DOI:
10.1039/D1PY00672J
(Communication)
Polym. Chem., 2021,
12, 4317-4325
Bromoform-assisted aqueous free radical polymerisation: a simple, inexpensive route for the preparation of block copolymers†
Received
18th May 2021
, Accepted 12th July 2021
First published on 13th July 2021
Abstract
In the quest for commercially relevant block copolymer additives, for which overall average molecular composition is key but molar mass distribution is of little importance, we present a straightforward, sulfur- and metal-free aqueous route to block copolymers using commercially available starting materials. Based on synthetic techniques first described in the 1950s for hydrophobic monomers in organic solvents, we have shown that bromoform (CHBr3) can be used to create block copolymers. Unlike common bromine-containing chain transfer agents such as carbon tetrabromide (CBr4), bromoform is partially water-miscible and relatively inexpensive. Herein, we demonstrate this new aqueous-based technology using N,N-dimethylacrylamide (DMA) and N-isopropylacrylamide (NIPAM) as exemplar monomers to synthesise PDMA-b-PNIPAM block copolymers of varying composition directly in water. This study demonstrates the potential for such a simple, inexpensive route to functional block copolymers where further research to decipher the detailed mechanism and true potential of this technique will be of great value.
The last three decades have marked unprecedented advances in polymer chemistry, with the advent of reversible-deactivation radical polymerisation (RDRP) techniques such as nitroxide-mediated polymerisation1–3 (NMP), atom transfer radical polymerisation2,4–8 (ATRP) and reversible addition–fragmentation chain transfer1,2,9–14 (RAFT) polymerisation, enabling the production of a wide range of well-defined block copolymers. Such well-defined macromolecules are crucial for structure–property relationship studies,15 bulk block copolymer self-assembly (where low mass dispersities are required to produce sharp nanophase boundaries16–19) and in the pursuit of sequence-controlled macromolecules for biomimicry.20–22 Despite the drive for narrow molar mass distributions, this is not always necessary to prepare materials with desired characteristics and performance.23–30 In industrial settings, polymers with broader molar mass distributions are often easier to process31 and the breadth and shape of the molar mass distribution32 can impart desirable physical properties (e.g. processability).33,34 Indeed, Parker et al.35 reported the synthesis of block copolymers via RAFT dispersion polymerisation with high molar mass dispersity values (Đ > 3) that self-assembled into extremely well-defined spherical nanoparticles which show promise as friction reduction agents in automotive engine oil.36
One of the drawbacks of conventional RDRP techniques is that they often rely on toxic transition metals,4 sulfur37–41 or unstable compounds42 to provide control and often produce inherently coloured polymers (e.g. in the case of RAFT). Indeed, recent work by Haddleton et al.43 elegantly highlights one of the challenges in polymer chemistry; the need to produce block copolymers without the use of sulfur or transition metals. Their group utilised ω-unsaturated oligomers as chain transfer agents, similar to studies of Rizzardo and co-workers44,45 who previously used this approach to prepare poly(butyl methacrylate)-b-poly(phenyl methacrylate) block copolymers with narrow molar mass distributions.46
Alkyl halides are useful compounds often used in RDRP techniques (such as ATRP, iodine transfer polymerisation (ITP),47–52 reverse iodine transfer polymerisation (RITP)53–58 and bromine-iodine transformation reversible-deactivation radical polymerisation (BIT-RDRP)59–62) due to the reversible homolytic scission of the carbon–halogen bond. ATRP uses alkyl halides as initiators whereas ITP employs typically unstable alkyl iodides as a chain transfer agents (CTAs). The alkyl iodides required in ITP processes are difficult to store due to their low bond dissociation enthalpies, which is why focus has shifted to explore the in situ generation of alky iodide compounds from the more stable alkyl bromides in both RITP and BIT-RDRP processes. Despite limited reports in water,63,64 RITP and BIT-RDRP reactions commonly employ organic solvents, high temperatures (50–110 °C (ref. 53, 55–57 and 60)) and metal-containing reagents (e.g. sodium iodide in BIT-RDRP). Herein we explore the use of a water-miscible alkyl halide reagent, bromoform, to overcome these issues and generate colourless (white) block copolymers via a simple, inexpensive route.
The use of bromine-based chain transfer agents in polymerisation formulations has been discussed in a small number of literature reports, dating back to the 1950s.65–67 Dunn et al.67 described the use of bromotrichloromethane (CBrCl3) and carbon tetrabromide (CBr4) in the preparation of polystyrene-block-poly(methyl methacrylate) (PS-b-PMMA) copolymers under UV and thermal conditions. However, only 10% monomer conversion was achieved in these seminal studies. Miller then explored graft65 and block66 copolymers using acrylamide, acrylonitrile and acrylic acid monomers, where the chain transfer capabilities of monobromoethane (C2H5Br), dibromomethane (CH2Br2) and bromoform (CHBr3) were investigated using photo-polymerisation. However, the UV lamp used in these studies may have heated the reaction solution up to 50 °C (over a 3 hours period, as discovered in our laboratory – data not shown), suggesting that thermal effects could also be influencing the polymerisation and thus the role of the bromine radical remains unclear. Both Dunn et al. and Millers’ studies were conducted in organic solvents and were limited by the capabilities of analytical techniques of that time. Three decades later in a patent, Wu et al.68 described the use of this bromoform-assisted copolymerisation technique in the formation of block copolymers containing 2-acrylamido-2-methylpropane sulfonic acid (AMPS) and acrylamide for use in oil recovery. However, the role of bromoform remains unclear. Today, there is a broader range of conventional analytical techniques that can be exploited to develop block copolymer synthesis strategies using these potentially straightforward methods.
Bromoform has been selected for use in this study as it is water-miscible (3.0 g L−1 at 20 °C,69,70 3.0 g L−1 at 25 °C (ref. 70 and 71) and 3.2 g L−1 at 30 °C (ref. 69 and 70)), readily available, inexpensive, stable (easily stored) and can be used directly at low and ambient temperatures, in contrast to other mediating agents (e.g. alkyl iodides or CTAs). Interestingly, bromoform has been reported to photodissociate under UV light (at wavelengths of 193,72 234,73 248,74 266,75 267
73 and between 266–324 nm (ref. 76)); primarily into Br2HC˙ and Br˙ radicals. Herein, we explore this property of bromoform and its potential to initiate or mediate the polymerisation under UV conditions. Preliminary work by Thananukul et al.77 reported the use of bromoform in the synthesis of polyacrylamide (PAM) homopolymer under UV conditions in water. Specifically, bromoform was suggested as a potential CTA due to the influence on the PAM molar mass. Therefore, the possibility exists to use this method in the synthesis of block copolymers owing to the potential of a photolytically reactive bromine-terminated chain end.
Whilst literature reports concerning bromoform and its chain transfer ability are limited, the foundation of this work appears promising. The work herein significantly advances this area, introducing a straightforward method to create commercially-relevant block copolymers, using free radical polymerisation without sulfur- or metal-containing compounds directly in water for the first time (Scheme 1).
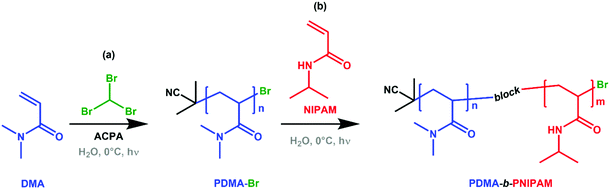 |
| Scheme 1 (a) Step 1: bromoform-assisted polymerisation of N,N-dimethylacrylamide (DMA). The proposed structure of the poly(N,N-dimethylacrylamide) macro-initiator (PDMA-Br) is based on the 4,4′-azobis(4-cyanopentanoic acid) (ACPA) radical fragment initiating the growth of a polymer chain and the bromoform-derived bromine radical (Br˙) capping the chain-end. (b) Step 2: polymerisation of N-isopropylacrylamide (NIPAM) using the PDMA-Br macro-initiator synthesised in Step 1 to produce poly(N,N-dimethylacrylamide)-block-poly(N-isopropylacrylamide) [PDMA-b-PNIPAM]. | |
Firstly, the free radical photopolymerisation of N,N-dimethylacrylamide (DMA) at varying bromoform concentration (0.0, 0.5, 1.0 and 2.0 mol% with respect to DMA) and fixed 4,4′-azobis(4-cyanopentanoic acid) (ACPA) concentration (1.0 mol% with respect to DMA) was investigated (Scheme 1(a), see ESI† for experimental procedure) to determine the optimum route for forming bromine-terminated PDMA (referred to as PDMA-Br). The effect of bromoform on these homopolymerisations was studied by monitoring monomer conversion and molar mass evolution using 1H nuclear magnetic resonance (NMR) spectroscopy (Fig. S1–S4†) and gel permeation chromatography (GPC) (Table 1 and Fig. S5†), respectively. High monomer conversions (≥93%) were achieved in each case and the final GPC traces indicated good repeatability of syntheses conducted at all bromoform concentrations (performed in triplicate, see Fig. S6†). It should be noted that the molar mass dispersity, Đ, of the final polymers was high in all cases (2.8–3.5), with no suggested relationship between Đ and bromoform content. As previously discussed, high Đ values may not inherently have an adverse impact on the desired application.15,23–30
Table 1 Summary of final monomer conversion, Mn and Đ data for the polymerisation of N,N-dimethylacrylamide at varied bromoform concentrations. All experiments were performed using 1.0 mol% ACPA (relative to monomer) in 25 mL deionized water at 0 °C for 1 hour of UV irradiation
Bromoforma (mol %) |
DMA monomer conversionb (%) |
PDMA Mnc (kg mol−1) |
PDMA Đc (Mw/Mn) |
Relative to DMA monomer.
Calculated using 1H NMR spectroscopy and eqn (S1).†
Determined by DMF GPC using poly(methyl methacrylate) standards.
|
0 |
97 |
246.7 |
3.4 |
0.5 |
95 |
294.8 |
2.8 |
1.0 |
93 |
271.2 |
2.8 |
2.0 |
96 |
242.3 |
3.5 |
Kinetic studies shown in Fig. 1(b) suggest that the evolution of molar mass with monomer conversion is in line with conventional free radical polymerisation,78–80 rather than RDRP,81–85 where the relationship would be linear. The final molar mass appears to increase on addition of 0.5 mol% bromoform, however, closer inspection of the broad GPC curves indicates that the molar mass profiles for the PDMA synthesised are near-identical in all cases (see Fig. S6†). It should be noted that the molar mass profiles exceed the upper limit of the GPC calibration range, which will affect the Mn values obtained from seemingly identical broad curves. Fig. 1(a) and (c) demonstrate little to no difference in polymerisation rate observed for all bromoform concentrations studied herein.
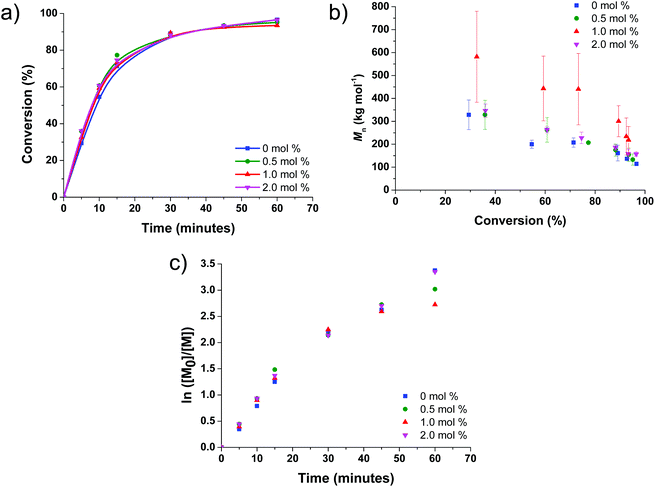 |
| Fig. 1 Kinetic data for the polymerisation of N,N-dimethylacrylamide at varied bromoform content: (a) monomer conversion with time; (b) Mnversus monomer conversion; and (c) semi-logarithmic first-order plot. | |
In parallel to our studies carried out on DMA, N-isopropylacrylamide (NIPAM) homopolymerisation at varying bromoform content was also explored (kinetic data are provided in the ESI: see Fig. S7–S13 and Table S1†).
Next, a large quantity (20 g) of PDMA was synthesised, in a single batch using 2 mol% bromoform, in an attempt to produce bromine-terminated PDMA (PDMA-Br) as a potential macro-initiator for the second step [Scheme 1(b)]. 2 mol% bromoform (relative to monomer) was selected to maximise the proportion of bromine-terminated PDMA chains within the usable bromoform miscibility range. PDMA-b-PNIPAM copolymers with varying block molar ratios were subsequently targeted in a polymerisation formulation containing PDMA-Br (see Fig. S14†), NIPAM monomer and water. Importantly, the PDMA-Br was purified by precipitation to remove any unreacted monomer, initiator and bromoform impurities, and no ACPA initiator was used in the second step (unlike in a RAFT polymerisation formulation). This purification step ensures that any subsequent polymerisation can only be initiated by the proposed PDMA-Br macro-initiator (and not residual ACPA or bromoform used in Step 1), as the NIPAM does not self-polymerise under these conditions (vide infra and ESI, Fig. S15†).
PDMA-b-PNIPAM copolymers with a target range of DMA
:
NIPAM molar ratios were synthesised as summarised in Table 2. DMF GPC analysis was performed to determine the Mn values for the PDMA-Br macro-initiator and subsequent PDMA-b-PNIPAM block copolymers. The mean degree of polymerisation (DP) of the PNIPAM block was determined using NIPAM monomer conversions as judged by 1H NMR spectroscopy (see eqn (S3)†). The length of the PNIPAM block increased when targeting higher DPs, as expected, and the monomer conversion was greater when longer PNIPAM blocks were targeted, which is attributed to increased NIPAM concentrations during the polymerisation.
Table 2 Summary of Mn, Đ and the target and achieved PNIPAM DP in PDMA-b-PNIPAM copolymers
Target polymera |
NIPAM conversionb (%) |
M
n
(kg mol−1) |
PNIPAM DPd |
Đ
(Mw/Mn) |
Target PNIPAM DP calculated using eqn (S2)† and GPC.
Calculated using 1H NMR spectroscopy and eqn (S1).†
Determined by DMF GPC using poly(methyl methacrylate) standards.
Calculated using 1H NMR spectroscopy and eqn (S3).† Values are rounded to the nearest ten.
PDMA macro-initiator synthesized using 2 mol% bromoform (relative to monomer), achieving a final DMA monomer conversion of 91%.
M
n appears smaller than expected due to broad GPC trace, suggesting a significant amount of unreacted PDMA is still present.
|
PDMA1500e |
— |
148.4 |
— |
3.9 |
PDMA1500-b-PNIPAM170 |
66 |
163.6 |
110 |
3.4 |
PDMA1500-b-PNIPAM380 |
70 |
165.8 |
270 |
3.4 |
PDMA1500-b-PNIPAM640 |
65 |
196.3 |
420 |
3.2 |
PDMA1500-b-PNIPAM1000 |
75 |
164.4f |
750 |
5.3f |
PDMA1500-b-PNIPAM1500 |
87 |
264.8 |
1310 |
4.1 |
PDMA1500-b-PNIPAM2300 |
89 |
392.6f |
2050 |
4.1f |
PDMA1500-b-PNIPAM3500 |
95 |
463.6 |
3330 |
3.9 |
PDMA1500-b-PNIPAM6000 |
85 |
603.7 |
5100 |
3.4 |
Although the GPC traces of the first two block copolymers (PNIPAM DP ≤ 270) in Fig. 2 suggest that little or no PDMA-Br chain extension was achieved, the Mn values (Table 2) suggest that block extension has been successful and the 1H NMR spectra confirm the presence of PNIPAM in all cases (Fig. S16†). The GPC traces for the purified PDMA-b-PNIPAM copolymers (PNIPAM DP > 270) suggest successful chain-extension of the PDMA-Br macro-initiator with NIPAM, but this alone is not conclusive evidence.86 Additionally, as the NIPAM content increases (determined by 1H NMR spectroscopy) the Mn (measured by GPC) increases, as expected for chain-extension.
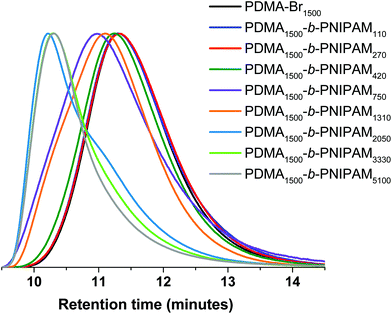 |
| Fig. 2 GPC traces of PDMA-Br macro-initiator and PDMA-b-PNIPAM copolymers with target PNIPAM DPs ranging from 170 to 6000. | |
Due to the inherent termination events of free-radical polymerisation and the observed uncontrolled nature of this bromoform-assisted reaction (Đ = 3.9 for the macro-initiator), we anticipate that there will be a portion of PDMA that is incapable of chain extension. Indeed, the GPC traces in Fig. 2 suggest that there could be some residual PDMA homopolymer in the block copolymer samples, particularly for PNIPAM DP = 2050, where a significant shoulder is observed. As aforementioned, this is attributed to competing termination events in Step 1 resulting in PDMA chains without the necessary bromine functionality. For example, the competing dissociation pathways of bromoform (between bromine and hydrogen transfer72–76), combination, disproportionation or unwanted chain transfer (e.g. to polymer, monomer or solvent) would also result in ‘dead’ PDMA rather than Br-terminated chains. It is important to note that the presence of homopolymer impurity is not of concern for industrially relevant additives, where blends are often employed to achieve desired properties.
To further confirm that PDMA-b-PNIPAM block copolymers were successfully synthesised (and not simply a mixture of PDMA and PNIPAM homopolymers that could arise from trace amounts of free-radical initiator that remained following purification in Step 1), important control experiments were conducted. Firstly, NIPAM homopolymerisations were attempted in the absence of ACPA photoinitiator (i.e. only NIPAM, bromoform and water present). Importantly, no polymerisation occurred after irradiation with UV light (Fig. S15†), which indicates that bromoform itself does not act as a photoinitiator under these conditions. This is contrary to previous reports by Miller66 and Wu et al.,68 who described the use of bromoform as a photoinitiator during the polymerisation of acrylamide, acrylonitrile, acrylic acid and 2-acrylamido-2-methylpropane sulfonic acid (AMPS). However, as previously discussed, these studies lack thermal control and the temperatures of up to 50 °C (reached during the UV irradiation) could be causing bromoform to behave as a thermal initiator. Secondly, polymerisations without APCA and bromoform (i.e. only NIPAM and water present) were attempted in order to determine whether NIPAM would self-polymerise. Using the previously described conditions and even extended UV exposure times, homopolymerisation of NIPAM did not take place (Fig. S15†). Thirdly, a control reaction using PDMA synthesised without using bromoform in Step 1 was conducted. When targeting PDMA1500-b-PNIPAM3500 under these conditions, no NIPAM polymerisation was observed (neither homopolymerisation nor PDMA chain-extension), indicating that bromoform is needed in Step 1 for the formation of the desired diblock copolymer (Fig. 3 and Fig. S17†). These control studies (summarised in Scheme 2) provide evidence that bromoform imparts bromine functionality onto PDMA chains in Step 1 to enable block copolymer synthesis in Step 2.
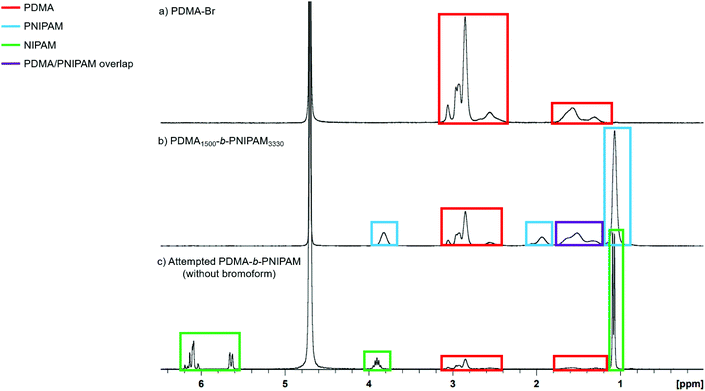 |
| Fig. 3
1H NMR spectrum showing (a) PDMA-Br after precipitation, (b) PDMA1500-b-PNIPAM3330 (using 2 mol% bromoform in step 1) after precipitation and (c) only NIPAM monomer peaks present for the attempted synthesis of PDMA-b-PNIPAM from PDMA (0 mol% bromoform in step 1). | |
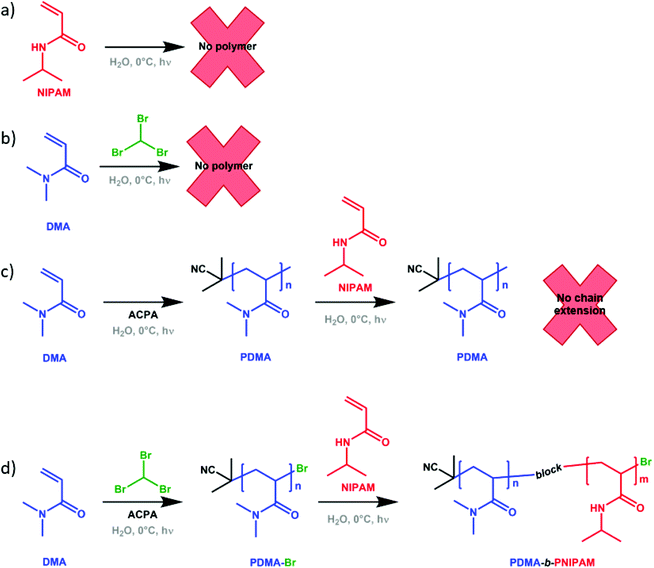 |
| Scheme 2 (a) Attempted synthesis of PNIPAM homopolymer in the absence of bromoform and ACPA photoinitiator. (b) Attempted synthesis of PDMA homopolymer in the absence of ACPA photoinitiator. (c) Attempted synthesis of PDMA-b-PNIPAM copolymers without bromoform in Step 1. (d) Successful two step synthesis of PDMA-b-PNIPAM copolymers using PDMA prepared using bromoform in Step 1. | |
Based on the observations from the control experiments we speculate the following mechanism for this bromoform-assisted technique. Step one, used to produce the PDMA macro-initiator, is more akin to free radical polymerisation favouring the formation of the DMA homopolymer (in line with a decrease in Mn with monomer conversion shown in Fig. 1b). In the latter stages of this reaction, at high monomer conversion, the chains are reversibly capped with a bromine functionality from the bromoform. In the second stage, in which no photoinitiator (ACPA) is present, the bromine chain end functionality is cleaved and subsequent polymerisation of the second block (NIPAM) occurs. Overall, resulting in the observed increase in molar mass.
In summary, we describe the synthesis of block copolymers via metal-free, sulfur-free, bromoform-assisted aqueous free-radical polymerisation. Specifically, a PDMA precursor was first synthesised in the presence of bromoform before being chain-extended with NIPAM to produce PDMA-b-PNIPAM copolymers with varying block molar ratios in a straightforward, wholly aqueous two step procedure. This study suggests that using bromoform generates bromine-terminated PDMA chains that are capable of chain-extension with NIPAM. When bromoform is not used in Step 1, no PDMA-b-PNIPAM block copolymers were produced under otherwise identical conditions. The competition between bromine and hydrogen transfer from bromoform to the PDMA precursor block, in addition to the inherent termination and chain transfer events of free-radical polymerisation, results in block copolymers of very high molar mass dispersity. Nevertheless, this straightforward, sulfur- and metal-free route to block copolymers is useful for the preparation of materials with desired characteristics for applications that do not require exquisite control over the molar mass distribution. Further investigations are required to elucidate detailed mechanistic information and identify the scope and limitations for this promising synthetic technique.
Conflicts of interest
There are no conflicts to declare.
Acknowledgements
This project has received funding from the European Union's Horizon 2020 research and innovation programme under the Marie Sklodowska-Curie grant agreement No. 871650 (MEDIPOL). This research was also supported by the Program Management Unit for Human Resources & Institutional Development, Research and Innovation, Office of National Higher Education, Science Research and Innovation Policy Council (NXPO) [Grant Number B16F640001] and the Center of Excellence in Materials Science and Technology, Chiang Mai University.
References
- E. Rizzardo and D. H. Solomon, On the Origins of Nitroxide Mediated Polymerization (NMP) and Reversible Addition–Fragmentation Chain Transfer (RAFT), Aust. J. Chem., 2012, 65, 945 CrossRef CAS.
- W. A. Braunecker, Controlled/Living Radical Polymerization: Features, Developments, and Perspectives, Prog. Polym. Sci., 2007, 32, 93–146 CrossRef CAS.
- J. Nicolas, Y. Guillaneuf, C. Lefay, D. Bertin, D. Gigmes and B. Charleux, Nitroxide-Mediated Polymerization, Prog. Polym. Sci., 2013, 38, 63–235 CrossRef CAS.
- J. S. Wang and K. Matyjaszewski, ‘Living’/Controlled Radical Polymerization. Transition-Metal-Catalyzed Atom Transfer Radical Polymerization in the Presence of a Conventional Radical Initiator, J. Am. Chem. Soc., 1995, 28, 7572–7573 CAS.
- K. Matyjaszewski, Atom Transfer Radical Polymerization (ATRP): Current Status and Future Perspectives, Macromolecules, 2012, 45, 4015–4039 CrossRef CAS.
- J. T. Rademacher, M. Baum, M. E. Pallack, W. J. Brittain and W. J. Simonsick, Atom Transfer Radical Polymerization of N,N-dimethylacrylamide, Macromolecules, 2000, 33, 284–288 CrossRef CAS.
- J. S. Wang and K. Matyjaszewski, Controlled/“Living” Radical Polymerization. Halogen Atom Transfer Radical Polymerization Promoted by a Cu(I)/Cu(II) Redox Process, Macromolecules, 1995, 28, 7901–7910 CrossRef CAS.
- M. Ouchi and M. Sawamoto, 50th Anniversary Perspective: Metal-Catalyzed Living Radical Polymerization: Discovery and Perspective, Macromolecules, 2017, 50, 2603–2614 CrossRef CAS.
- L. Hutson, J. Krstina, C. L. Moad, G. Moad, G. R. Morrow, A. Postma, E. Rizzardo and S. H. Thang, Chain Transfer Activity of ω-Unsaturated Methacrylic Oligomers in Polymerizations of Methacrylic Monomers, Macromolecules, 2004, 37, 4441–4452 CrossRef CAS.
- G. Moad, Y. K. Chong, A. Postma, E. Rizzardo and S. H. Thang, Advances in RAFT Polymerization: The Synthesis of Polymers with Defined End-Groups, Polymer, 2005, 46, 8458–8468 CrossRef CAS.
- G. Moad, J. Chiefari, Y. K. Chong, J. Krstina, R. T. A. Mayadunne, A. Postma, E. Rizzardo and S. H. Thang, Living Free-Radical Polymerization by Reversible Addition−Fragmentation Chain Transfer: The RAFT Process, Macromolecules, 1998, 31, 5559–5562 CrossRef.
- G. Gody, T. Maschmeyer, P. B. Zetterlund and S. Perrier, Pushing the Limit of the RAFT Process: Multiblock Copolymers by One-Pot Rapid Multiple Chain Extensions at Full Monomer Conversion, Macromolecules, 2014, 47, 3451–3460 CrossRef CAS.
- G. Gody, T. Maschmeyer, P. B. Zetterlund and S. Perrier, Exploitation of the Degenerative Transfer Mechanism in RAFT Polymerization for Synthesis of Polymer of High Livingness at Full Monomer Conversion, Macromolecules, 2014, 47, 639–649 CrossRef CAS.
- G. Gody, T. Maschmeyer, P. B. Zetterlund and S. Perrier, Rapid and Quantitative One-pot Synthesis of Sequence-Controlled Polymers by Radical Polymerization, Nat. Commun., 2013, 4, 1–9 Search PubMed.
- M. Destarac, Industrial Development of Reversible-Deactivation Radical Polymerization: Is the Induction Period over?, Polym. Chem., 2018, 9, 4947–4967 RSC.
- N. A. Lynd and M. A. Hillmyer, Influence of Polydispersity on the Self-Assembly of Diblock Copolymers, Macromolecules, 2005, 38, 8803–8810 CrossRef CAS.
- J. M. G. Swann and P. D. Topham, Design and Application of Nanoscale Actuators Using Block-Copolymers, Polymers, 2010, 2, 454–469 CrossRef CAS.
-
D. J. Adams and P. D. Topham, in Supramolecular Chemistry, John Wiley & Sons, Ltd, 2012 Search PubMed.
- H. A. Klok and S. Lecommandoux, Supramolecular Materials via Block Copolymer Self–Assembly, Adv. Mater., 2001, 13, 1217–1229 CrossRef CAS.
- J. De Neve, J. J. Haven, L. Maes and T. Junkers, Sequence-Definition from Controlled Polymerization: The Next Generation of Materials, Polym. Chem., 2018, 9, 4692–4705 RSC.
- J. F. Lutz, M. Ouchi, D. R. Liu and M. Sawamoto, Sequence-Controlled Polymers, Science, 2013, 341, 1238149 CrossRef PubMed.
- J. F. Lutz, Sequence-Controlled Polymerizations: The Next Holy Grail in Polymer Science?, Polym. Chem., 2010, 1, 55–62 RSC.
- K. E. B. Doncom, L. D. Blackman, D. B. Wright, M. I. Gibson and R. K. O'Reilly, Dispersity Effects in Polymer Self-Assemblies: A Matter of Hierarchical Control, Chem. Soc. Rev., 2017, 46, 4119–4134 RSC.
- R. Whitfield, K. Parkatzidis, N. P. Truong, T. Junkers and A. Anastasaki, Tailoring Polymer Dispersity by RAFT Polymerization: A Versatile Approach, Chem, 2020, 6, 1340–1352 CAS.
- D. T. Gentekos and B. P. Fors, Molecular Weight Distribution Shape as a Versatile Approach to Tailoring Block Copolymer Phase Behavior, ACS Macro Lett., 2018, 7, 677–682 CrossRef CAS.
- S. I. Rosenbloom and B. P. Fors, Shifting Boundaries: Controlling Molecular Weight Distribution Shape for Mechanically Enhanced Thermoplastic Elastomers, Macromolecules, 2020, 53, 7479–7486 CrossRef CAS.
- D. T. Gentekos, L. N. Dupuis and B. P. Fors, Beyond Dispersity: Deterministic Control of Polymer Molecular Weight Distribution, J. Am. Chem. Soc., 2016, 138, 1848–1851 CrossRef CAS PubMed.
- S. Han, J. Wu, Y. Zhang, J. Lai, Y. Chen, L. Zhang and J. Tan, Utilization of Poor RAFT Control in Heterogeneous RAFT Polymerization, Macromolecules, 2021, 1–13 CAS.
- B. R. Parker, M. J. Derry, Y. Ning and S. P. Armes, Exploring the Upper Size Limit for Sterically Stabilized Diblock Copolymer Nanoparticles Prepared by Polymerization-Induced Self-Assembly in Non-Polar Media, Langmuir, 2020, 36, 3730–3736 CrossRef CAS PubMed.
- M. J. Derry, T. Smith, P. S. O'Hora and S. P. Armes, Block Copolymer Nanoparticles Prepared via Polymerization-Induced Self-Assembly Provide Excellent Boundary Lubrication Performance for Next-Generation Ultralow-Viscosity Automotive Engine Oils, ACS Appl. Mater. Interfaces, 2019, 11, 33364–33369 CrossRef CAS PubMed.
- S. Harrisson, The Downside of Dispersity: Why the Standard Deviation is a Better Measure of Dispersion in Precision Polymerization, Polym. Chem., 2018, 9, 1366–1370 RSC.
- S. S. Rane and P. Choi, Polydispersity Index: How Accurately does it Measure the Breadth of the Molecular Weight Distribution?, Chem. Mater., 2005, 17, 926 CrossRef CAS.
- M. Stürzel, S. Mihan and R. Mülhaupt, From Multisite Polymerization Catalysis to Sustainable Materials and All-Polyolefin Composites, Chem. Rev., 2016, 116, 1398–1433 CrossRef PubMed.
- S. I. Rosenbloom, R. J. Sifri and B. P. Fors, Achieving Molecular Weight Distribution Shape Control and Broad Dispersities using RAFT Polymerizations, Polym. Chem., 2021, 1–6 Search PubMed.
- B. R. Parker, M. J. Derry, Y. Ning and S. P. Armes, Exploring the Upper Size Limit for Sterically Stabilized Diblock Copolymer Nanoparticles Prepared by Polymerization-Induced Self-Assembly in Non-Polar Media, Langmuir, 2020, 36, 3730–3736 CrossRef CAS PubMed.
- M. J. Derry, T. Smith, P. S. O'Hora and S. P. Armes, Block Copolymer Nanoparticles Prepared via Polymerization-Induced Self-Assembly Provide Excellent Boundary Lubrication Performance for Next-Generation Ultralow-Viscosity Automotive Engine Oils, ACS Appl. Mater. Interfaces, 2019, 11, 33364–33369 CrossRef CAS PubMed.
- D. B. Thomas, A. J. Convertine, R. D. Hester, A. B. Lowe and C. L. McCormick, Hydrolytic Susceptibility of Dithioester Chain Transfer Agents and Implications in Aqueous RAFT Polymerizations, Macromolecules, 2004, 37, 1735–1741 CrossRef CAS.
- Y. K. Chong, J. Krstina, T. P. T. Le, G. Moad, A. Postma, E. Rizzardo and S. H. Thang, Thiocarbonylthio Compounds [SC(Ph)S−R] in Free Radical Polymerization with Reversible Addition-Fragmentation Chain Transfer (RAFT Polymerization). Role of the Free-Radical Leaving Group (R), Macromolecules, 2003, 36, 2256–2272 CrossRef CAS.
- M. Destarac, D. Charmot, X. Franck and S.
Z. Zard, Dithiocarbamates as Universal Reversible Addition-Fragmentation Chain Transfer Agents, Macromol. Rapid Commun., 2000, 21, 1035–1039 CrossRef CAS.
- A. V. Fuchs and K. J. Thurecht, Stability of Trithiocarbonate RAFT Agents Containing Both a Cyano and a Carboxylic Acid Functional Group, ACS Macro Lett., 2017, 6, 287–291 CrossRef CAS.
- V. K. Patel, N. K. Vishwakarma, A. K. Mishra, C. S. Biswas, P. Maiti and B. Ray, Synthesis of Alkyne-Terminated Xanthate RAFT Agents and Their Uses for the Controlled Radical Polymerization of N-vinylpyrrolidone and the Synthesis of its Block Copolymer Using Click Chemistry, J. Appl. Polym. Sci., 2013, 127, 4305–4317 CrossRef CAS.
- R. B. Grubbs, Nitroxide-Mediated Radical Polymerization: Limitations and Versatility, Polym. Rev., 2011, 51, 104–137 CrossRef CAS.
- G. Patias, A. M. Wemyss, S. Efstathiou, J. S. Town, C. J. Atkins, A. Shegiwal, R. Whitfield and D. M. Haddleton, Controlled Synthesis of Methacrylate and Acrylate Diblock Copolymers via End-Capping using CCTP and FRP, Polym. Chem., 2019, 10, 6447–6455 RSC.
- C. L. Moad, G. Moad, E. Rizzardo and S. H. Thang, Chain Transfer Activity of ω-Unsaturated Methyl Methacrylate Oligomers, Macromolecules, 1996, 29, 7717–7726 CrossRef CAS.
- P. Cacioli, D. G. Hawthorne, R. L. Laslett, E. Rizzardo and D. H. Solomon, Copolymerization of o-Unsaturated Oligo(methy methacrylate): New Macromonomers, J. Macromol. Sci., Part A: Pure Appl.Chem., 1986, 23, 839–852 CrossRef.
- J. Krstina, G. Moad, E. Rizzardo, C. L. Winzor, C. T. Berge and M. Fryd, Narrow Polydispersity Block Copolymers by Free-Radical Polymerization in the Presence of Macromonomers, Macromolecules, 1995, 28, 5381–5385 CrossRef CAS.
- K. Matyjaszewski, S. Gaynor and J. S. Wang, Controlled Radical Polymerizations: The Use of Alkyl Iodides in Degenerative Transfer, Macromolecules, 1995, 28, 2093–2095 CrossRef CAS.
- C. Farcet, M. Lansalot, R. Pirri, J. P. Vairon and B. Charleux, Polystyrene-block-poly(butyl acrylate) and Polystyrene-block-poly[(butyl acrylate)-co-styrene] Block Copolymers Prepared via Controlled Free-Radical Miniemulsion Polymerization Using Degenerative Iodine Transfer, Macromol. Rapid Commun., 2000, 21, 921–926 CrossRef CAS.
- K. Koumura, K. Satoh, M. Kamigaito and Y. Okamoto, Iodine Transfer Radical Polymerization of Vinyl Acetate in Fluoroalcohols for Simultaneous Control of Molecular Weight, Stereospecificity and Regiospecificity, Macromolecules, 2006, 39, 4054–4061 CrossRef CAS.
-
M. Tatemoto, in Polymeric Materials Encylcopedia, Elsevier Science, Amsterdam, 1996, 3847–3862 Search PubMed.
- V. Percec, A. V. Popov, E. Ramirez-Castillo, J. F. J. Coelho and L. A. Hinojosa-Falcon, Non-transition Metal-Catalyzed Living Radical Polymerization of Vinyl Chloride Initiated with Iodoform in Water at 25 °C, J. Polym. Sci., Part A: Polym. Chem., 2004, 42, 6267–6282 CrossRef CAS.
-
P. Lacroix-Desmazes, R. Severac and B. Boutevin, in Advances in Controlled/Living Polymerization, American Chemical Society, Michigan, 2003, pp. 570–585 Search PubMed.
- B. N. Patra, D. Rayeroux and P. Lacroix-Desmazes, Synthesis of Cationic Amphiphilic Diblock Copolymers of Poly(vinylbenzyl triethylammonium chloride) and Polystyrene by Reverse Iodine Transfer Polymerization (RITP), React. Funct. Polym., 2010, 70, 408–413 CrossRef CAS.
- J. Tonnar, P. Lacroix-Desmazes and B. Boutevin, Controlled Radical Ab Initio Emulsion Polymerization of n–Butyl Acrylate by Reverse Iodine Transfer Polymerization (RITP): Effect of the Hydrolytic Disproportionation of Iodine, Macromol. Rapid Commun., 2006, 27, 1733–1738 CrossRef CAS.
- J. Hui, Y. Shi, T. Li, J. Wu and Z. Fu, Reverse Iodine Transfer Polymerization (RITP) of Chloroprene, RSC Adv., 2015, 5, 44326–44335 RSC.
- L. Wang, Y. Li, L. Chen, C. Ban, G. Li and J. Ni, Fabrication of Honeycomb-Patterned Porous Films from PS-b-PNIPAM Amphiphilic Diblock Copolymers Synthesized via RITP, J. Colloid Interface Sci., 2014, 420, 112–118 CrossRef CAS PubMed.
- D. Rayeroux, B. N. Patra and P. Lacroix-Desmazes, Synthesis of Anionic Amphiphilic Diblock Copolymers of Poly(styrene)and Poly(acrylic acid) by Reverse Iodine Transfer Polymerization (RITP) in Solution and Emulsion, J. Polym. Sci., Part A: Polym. Chem., 2013, 51, 4389–4398 CrossRef CAS.
- M. F. Cunningham, Controlled/Living Radical Polymerization in Aqueous Dispersed Systems, Prog. Polym. Sci., 2008, 33, 365–398 CrossRef CAS.
- H. Li, Q. Xu, X. Xu, L. Zhang, Z. Cheng and X. Zhu, One-Step Photocontrolled Polymerization-Induced Self-Assembly (Photo-PISA) by Using In Situ Bromine-Iodine Transformation Reversible-Deactivation Radical Polymerization, Polymers, 2020, 12, 1–10 CAS.
- L. Xiao, K. Sakakibara, Y. Tsujii and A. Goto, Organocatalyzed Living Radical Polymerization via In Situ Halogen Exchange of Alkyl Bromides to Alkyl Iodides, Macromolecules, 2017, 50, 1882–1891 CrossRef CAS.
- Q. Xu, C. Tian, L. Zhang, Z. Cheng and X. Zhu, Photo-Controlled Polymerization-Induced Self-Assembly (Photo-PISA): A Novel Strategy Using In Situ Bromine-Iodine Transformation Living Radical Polymerization, Macromol. Rapid Commun., 2019, 40, 1800327 CrossRef PubMed.
- H. Li, H. Zhao, L. Yao, L. Zhang, Z. Cheng and X. Zhu, Photocontrolled Bromine–Iodine Transformation Reversible-Deactivation Radical Polymerization: Facile Synthesis of Star Copolymers and Unimolecular Micelles, Polym. Chem., 2021, 1–11 Search PubMed.
- X. Liu, Q. Xu, L. Zhang, Z. Cheng and X. Zhu, Visible-Light-Induced Living Radical Polymerization Using in situ Bromine-Iodine Transformation as an Internal Boost, Polym. Chem., 2017, 8, 2538–2551 RSC.
-
P. Lacroix-Desmazes, J. Tonnar and B. Boutevin, in Macromolecular Symposia, John Wiley & Sons, Ltd, 2007, vol. 248, pp. 150–157 Search PubMed.
- M. L. Miller, Block and Graft Polymers: I. Graft Polymers from Acrylamide and Acrylonitrile, Can. J. Chem., 1958, 36, 303–308 CrossRef CAS.
- M. L. Miller, Block and Graft Polymers II. Block Polymers from Acrylamide and Acrylonitrile and Acrylamide and Acrylic Acid, Can. J. Chem., 1958, 36, 309–315 CrossRef CAS.
- A. S. Dunn, B. D. Stead and H. W. Melville, The Synthesis of Block Copolymers of Styrene and Methyl Methacrylate, Trans. Faraday Soc., 1954, 50, 279 RSC.
-
M. M. Wu and L. E. Ball, European Patent, 4540498, 1983 Search PubMed.
-
International Agency for Research on Cancer, Chlorinated Drinking-water; Chlorination By-products; Some Other Halogenated Compounds; Cobalt and Cobalt Compounds, World Heal. Organ., 1991, vol. 52, p. 214 Search PubMed.
-
S. H. Yalkowsky, Y. He and P. Jain, Handbook of Aqueous Solubility Data, CRC Press, USA, 2nd edn, 2016 Search PubMed.
-
W. M. Haynes, CRC Handbook of Chemistry and Physics, CRC Press, Boca Raton, 92nd edn, 2011 Search PubMed.
- W. Sean McGivern, O. Sorkhabi, A. G. Suits, A. Derecskei-Kovacs and S. W. North, Primary and Secondary Processes in the Photodissociation of CHBr3, J. Phys. Chem. A, 2000, 104, 10085–10091 CrossRef.
- T. Ying, J. Lei, T. Bi-Feng, Z. Rong-Shu, Z. Song and Z. Bing, Photodissociation of Alkyl Bromides at UV Scope, Acta Phys.-Chim. Sin., 2004, 20, 344–349 Search PubMed.
- P. Zou, J. Shu, T. J. Sears, G. E. Hall and S. W. North, Photodissociation of Bromoform at 248 nm: Single and Multiphoton Processes, J. Phys. Chem. A, 2004, 108, 1482–1488 CrossRef CAS.
- S. X. Yang, G. Y. Hou, J. H. Dai, C. H. Chang and B. C. Chang, Spectroscopic Investigation of the Multiphoton Photolysis Reactions of Bromomethanes (CHBr3, CHBr2Cl, CHBrCl2, andCH2Br2) at Near-Ultraviolet Wavelengths, J. Phys. Chem. A, 2010, 114, 4785–4790 CrossRef CAS PubMed.
- K. D. Bayes, R. R. Friedl, S. P. Sander and D. W. Toohey, Measurements of Quantum Yields of Bromine Atoms in the Photolysis of Bromoform from 266 to 324 nm, J. Geophys. Res.: Atmos., 2003, 3, 1–6 Search PubMed.
- K. Thananukul, J. Porkaew, P. Punyamoonwongsa, R. Molloy and B. J. Tighe, Kinetic Studies of the Photopolymerisation of Acrylamide in Aqueous Solution: Effects of Bromoform as a Chain Transfer Agent, Chiang Mai J. Sci., 2014, 41, 1352–1360 CAS.
-
K. Matyjaszewski, in Controlled/Living Radical Polymerization, Oxford University Press, Washington, 2000, vol. 768, pp. 2–26 Search PubMed.
-
M. Gilbert, Brydson's Plastic Materials, Elsevier, London, 8th edn, 2017 Search PubMed.
-
P. L. Silveston and R. R. Hudgins, Periodic Operation of Chemical Reactors, Butterworth-Heinemann, London, 2012 Search PubMed.
- A. Ghadban and L. Albertin, Synthesis of Glycopolymer Architectures by Reversible-Deactivation Radical Polymerization, Polymers, 2013, 5, 431–526 CrossRef.
- S. Aksakal, V. P. Beyer, R. Aksakal and C. Remzi Becer, Copper Mediated RDRP of Thioacrylates and their Combination with Acrylates and Acrylamides, Polym. Chem., 2019, 10, 6622–6629 RSC.
- A. Goto and T. Fukuda, Kinetics of Living Radical Polymerization, Prog. Polym. Sci., 2004, 29, 329–385 CrossRef CAS.
- N. Pullan, M. Liu and P. D. Topham, Reversible Addition-Fragmentation Chain Transfer Polymerization of 2-chloro-1,3-butadiene, Polym. Chem., 2013, 4, 2272–2277 RSC.
- J. Kolomanska, P. Johnston, A. Gregori, I. Fraga Domínguez, H. J. J. Egelhaaf, S. Perrier, A. A. Rivaton, C. Dagron-Lartigau and P. D. Topham, Design, Synthesis and Thermal Behaviour of a series of Well-Defined Clickable and Triggerable Sulfonate Polymers, RSC Adv., 2015, 5, 66554–66562 RSC.
- K. Philipps, T. Junkers and J. J. Michels, The Block Copolymer Shuffle in Size Exclusion Chromatography: The Intrinsic Problem with using Elugrams to Determine Chain Extension Success, Polym. Chem., 2021, 12, 2522–2531 RSC.
Footnote |
† Electronic supplementary information (ESI) available. See DOI: 10.1039/d1py00672j |
|
This journal is © The Royal Society of Chemistry 2021 |