DOI:
10.1039/D0FD00132E
(Paper)
Faraday Discuss., 2021,
230, 344-359
Enhanced bio-production from CO2 by microbial electrosynthesis (MES) with continuous operational mode†
Received
10th December 2020
, Accepted 11th January 2021
First published on 29th January 2021
Abstract
Technologies able to convert CO2 to various feedstocks for fuels and chemicals are emerging due to the urge of reducing greenhouse gas emissions and de-fossilizing chemical production. Microbial electrosynthesis (MES) has been shown a promising technique to synthesize organic products particularly acetate using microorganisms and electrons. However, the efficiency of the system is low. In this study, we demonstrated the simple yet efficient strategy in enhancing the efficiency of MES by applying continuous feeding regime. Compared to the fed-batch system, continuous operational mode provided better control of pH and constant medium refreshment, resulting in higher acetate production rate and more diverse bio-products, when the cathodic potential of −1.0 V Ag/AgCl and dissolved CO2 were provided. It was observed that hydraulic retention time (HRT) had a direct effect on the pattern of production, acetate production rate and coulombic efficiency. At HRT of 3 days, pH was around 5.2 and acetate was the dominant product with the highest production rate of 651.8 ± 214.2 ppm per day and a significant coulombic efficiency of 90%. However at the HRT of 7 days, pH was lower at around 4.5, and lower but stable acetate production rate of 280 ppm per day and a maximum coulombic efficiency of 80% was obtained. In addition, more diverse and longer chain products, such as butyrate, isovalerate and caproate, were detected with low concentrations only at the HRT of 7 days. Although microbial community analysis showed the change in the planktonic cells communities after switching the fed-batch mode to continuous feeding regime, Acetobacterium still remained as the responsible bacteria for CO2 reduction to acetate, dominating the cathodic biofilm.
Introduction
Adverse effects of CO2 emissions in the atmosphere on the environment such as climate change have raised the urgent demand on new technologies to capture CO2. Different methods have been suggested and studied in order to either capture or utilize CO2 from the atmosphere.1 Electrochemical technologies are one of the known techniques for CO2 reduction using chemical catalysts. However the utilisation of these chemicals is associated with sustainability issues and lack of robustness, in addition to the cost of the chemical catalysts themselves. Additionally, products from electrochemical methods are typically limited to one-carbon compounds such as formate, methanol, and unsaturated two-carbon compounds such as ethylene, as recently reported.2 Bio-electrochemical systems (BESs) have provided a biological and electrochemical approach towards CO2 reduction using microorganisms as catalysts.3,4 Acetogenic microorganisms are able to produce medium and long chain organics such as acetate, butyrate, caproate and their respective alcohols from CO2 as a carbon source and a polarised cathode as an energy source.5 Production of these valuable compounds with significant commercial applications from CO2 can contribute towards a circular economy. Acetate is the major product of CO2 reduction via microbial electrosynthesis (MES) following the Wood–Ljungdahl pathway.6 Production of longer chain organics than acetate through MES was reported in previous studies by controlling fermentation pathways.3,7 However, chain elongation in BES and selectivity in production of C4 or C6 organics is still the major challenge. It has been reported in previous studies that operational conditions provided in BES have an important effect not only on enrichment of desired microbial communities but also on selectivity of products.8 pH is one of the key operational parameters affecting microbial metabolisms. Optimal pH for production of acetate through acetogenesis mechanism is reported as between 5.0 and 7.0.9 On the one hand, it was reported that acidic pH can inhibit metabolisms of Clostridium and subsequently chain elongation through acetate.10 On the other hand, it was proposed that it can enhance the production of alcohols in the system by switching microbial metabolism from acetogenesis to solventogenesis.10–13 To prevent the accumulation of acidic products and control the pH of the catholyte, different strategies such as designing three-chamber reactors were investigated.14,15 Continuous operational mode is another solution for controlling the pH in BES. Continuous feeding of fresh medium is a proper method not only to extract the products accumulated in the system, but also to constantly provide fresh growth medium and CO2 to microbial communities involved in the MES processes. We have shown in our previous study that compared with bicarbonate, gaseous CO2 leads to higher abundance of Acetobacterium, by improving the protein synthesis of bacteria.16 In addition, availability of inorganic carbon for bacterial communities is important as some acetogens cannot grow unless CO2 is promptly accessible.6 However, selection of hydraulic retention time (HRT) is an important factor in continuous mode, as a fast flow rate can wash out the microbial communities from a cathodic compartment in the case of absence of biofilm and presence of planktonic cells responsible for MES. In a previous study by Bajracharya et al.,17 higher acetate production rate (149 mg per L per day) was achieved over batch experiments compared to that in continuous operational mode. However in their study, the acetate production was not stable over the long-term continuous mode due to the removal of microbial communities in suspension responsible for MES. Similar results were reported by Arends et al.18 As planktonic microbial cells were responsible for MES in their study, the authors reported the decrease in biomass and the consequent drop in acetate production at the HRT of 2.9 ± 0.3 days.18 Conversely, in our previous study, we showed that microbial communities responsible for MES processes were enriched at the surface of the cathode, developing a dense and highly conductive biofilm.16 Almost 50% of the bacterial communities in the biofilm were composed of Acetobacterium, and acetate was the major product with the average coulombic efficiency of 69% while pH was around 5.8. However, lower pH than 5.8 seemed to decrease the efficiency.16 Additionally, chemical electron donors such as ethanol provided in BES were shown as key energy sources for production of longer chain compounds than acetate under controlled pH although with negligible concentrations.19 Therefore, in order to control the pH and optimize the system’s efficiency regarding acetate production, and particularly steering the production towards longer chain compounds, continuous feeding strategy was carried out in this work. We hypothesized that switching from fed-batch to continuous operational mode could provide optimal conditions for the cathodic biofilm by removing the acidic products and flushing fresh nutrients, leading to an improvement of the production from CO2. Therefore, the effect of continuous operational mode on production and efficiency was investigated in this study. Due to the importance of HRT in controlling the pH, different HRTs were applied during the operation and their effects on production were investigated. In addition, the electrochemical feature of the biofilm developed during the continuous feeding regime and microbial composition in the catholytes were compared with those observed after fed-batch mode.
Material and methods
BES operation
Reactors in this study were designed and operated as previously described.16 Briefly, each reactor was a Perspex® dual chamber cell with net volume of 80 mL. Each chamber had a 70 mL glass vessel attached on top used as a headspace. Chambers were separated by a cation exchange membrane (FUMASEP-FKB-PK-130, Fumatech, Germany). Cathode was graphite felt (VWR, Cat. No. 43200.RR, Alfa Aesar) with a projected surface area of 4.9 cm2 and anode was a platinum mesh with the same size as the cathode connected to titanium wire as a current collector. Reference electrode of Ag/AgCl saturated in 3 M NaCl (+0.210 V vs. Standard Hydrogen Electrode, SHE, RE-5B, BASi, USA) was placed in the cathodic compartment. All the potentials in this study are reported vs. Ag/AgCl. The BESs were operated in duplicate and all the results were reported as average of the replicates.
The cathodic medium consisted of K2HPO4 (0.35 g L−1), KH2PO4 (0.25 g L−1), NH4Cl (0.25 g L−1), KCl (0.5 g L−1), CaCl2·2H2O (0.15 g L−1), MgCl2·6H2O (0.6 g L−1), NaCl (1.2 g L−1), yeast extract (0.01 g L−1), trace metal solution (1 mL L−1), vitamin solution (2.5 mL L−1), tungstate–selenium solution (0.1 mL L−1).20 Cathodic compartments were inoculated by the catholyte (40 mL) of the reactors in our previous study.16 pH of the initial medium before starting the experiment was adjusted to around 5.8. No chemical methane inhibitor was added to the cathodic medium, as an acidic pH was shown as an efficient method to suppress methanogens.16,21 The oxygen produced throughout the experiment in the anodic compartment was removed by purging the anolyte continuously by N2. Reactors were operated at the controlled temperature of 35 °C by employing an incubator.
Reactors were started and operated in fed-batch mode until acetate production was observed (14 days) by purging CO2/N2 (90/10) in catholytes for 20 minutes every 2.5 ± 0.5 days. Afterwards operational mode was switched to continuous feeding regime using an aquarium dosing pump. HRT of the pump was first adjusted at 3 days, which was sufficient for maintaining the catholyte’s pH at around 5.8. After 42 days at the HRT of 3 days, retention time was changed to 7 days to compare the production at different retention times.
Analytical methods
Concentrations of organic acids (C1 to C6) in liquid samples were measured using ion chromatography (Eco IC, Metrohm, Switzerland) equipped with the METROHM 6.1005.200 column and autosampler. Gas chromatography (GC-2010, Shimadzu Tracera, Japan) equipped with Barrier Discharge Ionization (BID) detector, autosampler and a Zebron ZB-WAXplus capillary column (Phenomenex) was used to measure and detect acetone and alcohols (C1 to C6). Gas samples were analysed using a gas chromatography (GC-2010, Shimadzu Tracera, Japan) equipped with Barrier Discharge Ionization (BID) detector and ShinCarbon ST micropacked column 80/100 (Restek) to detect the gas (H2, N2, O2, CH4 and CO2) in the headspaces of the reactors. To measure total inorganic and organic carbon in the solutions, a TOC 5050A Total Organic Carbon analyser (Shimadzu, Japan) equipped with ASI-5000 autosampler was used. pH of the liquid samples were measured after each sampling, using HI 9025 pH meter (Hanna Instruments, USA) with a pH probe (11542543; Fisher-Scientific, UK) calibrated between 2.0 and 9.0.
Electrochemical analysis
To compare the electrochemical activity of the biofilm formed after the fed-batch mode and the biofilm developed further after the continuous feeding regime, cyclic voltammetry (CV) was performed from the cathodes at the end of continuous operational mode experiment and compared with the CV performed at the end of batch-fed mode (previous study16) and plain electrode. Before the analysis, 50% of the catholyte was refreshed and pH was adjusted to around 6.7 to discard the effect of pH on the CV results. The scan rate was set at 2 mV s−1 with the potential step of 0.5 mV and the second scan was reported.
Biological analysis
At the end of experiment in this study, 3 mL samples were collected from the catholytes for further biological analysis. Samples for cell count analysis were fixed in 50% ethanol immediately after collection. The number of the planktonic cells was measured using flow cytometry with Accuri C6 flow cytometer (BD Biosciences). Samples were prepared for flow cytometry following the sample preparation step as reported in the previous study22 and stained by SYTO 9 green fluorescent nucleic acid stain. Negative control (stained sterile filtered EDTA) was prepared to separate the signals of the cells from the background noise through electronic gating. To verify the identity of bacterial communities in the planktonic cells, DNeasy PowerWater Kit (14900-100-NF, QIAGEN, Germany) was used to extract DNA of the samples by filtering the samples through 0.2 μm nylon membrane filters using filter holders (Swinnex, China) and placing the filters in the kits. The communities of samples were sequenced by Illumina MiSeq sequencing through evaluating the V4 region of 16S rRNA (Northumbria University, UK). The Illumina MiSeq sequencer produced 2 × 250 bp paired end reads with V2 chemistry. The data from Illumina MiSeq were processed using the pipeline of the “Dada 2” package in the R statistical platform. The representative sequences in each amplicon sequence variant (ASV) files were assigned using the Ribosomal Database Project (RDP) with the bootstrap confidence of 80. After taxonomy assignment, the relative abundance of each taxon was determined and plotted using the “phyloseq” package in the R statistical platform.23 To plot a phylogenetic tree for selected sequences of this study and compare to highly similar sequences recovered from the NCBI nucleotide database collection, the “ape” package after sequences alignment using the “msa” package in the R statistical platform was used.24–26 Analysis of variance (ANOVA) tests were performed to assess the reliability of the data. Accuracy of the analysis was controlled by a positive control using a mock bacterial community with specific composition of bacteria (ZymoBIOMICS Microbial Community Standard). Three negative controls with no sample were used for detecting the contamination during the DNA extraction processes. When the similarity of the samples and negative controls was less than 10%, samples were considered as non-contaminated. However, higher similarity showed the contamination of the samples, therefore were removed from the analysis.
Results and discussion
Effect of continuous operational mode on production through MES (HRT: 3 days)
Reactors were started on fed-batch mode for 2 weeks until notable acetate production was observed. Inoculating from the active and developed biocathode led to the rapid start-up of the BESs in cathodic electron uptake and production of acetate. Cathodic current started from −0.6 ± 0.0 mA cm−2 immediately after starting the BESs, and gradually increased to around −1.0 ± 0.5 mA cm−2 at day 14 (Fig. S1†). After 14 days, operational mode was switched to continuous feeding regime with HRT: 3 days. Retention time of 3 days was selected according to our previous observations, where the catholyte pH was decreasing to around 4.8–5.0 after approximately 3 days. Therefore, HRT of 3 days was chosen to prevent the highly acidic condition in the catholytes. During the experiment of continuous feeding regime, cathodic current varied between −1.0 and −1.5 mA cm−2 until day 56, the last day of applying HRT of 3 days.
During the first 14 days of the experiment acetate was the major product detected in the catholytes, reaching the concentration of 2297.3 ± 29.5 ppm (76.5 ± 0.9 mmol C per L) at day 14, with acetate production rate of 155.2 ± 71.0 and 102.1 ± 30.2 ppm per day at day 7 and 14, respectively (Fig. 1). After switching the operational mode to continuous on day 14, acetate concentration began to decrease due to removal of the acetate accumulated in the catholytes. However, after day 35, acetate production rate began to increase again, reaching the maximum production rate of 651.8 ± 214.2 ppm per day (21.7 ± 7.14 mmol C L−1 per day), with the maximum of 803.3 ppm per day (26.8 mmol C L−1 per day) in one of the duplicates on day 44, 30 days after switching the operation to continuous mode. In addition, over the first 14 days of fed-batch mode the catholyte pH dropped from 5.6 ± 0.0 at day 1 to 4.4 ± 0.4 at day 14 (Fig. 1). After switching from fed-batch to continuous mode, the constant removal of products from the catholytes and feeding fresh medium could successfully adjust the pH around 5.5. The higher acetate production rate observed in continuous mode was also associated with a significant increase in coulombic efficiency (Fig. 2). In fed-batch mode, the coulombic efficiency was 58.4 ± 23.0% (averaged over the first 14 days). After switching the operational mode to continuous with HRT of 3 days, acetate concentration in the solution began to decrease (average of 55.2 ± 14.8% coulombic efficiency between days 14 and 35) most likely due to the removal of biomass. However, coulombic efficiency slowly increased and reached the average value of 83.2 ± 10.5% between days 35 and 56, which was the highest value during the experiment (maximum of 90% in one of the duplicates). This was the highest coulombic efficiency achieved in this study, indicating the positive impact of continuous feeding regime on the efficiency of MES.
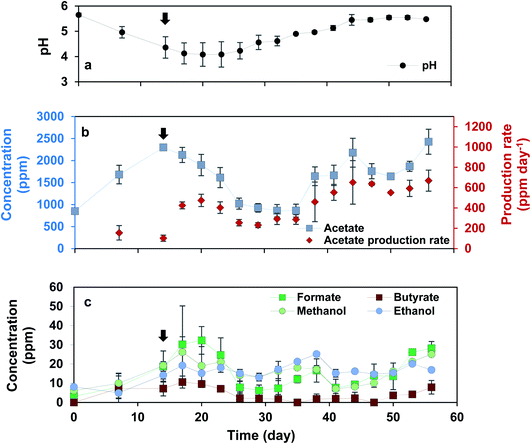 |
| Fig. 1 (a) pH, (b) acetate concentration and production rate and, (c) other products than acetate detected in the BESs poised at −1.0 V and fed by CO2 before and after changing to continuous operational mode at HRT of 3 days. Black arrows show the time when operational mode was switched from fed-batch to continuous. The error bars represent the standard deviation calculated from duplicate samples in two reactors. | |
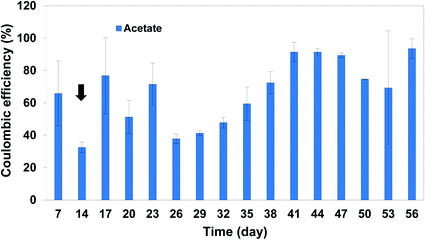 |
| Fig. 2 Coulombic efficiency of BESs during the experiment of continuous operational mode (HRT: 3 days). Black arrow shows the time when operational mode was switched from fed-batch to continuous. The error bars represent the standard deviation calculated from duplicate samples in two reactors. | |
Over 14 days of fed-batch mode and 42 days of continuous operational mode with HRT: 3 days, acetate was the major product detected in the solution, and no H2 was found in the headspaces. Trace concentration (less than 40 ppm) of formate, butyrate, methanol and ethanol were detected irregularly in the catholytes, with no significant change in the production patterns over the first 56 days (Fig. 1(c)). The number of cells in the catholytes measured by flow cytometry on day 14, before switching the operational mode, and on day 56, last day of continuous mode at HRT of 3 days, was almost similar, indicating the persistent cells growth. The cells number was around 5.5 × 107 ± 4.5 × 107 on day 14 and 1.1 × 107 ± 4.6 × 106 on day 56. Slight decrease in the number of the cells could be due to the removal of biomass accumulated in suspension.
Effect of continuous operational mode on production through MES (HRT: 7 days)
After 42 days operation of continuous flow with HRT 3 days, the retention time was changed to a longer period of 7 days (Fig. 3). Comparing the results of HRT 3 and 7 days could show the impact of retention time on production through MES.
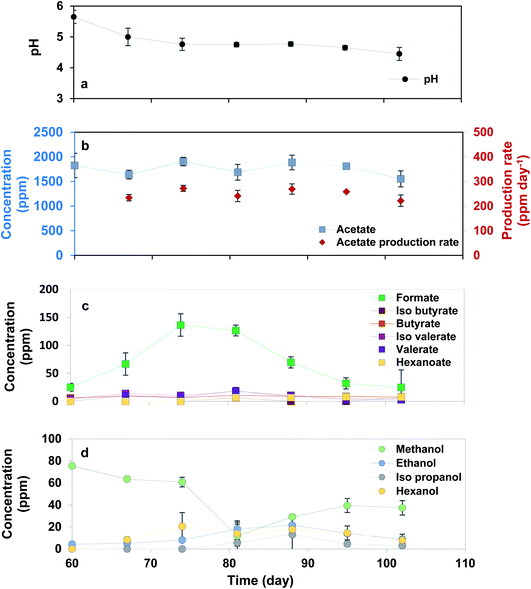 |
| Fig. 3 (a) pH, (b) acetate concentration and production rate, (c) carboxylic acids, and (d) alcohols detected in the BESs poised at −1.0 V and fed by CO2 at continuous operational mode at HRT of 7 days. The error bars represent the standard deviation calculated from duplicate samples in two reactors. | |
Current density at HRT: 7 days was around −1.0 mA cm−2 (Fig. S2†). During the experiment at HRT: 7 days, acetate concentration detected in the solution was lower but more stable than that detected in BESs at HRT: 3 days. The highest acetate concentration (1904.1 ± 86.2 ppm (63.5 ± 2.8 mmol C per L)) was achieved on day 74 of the experiment corresponding to a production rate of 272.0 ± 12.3 ppm (9.1 ± 0.4 mmol C per L). This difference in acetate production could be due to an accumulation of products and a drop in pH during HRT of 7 days (less than 5), which is known for providing toxic conditions for acetogenic activity.27–29 Formate was detected with higher concentration than that observed in HRT: 3 days, at around 140 ppm, 15 days after switching the retention time to 7 days, however its concentration was not stable. Applying longer retention time led to pH drop to around 4.3–4.7 during the experiment. This was associated with production of diverse products of butyrate, isobutyrate, valerate, isovalerate, hexanoate and hexanol, which were not detected in the reactors at HRT of 3 days. Although the concentration of these products was less than 25 ppm, it was the first time that C6 products were detected during this experiment. It is highly probable that acetate was converted to ethanol under highly acidic condition over a solventogenesis mechanism, which was further consumed for production of C4 products with acetate.30 Presence of butyrate and ethanol in the solution could result in further chain elongation and production of C6 products.30 However, the trace concentration of C4 and C6 products in our reactors could be related to the negligible abundance of Clostridium (<1%) compared to the acetate producing community of Acetobacterium found in the initial inoculum.16 Similar observation was reported in a previous study,10 in which the authors discussed that pH lower than 5.0 ceased the production of long chain VFAs and promoted the production of alcohols through a solventogenesis mechanism. The production of C5 products in our study was highly related to Prevotella found in the initial inoculum and known for production of propionate as discussed elsewhere.16 Propionate can be further elongated to C5 products using methanol or ethanol.31 In addition, the number of cells in the catholytes increased slightly to 3.9 × 107 ± 1.8 × 106 after 42 days operation at HRT: 7 days compared to that observed over HRT: 3 days, most likely indicating the accumulation of growing biomass in the suspension. However, coulombic efficiency of the system in acetate production improved during continuous feeding regime compared to that at fed-batch mode. Due to the low concentration of other products than acetate and lack of detection of hydrogen in BESs, coulombic efficiency was calculated considering the acetate production as illustrated in Fig. 4. Similar to HRT: 3 days, coulombic efficiency was higher than that obtained for fed-batch mode, with the highest value of approximately 81% over the last 21 days. This was slightly lower than that calculated for HRT: 3 days, which can be explained by the fact that acetate (used for the coulombic efficiency calculations) was further elongated to longer compounds.
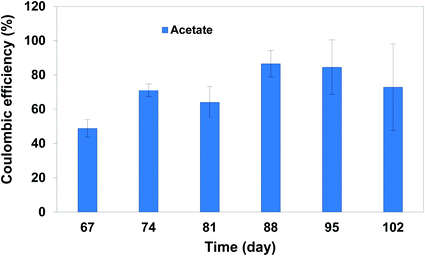 |
| Fig. 4 Coulombic efficiency of BESs during the experiment over the continuous operational mode (HRT: 7 days). The error bars represent the standard deviation calculated from duplicate samples in two reactors. | |
Effect of continuous operational mode on electrochemical properties of biofilms
During the continuous feeding regime, mature biofilms developed at the surface of cathodes which were visible to the naked eye (Fig. S3†). Formation of dense biofilms at the cathode could be a result of constant supply of fresh nutrients for the microbial communities. The significant correlation between the biofilm density developed at the cathode and acetate production yield was clearly demonstrated in our previous study,16 which justifies the superior performance of the BESs over continuous feeding regime. Development of a dense biofilm at the surface of the cathodes raised the question of the difference in electrochemical properties of the biofilms developed in different operational mode conditions. Therefore, CV was performed on the plain electrode prior to the beginning of the experiment and on the electrode with the biofilm after 102 days of experiment (Fig. 5). The voltammograms were also compared with that from the electrodes covered with a biofilm after 104 days of fed-batch mode reported in our previous study.16 For CV analysis, pH in the reactors was manually adjusted to around 6.5 to avoid any implication of pH on the CV results. As observed in Fig. 5, a significant shift in the H2 evolution onset potential could be observed. The onset potential of H2 evolution was near −1.0 V on the plain electrode and shifted to −0.8 V at the end of the fed-batch experiment. This shift was even more significant at the end of the continuous feeding regime experiment to ca. −0.6 V. This could be related to the significant abundance of Acetobacterium, since it was shown its enzymatic activity at the surface of the cathode led to higher enzymatic production of intercellular H2.16 H2 is an energy source for acetogens according to eqn (1). | 2CO2 + 4H2 → CH3COOH + 2H2O | (1) |
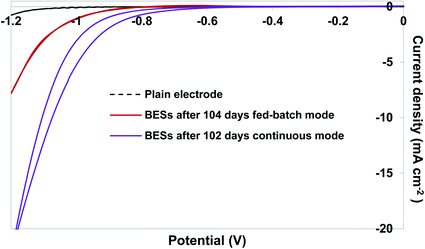 |
| Fig. 5 Cyclic voltammograms recorded on the plain electrode, cathode with biofilm formed after 102 days at continuous operational mode and cathode with biofilm formed after 104 days at fed-batch mode (our previous study16). | |
Although it was primarily suggested that acetogens can uptake electrons directly from the polarised cathode in BES,32 we showed in our previous study that acetogens gain electrons most likely in the form of H2 produced extracellularly and also intracellularly for CO2 reduction.16 Considering the importance of H2 as an energy source in MES, CV results in this study strongly confirmed the positive effect of the continuous feeding regime on biological production of H2, even though no H2 was detected in the headspaces of the BESs showing its consumption through MES processes. This justifies the more significant production rate of acetate during continuous operational mode compared to fed-batch mode.
Bacterial communities in planktonic cells
Initial inoculum used for this study was dominated by Acetobacterium responsible for production of acetate through MES. It was shown in our previous study that Acetobacterium grow as a dense and efficient biofilm at the surface of polarised cathodes under optimal conditions (gaseous CO2, cathodic potential of −1.0 V, and slightly acidic pH (ca. 5.8)), while lower abundance of Acetobacterium (ca. 7%) was still detected in the bulk.16 Therefore, it was assumed that inoculating the BES with previously enriched catholyte and imposing the favourable conditions for Acetobacterium growth would lead to the formation of biofilm also dominated by Acetobacterium while lower abundance would be found in the catholytes.
The relative abundance of Acetobacterium in planktonic cells of the catholytes (ca. 1%) in this study was slightly lower than that in fed-batch mode (Fig. 6). This could be due to the removal of biomass from the solution over continuous operation. In addition, this could confirm the fact that Acetobacterium tend to grow and form biofilm at the surface of the cathode, where H2 produced intercellularly and extracellularly is highly available as an energy source,16 justifying the significant acetate production over continuous mode. However, the most significant change in microbial communities of planktonic cells was related to development of Pullulanibacillus. Detected as the most abundant genus in the catholytes, it comprised 69.8 ± 1.3% of microbial communities, while it was only 23.5 ± 2.2% in the initial inoculum. Pullulanibacillus is classified in the family of Bacillaceae_2 which is the spore-forming bacteria from Firmicutes with the optimum temperature of 37 °C and acidic pH of around 4.5.33–35 By far, little is known about Pullulanibacillus, however a highly similar sequence was found in the acetate producing bioreactors from CO2 and H2 with acidic pH similar to operational conditions in the BESs of our study.35 In addition, continuous flushing of fresh medium could decrease the abundance of a fermentative bacteria such as Amnibacterium or Rummeliibacillus known to be correlated to the presence of butyrate and isopropanol as discussed by Arends et al.18 Results from the community analysis of planktonic cells in the catholytes, together with the significant enhancement in acetate production over the continuous mode in our study proposed that continuous mode provided the optimal condition for the growth of Acetobacterium not in the catholytes but at the cathodes, where more H2 is available. However, long-term acidic condition in the cathodic compartment seemed to develop a fermenter of Pullulanibacillus and its role in MES requires further studies.
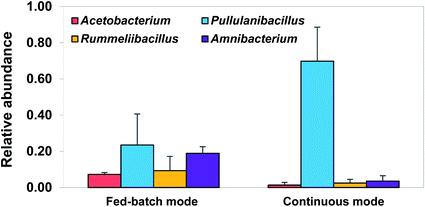 |
| Fig. 6 Relative abundance of the most abundant microbial communities obtained from Illumina sequencing of 16S rRNA genes from planktonic cells of bio-electrochemical reactors after 102 days experiment of continuous operational mode compared to planktonic cells after 104 days in fed-batch mode from our previous study.16 The error bars represent the standard deviation calculated from duplicate samples in two reactors. | |
It is worth mentioning that, although no chemical methane inhibitor was used in the medium, no Archaea was found in the catholytes of this study confirming the successful suppression of methanogens in the BESs under acidic conditions. Additionally, negligible concentration of longer chain products than acetate seemed to be due to the low abundance of Clostridium (<2%) found in the reactors.
Effect of continuous operational mode on BES performance
It was previously observed that a slightly acidic medium (pH between 5.5 and 5.8) could provide optimal conditions for acetogenic growth and subsequently MES processes.16 Similar result was also reported in other studies.36,37 In this work, switching the operational mode from batch to continuous feeding regime showed the promising strategy for improvement in acetate production and efficiency of the system. Highest acetate production rate (803.3 ppm per day) in the experiment was achieved with the continuous operational mode at HRT of 3 days when pH was stabilised at around 5.5. In addition, achieving a coulombic efficiency of more than 80% showed the significant improvement of the efficiency of the system with the continuous operational mode at both retention times of 3 and 7 days in comparison with that (maximum ∼69%) over fed-batch operation observed previously.16 The Wood–Ljungdahl pathway is the only CO2 fixation pathway that is contributing to energy conservation.38 Indeed, cell growth and production of acetate from CO2 is coupled with ATP formation. Therefore, higher biomass turnover rate can lead to enhancement in the performance of BESs in terms of electron recovery and acetate production.38 By comparing two HRTs of 3 and 7 days, it could be concluded that a shorter retention time implied higher removal rate of biomass and products, and providing soluble CO2 constantly available, leading to higher energy demands for microorganisms and thus higher growth rate. Providing soluble CO2 is postulated as a key reason for optimizing the system. Removal of acetate from the catholytes, the major product during continuous flow experiment, and providing soluble CO2 could divert the acetate production reaction (eqn (1)) from CO2 to higher production of acetate to achieve the equilibrium. Similar trend was observed in other studies, in which continuous operational mode enhanced the production of organics from soluble CO2 and chain elongation (production of C4 and C6 products) significantly,8 or C4 production in the bio-electrochemical reactor.39 Arends et al.18 reported the highest acetate production rate at the lowest HRT (3.3 days), and detection of longer chain products such as isopropanol and butyrate during longer retention time than 3.3 days. However, as H2 was the second significant product in their study, coulombic efficiency based on acetate production (∼63%) was lower than that calculated in our study. Although continuous feeding regime strategy in our study was applied to steer the production towards longer chain products, acetate was still the major product most likely due to the microbial communities dominated by Acetobacterium enriched from initial inoculum. This could also be related to the shorter term of experiment (102 days) in our study compared to previous studies operated for more than 200 days.8,40,41
Selective and efficient production of organic compounds from CO2 through MES is one of the challenges in cathodic applications of BESs. So far, different studies reported the production of organic compounds from CO2 through MES.1 However, reaching a high selectivity, particularly towards compounds longer than acetate is still a challenge. Several methods have been proposed and investigated to steer the production towards longer chain organics, such as controlling pH, optimising different operational modes or developing strategies to quickly and efficiently grow mature biofilms through anodic reactions before switching polarisation.42 It should be noted that switching the polarity of the electrode to develop cathodic biofilms typically leads to development of methanogens.43 However, from an economical point of view, the production of organic fatty acids is more favourable than methane. Feeding regime optimisation seems to be one of the key methods for the selectivity of the products, not only by providing constant CO2 for bacterial activity, but also by regularly flushing fresh nutrients for microorganisms, in addition to removing the products which provide toxic conditions for microorganisms.44,45 In addition, the constant removal of the products also affects the pH of the catholyte, an important factor on microbial activities. Jourdin et al. reported maximum acetate, butyrate and caproate production rates of 9.8 ± 0.65 g per L per day, 3.2 ± 0.1 g per L per day, and 0.95 ± 0.05 g per L per day, respectively.40 The authors suggested that the continuous extraction of products and providing fresh nutrients significantly contributed to the formation of a dense biofilm responsible for such a high production. The selective production of butyrate and caproate was further studied by Jourdin et al. by controlling the HRT and CO2 feeding.8 Although operational conditions play key roles in the selectivity of the products as observed in this study, the nature and selectivity of the products strongly depend on the microbial communities enriched in biocathodes, either in biofilms or planktonic cells. For instance, the production of longer chain products such as caproate and caprylate in BES was explained by Clostridium kluyveri dominating mixed population of microorganisms, in which both acetate and CO2 were provided for microbial communities.46 However, it seemed that optimizing the operational conditions of BES by applying continuous feeding regime in our study could not improve the growth of Clostridium, leading to low concentration of longer chain compounds than acetate. One reason could be the H2 partial pressure affecting the growth of different microbial communities. It was discussed in a recent study that Clostridium requires high H2 partial pressure for growth,47 which explains the enrichment of Clostridium from mixed communities and in turn the production of C4 and C6 compounds observed in gas fermentation bioreactors,48,49 in contrast to lower H2 partial pressure in cathodic BES compartments. Although H2 production increased significantly according to CV results in our system, it probably was consumed for acetate production mainly by Acetobacterium dominating the biofilm communities. However, compared to gas fermentation bioreactors, BESs have the advantage of providing an available and constant energy source.50–52 In addition, as H2 seems to be the main electron mediator between the cathode and microbial communities,16 enhancing H2 production at the cathode could force the cathodic reactions towards long chain compounds production. This was suggested by Kracke et al. by studying the biocompatible catalysts for improvement of H2 evolution.53 At the cathodic potential of −1.1 V, H2 production and consequently acetate and methane production from S. ovata and M. maripaludis, respectively, were significantly enhanced through MES at a fed-batch mode.53 Combining such a technology under continuous feeding regime can be an interesting approach to improve Clostridium enrichment from mixed population of microorganisms in bio-electrochemical reactors, contributing in steering the production toward longer chain organics.
Conclusion
In this study, we demonstrated a simple yet effective strategy for improving the efficiency of microbial electrosynthesis particularly for acetate production by applying continuous feeding mode. Hydraulic retention time was shown to have an important effect on the production by helping to control the pH. Production of acetate from CO2 in the bio-electrochemical system was increased significantly at the retention time of 3 days and reached the maximum coulombic efficiency of ca. 90%. Lower coulombic efficiency for acetate production (ca. 81%) but more diverse products (butyrate, isobutyrate, valerate, isovalerate, hexanoate and hexanol), although with trace concentrations, were observed at the retention time of 7 days. No H2 was detected in the reactors during 102 days of experiment, when cyclic voltammetry showed a notable shift in H2 onset potential (ca. 400 mV) at the end of experiment, indicating its consumption over the organics production, which justified the significant improvement of the overall efficiency of the system.
Conflicts of interest
There are no conflicts to declare.
Acknowledgements
The authors thank EPSRC LifesCO2R project (EP/N009746/1), EPSRC NECEM (EP/R021503/1), NERC MeteoRR (NE/L014246/1) and NBIC 002POC19034. P. I thanks the Doctoral Training Awards (SAgE DTA, 2015 cohort) from Faculty of Science, Agriculture and Engineering, Newcastle University for supporting her PhD study. The authors also thank funding support from NBIC 002POC19034 and the Open Fund Project for State Key Laboratory of Clean Energy Utilization with Zhejiang University, project number ZJUCEU2019004.
References
- Y. Jiang, H. D. May, L. Lu, P. Liang, X. Huang and Z. J. Ren, Water Res., 2018, 149, 42–55 Search PubMed.
- H. S. Jeon, S. Kunze, F. Scholten and B. Roldan Cuenya, ACS Catal., 2018, 8, 531–535 Search PubMed.
- J.-M. Fontmorin, P. Izadi, S. Rasul and E. H. Yu, Transformations, 2019, 27, 561–582 Search PubMed.
- P. Izadi and E. Yu, Joule, 2020, 4, 2085–2087 Search PubMed.
- Y. Jiang and R. J. Zeng, Bioresour. Technol., 2018, 269, 503–512 Search PubMed.
-
H. L. Drake, K. Küsel and C. Matthies, in The prokaryotes, Springer, 2006, pp. 354–420 Search PubMed.
- W. de Araújo Cavalcante, R. C. Leitão, T. A. Gehring, L. T. Angenent and S. T. Santaella, Process Biochem., 2017, 54, 106–119 Search PubMed.
- L. Jourdin, M. Winkelhorst, B. Rawls, C. J. Buisman and D. P. Strik, Bioresour. Technol. Rep., 2019, 7, 100284 Search PubMed.
-
A. Balows, H. G. Trüper, M. Dworkin, W. Harder and K.-H. Schleifer, The prokaryotes: a handbook on the biology of bacteria: ecophysiology, isolation, identification, applications, Springer Science & Business Media, 2013 Search PubMed.
- R. Ganigué, P. Sánchez-Paredes, L. Bañeras and J. Colprim, Front. Microbiol., 2016, 7, 702 Search PubMed.
- I. Vassilev, P. A. Hernandez, P. Batlle Vilanova, S. Freguia, J. O. Krömer, J. Keller, P. Ledezma and B. Virdis, ACS Sustainable Chem. Eng., 2018, 6, 8485–8493 Search PubMed.
- H. Richter, M. Martin and L. Angenent, Energies, 2013, 6, 3987–4000 Search PubMed.
- M. E. Martin, H. Richter, S. Saha and L. T. Angenent, Biotechnol. Bioeng., 2016, 113, 531–539 Search PubMed.
- S. Gildemyn, K. Verbeeck, R. Slabbinck, S. J. Andersen, A. Prévoteau and K. Rabaey, Environ. Sci. Technol. Lett., 2015, 2, 325–328 Search PubMed.
- I. Vassilev, F. Kracke, S. Freguia, J. Keller, J. O. Krömer, P. Ledezma and B. Virdis, Chem. Commun., 2019, 55, 4351–4354 Search PubMed.
- P. Izadi, J.-M. Fontmorin, A. Godain, E. H. Yu and I. M. Head, npj Biofilms Microbiomes, 2020, 6, 1–15 Search PubMed.
- S. Bajracharya, K. Vanbroekhoven, C. J. Buisman, D. P. Strik and D. Pant, Faraday Discuss., 2017, 202, 433–449 Search PubMed.
- J. B. A. Arends, S. A. Patil, H. Roume and K. Rabaey, J. CO2 Util., 2017, 20, 141–149 Search PubMed.
- P. Izadi, J.-M. Fontmorin, B. Virdis, I. M. Head and E. H. Yu, Appl. Energy, 2020, 116310 Search PubMed.
- S. A. Patil, J. Arends, I. Vanwonterghem, J. Van Meerbergen, K. Guo, G. W. Tyson and K. Rabaey, Environ. Sci. Technol., 2015, 49, 8833–8843 Search PubMed.
- D. A. Jadhav, A. D. Chendake, A. Schievano and D. Pant, Bioresour. Technol., 2018, 277, 148–156 Search PubMed.
- M. Vignola, D. Werner, F. Hammes, L. C. King and R. J. Davenport, Water Res., 2018, 143, 66–76 Search PubMed.
- P. J. McMurdie and S. Holmes, PLoS One, 2013, 8, e61217 Search PubMed.
- E. Paradis, J. Claude and K. Strimmer, Bioinformatics, 2004, 20, 289–290 Search PubMed.
-
E. Paradis, S. Blomberg, B. Bolker, J. Brown, J. Claude, H. S. Cuong and R. Desper, Analyses of phylogenetics and evolution, version, 2019, vol. 2 Search PubMed.
- U. Bodenhofer, E. Bonatesta, C. Horejš-Kainrath and S. Hochreiter, Bioinformatics, 2015, 31, 3997–3999 Search PubMed.
- M. Demler and D. Weuster-Botz, Biotechnol. Bioeng., 2011, 108, 470–474 Search PubMed.
- S. Ge, J. G. Usack, C. M. Spirito and L. T. Angenent, Environ. Sci. Technol., 2015, 49, 8012–8021 Search PubMed.
- L. A. Kucek, M. Nguyen and L. T. Angenent, Water Res., 2016, 93, 163–171 Search PubMed.
- R. González-Cabaleiro, J. M. Lema, J. Rodríguez and R. Kleerebezem, Energy Environ. Sci., 2013, 6, 3780–3789 Search PubMed.
- S. M. De Smit, K. D. De Leeuw, C. J. Buisman and D. P. Strik, Biotechnol. Biofuels, 2019, 12, 132 Search PubMed.
- K. P. Nevin, S. A. Hensley, A. E. Franks, Z. M. Summers, J. Ou, T. L. Woodard, O. L. Snoeyenbos-West and D. R. Lovley, Appl. Environ. Microbiol., 2011, 77, 2882–2886 Search PubMed.
- S. G. Pereira, L. Albuquerque, M. F. Nobre, I. Tiago, A. Veríssimo, A. Pereira and M. S. da Costa, Int. J. Syst. Evol. Microbiol., 2013, 63, 158–162 Search PubMed.
- M. Y. Galperin, Microbiol. Spectrum, 2013, 1, TBS-0015-2012 Search PubMed.
- F. Zhang, J. Ding, N. Shen, Y. Zhang, Z. Ding, K. Dai and R. J. Zeng, Appl. Microbiol. Biotechnol., 2013, 97, 10233–10240 Search PubMed.
- H. N. Abubackar, M. C. Veiga and C. Kennes, Biofuels, Bioprod. Biorefin., 2011, 5, 93–114 Search PubMed.
- P. Batlle-Vilanova, S. Puig, R. Gonzalez-Olmos, M. D. Balaguer and J. Colprim, J. Chem. Technol. Biotechnol., 2016, 91, 921–927 Search PubMed.
- K. Schuchmann and V. Müller, Nat. Rev. Microbiol., 2014, 12, 809 Search PubMed.
- S. M. Raes, L. Jourdin, C. J. Buisman and D. P. Strik, ChemElectroChem, 2017, 4, 386–395 Search PubMed.
- L. Jourdin, S. M. T. Raes, C. J. N. Buisman and D. P. Strik, Front. Energy Res., 2018, 6, 7 Search PubMed.
- S. Srikanth, M. Kumar, D. Singh, M. Singh, S. Puri and S. Ramakumar, Bioresour. Technol., 2018, 265, 66–74 Search PubMed.
- Y. Jiang and R. J. Zeng, Bioresour. Technol., 2018, 271, 439–448 Search PubMed.
- J. M. Pisciotta, Z. Zaybak, D. F. Call, J.-Y. Nam and B. E. Logan, Appl. Environ. Microbiol., 2012, 78, 5212–5219 Search PubMed.
- E. V. LaBelle, C. W. Marshall, J. A. Gilbert and H. D. May, PLoS One, 2014, 9, e109935 Search PubMed.
- L. T. Angenent, H. Richter, W. Buckel, C. M. Spirito, K. J. Steinbusch, C. M. Plugge, D. P. Strik, T. I. Grootscholten, C. J. Buisman and H. V. Hamelers, Environ. Sci. Technol., 2016, 50, 2796–2810 Search PubMed.
- M. C. A. A. Van Eerten-Jansen, A. Ter Heijne, T. I. M. Grootscholten, K. J. J. Steinbusch, T. H. J. A. Sleutels, H. V. M. Hamelers and C. J. N. Buisman, ACS Sustainable Chem. Eng., 2013, 1, 513–518 Search PubMed.
- J. Philips, Front. Microbiol., 2020, 10, 2997 Search PubMed.
- D. González-Tenorio, K. M. Muñoz-Páez, G. Buitrón and I. Valdez-Vazquez, J. CO2 Util., 2020, 42, 101314 Search PubMed.
- D. Vasudevan, H. Richter and L. T. Angenent, Bioresour. Technol., 2014, 151, 378–382 Search PubMed.
- M. Yasin, Y. Jeong, S. Park, J. Jeong, E. Y. Lee, R. W. Lovitt, B. H. Kim, J. Lee and I. S. Chang, Bioresour. Technol., 2015, 177, 361–374 Search PubMed.
- S. Bajracharya, S. Srikanth, G. Mohanakrishna, R. Zacharia, D. P. Strik and D. Pant, J. Power Sources, 2017, 356, 256–273 Search PubMed.
- M. T. Agler, B. A. Wrenn, S. H. Zinder and L. T. Angenent, Trends Biotechnol., 2011, 29, 70–78 Search PubMed.
- F. Kracke, A. B. Wong, K. Maegaard, J. S. Deutzmann, M. A. Hubert, C. Hahn, T. F. Jaramillo and A. M. Spormann, Commun. Chem., 2019, 2, 1–9 Search PubMed.
Footnote |
† Electronic supplementary information (ESI) available. See DOI: 10.1039/d0fd00132e |
|
This journal is © The Royal Society of Chemistry 2021 |