.242 When the original fluoride content was 50 mg L−1, the fluoride removal from the synthetic solution was 80.6% under the optimal conditions. According to the Langmuir model, the best adsorption efficiency for fluoride on activated quick lime was noted to be 16.67 mg g−1. According to the XRD examinations and SEM graphs, the elimination of fluoride was primarily because of chemisorption and precipitation. PO43− > SO42− > NO3− were the anions that decreased the fluoride adsorption in that order. Gogoi and Dutta243 transformed limestone powder hydrothermally using phosphoric acid. The FTIR and XRD measurements revealed that hydroxyapatite (HAP) was produced during the hydrothermal treatment. The hydrothermally treated adsorbent had an adsorption capability of 6.45 mg g−1. The isotherm model was used for governing the process through physical adsorption on interacting HAP to fluoride ions. According to the data, second-order kinetics was observed. The adsorption process proven to be spontaneous, endothermic, and irreversible according to the thermodynamic analysis. Jayarathne et al.244 employed natural apatite for the adsorption of fluoride. The pH of the adsorption method was found to be very important, and the ideal pH for the maximum removal was found to be 6. The adsorption increased with an increase in adsorbent dosage due to the increase in the number of adsorbent sites, which is also illustrative. With a contact time of 10 min, the maximum adsorption was obtained at pH 6 for a solution containing 15 mg L−1 fluoride. The Langmuir model was well-suited to fit the adsorption data, which indicated an adsorption capacity value of 0.212 mg g−1. Pseudo-second order kinetics were seen in the experimental data.
Table 2 shows a comparison of several calcium-based adsorbents for fluoride removal under ideal experimental circumstances. Based on the physical factors that impacted the complicated chemistry of fluoride in water, the performance of different adsorbents may vary.
Table 2 Optimum experimental conditions of various calcium-based adsorbents with their maximum adsorption capacities and fluoride removal efficiency
S. no. |
Adsorbent |
Adsorption capacity [mg g−1] |
pH |
Dose [g L−1] |
Contact time (h) |
Initial fluoride conc. [mg L−1] |
Langmuir adsorption |
Freundlich adsorption |
1st Order model |
2nd Order model |
Characterization |
Removal [%] |
Regeneration |
Reference |
1 |
Quick lime |
16.67 |
12.71 |
5 |
1.25 |
50 |
Yes |
No |
Yes |
No |
XRD, SEM, BET |
80.6 |
6 |
242
|
2 |
Ca-deficient hydroxyapatite |
26.11 |
4–7 |
— |
— |
— |
Yes |
No |
No |
Yes |
BET, SEM, EDS, XRD, UV-Vis |
85 |
— |
245
|
3 |
Hydroxyapatite |
4.7 |
5–7.3 |
0.1 |
16 |
— |
No |
Yes |
No |
Yes |
XRD |
96 |
50 |
246
|
4 |
Glass derived hydroxyapatite |
17.34 |
6.72 |
5 |
12 |
100 |
No |
Yes |
No |
Yes |
XRD, SEM, IRS |
96.6 |
— |
247
|
5 |
Aluminum modified hydroxyapatite |
32.57 |
5 |
0.5 |
— |
10 |
Yes |
No |
No |
Yes |
XRD, TEM, FTIR |
75 |
— |
248
|
6 |
Bone char |
2.8 |
6 |
0.6 |
— |
2 |
Yes |
Yes |
Yes |
Yes |
— |
— |
— |
249
|
7 |
Crustacean derived calcium phosphate systems |
18.2 |
0.2 |
2 |
180 |
5 |
Yes |
Yes |
Yes |
Yes |
FTIR, XRD |
92 |
— |
250
|
8 |
Gypsiferous limestone |
1.07 |
3–5 |
15 |
12 |
11.28 |
Yes |
No |
Yes |
No |
— |
89 |
86 |
251
|
9 |
Charred beef shoulder blade bones |
3.15 |
— |
8 |
3 |
10 |
No |
Yes |
No |
Yes |
— |
60.27 |
— |
252
|
10 |
Natural crystalline apatite |
0.212 |
6 |
— |
0.166 |
15 |
Yes |
No |
No |
Yes |
FTIR, XRD, XRF |
65 |
— |
244
|
11 |
Hydroxyapatite (Hap) |
3.12 |
4.16 |
0.28 |
1.5 |
20 |
Yes |
Yes |
No |
Yes |
FTIR, XRD, pHpc |
86.34 |
— |
253
|
12 |
Hydroxyapatite (HAP) |
12.4 |
12.5 |
4 |
4 |
4.6 |
Yes |
Yes |
— |
— |
— |
94.5 |
— |
254
|
13 |
Magnesium-incorporated hydroxyapatite |
1.16 |
7 |
10 |
3 |
10 |
Yes |
No |
No |
Yes |
FTIR, XRD, TEM, EDX |
94.5 |
91 |
255
|
14 |
Ce–Zr oxide nanospheres encapsulating calcium alginate beads |
137.6 |
7 |
0.5 |
4 |
10 |
Yes |
Yes |
No |
Yes |
FTIR, XPS, XRD, SEM, TEM |
78 |
63 |
256
|
6.1.6.3 Oxide/hydroxide-based adsorbent.
According to many studies, it has been observed that metal oxides/hydroxides have a high capability for the adsorption of fluoride.257 Kumar et al.258 utilized granular ferric hydroxide (GFH) for the adsorption of fluoride. At 25 °C and pH in the range of 4–8, the most outstanding fluoride removal was 7 mg g−1. The isotherm supported the Langmuir isotherm, and the kinetic analysis indicated that pore diffusion governs the pseudo-first-order isotherm. The presence of phosphate ions had the most significant impact on the fluoride adsorption on GFH, followed by carbonate and sulfate ions. Tang and Zhang259 synthesized and analyzed Ce–Fe bimetal oxides with a hierarchical pore structure and good fluoride removal efficiency. XRD, XPS, and HRTEM were used to determine the properties of the adsorbent. The maximum capability for defluoridation was observed to be 60.97 mg g−1. The Langmuir model explained the experimental data well, and the kinetic analysis showed the pseudo-second-order model. Carbonate ions had the most significant impact on the adsorption followed by nitrate, bicarbonate, sulfate, and chloride ions. Hussain et al.260 investigated the effectiveness of the fluoride adsorption by co-precipitated Mg(III)–Al(III)–La(III) triple-metal hydrous oxide. In the pH range of 2–12, the adsorbent effectively removed 98.28% of fluoride from a fluoride solution of 20.66 mg L−1. The adsorption was endothermic and suited the Langmuir model. Also, the adsorption followed the pseudo-second-order model. The adsorbent could be readily regenerated with a combination of methanol and HCl until 95.71% recovery.
Table 3 shows a comparison of several oxide/hydroxide-based adsorbents for fluoride removal under ideal experimental circumstances. Based on the physical factors that impacting the complicated chemistry of fluoride in water, the performance of different adsorbents may vary.
Table 3 Optimum experimental conditions of various oxide/hydroxide-based adsorbents with their maximum adsorption capacities and fluoride removal efficiency
S. no. |
Adsorbent |
Adsorption capacity [mg g−1] |
pH |
Dose [g L−1] |
Contact time (h) |
Initial fluoride conc. [mg L−1] |
Langmuir adsorption |
Freundlich adsorption |
1st Order model |
2nd Order model |
Characterization |
Removal [%] |
Regeneration |
Reference |
1 |
Aluminium hydroxide |
7 |
— |
1.6 |
1 |
20 |
No |
Yes |
No |
Yes |
— |
90 |
— |
261
|
2 |
Iron-aluminium-cerium trimetal oxide |
178 |
7 |
1.5 |
— |
84.5 |
Yes |
No |
— |
— |
ASAP, XRD |
90 |
97 |
262
|
3 |
Aluminium (hydro)oxide |
4.10 |
6 |
2 |
2 |
6 |
Yes |
Yes |
Yes |
Yes |
EXD, XRD, FTIR, SEM |
98 |
97.36 |
263
|
4 |
Hydrous iron- tin mixed oxide |
10.47 |
6.4 |
0.8 |
2 |
25 |
Yes |
No |
No |
Yes |
FTIR, XRD |
95.36 |
75 |
264
|
5 |
Hydrated iron-aluminium-chromium ternary mixed oxide |
31.89 |
5.6 |
4 |
1.5 |
10 |
Yes |
No |
No |
Yes |
FTIR |
95.7 |
91 |
265
|
6 |
Lithium-aluminium layered double hydroxide |
47.24 |
7 |
2 |
1 |
20 |
Yes |
Yes |
No |
Yes |
TEM, XRD, TGA |
97.36 |
— |
266
|
7 |
Crystalline titanium dioxide |
15.35 |
7–8 |
1 |
3 |
5 |
Yes |
No |
No |
Yes |
XRD, SEM |
75 |
79 |
267
|
8 |
Hydrous bismuth oxide |
1.93 |
4–12 |
50 |
3 |
10 |
Yes |
No |
No |
Yes |
XRD, FTIR, SEM |
65 |
— |
268
|
9 |
Mg–Cr–Cl layered double hydroxide |
13.15 |
6 |
6 |
0.66 |
10 |
Yes |
No |
No |
Yes |
FTIR, XRD, SEM |
88.5 |
2 |
269
|
10 |
Alumina-magnesia mixed hydroxide |
5.62 |
7 |
6 |
24 |
5.13 |
Yes |
No |
No |
Yes |
— |
90 |
98 |
270
|
11 |
ZnCr3–NO3 layered double hydroxide |
31 |
3–10 |
1 |
3 |
10 |
Yes |
No |
No |
Yes |
XRD, FTIR |
95 |
80 |
271
|
12 |
Aluminium oxide-manganese oxide |
18.6 |
5–7 |
4 |
2 |
— |
No |
Yes |
No |
Yes |
XRD, TGA, SEM |
96.4 |
— |
272
|
13 |
Calcined layered double hydroxide |
79.37 |
6–7 |
0.5 |
1 |
10 |
Yes |
No |
— |
— |
XRD |
99.2 |
— |
273
|
14 |
Non-thermal plasma modified Ni–Al layered double hydroxide |
93.41 |
3–7 |
2 |
0.5 |
60 |
— |
— |
No |
Yes |
XRD, FTIR, TG-DTA |
37.12 |
— |
274
|
15 |
Calcium–aluminium–lanthanum composite |
29.30 |
6.8 |
1 |
3 |
10 |
Yes |
No |
No |
Yes |
XRD, FTIR, SEM, XPS |
96.65 |
— |
275
|
16 |
Trimetal oxide |
2.19 |
7 |
1 |
1.16 |
10 |
Yes |
No |
No |
Yes |
XRD, XRF |
78 |
— |
276
|
17 |
Fe3O4@LDH/poly |
167.62 |
7.8 |
0.005 |
0.66 |
10 |
Yes |
Yes |
Yes |
Yes |
FTIR, XRD, SEM |
— |
— |
277
|
18 |
β-Cyclodextrin modified hydrus zirconium oxide |
31.45 |
5.5 |
0.5 |
2 |
10 |
Yes |
No |
No |
Yes |
FTIR, XRD |
94 |
95 |
278
|
19 |
Fe–Mg–La trimetal composite |
270 |
4 |
0.1 |
5 |
10 |
Yes |
No |
— |
— |
FTIR, XPS |
85 |
96.7 |
279
|
20 |
Micron-sized magnetic adsorbent |
41.8 |
3 |
1 |
2 |
4 |
Yes |
No |
No |
Yes |
SEM, XPS |
92.4 |
96.8 |
280
|
21 |
Calcined Mg–Al layered double hydroxide |
55.22 |
7 |
4 |
2.5 |
10 |
No |
Yes |
No |
Yes |
SEM, XRD, FTIR, TG-DTA, TEM |
93.92 |
86 |
281
|
22 |
Fe-modified clinoptilolite |
1.72 |
11 |
2 |
5 |
50 |
Yes |
Yes |
Yes |
Yes |
XFS,SEM,XRD,BET |
80.23 |
— |
282
|
23 |
Goethite anchoring regenerated grapheme oxide |
24.67 |
10.90 |
— |
1 |
50 |
Yes |
No |
No |
Yes |
XPS, FTIR, XRD, FTIR, SEM, TEM |
87.4 |
95 |
283
|
6.1.6.4 Carbon-based adsorbents.
Carbon-based adsorbents have been explored to remove fluoride given that carbon possesses a strong affinity towards fluoride anions. Karthikeyan and Elango284 utilized graphite in adsorbents to remove fluoride from solution. The optimum fluoride adsorption was achieved at a low pH value and high temperature. The adsorption data fit the Freundlich and Langmuir models, and the pseudo-first-order model was observed. According to the thermodynamics, the adsorption is an exothermic reaction. Zirconyl-impregnated activated carbon was created by Joshi et al.285 Lapsi (Choerospondias axillaris) seed stone provided the activated carbon. The maximum defluoridation was discovered in the pH range of 3–4 after a 3 hour contact duration. The adsorption isotherm results matched well with the Langmuir isotherm model. Said and Machunda286 investigated the defluoridation of water using activated carbon from coconut shells. Batch studies were performed to determine how different adsorbents affected the adsorption efficiency. The acidic range favored adsorption, with the highest adsorption of 58.4% found at a pH value of 2. The Freundlich and Langmuir models were an excellent match to explain the fluoride adsorption on activated carbon from coconut shells.
Table 4 shows a comparison of several carbon-based adsorbents for fluoride removal under ideal experimental circumstances. Based on the physical factors that impact the complicated chemistry of fluoride inside water, the performance of different adsorbents may vary.
Table 4 Optimum experimental conditions of various carbon-based adsorbents with their maximum adsorption capacities and fluoride removal efficiency
S. no. |
Adsorbent |
Adsorption capacity [mg g−1] |
pH |
Dose [g L−1] |
Contact time (h) |
Initial fluoride conc. [mg L−1] |
Langmuir adsorption |
Freundlich adsorption |
1st Order model |
2nd Order model |
Characterization |
Removal [%] |
Regeneration |
Reference |
1 |
KMnO4 modified activated carbon derived from steam pyrolysis of rice straw |
23.3 |
2 |
1.5 |
3 |
20 |
Yes |
Yes |
No |
Yes |
IC, pHpzc, BET |
100 |
— |
287
|
2 |
Waste carbon slurry |
4.86 |
— |
1 |
— |
15 |
Yes |
No |
No |
Yes |
XRD, SEM |
— |
92 |
288
|
3 |
Zirconium ion-impregnated coconut fiber carbon |
1.95 |
4 |
20 |
6 |
20 |
Yes |
No |
No |
Yes |
SEM, EDX, BET, pHpzc |
93 |
100 |
289
|
4 |
Zirconium-impregnated coconut shell carbon |
0.99 |
4 |
1 |
6 |
10 |
Yes |
No |
No |
Yes |
EDX, BET |
91 |
100 |
290
|
5 |
Zirconium-impregnated cashew nut shell carbon |
1.83 |
7.6 |
0.015 |
3 |
3 |
Yes |
No |
No |
Yes |
XRD, FTIR |
80.33 |
96.2 |
291
|
6 |
Activated carbon from Bermuda grass |
4.702 |
5–5.5 |
12.5 |
1.75 |
3 |
Yes |
No |
— |
— |
— |
83.77 |
67.4 |
292
|
7 |
Iron-impregnated granular ceramics |
2.16 |
4 |
20 |
48 |
10 |
Yes |
Yes |
No |
Yes |
SEM, BET, EDX |
94 |
— |
293
|
8 |
Microwave assisted activated carbon derived from Acacia auriculiformis scrap wood |
18.95 |
4 |
2 |
0.33 |
— |
Yes |
No |
No |
Yes |
— |
97.2 |
— |
294
|
9 |
Zirconium-impregnated walnut shell carbon |
6.38 |
3 |
15 |
3 |
3 |
No |
Yes |
No |
Yes |
XRD, SEM, EDAX |
94 |
96.2 |
295
|
10 |
Carbon nanotubes/alumina |
9.6 |
6 |
0.5 |
1.25 |
10.3 |
No |
Yes |
— |
— |
— |
— |
— |
296
|
11 |
Aligned carbon nanotubes (ACNTs) |
4.5 |
7 |
2 |
1 |
15 |
Yes |
Yes |
Yes |
Yes |
SEM, HRTEM |
94 |
— |
297
|
12 |
Activated bagasse carbon |
1.15 |
6 |
4 |
1 |
2.5 |
No |
Yes |
No |
Yes |
— |
57.6 |
— |
298
|
13 |
Zirconium-impregnated activated carbon mixed with oxalic acid |
7.40 |
7 |
— |
— |
40 |
Yes |
Yes |
— |
— |
BET, FTIR, XPS, SEM, pHpzc, XRD, DFT, ICP-OES |
94 |
— |
299
|
14 |
Cow dung carbon @ 500 °C |
15 |
7.53 |
2 |
0.66 |
2 |
No |
Yes |
No |
Yes |
SEM, XRD, FTIR, EDS, pHpzc |
99 |
100 |
300
|
15 |
Powder activated charcoal |
— |
2 |
2.0 |
2 |
|
Yes |
Yes |
Yes |
Yes |
— |
94 |
— |
301
|
16 |
Natural coal |
5.9 |
4 |
3 |
1 |
5 |
Yes |
No |
— |
— |
— |
82 |
— |
302
|
17 |
Physically activated coal |
8.36 |
2 |
3 |
1 |
5 |
Yes |
No |
— |
— |
— |
86 |
— |
303
|
18 |
Chitosan doped graphite composite (FCDGNC) |
3.5 |
6.5 |
2.5 |
2.33 |
— |
Yes |
No |
— |
— |
FTIR, SEM, TGA, XRD |
91 |
44 |
304
|
19 |
Activated carbon prepared from bael shell |
2.4 |
6 |
2 |
1 |
8 |
Yes |
No |
No |
Yes |
SEM, FTIR |
52 |
8 |
305
|
20 |
Activated carbon derived from bark of Ficus racemosa plant |
1.65 |
7 |
4 |
1 |
5 |
Yes |
No |
Yes |
No |
SEM, FTIR, EDX, BET, FESEM |
88 |
— |
306
|
6.1.6.5 Natural material-based adsorbents.
Various natural materials have been employed as adsorbents to remove fluoride from solution, including dirt, chitosan, clay, and zeolite due to their large surface area, mechanical and chemical durability, molecular sieve arrangement, and diverse range of surface and structural features.307 The ability of clay and its minerals to eliminate fluoride has been widely demonstrated by several researchers. According to Peter,308 bauxite-rich soil possesses a higher adsorption efficiency compared to kaolinite-rich soil. In both kaolinite-rich and bauxite-rich soil, activated soil was showed added extraordinary adsorption ability compared to non-activated soil. At low fluoride concentrations, bauxite-rich soil was more efficient than at high fluoride concentrations. Due to the enhanced and stabilized positive sites, magnesium-enriched clay has higher adsorption effectiveness than raw clay, according to Atasoy and Sahin.309 Also, calcination changes the properties of clay.
Table 5 shows a comparison of several natural-based adsorbents for the removal of fluoride under ideal experimental circumstances. Based on the physical factors impacting the complicated chemistry of fluoride in water, the performance of different adsorbents may vary.
Table 5 Optimum experimental conditions of various natural-based adsorbents with their maximum adsorption capacities and fluoride removal efficiency
S. no. |
Adsorbent |
Adsorption capacity [mg g−1] |
pH |
Dose |
Contact time (h) |
Initial fluoride conc. [mg L−1] |
Langmuir adsorption |
Freundlich adsorption |
1st Order model |
2nd Order model |
Characterization |
Removal [%] |
Regeneration |
Reference |
1 |
Montmorillonite |
0.263 |
6 |
8 |
3 |
4 |
No |
Yes |
— |
— |
— |
65 |
93 |
310
|
2 |
Laterite soil |
0.8461 |
6.8 |
1 |
— |
20 |
Yes |
No |
Yes |
No |
XRD, SEM |
80.4 |
80.4 |
311
|
3 |
Bauxite |
33.6 |
4 |
12.5 |
2 |
8 |
Yes |
No |
Yes |
No |
XRD, TGA |
93.8 |
— |
312
|
4 |
Montmorillonite clay |
1.836 |
2 |
70 |
0.83 |
3 |
Yes |
No |
— |
— |
— |
97 |
— |
313
|
5 |
Clay soil |
93.45 |
3 |
— |
24 |
— |
Yes |
No |
— |
— |
— |
85 |
— |
314
|
6 |
Sodium activated Algerian non calcium montmorillonite clay |
1.324 |
4 |
3 |
0.5 |
6 |
Yes |
No |
— |
— |
FTIR, XRD, BET |
88 |
— |
315
|
7 |
Ceramic adsorbent |
2.16 |
4–11 |
20 |
48 |
10 |
Yes |
Yes |
No |
Yes |
BET, SEM, XRD, EDS, UV-Vis |
94.4 |
— |
316
|
8 |
Magnesium incorporated bentonite clay |
2.26 |
3–10 |
3 |
12 |
5 |
Yes |
No |
Yes |
No |
XRD, SEM |
95.47 |
97 |
317
|
9 |
Acid treated diatomaceous earth |
51.1 |
3.4 |
500 |
10 |
400 |
Yes |
No |
— |
— |
— |
92 |
— |
318
|
10 |
Pumice |
0.31 |
7 |
20 |
3 |
7 |
No |
Yes |
No |
Yes |
— |
85.75 |
— |
319
|
11 |
Acid treated raw laterite |
39.10 |
5 |
0.5 |
24 |
10 |
Yes |
Yes |
— |
— |
FTIR, SEM, SAA |
96 |
96 |
320
|
12 |
Pyrophyllite |
2.2 |
4.9 |
4 |
2 |
10 |
Yes |
No |
No |
Yes |
BET, XRD, ZPC |
85 |
— |
321
|
13 |
Kanuma mud |
3.06 |
5–7 |
20 |
2 |
10 |
No |
Yes |
No |
Yes |
BET, TEM, EDS, BJH |
90 |
85 |
322
|
14 |
Calcined meixnerite |
16.1 |
— |
— |
50 |
75 |
Yes |
Yes |
Yes |
No |
XRD, SEM |
97.3 |
61 |
323
|
15 |
Siliceous material |
12.4 |
3.4 |
500 |
0.33 |
200 |
Yes |
No |
— |
— |
— |
100 |
— |
324
|
16 |
Hydrogen peroxide modified pumice |
11.765 |
6 |
6 |
2.5 |
10 |
No |
Yes |
No |
Yes |
SEM, XRD, FTIR, BET, XRF, PZC |
70.8 |
98 |
325
|
17 |
Raw bauxite |
0.275 |
5.5 |
120 |
1.5 |
6.17 |
— |
— |
Yes |
No |
— |
96.1 |
— |
326
|
18 |
Al3+ modified bentonite clay |
5.7 |
2–12 |
10 |
0.5 |
60 |
Yes |
Yes |
No |
Yes |
XRD, XRF, BET, EDS, CEC, PZC |
99.97 |
— |
327
|
19 |
Calcium aluminium modified zeolite |
8.03 |
4–8 |
1.5 |
6 |
10 |
No |
Yes |
No |
Yes |
— |
96 |
90 |
328
|
20 |
Clay mixed hydroxyapatite |
18.409 |
6 |
1.25 |
0.5 |
5 |
No |
Yes |
No |
Yes |
— |
96.5 |
— |
329
|
21 |
Vermiculite |
2.36 |
4 |
2 |
1.16 |
4 |
No |
Yes |
No |
Yes |
FTIR, SEM |
51 |
— |
330
|
22 |
Iron ore |
1.72 |
6 |
5 |
2 |
14.22 |
No |
Yes |
Yes |
No |
XRF |
89 |
— |
331
|
23 |
Lanthanum-impregnated bauxite |
18.18 |
8.5 |
2 |
120 |
20 |
Yes |
No |
No |
Yes |
— |
99 |
95 |
332
|
24 |
Granular aluminium coated bauxite |
0.426 |
2 |
10 |
176 |
5 |
Yes |
No |
No |
Yes |
SEM, XRD, EDS, XRF, BET, FTIR |
94.1 |
— |
333
|
25 |
Chitosan iron complex |
2.34 |
3–10 |
10 |
1.5 |
50 |
Yes |
Yes |
No |
Yes |
FTIR, SEM |
90 |
84.3 |
334
|
26 |
Brushite |
36.26 |
5.36 |
1.5 |
2 |
49.06 |
Yes |
No |
No |
Yes |
XRD, SEM, EDX |
90.83 |
— |
335
|
27 |
Zirconium modified zeolite |
28.57 |
7 |
10 |
1 |
40 |
No |
Yes |
No |
Yes |
XRD, SEM, BET |
97.62 |
— |
336
|
28 |
Mg/Ce/Mn oxide-modified diatomaceous earth |
12.633 |
6.52 |
6 |
1 |
40 |
Yes |
No |
No |
Yes |
SEM, EDX, BET, XRD, FTIR, PZC |
97.1 |
60.8 |
337
|
29 |
Fe3+ modified bentonite clay |
2.91 |
2–10 |
20 |
0.5 |
10 |
Yes |
No |
— |
— |
XRF, XRD, PZC, CEC, BET, SEM |
100 |
— |
338
|
6.1.6.6 Agriculture and biomass-based adsorbents.
In recent years, products of agriculture and biomass have been extensively employed by scientists to remove fluoride from water. These materials are widely employed in practice because of their widespread accessibility, natural biodegradability, and commercial viability. Several studies were carried out to modify plant-based adsorbents through an appropriate chemical to improve fluoride removal efficiency. Singh et al.339 examined the fluoride adsorption capability of sugarcane bagasse in a batch adsorption experiment. The contact duration, pH, temperature, adsorbent dosage, and starting concentration of fluoride were all investigated. The highest fluoride adsorption was determined to be 4.12 mg g−1. The adsorption rate of fluoride was highly characterized by the Redlich–Peterson isotherm theory and the pseudo-second-order equation. The thermodynamic parameter indicated that the adsorption procedure is endothermic. For the defluoridation of drinking water, Pandey et al.340 produced biomasses from Tinospora cordifolia. The pH value of 7, the adsorbent dosage of 7 g/50 mL, and 120 minutes of contact duration were determined to be the best conditions. The Langmuir and Freundlich isotherms accurately reflected the experimental results. Fluoride binding was found in several frequency ranges using FT-IR spectrum analysis.
Table 6 shows a comparison of several agricultural and biomass-based adsorbents for fluoride removal under ideal experimental circumstances. Based on the physical factors that impact the complicated chemistry of fluoride in water, the performance of different adsorbents may vary.
Table 6 Optimum experimental conditions of various agricultural and biomass-based adsorbents with their maximum adsorption capacities and fluoride removal efficiency
S. no. |
Adsorbent |
Adsorption capacity [mg g−1] |
pH |
Dose |
Contact time (h) |
Initial fluoride conc. [mg L−1] |
Langmuir adsorption |
Freundlich adsorption |
1st Order model |
2nd Order model |
Characterization |
Removal [%] |
Regeneration |
Reference |
1 |
Al3+ loaded saponified orange juice residue |
1.03 |
6 |
1 |
4 |
0.52 |
Yes |
No |
— |
— |
FTIR |
80 |
— |
341
|
2 |
Bark of babool |
1.12 |
8 |
5 |
8 |
5 |
Yes |
No |
No |
Yes |
— |
77.04 |
— |
342
|
3 |
Activated neem stem charcoal |
1.27 |
2 |
5 |
3 |
2 |
Yes |
Yes |
No |
Yes |
— |
94 |
— |
343
|
4 |
Carbon obtained from pecan nut shells modified with a calcium solution extracted from egg shells |
2.3 |
7 |
8 |
— |
20 |
Yes |
No |
— |
— |
EDX, SEM, XRD |
84 |
— |
344
|
5 |
Tea-Al |
13.79 |
4–8 |
2.0 |
2 |
10 |
Yes |
Yes |
Yes |
Yes |
SEM, EDS, XPS, XRD |
— |
— |
345
|
6 |
Banana peel |
1.340 |
6 |
14 |
1 |
20 |
Yes |
No |
No |
Yes |
— |
94.34 |
— |
346
|
7 |
Carbonized cattle dung (CDC) |
15.0 |
7.53 |
2.0 |
— |
2 |
Yes |
Yes |
Yes |
Yes |
SEM, BET, XRD, FTIR, elemental analyses |
99 |
100 |
347
|
8 |
Palm kernel shell |
285.7 |
7 |
0.2 |
2 |
100 |
No |
Yes |
No |
Yes |
FESEM-EDX, XRD, FTIR, XPS |
95 |
— |
348
|
9 |
Mechanically modified guava seeds |
15.6 |
6 |
80 |
6 |
100 |
Yes |
Yes |
No |
Yes |
— |
85 |
— |
349
|
10 |
Mosambi peel powder |
1.35 |
7 |
10 |
0.5 |
5 |
No |
Yes |
No |
Yes |
— |
82.5 |
— |
350
|
11 |
Restructured lignite |
15.8 |
7.93 |
8 |
— |
3 |
Yes |
No |
No |
Yes |
BET, SEM, XRD |
95 |
— |
351
|
12 |
Peepal leaf powder |
2.24 |
7 |
10 |
0.75 |
20 |
No |
Yes |
— |
— |
— |
85.7 |
— |
352
|
13 |
Grape pomace |
7.54 |
3 |
17.5 |
1 |
19.91 |
Yes |
Yes |
Yes |
Yes |
FTIR, SEM, EDS |
— |
— |
353
|
14 |
Pyrolyzed Delonix regia pod carbon |
33.34 |
2 |
15 |
5 |
2–10 |
No |
Yes |
No |
Yes |
BET, ZPC, FTIR, SEM, EDAX |
97 |
— |
354
|
15 |
Lanthanum modified carbon from Sargassum sp. |
94.34 |
7 |
0.4 |
4 |
20 |
Yes |
No |
No |
Yes |
EDX, ZPC, XPS |
90 |
— |
355
|
16 |
Sal leaf powder |
1.28 |
7.5 |
60 |
0.42 |
5 |
No |
Yes |
— |
— |
— |
98.6 |
— |
356
|
17 |
Zirconium[IV] loaded carboxylated orange peel |
5.605 |
6 |
0.7 |
0.83 |
10 |
Yes |
No |
No |
Yes |
FTIR, TGA, SEM, XRD |
97.2 |
91 |
357
|
18 |
Pithacelobiumdulce carbon |
0.81 |
7 |
3 |
0.66 |
3 |
Yes |
No |
Yes |
No |
— |
81.60 |
— |
358
|
19 |
Alkali steam-treated elephant grass |
7 |
2–10 |
1.5 |
2.5 |
5 |
Yes |
No |
No |
No |
PZC, FTIR, XRD, SEM |
85 |
— |
359
|
20 |
Carbonised Punica granatum carbon |
1.68 |
7 |
0.75 |
1.25 |
2–10 |
No |
Yes |
— |
— |
— |
78.1 |
— |
360
|
21 |
Padina sanctae crucis algae |
1.65 |
7 |
48 |
0.08 |
8 |
No |
Yes |
No |
Yes |
FTIR |
97 |
— |
361
|
6.1.6.7 Building material-based adsorbents.
Several studies have investigated the fluoride removal capacity of various construction materials, including brick powder, cement, fly ash, sand, and concrete, with and without treatment and modification. The capacity of hydrated cement for eliminating fluoride from drinkable water was investigated by Bibi et al.362 With a 60 minute contact period and a 30 g L−1 adsorbent dosage, the adsorbent was shown to have an 80% fluoride removal efficacy at pH 7. 1.72 mg g−1 was determined as the maximum fluoride absorption. Because the adsorbent is affordable, no regeneration was necessary. Using batch adsorption tests, Kagne et al.363 examined the potential of hydrated cement to remove fluoride from aqueous solution at different intervals. They discovered that the hydrated cement removed considerable amounts of fluoride in a broad pH range (3–10). The linear transformed Freundlich and Langmuir isotherms were successfully fitted by the experimental data collected from the batch adsorption studies. Togarepi et al.364 carried out an experiment comprised of sand to remove fluoride. It was treated both chemically and thermally for use as an adsorbent. Different parameters were examined by performing batch experiments including pH, initial fluoride, and adsorbent dose. It was observed that the experimental data of the activated sand fitted well with the Freundlich isotherm and the multilayer sorption.
Table 7 shows a comparison of several building-based adsorbents for the removal of fluoride under ideal experimental circumstances. Based on the physical factors that impacted the complicated chemistry of fluoride in water, the performance of different adsorbents may vary.
Table 7 Optimum experimental conditions of various building-based adsorbents with their maximum adsorption capacities and fluoride removal efficiency
S. no. |
Adsorbent |
Adsorption capacity [mg g−1] |
pH |
Dose |
Contact time (h) |
Initial fluoride conc. [mg L−1] |
Langmuir adsorption |
Freundlich adsorption |
1st Order model |
2nd Order model |
Characterization |
Removal [%] |
Regeneration |
Reference |
1 |
Alum sludge |
0.53 |
3–8 |
16 |
1 |
10 |
— |
— |
No |
Yes |
— |
85 |
— |
365
|
2 |
Broken concrete cubes |
1.23 |
7 |
60 |
2 |
8 |
— |
— |
— |
— |
— |
80 |
— |
366
|
3 |
Surface-functionalized polyurethane foam |
7.8 |
6.7 |
10 |
1 |
20 |
— |
— |
— |
— |
SEM, FTIR |
96 |
96 |
367
|
4 |
Basic oxygen furnace slag |
8.07 |
6–10 |
5 |
0.58 |
10 |
Yes |
No |
No |
Yes |
SEM, XRD, ICP-AES, BET |
93 |
4 |
368
|
5 |
Bottom ash |
16.26 |
6 |
0.7 |
1.75 |
— |
Yes |
No |
— |
— |
— |
83.2 |
— |
369
|
6 |
Gas concrete |
10.3 |
6.9 |
40 |
1 |
120 |
— |
— |
— |
— |
SEM, XPS |
96 |
— |
370
|
7 |
Zirconium hydroxide modified red mud porous material |
0.6 |
3 |
— |
1 |
20 |
Yes |
No |
No |
Yes |
XRD, EDX, SEM |
90 |
90 |
371
|
8 |
Low weight concrete |
5.15 |
6.9 |
— |
1 |
2.5 |
Yes |
Yes |
— |
— |
BET |
— |
— |
372
|
9 |
Calcium hydroxide treated fly ash |
10.86 |
7 |
3 |
2 |
10 |
Yes |
No |
No |
Yes |
PZC |
80 |
— |
373
|
10 |
Calcinated electro coagulation sludge |
44.25 |
6 |
4 |
2 |
25 |
Yes |
Yes |
No |
Yes |
— |
99.99 |
— |
374
|
11 |
Alumina cement granules (ALC) |
10.215 |
7 |
2 |
— |
8.65 |
Yes |
No |
— |
— |
— |
— |
— |
375
|
12 |
Heat-activated Mahabir colliery shale |
0.358 |
3 |
70 |
24 |
10 |
No |
Yes |
No |
Yes |
XRD, BET, SEM, PZC |
88.3 |
0 |
376
|
6.1.6.8 Nanomaterial-based adsorbents.
The advancement of nanotechnology in the present scenario has inspired researchers. The area of nanotechnology involves the synthesis, development, and classification of nano-sized particles, generally with a size of 1 to 100 nm, which has been found to be a highly active avenue for researcher to purify contaminated water. Patel et al.377 blended CaO nanoparticles through the sol–gel method. The adsorption capacity was 92% with 0.6 g L−1 dose, 30 min contact duration, and 100 mg L−1 initial fluoride concentration. The Freundlich model and pseudo-first-order model best-fit the experimental results. The reaction was spontaneous and endothermic, according to the thermodynamic parameters. Fluoride absorption is most likely caused by an ion-exchange process, which produces CaF2 by substituting OH− ions with F− ions from CaO nanoparticles. On the addition of 0.1 M NaOH or 0.1 M HCl, 95% desorption can be attained due to the adjusted pH range between 2 and 12. Devi et al.378 explored nano-MgO for the adsorption of fluoride. On employing a 0.6 g L−1 dose, the adsorption efficacy reached 90% for nano-MgO. The availability of OH− ions influences the fluoride adsorption through nano-MgO. The adsorption of fluoride was negligible owing to the variations in pH and the occurrence of other ions. It was also observed that the equilibrium data followed the Freundlich model rather than the Langmuir model, suggesting that the multilayer adsorption followed the pseudo-second-order model. The regeneration study showed that 1 M HCl was the appropriate material for fluoride removal with a desorption capacity of 95% and 2 M NaOH had 25% adsorbent regeneration. Kumar et al.379 performed batch experiments involving the parameter pH, co-existing anions, temperature, and contact time to synthesize nano-alumina for fluoride removal efficiency. XRD, EDX, FTIR, SEM were used to analyze the structural characteristics of nano-alumina. The obtained results clarified that at 25 °C and pH of 6.15, fluoride of 14 mg g−1 was removed. The adsorption process revealed a pseudo-second-order model and followed the Langmuir model. In the presence of carbonate, sulfate and phosphate ions, the adsorption efficacy of nano-alumina was enhanced.
Table 8 shows a comparison of several nanomaterial-based adsorbents for the removal of fluoride under the ideal experimental circumstances. Based on the physical factors that impact the complicated chemistry of fluoride in water, the performance of different adsorbents may vary.
Table 8 Optimum experimental conditions of various nanomaterial-based adsorbents with their maximum adsorption capacities and fluoride removal efficiency
S. no. |
Adsorbent |
Adsorption capacity [mg g−1] |
pH |
Dose |
Contact time (h) |
Initial fluoride conc. [mg L−1] |
Langmuir adsorption |
Freundlich adsorption |
1st Order model |
2nd Order model |
Characterization |
Removal [%] |
Regeneration |
Reference |
1 |
Nano-scale aluminium oxide hydroxide |
3.25 |
5.2 |
5 |
6 |
13 |
Yes |
No |
No |
Yes |
XRD, TEM, NAD, PZC, FTIR, XPS, BET |
90 |
97 |
380
|
2 |
Manganese oxide modified aluminium oxy (hydroxide) |
18.62 |
5–7 |
— |
— |
— |
Yes |
Yes |
Yes |
Yes |
SEM, XRD |
94.8 |
86.2 |
381
|
3 |
Nano-hydroxyapatite |
2.30 |
5 |
4 |
1.66 |
10 |
Yes |
Yes |
No |
Yes |
XRD, FTIR, TEM, SEM |
91 |
— |
382
|
4 |
Nano-sized goethite |
59 |
— |
1 |
2 |
30 |
No |
Yes |
No |
Yes |
XRD, TEM |
49 |
— |
383
|
5 |
CeO2/Al2O3 |
37.0 |
3–10 |
2 |
24 |
120 |
No |
Yes |
Yes |
Yes |
— |
— |
— |
384
|
6 |
Multi-walled carbon nanotubes |
3.5 |
5 |
— |
0.3 |
— |
No |
Yes |
— |
— |
— |
94 |
— |
385
|
7 |
Zirconia/multi-walled carbon nanotubes |
4.94 |
3 |
2 |
5 |
10 |
Yes |
No |
— |
— |
EDX, SEM, FTIR, BET, XPS |
99.67 |
Small regeneration |
386
|
8 |
Single-walled carbon nanotubes |
3 |
5 |
0.5 |
1.16 |
1 |
— |
— |
— |
— |
— |
58 |
— |
387
|
9 |
Iron-titanium oxide |
47 |
— |
1 |
12 |
50 |
Yes |
No |
— |
— |
EDS, BET, SEM |
98.7 |
— |
388
|
10 |
Mn–Ce powder |
79.5 |
6 |
0.01 |
3 |
6 |
— |
— |
— |
— |
FTIR, XPS |
90 |
92 |
389
|
11 |
Iron oxide-hydroxide nanoparticles |
16.7 |
7.28 |
1 |
3 |
10 |
No |
Yes |
— |
— |
— |
80 |
76 |
390
|
12 |
Magnesium oxide coated magnetite nanoparticles |
10.96 |
6 |
2 |
2.5 |
13.6 |
Yes |
No |
No |
Yes |
SEM, EDAX |
98.6 |
— |
391
|
13 |
Nano-scale aluminium oxide hydroxide |
11.88 |
7 |
1.6 |
1 |
20 |
Yes |
No |
No |
Yes |
XRD, TGA |
95 |
— |
392
|
14 |
Nanoscale zero-valent iron |
18.91 |
4 |
0.6 |
0.58 |
— |
No |
Yes |
— |
— |
— |
84 |
— |
393
|
15 |
Hybrid Fe–Ce–Ni nanoporous adsorbent |
285.7 |
7 |
0.4 |
0.5 |
10 |
No |
Yes |
No |
Yes |
— |
98.7 |
95 |
394
|
16 |
MgO nanoparticles loaded with mesoporous alumina |
37.35 |
6.8 |
3 |
8 |
30 |
Yes |
Yes |
No |
Yes |
XRD, NAD, TEM, BET, BJH, HRTEM, EDS, PZC |
90 |
— |
395
|
17 |
Cupric oxide nanoparticles |
357 |
5 |
0.05 |
1.33 |
20 |
No |
Yes |
No |
Yes |
— |
89 |
— |
396
|
18 |
Nanoparticles of gamma alumina |
14 |
7 |
0.1 |
1.5 |
— |
Yes |
No |
No |
Yes |
TGA, DSC, XRD, FESEM, FTIR, BET, SEM |
85 |
— |
397
|
19 |
Nano-sized hydroxyapatite on to graphene oxide seeds |
44.06 |
3–7 |
0.5 |
0.42 |
50 |
No |
Yes |
No |
Yes |
XRD, TEM, SEM, EDAX, FTIR |
96.3 |
95 |
398
|
20 |
Nano gamma alumina |
32 |
4 |
1 |
1 |
10 |
No |
Yes |
No |
Yes |
XRD, BET, SEM |
96 |
98 |
399
|
21 |
Single-walled carbon nanotubes |
2.4 |
5 |
0.5 |
0.5 |
1 |
No |
Yes |
No |
Yes |
SEM, BET, TEM, XRD, FTIR |
58 |
— |
400
|
22 |
HA-multi-walled carbon nanotubes |
39.22 |
7 |
0.25 |
3 |
5 |
Yes |
Yes |
No |
Yes |
SEM, TEM, XRD,BET, FTIR |
— |
— |
401
|
23 |
Single-walled carbon nanotubes |
16.63 |
6 |
0.6 |
0.42 |
1 |
Yes |
No |
No |
Yes |
— |
100 |
— |
402
|
24 |
Tritinate nanotubes |
58 |
2 |
1 |
2 |
10 |
Yes |
No |
No |
Yes |
— |
95 |
— |
403
|
The following sections examine the various strategies for removing F−, and Table 9 summarises the areas of interest and limitations of each methodology. The following observations were concluded regarding the different methodologies for fluoride removal based on the literature reviewed.
Table 9 Various methods for F− removal and their advantages and disadvantages
Method |
Advantages |
Disadvantages |
Adsorption |
• Cost effective |
• To achieve good and adjustment of pH is required |
• Higher accessibility |
• Fluoride adsorption are sometime hundred due to some other ion present |
• Easy operation |
• Reduce in removal % of regenerated adsorbent |
• Large number of adsorbents |
• Interference of other inorganic ions |
• Locally available and cheap |
• Treated water comprises greater total dissolved solids (TDS) |
Ion exchange |
• High efficiency |
• Costly |
• Removal efficacy up to 95% |
• Easy exposed to interfering ions (bicarbonate, sulphate, chloride etc.) |
• Colour and taste of water continue complete |
• Filter media to replace after multiple recycle, presence of toxic solid waste used filter media, development of toxic liquid waste due to the regeneration of media, efficiency depends to large extent on pH |
• Highly effective and efficient process |
• Treated water becomes acidic |
• Low running cost |
• Treated water exhibits greater chloride concentration |
Coagulation/precipitation |
• Efficiency is high |
• Costly and largely depends on the co-ion and also pH of water |
• Chemical used are available commercially |
• High amount of residual aluminum obtained |
• Work at domestic and community level |
• Sludge obtained contain toxic aluminum fluoride complex |
• Simple design |
• Large amount of water is retained and hence dewatering is before disposal |
• Simple operation process |
• High chemical dosages |
Reverse osmosis |
• Quick regeneration of membrane |
• High cost |
• N > 90% helping in eliminating other dissolved solids also wide range of pH |
• Saline solution is used to treat water |
• Chemical process is not used |
• Salt may be creating issue |
• Work under wide range of pH |
• Valuable mineral is needed after treatment for demineralization |
• No chemical assist |
• Needs pH enhancement |
Nano-filtration |
• No chemical is used |
• High cost |
• Productive is very high |
• Issue of membrane degradation scaling and fouling |
• No ion interference is noted |
• Some ions helpful in ordinary development also get removed |
• Divider for organic micro pollutant |
• May necessitate remineralisation of treated water |
• Inorganic toxic suspended solid |
• Discards all vital minerals |
(1) A perusal of the above-mentioned table shows that adsorption gives favorable results in efficiency, technology, and cost, but the main issue is the dumping of the sludge generated in the process.
(2) The ion-exchange method removes fluoride very efficiently, but the treated water is found to have a high chloride residue, and this method is also expensive.
(3) The coagulation/precipitation technique has the limitation of fluoride removal of up to 33% and also produces a large quantity of residue, having an excess amount of residual aluminum.
(4) Reverse osmosis and nanofiltration techniques have higher efficiency for fluoride removal, but they are expensive and few essential ions are removed.
(5) The electrocoagulation technique is the most suitable for removing fluoride if the above-mentioned issues are considered, but the continuous supply of power required is one of its drawbacks.
(6) In the last few years, the adsorption technique for the removal of fluoride has attracted significant attention due to the very high adsorption power shown by some of the adsorbents, but the main issue is to choose an appropriate adsorbent because commonly, a particular adsorbent gives excellent adsorption in the laboratory but simultaneously fails in the field.
(7) Lastly, it should be noted that every technique has limitations, and hence a single technique is not suitable for all countries because different countries have different concentrations of fluoride and other ions present in their groundwater.
A broad overview of the available technologies for fluoride removal and the advantages and limitations of each one were presented based on a literature survey and the experiments conducted in the laboratory with several processes. It has been concluded that the selection of the treatment process should be site-specific according to the local needs and prevailing conditions given that each technology has some limitations and no one process can serve this purpose in diverse conditions.
6.2 Defluoridation through biological mediators
6.2.1 Biosorption.
Because of the constraints associated with traditional defluoridation approaches, microbial treatment or biosorption of F− has become an economically feasible and ecologically beneficial alternative in recent years.404 Numerous skeletal fluorosis adsorbents have been created for groundwater defluoridation, which can be divided into several categories, including bacteria, algae, fungus, and agricultural goods.405 There has been a constant increase in the interest in overcoming the many technological challenges preventing biosorption systems from being commercially viable. The cost-effectiveness, availability, practicality, and defluoridation efficiency of a specific type of bio adsorbent play a role in its selection.404 The contaminants linked to biomass are often eradicated, and the bio-adsorbents are repeatedly rejuvenated.405 Granular bio-adsorbents are helpful because they work similarly to ion exchange resins.406 Non-living algal biomass with more excellent F adsorption capabilities, such as Ulva fasciata,407Spirogyra IO2,408Spirogyra IO1,409 and Nostoc sp. BTA394 (ref. 410) has been observed. Dead biomass is preferable over live microbes because it requires less care and nutrients.411 Although Mukherjee et al.410 employed live Nostoc sp. (BTA934) microalgae to remove fluoride, defluoridation by utilizing live microalgal species is uncommon.
6.2.2 Bioremediation.
Bioremediation of F− utilizing bacterial strains is still in progress.410 Different resistance mechanisms, namely bioaccumulation and biotransformation, allow some bacterial species to tolerate greater F− concentrations.405 However, there are few reports on the possible bioremediation of F utilizing live bacterial cells. Acinetobacter sp. RH5, Pseudomonas aeruginosa, and KX926492 are the bacterial strains employed for the successful defluoridation of wastewater.412 The complexation of the F− ion may be enabled by critical functional elements in biomass molecules, such as acetamido and sulfhydryl carboxyl.413 Active absorption of F− ions by ionophores is followed by creating organic/inorganic fluoro-complexes within the cytoplasm during defluoridation with bacteria. F− ions are transported to the cell vacuole, and deposited as permitted F− complexes or ions in the second step.405 Both the biomass production and the specific F− absorption rate are affected by the concentration of F− ions.414
Fungi are more suitable for defluoridation than bacteria because they have shorter multiplication cycles and more natural growing procedures. Fungal biomass has a high proportion of cell-wall components, which increase its surface area and diversity of functional groups, supporting its biosorption capacity.415 The fungal classes with defluoridation possibly comprise Pleurotus ostreatus 1804.416
Defluoridation potential has also been boosted by the waste produced and processed from agricultural items such as fruits and crops.417 Agricultural leftovers have unique chemical compositions including lipids and hemicellulose and are cost-effective and ecologically friendly. Some functional groups in these polymers promote F-binding through biosorption.405
6.2.3 Phytoremediation.
Phytoremediation is a low-cost and efficient remediation approach for decontaminating soils from contaminants.418 According to the suggestion by Agarwal and Chauhan,419 the highest bioaccumulation of F− of 9.948 mg kg−1 was found in the leaves of Hordeum vulgare diversity RD 2052, while the lowest bioaccumulation of F− of 6.302 mg kg−1 was found in the grains of Hordeum vulgare diversity RD 2052 in the presence of 18 mg kg−1 NaF.
Boukhris et al.420 found that the fluoride accumulation in aerial native plants in the soil was more in coastal superphosphate factories, which was in the range of 37 and 360 mg kg−1. Spirodela polyrhiza was shown to remove 12.71–19.87% F− from 3 mg F− L−1 polluted water by Karmakar et al.421
6.3 Integrated methods
Integrated methods, which combine two or more procedures to improve the F− decontamination efficiency, are gaining traction compared to employing a single technology. When the sprouting root of Amaranthus was submerged in F−-contaminated water containing 0.1 mM, 1 mM, and 10 mM FeCl3, Kapenja et al.422 showed an increase in F coagulation. After only 12 hours, the removal efficiency was noted to be 20%, 37%, and 40%, respectively, but it was just 10% without the plant. The development of Fe(III) oxide coating on the root surface may improve the removal effectiveness by containing organic molecules and CO2. When altered with various microbial consortiums, P. juliflora was clarified to have a bioaccumulation factor in the range of 0.12 to 3.3.418 From a starting concentration of 20 mg L−1, the SABA approach employing leaves and Citrus limetta peels together with the bacteria lowered the F level below 1.5 mg L−1.423
7. Regeneration issue
Further regeneration studies are needed to recover the adsorbent under field settings and increase the economic viability of the process. Based on this review, four technological adsorption techniques can be considered, as shown in Fig. S7,† including nano-surface effect, structural memory effect, anti-competitive adsorption, and ionic sieve effect. These four methodologies for designing adsorbents may considerably increase the fluoride removal efficiency in water and give guidelines for developing novel efficient fluoride removal technologies.
8. Sustainable technologies for the removal of fluoride
A worldwide challenge prevailing nowadays is the unavailability of safe water for consumption. The goal was to halve the “environmental sustainability” by the end of 2015 by providing safe and pure water for drinking. In this case, to provide safe, clean, high-quality water, the 6th United Nations Sustainable Development Goal (UNSDG) was aimed at 2.8 billion people by the year 2030. It was found that a minimum sustainable option was present for removing fluoride in developing countries given that they are concerned about its high impact. The WHO has limited the minimum concentration level of fluoride in drinking water to 1 mg L−1 to prevent dental decay, a deliberating topic and matter of concern.424
Fluoride-containing sludge is manufactured from municipal and industrial wastewater. Water fluoridation can lead to fluoride levels in the municipal wastewater treatment plant (MWTP), and it is observed that most fluoride is released from industrial wastewater.425 The projection of wastewater discharge was estimated to be between 75–80% for the supplied water, which infers that the water obtained will accumulate in the wastewater treatment plant (WWTP). The above-mentioned process suggests that the fluoride level of the WWTP depends on the overall grade. The non-accumulated water is targeted as a section of run-off and pipe leaks that enter the environment without any full-fledged treatment. Later, the fluoride constrains the process of nitrification where the fluoride removal is not reported in the primary treatment phase.426 The leading cause of this action is the availability of bacteria in the anaerobic digestion, which are very sensitive towards fluoride, resulting in about 50% reduced microbial metabolism.426 Therefore, it can be inferred that the level of fluoride removal in persistent pollution in conventional municipal wastewater treatment plants (CMWTP) also leads to disadvantageous effects on WWTP efficacy.
The above-mentioned industrial procedures generate fluoride as wastewater effluents. Therefore, various technologies were formulated for removing fluoride and even for its reuse. It was found that the fertilizer industries used precipitation to remove fluoride in their effluents and recovered fluoride to obtain economic welfare. Sorbents were used for water containing fluoride. The absorbent and sludge-producing water treatment were responsible for synthesizing a high quantity of waste using landfill disposal. Another method for sludge disposal besides landfill is incineration, which is responsible for sterilizing and reducing the weight and volume, and later transforming sludge into ash, basically burning substances at 1000 °C.427 The substantial leaching of sewage sludge ash varies given that it contains inert waste, namely fluoride. Therefore, it is observed that highly soluble fluoride-containing waste is not suitable for direct discharge in the sanitary landfill. The most convenient source to avoid fluoride leaching is pre-treating the waste through stabilization and solidification. This pre-treatment method for fluoride-containing water is usually employed in pesticide industry.428
Hence, redirecting fluoride sludge to its recovery and reuse is emerging nowadays. Recusing calcium fluoride sludge (CFS) has garnered interest from researchers worldwide because of the harmful and pollution potential of fluoride and the sudden decline in landfill capacity. The possibility of replacing cement with CFS has also been considered.429
The respective features represent that the development of fluoride treatment is based on its incineration and landfill disposal. However, it is observed that the recent methodologies do not guarantee absolute its confinement and reintroduce the contaminant in a different way in the environment, finally requiring further development. The contemporary methods are responsible for slowing the entry into the background again. The recovery of fluoride is still limited in practice and technical aspects, and thus investigations are an essential but continuous challenge towards sustainability. It has been found that the mechanism of disposal is not a sustaining aspect with the rapid growth of waste volume in developing countries.
Regulatory bodies play a significant role in defining the characteristics and parameters of fluoride. The fluoride regulation for drinking water varies from country to agency. However, it is the same regarding the standard for effluents and environmental levels. According to the consent of public health, there are varying perspectives on the level of fluoride based on human health, from non-nutrient to micronutrient and essential nutrients.430 A literature survey revealed that regular investigations are carried out to overcome the negative effects and more researchers are interested in this area based on its valuable aspects. The use of fluoride-containing dentifrice shows minimum risk, whereas the use of municipal fluoridation is a severe threat, which requires policy making. Thus, an organized definition of fluoride is needed with perceptible objectives.
According to the analysis done in the literature, the shift in the linear economy (LE) and circular economy (CE) shows great potentials. As an efficient specification of sustainability development (SD), the CE is not capable of coping with economic stability and is included for environmental protection and social equity.431 The economic benefits are the main reason for CE, highlighting the ecological and the beneficial social aspects, generating the momentum for the use of the CE. The maintenance of public health is recognized as an important node on the web. The use of fluoride may lead to hazardous health issues, creating public health problems that also impact macroeconomics. The environmental degradation arising because fluoride affects plants, animals, agriculture, aquaculture, and stocks shows economic and ecological connections. The protection method shows more expenditure efforts and has benefited the economy. Many scholars from the last few decades have already reported the poverty–environmental–deterioration relation, whose web linkage cannot be overestimated or neglected.432 Environmental deterioration (ED) can be a problem for public health, affecting the poorer class and posing socio-economic and ecological impacts. Therefore, the deterioration in the lifestyle of people with health issues has deteriorated the unexploited labour quality in the entire country.
Finally, artificial municipal water fluoridation is a significant problem and is considered the critical node for the network of fluoride, showing enormous considerable aspects. The re-consumption of fluoride from industrial by-products helps adhere to the economic benefits. It is believed that municipal fluoridation is not capable of reflecting the principle of CE. Given that the fluoride is retrieved from a fluoride conduit, it finally ends up at the disposal area, making its reuse economically appealing. Water is the natural medium for transporting fluoride in various forms such as agriculture, food, and beverages.
The fluoridation technique may undergo social justice because of ethical problems and disregarding the poor. The method of artificial fluoridation is practiced for the unprivileged society without any access to dental care. However, a lack of understanding and knowledge can also avoid the primary objective of helping the poor. According to a literature study, the fate of fluoride in humans and its severe effect leads to disease. The resource governance is working to develop advanced resource flows and involves comprehensive aspects in a diverse range.433 Following the CE, it is recommended to shift from artificial fluoridation towards other fluoride applications.
Technological development plays a significant role in improving the recovery of fluoride and emerges as a challenging aspect for the disposal of fluoride. Multiple applications must pose inclusive, deliberate, and aligned principles. Thus, the decision-making body needs to create a strong and mutual linkage to attain this task.
Therefore, the critical problem of developing sustainable defluoridation technologies confronts the global scientific assets for a long duration.
9. Preventive procedures
A highly organized way to deal with the problem based on fluoride is represented in Fig. 6. For the prevention and management of excessive fluoride ingestion, the following actions should be implemented:
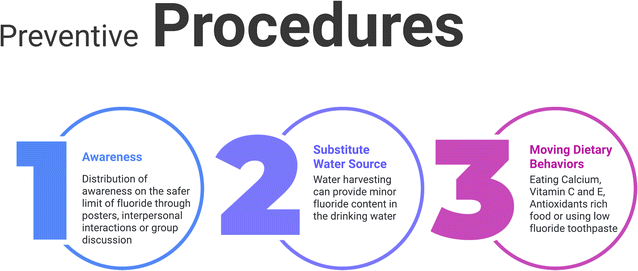 |
| Fig. 6 Schematic diagram of preventive procedures. | |
9.1 Awareness
Raising the awareness on the safer limit of fluoride content in drinking water and about its incurable health effects using posters, interpersonal interactions and group discussion in villages and school, and other is the first step in preventing the adverse health effects of fluoride contamination.
9.2 Substitute water source
Water harvesting is the best technique for providing residents with a minor fluoride content in drinking water. In general, groundwater contains a higher fluoride level than surface water utilized after typical surface water treatment.
9.3 Moving the dietary behaviors
If the amelioration procedure fails to reduce the fluoride level to an acceptable level, residents may take the following precautions by changing their eating habits.
9.3.1 Calcium.
In fluorosis areas, eat calcium-rich foods such as curd and sesame seeds. Fluoride absorption is lowered when the calcium consumption was high.
9.3.2 Vitamin C and E.
Vitamin C and E have been shown to prevent oxidative stress and endometrial injury in fluoride-intoxicated rats.434 Vitamin C is abundant in oranges and tomatoes, whereas vitamin E is found in almonds and cereals, among other foods.
9.3.3 Antioxidants.
Antioxidants such as ginger, pumpkin, and others have an aggressive action, preventing fluorosis.435
9.3.4 Toothpaste.
4–6 year-old youngsters in fluorite belt areas may employ toothpaste with a low fluoride content for dentifrices.436 Various dental specialists recommend using toothpaste with a low fluoride concentration (500–550 ppm) instead of the conventional fluoride concentration (1.0–1.1 ppm).437
10. Fluorosis mitigation programmes
Fluorosis mitigation is gaining a better awareness of the health effects of high fluoride ingestion via education, communication, and information. Since the late 1980s, government and non-governmental groups have attempted to fight fluorosis. However, given that most activities concentrate on water defluoridation, it cannot be recommended as the only or most effective method of fluoride prevention. The current research focuses on skeletal fluorosis, which has become more common in recent years. It also includes fresh discoveries and existing techniques for battling skeletal fluorosis and data that may aid research into successful fluorosis treatment and the development of cost-effective and straightforward methods for fluoride removal from water.
10.1 Worldwide scenario
If fluoride mitigation is deemed necessary, one or more of the following options438 may be used: providing a new or alternative source of water with acceptable levels, blending the existing water supply with another containing lower levels of fluoride, providing bottled water, treating the level of water at the domestic level in small treatment devices such as a domestic defluoridation unit, treatment of water at the point-of-use at the industrial level, and treatment of water at the point-of use. Table S4† shows the various fluorosis mitigation programs that have been implemented across the globe.439
10.1.1 Defluoridation unit in Ngurdoto Village, Tanzania.
The Tanzanian Water Authorities, University of Dar es Salaam, and the Technical University of Denmark collaborated to initiate this defluoridation initiative. Due to its economic efficiency and simplicity of handling, the Nalgonda process was used to defluoridate this project. However, this approach had a few drawbacks, as follows: (1) fluoride limits that exceeded the standard ranges of the process and (2) the addition of lime in the suggested concentration raised the pH to levels that were much higher than the ideal pH necessary for fluoride removal.
A device with taps and a cotton cloth put on the sieve to which alum and lime were introduced concurrently and combined is known as the “two-bucket” defluoridation procedure. Flocculation occurs after quick mixing, gradual stirring, and settling for roughly 1 hour. Few observations have shown that the fluoride trapped in the flocs may be released back into the water; hence, the two buckets are utilized to guarantee that the treated water is quickly separated.440
10.1.2 ICOH mobile bus unit project.
This initiative began in Thailand, intending to raise public awareness about the harmful effects of fluoride on human health, conduct on-site analyses of fluoride levels in water samples, and resolve the issue with feasible alternatives. The team is comprised health experts who work to raise awareness among the general public.
10.1.3 National rural drinking water program.
The Government of India initiated this initiative on April 1, 2009, intending to provide enough clean water to all rural residents for drinking, cooking, and other basic requirements. It has been implemented in all states and the Andaman and Nicobar Islands and Pondicherry, which are both union territories. In 2017, the program was restructured to be more result-oriented and outcome-specific. By 2030, the program's objective is to provide universal and equal access to clean and inexpensive drinking water.
10.2 Indian scenario
The Indian government has established many nationwide programs to provide the population with clean drinking water and to tackle the issue of fluorosis, including Project SARITA and Sachetana Plus. Various government and non-governmental organizations have worked to combat fluorosis during the past several decades. Table S5† gives a quick summary of the fluorosis mitigation programs in India.439
11. Role of the dentist in fluorosis mitigation
The role of dentists in fluorosis prevention, particularly public health dentists, is because they are uniquely qualified to address the root of this problem. Dentists may teach and urge patients to adopt safe drinking water habits as part of their usual clinical practice. Public health dentists may use their experience and numerous educational resources to educate people about the dangers of fluorosis, highlighting the necessity of drinking clean water via regular surveys or at dental camps. Public health dentists may play a critical role in policy development and implementation, such as the NRHM, NRDWP, and other initiatives.441
Combating skeletal fluorosis on a large scale remains a severe challenge due to the lack of awareness and costly therapy. Thus, research should focus on developing cost-effective and straightforward methods for treating skeletal fluorosis or removing this element from drinking water.
Not only does public knowledge play an essential role in the containment of this huge hazard, but also government and non-governmental organizations. As part of their curriculum, pupils are taught fluorosis mitigation measures, as an effort of the Rajasthan Government in India to raise awareness among young people. During regular surveys, public health officials should come across afflicted patients. It is necessary to organize camps and programs to educate the public and raise awareness about the dangers of fluorosis and the need to drink healthy water. In locations where water seems to be the primary cause of skeletal fluorosis, and there is no alternative water supply and health insurance, and thus the medical community should be educated to handle this issue and limit its effects. The National Rural Health Insurance Program is one such insurance scheme (under NRHM). Besides the steps mentioned above, healthcare staff should be well trained to diagnose fluorosis and prevent preventative actions accurately.
12. Perspective and future research direction
Here, we provide perspectives for fluoride removal and suggest water management and future research-based directions, as follows:
(1) There is little literature study on the data on fluoride levels in the environment and the monitoring of it. Furthermore, there is no recent literature study.
(2) Water contamination with fluoride ions is a significant health-related problem in arid and semi-arid areas. The fluoride concentration in groundwater is 20 mg L−1 and even more in some regions of China, India, and North Africa. This level is considerably more than the limit set by WHO, which is 1.5 mg L−1.
(3) The contaminants in drinking water lead to various disease and health-based issues such as skeletal, dental, and non-skeletal fluorosis, which is caused by fluoride in groundwater. Because of its effect, WHO has limited the concentration of fluoride to 1.5 mg L−1, and the BIS has determined it to be less than 1 mg L−1.
(4) Various methods for fluoride ion removal have been proposed for reducing its acuity towards damage to human health and the environmental risk. However, due to the high variability of fluoride in drinking water, the conventional methods are expensive and inadequate. Hence, there is an urgent need to develop efficient and cheap strategies for fluoride water treatment.
(5) Many technologies are shown on the laboratory scale. However, wastewater with a high fluoride concentration has a critical effect on the environment and human health. This is later worsened by the inter-connection of the water-based ecosystem, which causes the toxic fluoride to be consumed in food and during recreational activities.
(6) Developing highly efficient and enhanced methods is required to generate more sanitation and treatment abilities. The developing techniques show higher potential for the 6th UNSDG.
(7) According to the literature, there is not enough data for interpolating or extrapolating the information based on different methods. The literature collected from various sources states fluoride removal by employing adsorption. However, there is very little specified about it and limited discussion on the cost-effective adsorbents.
(8) The bioremediation technique is recognized for the indirect use of fluoride-containing effluent in WWT as the organisms have certain requirements and limited fluoride resistance. Bioremediation is integrated with water management by trapping free fluoride on the surface of water, avoiding the leaching of fluoride into the soil. The recent studies suggest the feasibility and sustaining material recovery of fluoride by the process of phytoremediation.
(9) The toxicity of fluoride is minimized through early diagnosis and reduced intake, generating enhanced nutrition in terms of calcium and vitamin D in the diet. Special attention is given to individuals living with painful and vulnerable conditions, resulting in physical, cultural, and social downfall.
(10) Highly developed research studies are needed for developing and implementing cost-effective, hybrid technology and sustaining features to eliminate the problems based on fluoride. Specific knowledge on fluoride disposal from groundwater is lacking, but it is observed that an innovative encapsulation method for fluoride isolation shows minimum risk and has a practical solution, which needs to be developed worldwide.
(11) Defluoridation needs various features to be followed, such as low-cost, highly advanced systems, with low waste, low procedure wastage, and high use of available waste on a large-scale basis.
(12) The content of fluoride arises from the food chain, and hence the nutritional status of most Indians is inferior. According to the facts listed, it is observed that fluoride contamination in India needs to be minimized to avoid hazardous health issues.
13. Conclusion
Fluoride-contaminated water is a severe concern affecting human health, requiring highly effective environmental protection and water management. Given that anthropogenic factors release fluoride in the environment, it is a severe health issue, and most of the problems result from ingesting fluoride from natural resources. The guide of the UNSDG for clean drinking water shows a cost-effective, efficient, viable method to remove fluoride and is mandatory. This method avoids the fundamental drawbacks. It is noticed that it is hard to provide precise and substantial criteria due to a lack of adequate data. Few researchers cannot focus on developing specified methods given that they combine various techniques to create synergistic methods for removing fluoride. This is responsible for bringing about growth and development in water engineering by employing the present and suitable methods, surpassing most minor efforts for practical use. Together with the literature, the narrative of this study is limited to fluoride removal method from aqueous solution, which cannot transcend the investigation on its recovery and re-use, and disposal of secondary polluting agents. The fluoride removal effectiveness varies depending on site-specific chemical, topographical, and economic factors; therefore, real applications may differ from the assumptions suggested. Any acceptable procedure in one location may not fulfil the standards in another. Consequently, all techniques should be evaluated using natural water treatment. Thus, local authorities and communities need to concentrate on community-based treatment methods. Local communities should be notified and informed to assist in developing appropriate and low-cost curative technologies. Defluoridation of water and minimizing fluoride consumption are also necessary for the integrated mitigation of fluorosis. There is a need to raise awareness in fluorosis-prone regions about the need to eat foods with a low fluoride content and avoid meals with high fluoride concentrations. It is recognized that a comprehensive approach to fluorosis mitigation is necessary and that an appropriate strategy for water defluoridation, fluoride consumption reduction via foodstuffs and consumable products, and nutritional supplementation may be required. Using an electrolytic defluoridation system for integrated fluorosis mitigation may be helpful in the long run. With adequate planning, monitoring, and continuous reinforcement, a public-private partnership project should be implemented based on the need. If any of these aspects fails, the initiative will only operate on paper and will not reach the intended recipients. Due to the lack of communication, initiatives and public awareness, combatting fluorosis on a large scale has remained a dream. Mass media and social media may aid in fluorosis prevention and control. A comprehensive strategy is required to address the threat posed by fluoride. However, the principle of “prevention is better than treatment” remains the ultimate answer to the fluoride problem. Furthermore, narratives from the reviewed literature are restricted in their assessment of possible fluoride recovery, re-use, and/or disposal of secondary pollutants created. Consequently, we advise future researchers to take a more thoughtful and comprehensive approach to future studies. Finally, by using a comprehensive approach to examine the complex challenges of F− pollution, our study indisputably proves the importance of diverse exposure pathways and human health hazards. Therefore, it is suggested to the upcoming researchers that they should have a more conscientious and holistic method to investigate the fluoride concentration.
Abbreviations
WHO | World Health Organization |
BIS | Bureau of Indian Standards |
ISI | Indian Standard Institution |
ICMR | Indian Council of Medical Research |
F2 | Fluorine |
F− | Fluoride ion |
HF2− | Bi-fluoride ion |
19F NMR | Fluorine-19 nuclear magnetic resonance spectroscopy |
ppm | Parts per million |
LOAEL | Lowest observed-adverse-effect level |
NOAEL | No-observed-adverse-effect limit |
UNICEF | United Nations International Children's Emergency Fund |
PO43− | Phosphate ion |
IQ | Intelligence quotient |
LH | Luteinizing hormone |
FSH | Follicle-stimulating hormone |
TH | Thyroid hormone |
EL | Estrogen level |
ER/AR | Estrogen receptor to androgen receptor |
NaF | Sodium fluoride |
CKD | Chronic kidney disease |
GPx3 | Serum glutathione peroxidise |
MDA | Malondialdehyde |
ROS | Reactive oxygen species |
EGCG | Epigallocatechin gallate |
Mip 2 | Macrophage inflammatory protein 2 |
US | United States |
UK | United Kingdom |
HF | Hydrofluoric acid |
DNA | Deoxyribonucleic acid |
CER | Ceralite IRA 400 |
IND | Resin FR 10 |
AIAA | Alum-impregnated activated alumina |
EDAX or EDS or EDX | Energy-dispersive analysis of X-rays |
MAAA | Magnesia-amended activated alumina |
XRD | X-ray powder diffraction |
SEM | Scanning electron microscopy |
BET | Brunauer–Emmett–Teller |
TEM | Transmission electron microscope |
FITR | Fourier transform infrared spectroscopy |
ATR-FTIR | Fourier transform infrared-attenuated total reflectance |
HRTEM | High-resolution transmission electron microscopy |
TGA | Thermogravimetric analysis |
EXAFS | Extended X-ray-absorption fine-structure |
MMS | Microfluidic modulation spectroscopy |
GAPA | Gas adsorption porosimetry analysis |
XPS | X-ray photoelectron spectroscopy |
IRS | Infrared spectra |
UV-Vis | Ultraviolet-visible spectroscopy |
XRF | X-ray fluorescence |
ASAP | Atmospheric solids analysis probe |
TG-DTA | Thermogravimetric analysis-differential thermal analysis |
IC | Ion chromatography |
DFT | Density-functional theory |
ICP-OES | Inductively coupled plasma-optical emission spectroscopy |
FESEM | Field emission scanning electron microscopy |
SAA | Surface area analyzer |
ZPC | Zero point of charge |
BJH | Barrett–Joyner–Halenda |
PZC | Point of zero charge |
CEC | Cation exchange capacity |
ICP-AES | Inductively coupled plasma-atomic emission spectrometry |
NAD | Nicotinamide adenine dinucleotide |
DSC | Differential scanning calorimetry |
HAP | Hydroxyapatite |
GFH | Granular ferric hydroxide |
Author contributions
Shaz Ahmad: conceptualization, investigation, data analysis, visualization, writing – original draft; Reena Singh: supervision, project administration, writing – review & editing; Tanvir Arfin: writing – review & editing; Krishna Neeti: investigation, data analysis, visualization, writing – original draft.
Conflicts of interest
The authors declare that they have no known competing financial interests or personal relationships that could have appeared to influence the work reported in this paper.
Acknowledgements
This research did not receive any specific grant from funding agencies in the public, commercial, or not-for-profit sectors. Authors acknowledge the Knowledge Resource Centre, CSIR-NEERI (CSIR-NEERI/KRC/2021/MARCH/HZC/1) for their support.
Notes and references
- H. Nakayama, Y. Yamasaki and S. Nakata, J. Hydrol., 2022, 612, 128026 CrossRef CAS.
- S. H. Avila-Rojas, O. E. Aparicio-Trejo, M. A. Sanchez-Guerra and O. C. Barbier, Environ. Toxicol. Pharmacol., 2022, 94, 103916 CrossRef CAS PubMed.
-
The Guidelines for Drinking-Water Quality, https://apps.who.int/iris/handle/10665/43428, accessed October 2021 Search PubMed.
- X. Tang, C. Zhou, W. Xia, Y. Liang, Y. Zeng, X. Zhao, W. Xiong, M. Cheng and Z. Wang, Chem. Eng. J., 2022, 446, 137299 CrossRef CAS.
- M. Rizzu, A. Tanda, C. Cappai, P. P. Roggero and G. Seddaiu, Sci. Total Environ., 2021, 787, 147650 CrossRef CAS.
- D. Raj and E. Shaji, Geosci. Front., 2017, 8, 117–124 CrossRef CAS.
- N. J. Raju, S. Dey, W. Gossel and P. Wycisk, Hydrol. Sci. J., 2012, 57, 1433–1452 CrossRef CAS.
- C. Singaraja, S. Chidambaram, P. Anandhan, M. V. Prasanna, C. Thivya, R. Thilagavathi and J. Sarathidasan, Appl. Water Sci., 2014, 4, 241–250 CrossRef CAS.
- P. Patel, N. J. Raju, B. S. R. Reddy, U. Suresh, W. Gossel and P. Wycisk, Environ. Earth Sci., 2016, 75, 1–24 CrossRef CAS.
- S. Ali, S. K. Thakur, A. Sarkar and S. Shekhar, Environ. Chem. Lett., 2016, 14, 291–315 CrossRef CAS.
- A. Mohammad and C. B. Majumder, Int. J. Renew. Energy Technol., 2014, 3, 776–785 Search PubMed.
- B. B. Mehari, A. O. Mayabi and K. B. Kakoi, Environ. Nat. Resour. Res., 2014, 4, 67–82 Search PubMed.
- S. S. Waghmare, T. Arfin, D. Lataye and S. Rayalu, Int. J. Innovat. Res. Sci. Eng. Technol., 2015, 4, 11998–12010 Search PubMed.
-
G. Jacks, in Groundwater Assessment, Modelling, and Management, ed. M. Thangarajan and V. P. SinghCRC Press, Boca Raton, 1st edn, 2016, ch. 22, pp.339–349 Search PubMed.
- D. Kanduti, P. Sterbenk and B. Artnik, Mater. Sociomed., 2016, 28, 133–137 CrossRef PubMed.
- S. S. Waghmare, T. Arfin, D. Lataye, S. Rayalu, N. Manwar and N. Labhsetware, Int. J. Adv. Res. Innov. Ideas Educ., 2015, 1, 904–925 Search PubMed.
- S. S. Waghmare and T. Arfin, Int. J. Mod. Trends Eng. Res., 2015, 2, 355–361 Search PubMed.
- S. Jagtap, M. K. Yenkie, N. Labhsetwar and S. Rayalu, Chem. Rev., 2012, 112, 2454–2466 CrossRef CAS PubMed.
- S. Waghmare, T. Arfin, N. Manwar, D. Lataye, N. Labhsetwar and S. Rayalu, Asian J. Adv. Basic Sci., 2015, 4, 12–24 Search PubMed.
-
T. Arfin and A. Tarranum, in Handbook of Nanomaterials for Industrial Application: An Overview, ed. C.M. Hussain, Elsevier, Netherlands, 2018, ch.6, pp. 127–134 Search PubMed.
-
Naturally Occurring Levels of Fluoride in Fresh Food, https://fluoridealert.org/content/fresh_foods/, accessed October 2021 Search PubMed.
- M. A. Tikki, Int. J. Sci. Eng. Res., 2014, 5, 515–519 Search PubMed.
- A. K. Susheela, K. T. Das, I. P. Gupta, R. K. Tandon, S. K. Kacker, P. Ghosh and R. C. Deka, Indian Acad. Sci., 1992, 25, 5–22 Search PubMed.
- S. Ayoob and A. K. Gupta, Crit. Rev. Environ. Sci. Technol., 2006, 36, 433–487 CrossRef CAS.
- K. F. Fung, Z. Q. Zhang, J. W. C. Wong and M. H. Wong, Environ. Pollut., 1999, 104, 197–205 CrossRef CAS.
- M. P. Whyte, K. Essmeyer, F. H. Gannon and W. R. Reinus, Am. J. Med., 2005, 118, 76–81 CrossRef PubMed.
- J. Cao, X. Bai, Y. Zhao, J. Liu, D. Zhou, S. Fang, M. Jia and J. Wu, Fluoride, 1996, 3, 139–142 Search PubMed.
- P. Gulatit, V. Singh, M. K. Gupta, V. Vaidya, S. Dass and S. Prakash, Sci. Total Environ., 1993, 138, 213–222 CrossRef.
- J. Cao, X. Bai, Y. Zhao, J. Liu, D. Zhou, S. Fang, M. Jia and J. Wu, Environ. Health Perspect., 1996, 104, 1340–1343 CrossRef CAS PubMed.
-
Health Effects of Ingested Fluoride, https://www.nap.edu/catalog/2204/health-effects-of-ingested-fluoride, accessed October 2021 Search PubMed.
- Y. Haikel, P. M. Cahen, J. C. Turlot and R. M. Franky, J. Dent. Res., 1989, 68, 1238–1241 CrossRef CAS.
-
Guidelines for Drinking-Water Quality, https://www.who.int/water_sanitation_health/dwq/2edvol2p1.pdf, accessed October 2021 Search PubMed.
- S. L. Gu, J. Rongli and C. Shouren, Biomed. Environ. Sci., 1990, 3, 384–390 CAS.
-
Appropriate Use of Fluorides for Human Health, https://apps.who.int/iris/handle/10665/39103, accessed October 2021 Search PubMed.
-
Toxicological Profile for Fluorides, Hydrogen Fluoride, and Fluorine, https://www.atsdr.cdc.gov/toxprofiles/tp11-p.pdf, accessed October 2021 Search PubMed.
-
Fluorides, https://inchem.org/documents/ehc/ehc/ehc227.htm, accessed October 2021 Search PubMed.
-
Fluorine in Swedish Agricultural Products, Soil, and Drinking Water, https://www.cabdirect.org/cabdirect/abstract/19541400280, accessed October 2021 Search PubMed.
- M. Watanabe, Y. Yoshida, M. Shimada and K. Kurimoto, Br. J. Ind. Med., 1975, 32, 316–320 CAS.
- L. S. Kaminsky, M. C. Mahoney, J. Leach, J. Melius and M. J. Miller, Crit. Rev. Oral Biol. Med., 1990, 1, 261–281 CrossRef CAS PubMed.
- H. H. Messer, W. D. Armstrong and L. Singer, J. Nutr., 1973, 103, 1319–1326 CrossRef CAS PubMed.
- G. M. Whitford and D. H. Pashley, Calcif. Tissue Int., 1984, 36, 302–307 CrossRef CAS PubMed.
- G. M. Whitford, Calcif. Tissue Int., 1994, 54, 421–425 CrossRef CAS PubMed.
- G. M. Whitford, D. H. Pashley and K. E. Reynolds, Am. J. Physiol., 1979, 236, 141–148 Search PubMed.
- D. M. O'Mullane, R. J. Baez, S. Jones, M. A. Lennon, P. E. Petersen, A. J. Rugg-Gunn, H. Whelton and G. M. Whitford, Community Dent. Health, 2016, 33, 69–99 Search PubMed.
- R. W. Dharmaratne, Hum. Exp. Toxicol., 2019, 38, 269–279 CrossRef CAS.
- R. Caldera, J. Chavinie, J. Fermanian, D. Tortrat and A. M. Laurent, Biol. Neonate, 1988, 54, 263–269 CrossRef CAS PubMed.
- J. Ekstrand, C. J. Spak and J. Flach, Caries Res., 1984, 18, 93–95 CrossRef CAS PubMed.
- S. J. Fomon and J. Ekstrand, J. Public Health Dent., 1999, 59, 229–234 CrossRef CAS PubMed.
- A. Oliveby, F. Lagerlof and J. Ekstrand, J. Dent. Res., 1989, 68, 146–149 CrossRef CAS.
- A. Oliveby, S. Twetman and J. Ekstrand, Caries Res., 1990, 24, 44–47 CrossRef CAS PubMed.
-
D. Henschler, W. Butter and J. Patz, in Calcium Metabolism, Bone and Metabolic Bone Diseases, ed. F. Kuhlencordt and H. P. Kruse, Springer Verlag, Berlin, 1975, ch. 18, pp. 111–121 Search PubMed.
- J. Ekstrand, M. Ehrnebo and L. O. Boreus, Clin. Pharm. Ther., 1978, 23, 329–337 CrossRef CAS PubMed.
- R. G. Schamschula, E. Sugar, P. S. H. Un, K. Toth, D. E. Batmes and B. L. Adkins, Community Dent. Oral Epidemiol., 1985, 13, 104–107 CrossRef CAS PubMed.
- G. M. Whitford, F. C. Sampaio and P. Arneberg, Caries Res., 1999, 33, 462–467 CrossRef CAS PubMed.
- M. A. R. Buzalaf, R. Fukushima, J. M. Granjeiro and J. A. Cury, Fluoride, 2002, 35, 185–192 CAS.
-
Fluorine and Fluorides, https://wedocs.unep.org/bitstream/handle/20.500.11822/29338/EHC36Fluorine.pdf?sequence=1, accessed October 2021 Search PubMed.
- M. Diesendorf and A. Diesendorf, Acc. Res., 1997, 5, 225–237 CrossRef PubMed.
- Y. Li, C. K. Liang, C. W. Slemenda, R. Ji, S. Sun, J. Cao, C. L. Emsley, F. Ma, Y. Wu, P. Ying, Y. Zhang, S. Gao, W. Zhang, B. P. Katz, S. Niu, S. Cao and C. C. Johnston, J. Bone Miner. Res., 2001, 16, 932–939 CrossRef CAS PubMed.
- P. Zhu, Z. Cao, Y. L. Ye, G. Qian, B. Lu, M. Zhou and J. Zhou, Waste Manage. Res., 2013, 31, 1154–1159 CrossRef CAS PubMed.
- T. Okamura and T. Matsuhisa, Hyg. Soc. Jpn., 1965, 6, 382–385 CrossRef.
- C. A. Full and F. M. Parkins, J. Dent. Res., 1975, 54, 192 CrossRef CAS.
- A. Chatterjee, S. Sarah, P. D. Sreedevi, A. Selles and S. Ahmed, Arabian J. Geosci., 2017, 10, 558 CrossRef.
- S. K. Jha, V. K. Mishra, D. K. Sharma and T. Damodaran, Rev. Environ. Contam. Toxicol., 2011, 211, 121–142 CAS.
- M. Vithanage and P. Bhattacharya, Environ. Chem. Lett., 2015, 13, 131–147 CrossRef CAS.
- G. T. Chae, S. T. Yun, B. Mayer, K. H. Kim, S. Y. Kim and J. Kwon, Sci. Total Environ., 2007, 385, 272–283 CrossRef CAS PubMed.
- D. Gastmans, A. Mira, R. Kirchheim, L. Vives, L. Rodroguez and G. Veroslavsky, Procedia Earth Planet. Sci., 2017, 17, 136–139 CrossRef.
- M. Kumar, A. Das, N. Das, R. Goswami and U. K. Singh, Chemosphere, 2012, 150, 227–238 CrossRef.
- W. B. Apambire, D. R. Boyle and F. A. Michel, Environ. Geochem., 1997, 33, 13–24 CAS.
-
A. Sarkar, S. Ali, S. kumar, S. Shekhar and S. V. N. Rao, in Groundwater Environment in Asian Cities: Concepts, Methods and Case Studies, ed. S. Shrestha, V. P. Pandey and S. Thatikonda, Elsevier, Amsterdam, 1st edn, 2016, ch. 5, pp. 77–108 Search PubMed.
- J. He, Y. An and F. Zhang, J. Geochem. Explor., 2013, 135, 22–30 CrossRef CAS.
- A. Chowdhury, M. K. Adak, A. Mukherjee, P. Dhak, J. Khatun and D. Dhak, J. Hydrol., 2019, 574, 333–359 CrossRef CAS.
- V. Saxena and S. Ahmed, Environ. Geol., 2003, 43, 731–736 CrossRef CAS.
-
W. M. Edmunds and P. L. Smedley, in Essentials of Medical Geology, ed. O. Selinus, Springer, Netherlands, 2013, ch. 13, pp. 311–336 Search PubMed.
- N. S. Rao, Hydrol. Sci. J., 1997, 42, 877–892 CrossRef CAS.
- X. Gao, Y. Wang, Y. Li and Q. Guo, Environ. Geol., 2007, 5, 795–803 CrossRef.
- P. Li, H. Qian, J. Wu, J. Chen, Y. Zhang and H. Zhang, Environ. Earth Sci., 2014, 71, 3133–3145 CrossRef CAS.
- B. Keshavarzi, F. Moore, A. Esmaeili and F. Rastmanesh, Environ. Earth Sci., 2010, 61, 777–786 CrossRef CAS.
- N. S. Rao, P. S. Rao, A. Dinakar, P. V. N. Rao and D. Marghade, Appl. Water. Sci., 2017, 7, 1467–1478 CrossRef CAS.
- H. V. Churchill, R. J. Rowley and L. N. Martin, Anal. Chem., 1948, 20, 69–71 CrossRef CAS.
- O. Araya, F. Wittwer and A. Villa, Vet. Hum. Toxicol., 1993, 35, 437–440 CAS.
- P. S. Datta, D. L. Deb and S. K. Tyagi, J. Contam. Hydrol., 1996, 24, 85–96 CrossRef CAS.
- M. Durand and J. Grattan, Lancet, 2001, 357, 164 CrossRef CAS.
- J. A. Camargo, Chemosphere, 2003, 50, 251–264 CrossRef.
- F. Shen, X. Chen, P. Gao and G. Chen, Chem. Eng. Sci., 2003, 58, 987–993 CrossRef CAS.
- G. D. L. Puente, J. J. Pis, J. A. Menendez and J. Anal, Appl. Pyrolysis, 1997, 43, 125–138 CrossRef.
- M. C. Kundu and B. Mandal, Environ. Monit. Assess., 2009, 152, 97–103 CrossRef CAS PubMed.
- K. M. K. Kut, A. Sarswat, A. Srivastava, C. U. Pittman and D. Mohan, Groundw. Sustain. Dev., 2016, 2–3, 190–212 CrossRef.
- J. Qian, Waterfront, 1999, 13, 11–13 Search PubMed.
- A. I. Alabdulaaly, A. I. Al-Zarah and M. A. Khan, Appl. Water Sci., 2013, 3, 589–595 CrossRef CAS.
- L. Borgnino, M. G. Garcia, G. Bia, Y. V. Stupar, Ph. Le Coustumer and P. J. Depetris, Sci. Total Environ., 2013, 443, 245–255 CrossRef CAS.
- A. Queste, M. Lacombe, W. Hellmeier, F. Hillermann, B. Bortulussi, M. Kaup, K. Ott and W. Mathys, Int. J. Hyg. Environ. Health, 2001, 203, 221–224 CrossRef CAS PubMed.
- V. Kimambo, P. Bhattacharya, F. Mtalo, J. Mtamba and A. Ahmad, Groundw. Sustain. Dev., 2019, 9, 100223 CrossRef.
- H. E. Shortt, G. R. McRobert, T. W. Barnard and A. S. M. Nayar, Indian J. Med. Res., 1937, 25, 553–568 CAS.
- D. Chakraborti, B. Das and M. T. Murrill, Environ. Sci. Technol., 2011, 45, 27–33 CrossRef CAS PubMed.
- S. S. Waghmare and T. Arfin, Int. J. Eng. Res. Gen. Sci., 2015, 3, 345–358 Search PubMed.
- S. Dey and B. Giri, Med. Clin. Rev., 2016, 2, 11 Search PubMed.
- D. Colin, J. Can. Dent. Assoc., 2003, 69, 722–725 Search PubMed.
- S. Peckham and N. Awofeso, Sci. World J., 2014, 2014, 293019 Search PubMed.
- W. Guissouma, O. Hakami, A. J. A. Rajab and J. Tarhouni, Chemosphere, 2017, 177, 102–108 CrossRef CAS PubMed.
- A. Narsimha and V. Sudarshan, Appl. Water Sci., 2017, 7, 2501–2512 CrossRef CAS.
- C. B. Dissanayake, Int. J. Environ. Stud., 2014, 19, 195–203 CrossRef.
- L. Kirkeskov, E. Kristiansen, H. Boggild, H. V. P. Hallermund, H. Sckerl, A. Carlsen, M. J. Larsen and S. Poulsen, Community Dent. Oral Epidemiol., 2010, 38, 206–212 CrossRef PubMed.
- S. Jones, B. A. Burt, P. E. Petersen and M. A. Lennon, Bull. W. H. O., 2005, 83, 670–676 Search PubMed.
- K. Y. Lin, J. J. Tang and C. Z. Bao, Chin. J. Endemiol., 2001, 20, 111–112 Search PubMed.
- O. Barbier, L. A. Mendoza and L. M. D. Razoa, Chem.-Biol. Interact., 2010, 188, 319–333 CrossRef CAS PubMed.
- S. L. Choubisa, Fluoride, 2001, 34, 61–70 Search PubMed.
- S. L. Choubisa, L. Choubisa and D. K. Choubisa, Indian J. Environ. Health, 2001, 43, 177–189 CAS.
- S. L. Choubisa and K. Sompura, Pollut. Res., 1996, 15, 45–47 CAS.
- P. Grandjean and P. J. Landrigan, Lancet, 2006, 368, 2167–2178 CrossRef CAS.
- S. Zhang, X. Zhang, H. Liu, W. Qu, Z. Guan, Q. Zeng, C. Jiang, H. Gao, C. Zhang, R. Lei, T. Xia, Z. Wang, L. Yang, Y. Chen, X. Wu, Y. Cui, L. Yu and A. Wang, Toxicol. Sci., 2015, 144, 238–245 CrossRef CAS PubMed.
- A. L. Choi, G. Sun, Y. Zhang and P. Grandjean, Environ. Health Perspect., 2012, 120, 1362–1368 CrossRef CAS PubMed.
- Y. M. Shivarajashankara, A. R. Shivashankara, P. G. Bhat and S. H. Rao, Fluoride, 2002, 35, 197–203 CAS.
- X. T. Cheng, S. X. Wang and J. G. Gao, Chin. J. Endemiol., 2002, 2, 357–358 Search PubMed.
- A. K. Susheela, A. Kumar, M. Bhatnagar and R. Bahudur, Fluoride, 1993, 26, 97–104 Search PubMed.
- J. A. Varner, K. F. Jensen, W. Horvath and R. L. Isaacson, Brain Res., 1998, 784, 284–298 CrossRef CAS PubMed.
- A. Neisi, M. Mirzabeygi, G. Zeyduni, A. Hamzezadeh, D. Jalili, A. Abbasnia, M. Yousefi and R. Khodadadi, Data Brief, 2018, 18, 713–718 CrossRef.
- D. O. Perez, M. R. Martinez, F. Martinez, V. H. B. Aburto, J. Castelo, J. I. Grimaldo, E. D. L. Cruz, L. Carrizales and F. D. Barriga, Environ. Res., 2003, 93, 20–30 CrossRef.
- H. Wang, Z. Yang, B. Zhou, H. Gao, X. Yan and J. Wang, Toxicol. Ind. Health, 2009, 25, 49–57 CrossRef.
- C. X. Jiang, Q. T. Fan, X. M. Cheng and L. X. Cui, Int. J. Hyg. Environ. Health, 2005, 34, 32–34 CAS.
- V. Pezzi, M. L. Panno, R. Sirianni, P. Forastieri, I. Casaburi, M. Lanzino, V. Rago, F. Giordano, C. Giordano, A. Carpino and S. Ando, J. Endocrinol., 2001, 170, 381–393 CAS.
- J. Gutknecht and A. Walter, Biochim. Biophys. Acta, Gen. Subj., 1981, 644, 153–156 CrossRef CAS.
- A. K. Susheela and P. Jethanandani, J. Toxicol., Clin. Toxicol., 1996, 34, 183–189 CrossRef CAS PubMed.
- A. Gofa and R. M. Davidson, J. Membr. Biol., 1996, 149, 211–219 CrossRef CAS PubMed.
- P. C. Pati and S. P. Bhunya, Caryologia, 2014, 40, 79–87 CrossRef.
- V. Dhurvey, V. Patil and M. Thakare, Fluoride, 2017, 50, 235–246 CAS.
- A. Kumar and S. Kumari, Soc. Appl. Sci., 2011, 2, 283–287 Search PubMed.
- R. W. Dharmaratne, Environ. Health Prev. Med., 2015, 20, 237–242 CrossRef CAS PubMed.
- R. Yu, T. Xia, A. Wang and X. Chen, Chin. J. Prev. Med., 2002, 36, 219–221 CAS.
- A. A. Oyagbemi, T. O. Omobowale, E. R. Asenuga, A. O. Adejumobi, T. O. Ajibade, T. M. Ige, B. S. Ogunpolu, A. A. Adedapo and M. A. Yakubu, Environ. Toxicol., 2017, 32, 1089–1101 CrossRef CAS.
- V. Soyseth and J. Kongerud, Br. J. Ind. Med., 1992, 49, 125–130 CAS.
- S. L. Choubisa and D. Choubisa, Environ. Monit. Assess., 2015, 187, 1–6 CrossRef CAS.
- W. Macnee and I. Rahman, Trends Mol. Med., 2001, 7, 55–62 CrossRef CAS.
- T. Shanmugam, M. Selvaraj and S. Poomalai, Int. Immunopharmacol., 2016, 39, 128–139 CrossRef CAS PubMed.
- J. X. Wang and Y. M. Bian, Environ. Pollut., 1988, 52, 11–18 CrossRef CAS.
- B. D. L. Fuente, M. Vazquez, R. A. Rocha, V. Devesa and D. Velez, Toxicol. In Vitro, 2016, 34, 81–87 CrossRef PubMed.
- N. S. Rao, J. Geol. Soc. India, 1992, 40, 462–467 Search PubMed.
- R. Ullah, M. S. Zafar and N. Shahani, Iran. J. Basic Med. Sci., 2017, 20, 841–848 Search PubMed.
- R. D. Reddy, K. Lahiri, R. M. Rao, K. N. Vedanayakam, L. N. Ebenezer and R. M. Suguna, Fluoride, 1985, 18, 135–140 Search PubMed.
- L. S. Kaminsky, M. C. Mahoney, J. Leach, J. Melius and M. J. Miller, Oral Biol. Med., 1990, 1, 261–281 CAS.
- A. Malhotra, A. Tewari, H. S. Chawla, K. Gauba and K. Dhall, J. Indian Soc. Pedod. Prev. Dent., 1993, 11, 1–3 CAS.
- T. F. Collins, R. L. Sprando, M. E. Shackelford, T. N. Black, M. J. Ames, J. J. Welsh, M. F. Balmer, N. Olejnik and D. I. Ruggles, Food Chem. Toxicol., 1995, 33, 951–960 CrossRef CAS.
- P. Whiting, M. MacDonagh and J. Kleijnen, BMC Public Health, 2001, 1, 1–8 CrossRef PubMed.
- K. Takahashi, Fluoride, 1998, 31, 61–73 Search PubMed.
- A. J. Malin, C. Lesseur, S. A. Busgang, P. Curtin, R. O. Wright and A. P. Sanders, Environ. Int., 2019, 132, 105012 CrossRef CAS PubMed.
- M. Juuti and O. P. Heinonen, Scand. J. Urol. Nephrol., 1980, 14, 181–187 CrossRef CAS PubMed.
- P. P. Singh, M. K. Barjatiya, S. Dhing, R. Bhatnagar, S. Kothari and V. Dhar, Urol. Res., 2001, 29, 238–244 CrossRef CAS PubMed.
- P. L. Jooste, M. J. Weight, J. A. Kriek and A. J. Louw, Eur. J. Clin. Nutr., 1999, 53, 8–12 CrossRef CAS PubMed.
- K. S. Sidhu and R. O. Kimmer, J. Environ. Health, 2002, 65, 16–21 CAS.
- S. Dasarathy, T. K. Das, I. P. Gupta, A. K. Susheela and R. K. Tandon, J. Gastroenterol., 1996, 31, 333–337 CrossRef CAS PubMed.
- G. N. Jenkins, Proc. Finn. Dent. Soc., 1991, 87, 571–579 CAS.
- D. Haguenauer, V. Welch, B. Shea, P. Tugwell, J. D. Adachi and G. Wells, Osteoporosis Int., 2002, 11, 727–738 CrossRef.
- P. T. C. Harrison, J. Fluorine Chem., 2005, 126, 1448–1456 CrossRef CAS.
- P. Grandjean, M. Horder and Y. Thomassen, J. Occup. Med., 1990, 32, 58–63 CrossRef CAS.
- N. Parimi, V. Viswanath, B. Kashyap and P. U. Patil, Int. J. Trichology, 2013, 5, 148–150 CrossRef PubMed.
- O. S. Idowu, R. M. Duckworth, R. A. Valentine and F. V. Zohoori, Caries Res., 2021, 55, 292–300 CrossRef CAS PubMed.
- M. Vidyadharan, J. S. Issac, A. M. Joseph, A. Joseph, D. John and V. K. Varadharaju, J. Int. Soc. Prev. Community Dent., 2020, 10, 269–278 CrossRef PubMed.
- S. S. Sankhala, R. Harshwal, P. Paliwal and A. Agarwal, Fluoride, 2014, 47, 235–240 CAS.
- C. H. Carlson, W. D. Armstrong and L. Singer, Exp. Biol. Med., 1960, 104, 235–239 CrossRef CAS PubMed.
- D. T. Zero, R. F. Raubertas, A. M. Pedersen, J. Fu, A. L. Hayes and J. D. Featherstone, J. Dent. Res., 1992, 71, 1546–1552 CrossRef CAS PubMed.
- P. J. Mullenix, P. K. Denbesten, A. Schunior and W. J. Kernan, Neurotoxicol. Teratol., 1995, 17, 169–177 CrossRef CAS PubMed.
- Y. H. Hu and S. S. Wu, J. Neurol., Neurosurg. Psychiatry, 1988, 51, 1591–1593 CrossRef CAS PubMed.
- J. Ekstrand, M. Ehrnebo, G. M. Whitford and P. O. Jarnberg, Eur. J. Clin. Pharmacol., 1980, 18, 189–194 CrossRef CAS PubMed.
- P. O. Jarnberg, J. Ekstrand and L. Irestedt, Anesthesiology, 1981, 54, 48–52 CrossRef CAS.
- H. Spencer, L. Kramer, D. Osis and E. Wiatrowski, J. Appl. Physiol., 1975, 38, 282–287 CrossRef CAS PubMed.
-
L. H. Weinstein and A. Davison, Fluorides in the Environment: Effects on Plants and Animals, CABI, Cambridge, UK, 2000 Search PubMed.
- Z. Toth, Z. Gintner and J. Banoczy, Fluoride, 2005, 38, 199–204 CAS.
-
R. Ranjan and A. Ranjan, Fluoride Toxicity in Animals, Springer, Switzerland, 2015 Search PubMed.
- G. E. Stoddard, G. Q. Bateman, L. E. Harris, J. L. Shupe and D. A. Greenwood, J. Dairy Sci., 1963, 46, 720–726 CrossRef CAS.
- H. Faraji, A. A. Mohammadi, B. A. Adergani, N. V. Saatloo, G. Lashkarboloki and A. H. Mahvi, Iran. J. Public Health, 2014, 43, 1664–1668 Search PubMed.
- H. Poureslami, P. Khazaeli, A. H. Mahvi, K. Poureslami, P. Poureslami, J. Haghani and M. Aghael, Fluoride, 2016, 49, 485–494 CAS.
- A. M. Khalil, Mutat. Res., 1995, 343, 67–74 CAS.
- Y. Zhang, X. Sun, G. Sun and S. L. L. Wang, Fluoride, 2006, 39, 191–194 CAS.
- S. K. Jain and A. K. Susheela, Environ. Res., 1987, 44, 117–125 CrossRef CAS.
- S. J. Challacombe, Community Dent. Health, 1996, 13, 69–71 Search PubMed.
- M. Bhatnagar, P. Rao, A. Saxena, R. Bhatnagar, P. Meena, S. Barbar, A. Chouhan and S. Vimal, Fluoride, 2006, 39, 280–284 CAS.
- M. L. Vani and K. P. Reddy, Fluoride, 2000, 33, 17–26 CAS.
- S. A. K. S. Mohamed, S. Upreti, S. V. Rajendra and D. Raman, Int. J. Pharm. Pharm. Sci., 2017, 1, 555–575 Search PubMed.
- S. Guth, S. Huser, A. Roth and J. G. Hengstler, Arch. Toxicol., 2020, 94, 1375–1415 CrossRef CAS PubMed.
- H. R. Kim, Y. J. Park, D. Y. Shin, S. M. Oh and K. H. Chung, Environ. Health Toxicol., 2013, 28, e2013003 CrossRef PubMed.
- T. K. Das, A. K. Susheela, I. P. Gupta, S. Dasarathy and R. K. Tandon, J. Clin. Gastroenterol., 1994, 18, 194–199 CrossRef CAS PubMed.
- K. Das and N. K. Mondal, Environ. Monit. Assess., 2016, 188, 218 CrossRef PubMed.
- M. Yousefi, M. Yaseri, R. Nabizadeh, E. Hooshmand, M. Jalilzadeh, A. H. Mahvi and A. A. Mohammadi, Biol. Trace Elem. Res., 2018, 185, 282–288 CrossRef CAS PubMed.
- Meenakshi and R. C. Maheshwari, J. Hazard. Mater., 2006, 137, 456–463 CrossRef CAS PubMed.
- S. S. Waghmare and T. Arfin, Int. J. Innov. Sci. Eng. Technol., 2015, 2, 560–571 Search PubMed.
- J. A. MacDonald and M. C. Kavanaugh, Environ. Sci. Technol., 1994, 28, 362–368 CrossRef.
- G. Jacks, P. Bhattacharya, V. Chaudhary and K. P. Singh, Appl. Geochem., 2005, 20, 221–228 CrossRef CAS.
- Y. Kim, M. Han, J. Kabubi, H. G. Sohn and D. C. Nguyen, Water Sci. Technol.: Water Supply, 2016, 16, 1110–1121 Search PubMed.
- S. S. Waghmare and T. Arfin, Int. J. Sci. Eng. Technol., 2015, 4, 3663–3677 Search PubMed.
- S. Ayoob, A. K. Gupta and V. T. Bhat, Crit. Rev. Environ. Sci. Technol., 2008, 38, 401–470 CrossRef CAS.
- O. Z. Ojekunle, O. V. Ojekunle, A. A. Adeyemi, A. G. Taiwo, O. R. Sangowusi, A. M. Taiwo and A. A. Adekitan, Springerplus, 2016, 5, 560 CrossRef.
- M. Chikuma, Y. Okabayashi, T. Nakagawa, A. Inoue and H. Tanaka, Chem. Pharm. Bull., 1987, 35, 3734–3739 CrossRef CAS.
- M. Chikuma and M. Nishimura, React. Polym., 1990, 13, 131–138 CrossRef CAS.
- L. N. Ho, T. Ishihara, S. Ueshima, H. Nishiguchi and Y. Takita, J. Colloid Interface Sci., 2004, 272, 399–403 CrossRef CAS.
- S. Meenakshi and N. Viswanathan, J. Colloid Interface Sci., 2007, 308, 438–450 CrossRef CAS PubMed.
- S. V. Jadhav, E. Bringas, G. D. Yadav, V. K. Rathod, I. Ortiz and K. V. Marathe, J. Environ. Manage., 2015, 162, 306–325 CrossRef CAS.
- W. G. Nawlakhe and R. Paramasivam, Curr. Sci., 1993, 65, 743–748 CAS.
- A. Bhatnagar, E. Kumar and M. Sillanpaa, Chem. Eng. J., 2011, 171, 811–840 CrossRef CAS.
- L. Aljerf and A. E. Choukaife, J. Int. Med. Res., 2017, 24, 407–410 CAS.
- M. Y. A. Mollah, R. Schennach, J. R. Parga and D. L. Cocke, J. Hazard. Mater., 2001, 84, 29–41 CrossRef CAS PubMed.
- D. Ghosh, C. R. Medhi and M. K. Purkait, Chemosphere, 2008, 73, 1393–1400 CrossRef CAS PubMed.
- S. Vasudevan, B. S. Kannan, J. Lakshmi, S. Mohanraj and G. Sozhan, J. Chem. Technol. Biotechnol., 2011, 86, 428–436 CrossRef CAS.
- S. Dubey, M. Agarwal and A. B. Gupta, Membr. Water Treat., 2021, 12, 83–93 Search PubMed.
- P. Sehn, Desalination, 2008, 223, 73–84 CrossRef CAS.
- B. Assefa, J. Eur. Econ. Assoc., 2006, 23, 1–6 Search PubMed.
- L. H. V. Jimenez, E. V. Alvarez, J. L. F. Arciniega, H. F. Zuniga and J. R. R. Mendez, Sep. Purif. Technol., 2015, 150, 292–307 CrossRef.
- M. Tahaikt, R. E. Habbani, A. A. Haddou, I. Achary, Z. Amor, M. Taky, A. Alami, A. Boughriba, M. Hafsi and A. Elmidaoui, Desalination, 2007, 212, 46–53 CrossRef CAS.
- K. Hu and J. M. Dickson, J. Membr. Sci., 2006, 279, 529–538 CrossRef CAS.
- C. K. Diawara, S. N. Diop, M. A. Diallo, M. Farcy and A. Deratani, J. Water Resour. Prot., 2011, 3, 912–917 CrossRef CAS.
- J. Hoinkis, S. V. Freitag, M. P. Caporgno and C. Patzold, Desalin. Water Treat., 2011, 30, 278–288 CrossRef CAS.
- J. Shen and A. Schafer, Chemosphere, 2014, 117, 679–691 CrossRef CAS.
- P. K. Obulapuram, T. Arfin, F. Mohammad, K. Kumari, S. K. Khiste, H. A. Al-Lohedan and M. Chavali, ACS Omega, 2021, 6, 33614–33626 CrossRef CAS PubMed.
- P. K. Obulapuram, T. Arfin, F. Mohammad, S. K. Khiste, M. Chavali, A. N. Albalawi and H. A. Al-Lohedan, Polymers, 2021, 13, 3490 CrossRef CAS PubMed.
-
T. Arfin, in Environmental
Applications of Carbon Nanomaterials-based Devices, ed. S. Mallakpour, and C. M. Hussain, Wiley-VCH Verlag GmBH & Co. KGaA, Weinhein, 2021, ch. 13, pp. 313–329 Search PubMed.
- S. S. Waghmare and T. Arfin, Int. Res. J. Eng. Technol., 2015, 2, 1179–1197 Search PubMed.
- S. S. Waghmare and T. Arfin, Int. J. Adv. Res. Innov. Ideas Educ., 2015, 1, 628–653 Search PubMed.
- S. Ghorai and K. K. Pant, Sep. Purif. Technol., 2005, 42, 265–271 CrossRef CAS.
- S. Ghorai and K. K. Pant, Chem. Eng. J., 2004, 98, 165–173 CrossRef CAS.
- N. Yadav, K. Rani, S. S. Yadav, D. K. Yadav, V. K. Yadav and N. Yadav, Int. J. Curr. Microbiol. Appl. Sci., 2018, 7, 1147–1162 CrossRef.
- M. Shams, R. N. Nodehi, M. H. Dehghani, M. Younesian and A. H. Mahvi, Fluoride, 2010, 43, 61–66 CAS.
- S. S. Waghmare and T. Arfin, J. Biol. Chem. Chron., 2015, 1, 01–11 Search PubMed.
- M. Mohapatra, S. Anand, B. K. Mishra, D. E. Giles and P. Singh, J. Environ. Manage., 2009, 91, 67–77 CrossRef CAS PubMed.
- A. Goswami and M. K. Purkait, Chem. Eng. Res. Des., 2012, 90, 2316–2324 CrossRef CAS.
- H. Farrah, J. Slavek and W. F. Pickering, Aust. J. Soil Res., 1987, 25, 55–69 CrossRef CAS.
- S. S. Tripathy, J. L. Bersillon and K. Gopal, Sep. Purif. Technol., 2006, 50, 310–317 CrossRef CAS.
- S. M. Maliyekkal, S. Shukla, L. Philip and I. M. Nambi, Chem. Eng. J., 2008, 140, 183–192 CrossRef CAS.
- H. Basu, R. K. Singhal, M. V. Pimple and A. V. R. Reddy, Water, Air, Soil Pollut., 2013, 224, 1–12 CrossRef CAS.
- N. Sakhare, S. Lunge, S. Rayalu, S. Bakardjiva, J. Subrt, S. Devotta and N. Labhsetwar, Chem. Eng. J., 2012, 203, 406–414 CrossRef CAS.
- S. Jagtap, M. K. N. Yenkie, N. Labhsetwar and S. Rayalu, Microporous Mesoporous Mater., 2011, 142, 454–463 CrossRef CAS.
- A. Bansiwal, P. Pillewan, R. B. Biniwale and S. S. Rayalu, Microporous Mesoporous Mater., 2010, 129, 54–61 CrossRef CAS.
- D. Dayananda, V. R. Sarva, S. V. Prasad, J. Arunachalam and N. N. Ghosh, Chem. Eng. J., 2014, 248, 430–439 CrossRef CAS.
- M. Nazari and R. Halladj, J. Taiwan Inst. Chem. Eng., 2014, 45, 2518–2525 CrossRef CAS.
- G. Tomar, A. Thareja and S. Sarkar, Int. J. Environ. Sci. Technol., 2015, 12, 2809–2818 CrossRef CAS.
- Q. Shi, Y. Huang and C. Jing, J. Mater. Chem. A, 2013, 1, 12797–12803 RSC.
- J. Cheng, X. Meng, C. Jing and J. Hao, J. Hazard. Mater., 2014, 278, 343–349 CrossRef CAS PubMed.
- S. S. Tripathy and A. M. Raichur, J. Hazard. Mater., 2008, 153, 1043–1051 CAS.
- S. Paiva, Y. B. O. Lima-Arsati and J. A. Cury, Community Dent. Oral Epidemiol., 2003, 31, 184–191 CrossRef PubMed.
- S. M. Maliyekkal, A. K. Sharma and L. Philip, Water Res., 2006, 40, 3497–3506 CrossRef CAS.
- S. X. Teng, S. G. Wang, W. X. Gong, X. W. Liu and B. Y. Gao, J. Hazard. Mater., 2009, 168, 1004–1011 CrossRef CAS PubMed.
- N. K. Mondal, R. Bhaumik and J. K. Datta, Alexandria Eng. J., 2015, 54, 1273–1284 CrossRef.
- S. S. Waghmare and T. Arfin, Int J. Innov. Res. Sci. Eng. Technol., 2015, 4, 8090–8102 CrossRef.
- N. Gandhi, D. Sirisha and K. B. Chandra Shekar, World J. Pharm. Pharm. Sci., 2013, 2, 3897–3914 Search PubMed.
- M. Islam and R. K. Patel, J. Hazard. Mater., 2007, 143, 303–310 CrossRef CAS PubMed.
- S. Gogoi and R. K. Dutta, J. Environ. Chem. Eng., 2016, 4, 1040–1049 CrossRef CAS.
- A. Jayarathne, R. Weerasooriya and R. Chandrajith, Environ. Earth Sci., 2015, 73, 8369–8377 CrossRef CAS.
- L. Li, Z. L. Zhu, Y. L. Qiu, H. Zhang and J. F. Zhao, HuanJing KeXue, 2010, 31, 1554–1559 CAS.
- M. J. Reyes and M. S. Rios, J. Hazard. Mater., 2010, 180, 297–302 CrossRef PubMed.
- W. Liang, L. Zhan, L. Piao and C. Russel, Mater. Res. Bull., 2011, 46, 205–209 CrossRef CAS.
- Y. N. C. Hu and C. Kong, J. Hazard. Mater., 2012, 233–234, 194–199 Search PubMed.
- N. A. Medellin-Castillo, R. Leyva-Ramos, R. Ocampo-Perez, R. F. G. Cruz, A. Aragon-Pina, J. M. Martinez-Rosales, R. M. Guerrero-Coronado and L. Fuentes-Rubio, Ind. Eng. Chem. Res., 2007, 46, 9205–9212 CrossRef CAS.
- A. W. Wagutu, R. Machundr and Y. A. C. Jande, J. Hazard. Mater., 2018, 347, 95–105 CrossRef CAS PubMed.
- F. Fufa, E. Alemayehu and B. Deboch, J. Environ. Occup., 2014, 3, 71–76 CrossRef.
- M. Gourouza, I. Natatou and A. Boos, J. Mater. Environ. Sci., 2014, 5, 416–425 CAS.
- M. Mourabet, A. E. Rhilassi, H. E. Boujaddy, M. B. Z. Ziatni, R. E. Hamri and A. Taitai, J. Saudi Chem. Soc., 2015, 19, 603–615 CrossRef.
- P. Melidis, Environ. Processes, 2015, 2, 205–213 CrossRef.
- P. Mondal, D. Mehta and S. George, Desalin. Water Treat., 2016, 57, 27294–27313 CrossRef CAS.
- L. Chen, K. Zhang, J. He, X. G. Cai, W. Xu and J. H. Liu, RSC Adv., 2016, 6, 36296–36306 RSC.
- S. S. Waghmare and T. Arfin, Int. J. Eng. Sci. Res. Technol., 2015, 4, 519–536 CAS.
- E. Kumar, A. Bhatnagar, M. Ji, W. Jung, S. H. Lee, S. J. Kim, G. Lee, H. Song, J. Y. Choi, J. S. Yang and B. H. Jeon, Water Res., 2009, 43, 490–498 CrossRef CAS PubMed.
- D. Tang and G. Zhang, Chem. Eng. J., 2016, 283, 721–729 CrossRef CAS.
- Z. Hussain, L. Daosheng and K. Jianxiong, RSC Adv., 2015, 5, 43906 RSC.
- B. Shimelis, F. Zewge and B. S. Chandravanshi, Bull. Chem. Soc. Ethiop., 2006, 20, 17–34 CAS.
- X. Wu, Y. Zhang, X. Dou and M. Yang, Chemosphere, 2007, 69, 1758–1764 CrossRef CAS PubMed.
- R. T. Iwar, O. T. Iorhemen, K. Ogedengbe and K. K. Katibi, Environ. Chem. Ecotoxicol., 2021, 3, 142–154 CrossRef CAS.
- K. Biswas, K. Gupta and U. C. Ghosh, Chem. Eng. J., 2009, 149, 196–206 CrossRef CAS.
- K. Biswas, K. Gupta, A. Goswami and U. C. Ghosh, Desalination, 2010, 255, 44–51 CrossRef CAS.
- T. Zhang, Q. Li, H. Xiao, H. Lu and Y. Zhou, Ind. Eng. Chem. Res., 2012, 51, 11490–11498 CrossRef CAS.
- K. Babaeivelni and A. P. Khodadoust, J. Colloid Interface Sci., 2013, 394, 419–427 CrossRef CAS PubMed.
- A. L. Srivastav, P. K. Singh, V. Srivastava and Y. C. Sharma, J. Hazard. Mater., 2013, 263, 342–352 CrossRef CAS.
- S. Mandal, S. Tripathy, T. Padhi, M. K. Sahu and R. K. Patel, J. Environ. Sci., 2013, 25, 993–1000 CrossRef CAS.
- G. V. Patankar, A. S. Tambe, B. D. Kulkarni, A. Malyshew and S. P. Kamble, Water, Air, Soil Pollut., 2013, 224, 1727 CrossRef.
- P. Koliraj and S. Kannan, Chem. Eng. J., 2013, 234, 406–415 CrossRef.
- S. Alemu, E. Mulugeta, F. Zewge and B. S. Chandravanshi, Environ. Technol., 2014, 35, 1893–1903 CrossRef CAS PubMed.
- N. Sadik, M. Mountadar and E. Sabbar, J. Mater. Environ. Sci., 2014, 6, 2239–2246 Search PubMed.
- H. Lu, Q. Li, H. Xiao, R. Wang and D. Xie, Am. J. Anal. Chem., 2014, 5, 547–558 CrossRef.
- W. Xiang, G. Zhang, Y. Zhang, D. Tang and J. Wang, Chem. Eng. J., 2014, 250, 423–430 CrossRef CAS.
- T. Rafique, K. M. Chadhar, T. H. Usmani, S. Q. Memon, K. Shirin and S. Kamaluddin, Desalin. Water Treat., 2014, 56, 1669–1680 CrossRef.
- N. Ammavasi and R. Mariappan, J. Environ. Chem. Eng., 2018, 6, 5645–5654 CrossRef CAS.
- I. Saha, A. Ghosh, D. Nandi, K. Gupta, D. Chatterjee and U. C. Ghosh, Chem. Eng. J., 2015, 263, 220–230 CrossRef CAS.
- Y. Yu, L. Yu and J. P. Chen, Chem. Eng. J., 2015, 262, 839–846 CrossRef CAS.
- C. Zhang, Y. Li, T. J. Wang, Y. Jiang and H. Wang, Appl. Surf. Sci., 2016, 363, 507–515 CrossRef CAS.
- L. Bo, Q. Li, Y. Wang, L. Gao, X. Hu and J. Yang, Environ. Prog. Sustainable Energy, 2016, 35, 1420–1429 CrossRef CAS.
- M. B. Baskan and A. R. Biyikli, Water Environ. Res., 2021, 93, 620–635 CrossRef.
- Y. Fan, D. Fu, S. Zhou, Y. Lu, X. Zhao, W. Jin and Y. Zhao, Desalin. Water Treat., 2016, 57, 28393–28404 CrossRef CAS.
- M. Karthikeyan and K. P. Elango, Indian J. Chem. Technol., 2008, 15, 525–532 CAS.
- S. Joshi, M. Adhikari and R. R. Pradhananga, J. Nepal Chem. Soc., 2012, 30, 13–23 CrossRef.
- M. Said and R. L. Machunda, Int. J. Sci. Res., 2014, 3, 2327–2331 Search PubMed.
- A. A. M. Daifullah, S. M. Yakout and S. A. Elreefy, J. Hazard. Mater., 2007, 147, 633–643 CrossRef CAS PubMed.
- V. K. Gupta, I. Ali and V. K. Saini, Water Res., 2007, 41, 3307–3316 CrossRef CAS PubMed.
- R. S. Sathish, S. Sairam, V. G. Raja, G. N. Rao and C. Janardhana, Sep. Sci. Technol., 2008, 43, 3676–3694 CrossRef CAS.
- R. S. Sathish, S. Sairam, V. G. Raja, G. N. Rao and C. Janardhana, Sep. Sci. Technol., 2007, 42, 769–788 CrossRef CAS.
- G. Alagumuthu and M. Rajan, Chem. J. Eng., 2010, 158, 451–457 CrossRef CAS.
- G. Alagumuthu, V. Veeraputhiran and R. Venkataraman, Arch. Appl. Sci. Res., 2010, 2, 170–185 CAS.
- N. Chen, Z. Zhang, C. Feng, M. Li, D. Zhu and N. Sugiura, Mater. Chem. Phys., 2011, 125, 293–298 CrossRef CAS.
- M. Dutta, T. Ray and J. K. Basu, Arch. Appl. Sci. Res., 2012, 4, 536–550 CAS.
- H. Han, Z. Sun, G. Luo, C. Wang, R. Wei and J. Wang, Chemosphere, 2015, 135, 297–303 CrossRef CAS PubMed.
- Y. Li, S. Wang, X. Zhao, J. Wei, C. Xu, Z. Luan, D. Wu and B. Wei, Environ. Technol., 2008, 24, 391–398 CrossRef PubMed.
- Y. H. Li, S. Wang, X. Zhang, J. Wei, C. Xu and Z. Luan, Mater. Res. Bull., 2003, 38, 469–476 CrossRef CAS.
- G. Alagumuthu and M. Rajan, Chem. Ind., 2010, 64, 294–304 Search PubMed.
- A. K. Yadav, R. Abbassi, A. Gupta and M. Dadashzadeh, Ecol. Eng., 2013, 52, 211–218 CrossRef.
- L. H. V. Velazquez, R. H. Hurt, J. Mantos and J. R. R. Mendez, Environ. Sci. Technol., 2014, 48, 1166–1174 CrossRef.
- A. R. Tembhurkar and S. Dongre, Indian J. Environ. Health, 2006, 48, 151–156 CAS.
- E. V. Alvarez, L. H. V. Jimenez, L. F. C. Ruiz, P. E. D. Flores and J. R. R. Mendez, J. Colloid Interface Sci., 2015, 455, 194–202 CrossRef.
- M. Regassa, F. Melak, W. Birke and E. Alemayehu, Int. Adv. Res. J. Sci. Eng. Technol., 2016, 3, 1–7 CrossRef.
- R. S. Dongre, Science, 2018, 7, 1–9 Search PubMed.
- K. Singh, D. H. Lataye and K. L. Wasewar, J. Fluorine Chem., 2017, 194, 23–32 CrossRef CAS.
- S. Ravulapalli and R. Kunta, J. Fluorine Chem., 2017, 193, 58–66 CrossRef CAS.
- A. Vinati, B. Mahanty and S. K. Behera, Appl. Clay Sci., 2015, 114, 340–348 CrossRef CAS.
- K. H. Peter, J. Eng. Appl. Sci., 2009, 4, 240–246 CAS.
- A. D. Atasoy and M. O. Sahin, Clean: Soil, Air, Water, 2014, 42, 415–420 CAS.
- A. Tor, Desalination, 2006, 201, 267–276 CrossRef CAS.
- M. Sarkar, A. Banerjee and P. P. Pramanick, Ind. Eng. Chem. Res., 2006, 45, 5920–5927 CrossRef CAS.
- S. M. Sajidu, W. R. L. Masamba, B. Thole and J. F. Mwatseteza, Int. J. Phys. Sci., 2008, 3, 001–011 Search PubMed.
- G. Karthikeyan, A. Pius and G. Alagumuthu, Indian J. Chem. Technol., 2005, 12, 263–272 CAS.
- N. Hamdi and E. S. Tunisia, Fluoride, 2009, 42, 39–45 CAS.
- A. Ramdani, S. Taleb, A. Benghalem and N. Ghaffour, Desalination, 2010, 250, 408–413 CrossRef CAS.
- N. Chen, Z. Zhang, C. Feng, M. Li, D. Zhu, R. Chen and N. Sugiura, J. Hazard. Mater., 2010, 183, 460–465 CrossRef CAS.
- D. Thakre, S. Rayalu, R. Kawade, S. Meshram, J. Subrt and N. Labhsetwar, J. Hazard. Mater., 2010, 180, 122–130 CrossRef CAS PubMed.
- E. W. Wambu and G. K. Muthakia, Fluoride, 2011, 44, 37–41 CAS.
- M. Malakootian, M. Moosazadeh, N. Yousefi and A. Fatehizadeh, Afr. J. Environ. Sci. Technol., 2011, 5, 299–306 CAS.
- A. Maiti, J. K. Basu and S. De, Desalination, 2011, 265, 28–36 CrossRef CAS.
- A. Goswami and M. K. Purkait, Sep. Sci. Technol., 2011, 46, 1797–1807 CrossRef CAS.
- N. Chen, Z. Zhang, C. Feng, M. Li, R. Chen and N. Sugiura, Desalination, 2011, 268, 76–82 CrossRef CAS.
- Q. Guo and E. J. Reardon, Appl. Clay Sci., 2012, 56, 7–15 CrossRef CAS.
- E. W. Wambu, C. O. Onindo, W. Ambusso and G. K. Muthakia, Clean: Soil, Air, Water, 2013, 41, 340–348 CAS.
- M. N. Sepehr, V. Sivasankar, M. Zarrabi and M. S. Kumar, Chem. Eng. J., 2013, 228, 192–204 CrossRef CAS.
- C. Kayira, S. Sajidu, W. Masamba and J. Mwatseteza, Clean: Soil, Air, Water, 2014, 42, 546–551 CAS.
- M. Vhahangwele, G. W. Mugera and N. Tholiso, Toxicol. Environ. Chem., 2014, 9, 1294–1309 CrossRef.
- S. Waghmare, T. Arfin, S. Rayalu, D. Lataye, S. Dubey and S. Tiwari, Int. J. Sci. Eng. Technol. Res., 2015, 4, 4114–4124 Search PubMed.
- A. Y. Dang-I, A. O. Boansi and M. Pedevoah, Int. J. Appl. Sci. Technol., 2015, 5, 45–55 Search PubMed.
- T. O. Ologundudu, J. O. Odiyo and G. E. Ekosse, Appl. Sci., 2016, 6, 277 CrossRef.
- B. Kebede, A. Beyene, F. Fufa, M. Megersa and M. Behm, Appl. Water Sci., 2014, 6, 57–65 CrossRef.
- C. M. V. Vardhan and M. Srimurali, SpringerPlus, 2016, 5, 1426 CrossRef.
- A. Salifu, B. Petrusevski, E. S. Mwampashi, I. A. Pazi, K. Ghebremichael, R. Buamah, C. Aubry, G. L. Amy and M. D. Kenedy, J. Environ. Manage., 2016, 181, 108–117 CrossRef CAS PubMed.
- S. Patnaik, P. C. Mishra, R. N. Nayak and A. K. Giri, J. Anal. Bioanal. Tech., 2016, 7, 1000326 Search PubMed.
- M. Mourabet, E. Rhilassi, H. E. Boujaady, M. B. Ziatni and A. Taitai, Arabian J. Chem., 2014, 10, S3292–S3302 CrossRef.
- H. Zhou, W. Chen, Z. Y. Gao and D. Chen, Asian J. Chem., 2014, 26, 8062–8068 CrossRef.
- W. M. Gitari, A. A. Izuagie and J. R. Gumbo, Arabian J. Chem., 2017, 13, 1–16 Search PubMed.
- W. M. Gitari, T. Ngulube, V. Masindi and J. R. Gumbo, Desalin. Water Treat., 2015, 53, 1578–1590 CrossRef CAS.
- K. Singh, D. H. Lataye and K. L. Wasewar, J. Hazard., Toxic Radioact. Waste, 2015, 20, 04015024 CrossRef.
- P. K. Pandey, M. Pandey and R. Sharma, J. Environ. Prot., 2012, 3, 610–616 CrossRef CAS.
- H. Paudyal, B. Pangeni, K. Inoue, H. Kawakita, K. Ohto, H. Harada and S. Alam, J. Hazard. Mater., 2011, 192, 676–682 CrossRef CAS PubMed.
- B. M. Mamilwar, A. G. Bhole and A. M. Sudame, Int. J. Eng. Res. Appl., 2012, 2, 334–338 Search PubMed.
- S. Chakrabarty and H. P. Sarma, Int. J. Chem. Tech. Res., 2012, 4, 511–516 CAS.
- V. H. Montoya, L. A. R. Montoya, A. B. Petriciolet and M. A. M. Moran, Biochem. Eng. J., 2012, 62, 1–7 CrossRef.
- H. Cai, G. Chen, C. Peng, Z. Zhang, Y. Dong, G. Shang, X. Zhu, H. Gao and X. Wan, Appl. Surf. Sci., 2015, 328, 34–44 CrossRef CAS.
- A. A. S. Nur, S. I. M. Halim, M. D. Kamal and S. Izhar, World Appl. Sci. J., 2009, 28, 1518–1530 Search PubMed.
- S. Rajkumar, S. Murugesh, V. Sivasankar, A. Darchen, T. A. M. Msagati and T. Chaabane, Arabian J. Chem., 2019, 12, 3004–3017 CrossRef CAS.
- C. E. Choong, K. T. Wong, S. B. Jang, I. W. Nah, J. Choi, S. Ibrahim, Y. Yoon and M. Jang, Chemosphere, 2020, 39, 124765 CrossRef PubMed.
- H. A. S. Sanchez, R. C. Martinez and R. A. C. Villanueva, Int. J. Sci. Basic Appl. Res., 2013, 11, 159–172 Search PubMed.
- S. Dwivedi, P. Mondal and C. Balomajumder, Res. J. Chem. Sci., 2014, 4, 50–58 Search PubMed.
- T. A. M. Msagati, B. B. Mamba, V. Sivasankar and K. Omine, Appl. Surf. Sci., 2014, 301, 235–243 CrossRef CAS.
- S. Dwivedi, P. Mondal and C. Balomajumder, Res. J. Chem. Sci., 2014, 4, 52–60 CAS.
- Y. Zhang and K. Huang, RSC Adv., 2019, 9, 7767–7776 RSC.
- M. A. T. Ajisha and K. Rajagopal, Int. J. Environ. Sci. Technol., 2015, 12, 223–236 CrossRef.
- Y. Yu, C. Wang, X. Guo and J. P. Chen, J. Colloid Interface Sci., 2015, 441, 113–120 CrossRef CAS PubMed.
- V. Patel and S. Gupta, Int. J. Eng. Res. Technol., 2020, 7, 4363–4368 Search PubMed.
- R. Jha, U. Jha, R. K. Dey, S. Mishra and S. K. Swain, Desalin. Water Treat., 2015, 53, 2144–2157 CrossRef CAS.
- K. A. Emmanuel, A. Veerabhadrarao, T. V. Nagalakshmi, M. G. Reddy, P. P. Diwakar and C. Sureshbabu, Der Pharma Chem., 2015, 7, 225–236 CAS.
- S. Manna, D. Roy, P. Saha and B. Adhikari, J. Taiwan Inst. Chem. Eng., 2015, 50, 215–222 CrossRef CAS.
- S. Kanaujia, B. Singh and S. K. Singh, J. Geosci. Environ. Prot., 2015, 3, 1 Search PubMed.
- S. Dobaradaran, M. A. Zazuli, M. Keshtkar, S. Noshadi, M. Khorsand, F. F. Ghasemi, V. N. Karbasdehi, L. Amiri and F. Soleimani, Desalin. Water Treat., 2016, 57, 28405–28416 CrossRef CAS.
- S. Bibi, A. Farooqi, K. Hussain and N. Haider, J. Cleaner Prod., 2015, 87, 882–896 CrossRef CAS.
- S. Kagne, S. Jagtap, P. Dhawade, S. P. Kamble, S. Devotta and S. S. Rayalu, J. Hazard. Mater., 2008, 154, 88–95 CrossRef CAS.
- E. Togarepi, C. Mahamadi and A. Mangombe, Afr. J. Environ. Sci. Technol., 2012, 6, 176–181 CrossRef CAS.
- W. Nigussie, F. Zewge and B. S. Chandravanshi, J. Hazard. Mater., 2007, 147, 954–963 CrossRef CAS PubMed.
- D. S. Shyamal and P. K. Ghosh, Groundw. Sustain. Dev., 2019, 9, 100248 CrossRef.
- R. J. Krupadam, M. S. Khan and S. Das, Water Sci. Technol., 2010, 62, 759–765 CrossRef CAS PubMed.
- M. Islam and R. Patel, Chem. Eng. J., 2011, 169, 68–77 CrossRef CAS.
- S. T. Ramesh, R. Gandhimathi, P. V. Nidheesh and M. Taywade, Environ. Res. Eng. Manag., 2012, 2, 12–20 Search PubMed.
- E. Oguz, J. Hazard. Mater., 2005, 117, 227–233 CrossRef CAS.
- G. Lv, L. Wu, L. Liao, Y. Zhang and Z. Li, Appl. Clay Sci., 2013, 74, 95–101 CrossRef CAS.
- E. Oguz, Colloids Surf., A, 2007, 295, 258–263 CrossRef CAS.
- C. K. Geethamani, S. T. Ramesh, R. Gandhimathi and P. V. Nidheesh, Desalin. Water Treat., 2014, 52, 3466–3476 CrossRef CAS.
- A. E. Yilmaz, B. A. Fil, S. Bayar and Z. K. Karakas, Global NEST J., 2015, 17, 18–197 Search PubMed.
- S. Ayoob and A. K. Gupta, Chem. Eng. J., 2007, 133, 273–281 CrossRef CAS.
- G. Biswas, M. Dutta, S. Dutta and K. Adhikari, Environ. Sci. Pollut. Res., 2016, 23, 9418–9431 CrossRef CAS PubMed.
- G. Patel, U. Pal and S. Menon, Sep. Sci. Technol., 2009, 44, 2806–2826 CrossRef CAS.
- R. R. Devi, I. M. Umlong, P. K. Raul, B. Das, S. Banerjee and L. Singh, J. Exp. Nanosci., 2012, 9, 512–524 CrossRef.
- E. Kumar, A. Bhatnagar, U. Kumar and M. Sillanpaa, J. Hazard. Mater., 2011, 186, 1042–1049 CrossRef CAS PubMed.
- S. Wang, Y. Ma, Y. Shi and W. Gong, J. Chem. Technol. Biotechnol., 2009, 84, 1043–1050 CrossRef CAS.
- N. Dash, S. Dasb, T. Patnaik, S. B. Patel and R. K. Dey, Adv. Appl. Sci. Res., 2013, 4, 387–399 CAS.
- S. Gao, J. Cui and Z. Wei, J. Fluorine Chem., 2009, 130, 1035–1041 CrossRef CAS.
- M. Mohapatra, K. Rout, S. K. Gupta, P. Singh, S. Anand and B. K. Mishra, J. Nanopart. Res., 2010, 12, 681–686 CrossRef CAS.
- T. Zhang, L. Qiurong, M. Zhenyu, X. Haiyan, L. Hongxiao and Z. Yuming, Desalin. Water Treat., 2014, 16–18, 3367–3376 CrossRef.
- M. Ansari, M. Kazemipour, M. Dehghani and M. Kazemipour, J. Fluorine Chem., 2011, 132, 516–520 CrossRef CAS.
- S. S. Ramamurthy, Y. Chen, M. K. Kalyan, G. N. Rao, J. Chelli and S. Mitra, J. Nanosci. Nanotechnol., 2011, 11, 3552–3559 CrossRef CAS PubMed.
- G. A. Haghighat, M. H. Dehghani, S. Nasseri, A. H. Mahvi and N. Rastkari, Indian J. Sci. Technol., 2012, 5, 2432–2435 CrossRef CAS.
- H. Chen, M. Yan, X. Yang, Z. Chen, G. Wang, D. S. Vogt, Y. Xu and J. Xu, J. Hazard. Mater., 2012, 235, 201–209 CrossRef PubMed.
- S. Deng, H. Liu, W. Zhou, J. Huang and G. Yu, J. Hazard. Mater., 2011, 186, 1360–1366 CrossRef CAS PubMed.
- P. K. Raul, R. R. Devi, I. M. Umlong, S. Banerjee, L. Singh and M. Purkait, J. Nanosci. Nanotechnol., 2012, 12, 3922–3930 CrossRef CAS PubMed.
- N. Minju, K. V. Swaroop, K. Haribabu, V. Sivasubramanian and P. S. Kumar, Desalin. Water Treat., 2013, 53, 2905–2914 CrossRef.
- F. Adeno, E. Mulugeta, F. Zewge and Y. Chebude, Bull. Chem. Soc. Ethiop., 2014, 28, 215–227 CrossRef.
- H. S. Jahin, Int. Water Technol., 2014, 4, 173–182 Search PubMed.
- A. Dhillon and D. Kumar, J. Mater. Chem., 2015, 3, 4215–4228 RSC.
- D. Dayananda, V. R. Sarva, S. V. Prasad, J. Arunachalam, P. Parameswaran and N. N. Ghosh, Appl. Surf. Sci., 2015, 329, 1–10 CrossRef CAS.
- E. Bazrafshan, D. Balarak, A. H. Panahi, H. Kamani and A. H. Mahvi, Flouride, 2016, 49, 233–244 CAS.
- I. B. Singh, A. Gupta, S. Dubey, M. Shafeeq, P. Banerjee and A. S. K. Sinha, J. Sol-Gel Sci. Technol., 2016, 77, 416–422 CrossRef CAS.
- S. M. Prabhu, S. D. Elanchezhiyan, G. Lee, A. Khan and S. Meenakshi, Chem. Eng. J., 2016, 300, 334–342 CrossRef CAS.
- P. Chinnakoti, A. L. A. Chunduri, R. K. Vankayala, S. Patnaik and V. Kamisetti, Appl. Water Sci., 2017, 7, 2413–2423 CrossRef CAS.
- M. H. Dehghani, G. A. Haghighat, K. Yetilmezsoy, G. McKay, B. Heibati, I. Tyagi, S. Agarwal and V. K. Gupta, J. Mol. Liq., 2016, 216, 401–410 CrossRef CAS.
- Z. Ruan, Y. Tian, J. Ruan, G. Cui, K. Iqbal, A. Iqbal, H. Ye, Z. Yang and S. Yan, Appl. Surf. Sci., 2017, 412, 578–590 CrossRef CAS.
- D. Balarak, Y. Mahdavi, E. Bazrafshan, A. H. Mahvi and Y. Esfandyari, Fluoride, 2016, 49, 71–83 CAS.
- P. Chinnakoti, R. K. Vankayala, A. L. A. Chunduri, L. R. Nagappagari, S. V. Muthukonda and V. Kamisetti, J. Environ. Chem. Eng., 2016, 4, 4754–4768 CrossRef CAS.
- A. Singh and R. Gothalwal, Water Environ. J., 2018, 32, 481–487 CrossRef CAS.
- S. Mukherjee and G. Halder, J. Environ. Chem. Eng., 2018, 6, 1257–1270 CrossRef CAS.
- M. A. Barakat, Arabian J. Chem., 2011, 4, 361–377 CrossRef CAS.
- G. Kalyani, G. B. Rao, B. V. Saradhi and Y. P. Kumar, Int. J. Appl. Environ. Sci., 2009, 4, 177–186 Search PubMed.
- S. V. Mohan, S. V. Ramanaiah, B. Rajkumar and P. N. Sarma, J. Hazard. Mater., 2007, 141, 465–474 CrossRef CAS.
- S. V. Mohan, S. V. Ramanaiah, B. Rajkumar and P. N. Sarma, Bioresour. Technol., 2007, 98, 1006–1011 CrossRef CAS PubMed.
- S. Mukherjee, M. Mondal, S. Banerjee and G. Halder, Process Saf. Environ. Prot., 2017, 107, 334–345 CrossRef CAS.
- S. Mukherjee, P. Sahu and G. Halder, Environ. Prog. Sustainable Energy, 2018, 37, 1573–1586 CrossRef CAS.
- S. Chouhan, U. Tuteja and S. J. S. Flora, Appl. Biochem. Biotechnol., 2012, 48, 43–50 CAS.
- M. Motaghi and P. Ziarati, Biomed. Pharmacol. J., 2016, 9, 739–749 CrossRef.
- S. Mukherjee, V. Yadav, M. Mondal, S. Banerjee and G. Halder, Appl. Water Sci., 2017, 7, 1923–1930 CrossRef CAS.
- S. Koshle, S. Mahesh and S. N. Swamy, J. Environ. Biol., 2016, 37, 135–140 CAS.
- P. G. Hiremath and T. Theodore, Adv. Chem., 2016, 2016, 6848693 Search PubMed.
- J. Singh, P. Singh and A. Singh, Arabian J. Chem., 2016, 9, 815–824 CrossRef CAS.
- K. Chaudhary and S. Khan, J. Plant Biol. Soil Health, 2016, 3, 8 Search PubMed.
- R. Agarwal and S. S. Chauhan, Int. J. Multidiscip. Res. Dev., 2015, 2, 16–21 Search PubMed.
- A. Boukhris, I. L. Schwob, I. Mezghan, L. E. Kadri, P. Prudent, A. Pricop, T. Tatoni and M. Chaieb, Chemosphere, 2015, 119, 217–223 CrossRef CAS PubMed.
- S. Karmakar, J. Mukherjee and S. Mukherje, Int. J. Phytorem., 2016, 18, 222–227 CrossRef CAS PubMed.
- A. J. Kapenja, L. C. Msigala and H. T. Mwakabona, Afr. J. Environ. Sci. Technol., 2017, 11, 207–212 CrossRef CAS.
- S. Dwivedi, P. Mondal and C. Balomajumder, J. Hazard., Toxic Radioact. Waste, 2017, 21, 04016023 CrossRef.
-
World Health Organization, Environmental Health Criteria, 2002, p. 227 Search PubMed.
-
G. Tjandraatmadja, C. Pollard, C. Sheedy and Y. Gozukara, Water for a Healthy Country Flagship Report, CSIRO, Canberra, 2010, pp. 1–118 Search PubMed.
- V. Ochoa-Herrera, Q. Banihani, G. Leon, C. Khatri, J. A. Fields and R. Sierra-Alvarez, Water Res., 2009, 43, 3177–3186 CrossRef CAS PubMed.
- D. Nakic, D. Vouk, S. Donatello and A. A. Vucinic, Eng. Rev., 2017, 37, 222–234 Search PubMed.
- Y. Li, H. Zhang, Z. Zhang, L. Shao and P. He, J. Environ. Sci., 2015, 31, 21–29 CrossRef CAS.
- W.-T. Lin, Constr. Build. Mater., 2019, 196, 564–573 CrossRef CAS.
- EFSA, EFSA J., 2013, 11, 3332 CrossRef.
- N. Millar, E. McLaughlin and T. Borger, Ecol. Econ., 2019, 158, 11–19 CrossRef.
- A. K. Duraiappah, World Dev., 1998, 26, 2169–2179 CrossRef.
- A. Flynn and N. Hacking, J. Cleaner Prod., 2019, 212, 1256–1267 CrossRef.
- M. Guney, B. Oral, H. Demirin, N. Karahan, T. Mungan and N. Delibas, Clin. Exp. Pharmacol. Physiol., 2007, 34, 467–474 CrossRef CAS PubMed.
- N. Kumar, N. Bansal and S. K. Sharma, Int. J. Pharm., Chem. Biol. Sci., 2014, 4, 576–592 Search PubMed.
- R. S. Villena, Pediatr. Dent. J., 2000, 22, 312–317 CAS.
- G. K. Stookey, M. S. Mau, R. L. Isaacs, C. G. Gierbolini, R. D. Bartizek and A. R. Biesbrock, Caries Res., 2004, 38, 542–550 CrossRef CAS.
-
B. T. Cummins, Controlling Fluoride Levels: A Literature Review, World Health Organization, Geneva, Switzerland, 1985 Search PubMed.
- S. Rawlani, S. Rawlani and S. Rawlani, Indian J. Community Med., 2010, 35, 298–301 CrossRef PubMed.
-
NEERI, Defluoridation. Technology Mission on Drinking Water in Villages and Related Water Management, National Environment Engineering Research Institute, Nagpur, 1987 Search PubMed.
- M. R. Khairnar, A. S. Dodamani, H. C. Jadhav, R. G. Naik and M. A. Deshmukh, J. Clin. Diagn. Res., 2015, 9, ZE05–ZE09 Search PubMed.
|
This journal is © The Royal Society of Chemistry 2022 |