Three promising applications of microbial electrochemistry for the water sector
Received
16th November 2016
, Accepted 14th February 2017
First published on 17th February 2017
Abstract
Microbial electrochemical technologies are based on the interactions between living microorganisms and electrodes. There is a wide range of possible applications and many are highly relevant for the water sector. The most well-known is probably the microbial fuel cell, which has been proposed as an environmentally-friendly process for simultaneous wastewater treatment and electrical energy production. However, full-scale implementation at wastewater treatment plants is very challenging and there are several other applications of microbial electrochemistry that are less well-known to people outside the research field, but potentially could be widely applied and make an impact on the water sector in a shorter time perspective. In this paper, we highlight three such applications: (i) sensors for biochemical oxygen demand, volatile fatty acids and toxicity; (ii) in situ bioremediation of contaminated sites; and (iii) removal and recovery of metals from wastewaters, leachates and brines.
Water impact
Microbial electrochemical technologies have a multitude of possible applications including water and wastewater treatment, resource recovery, chemicals production, and sensing. Many of the applications are highly relevant for the water sector but scale-up and practical implementation is challenging. In this paper, we discuss and review three applications that appear particularly promising: sensors, in situ bioremediation, and metal removal and recovery.
|
1. Introduction
Provision of clean drinking water and treatment of wastewater are essential services that the water sector provides to society. A range of technical processes are applied to remediate contaminated raw water sources, produce clean drinking water, and treat wastewater. Research to improve existing processes and to develop new, more robust and resource-efficient processes is continuously ongoing. In recent years, microbial electrochemical technologies (MET) have received a lot of attention as emerging, environmentally-friendly processes that potentially could contribute to e.g. resource recovery from wastewater. The microbial fuel cell (MFC) is probably the most well-known example. In an MFC, microorganisms oxidize organic compounds and produce electrical current at the anode. Oxygen is reduced at the cathode, which allows production of electrical power.1 Another example is the microbial electrolysis cell (MEC). In an MEC, a small amount of electrical power is invested to drive hydrogen gas production at the cathode.2 A search for the terms “microbial fuel cell”, “microbial electrolysis cell”, and “bioelectrochemical system” in a database such as Scopus or Web of Science gives over 6000 hits (December 2016). About 95% of these papers were published in the last 10 years, which shows the rapid growth of this field in recent years (Fig. 1). Many of the studies have aimed to develop METs for domestic wastewater treatment. Theoretically, such systems could be used to turn wastewater treatment plants into net producers of energy. The energy-consuming activated sludge process, which typically is used to remove organic contaminants from wastewater, could be replaced by METs that recover the energy content of the organic matter, producing electricity or energy carriers. The concept is very attractive and has driven much of the research in the field. However, moving such systems into practice is very challenging for several reasons:
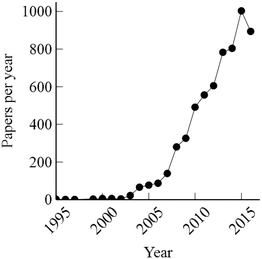 |
| Fig. 1 Papers published per year including at least one of the keywords “bioelectrochemical system”, “microbial fuel cell”, and “microbial electrolysis cell”. The search was done in December 2016 using the Scopus database. | |
• High capital costs. METs require electrodes, current collectors, wiring and membranes and will be more expensive than conventional reactors for wastewater treatment. If an MET is to fully or partially replace an activated sludge system the value generated from the product and the savings from reduced aeration requirements must be high enough to allow for a reasonable payback time of the initial investment in the system.3,4
• Low performance with real wastewater. High current densities and energy efficiencies have been achieved in reactors operated with synthetic nutrient media containing acetate.5 However, the performance is usually much lower with real wastewater.6 The low conductivity of domestic wastewater leads to large potential losses.7 Low alkalinity can also lead to localized pH drops that limit microbial activity.8 Particles and excess microbial growth can cause problems with clogging of pipes and fouling of separators.9 Furthermore, the complexity of organic substrates in real wastewater means that interactions and competitions between functional groups of microorganisms determine the biological current generation. The interaction between fermenters and electroactive bacteria promotes current generation. In an MFC fed with glucose it was shown that glucose was first fermented to acetate and hydrogen, which were used as substrates for current generation by electroactive bacteria.10 The same occurred in reactors fed with ethanol.11 Competition between electroactive bacteria and methanogens can have negative consequences for current generation. Methanogens can utilize hydrogen and acetate as substrates. Particularly hydrogen-utilizing methanogens appear to be significant competitors to electroactive bacteria and their activities can reduce the coulombic efficiencies of biological anodes.11
• Insufficient effluent quality. The primary goal of wastewater treatment plants is to treat wastewater to meet certain effluent limits. Although energy efficiency is an increasingly important aspect, it is not the main consideration for treatment plant operators. Effluent from METs will require post-treatment to meet the limits for biochemical oxygen demand (BOD), nitrogen and phosphorous.12
• Competition from existing processes. The purpose of METs is to reduce energy requirements and increase energy recovery from wastewater. However, the same things can be accomplished with anaerobic digestion, which is a mature process that can operate at higher COD removal rates. Furthermore, large scale production of renewable energy can be accomplished by a wide range of established and emerging technologies such as photovoltaics, wind turbines, water- and wave power.
In addition to large-scale energy recovery from wastewater, microbial electrochemistry has a range of potential applications in the water sector; for example, nutrient removal and recovery, chemicals production, desalination, and degradation of recalcitrant pollutants. Several of these other applications do not suffer from all the challenges listed above and may therefore be applied in practice in a shorter time perspective. In this paper, we highlight three such applications: sensors, in situ bioremediation, and metal removal and recovery. Sensors can be used to monitor water quality and control processes in wastewater treatment plants; in situ bioremediation is used to treat contaminated soil and sediments and is important to prevent contamination of groundwater sources; metal removal and recovery is needed in the treatment of many industrial wastewaters, brines, and leachates. The three highlighted applications of METs are briefly reviewed in sections 2–4 below. Other possible applications of microbial electrochemistry are presented in section 5. Finally, the future of the three highlighted technologies is discussed in section 6.
2. Microbial electrochemical sensors
The ease with which electrical current and potential can be monitored in real-time makes METs attractive as sensors for a range of parameters. METs have been investigated as sensors for BOD, volatile fatty acids (VFA), toxicity, corrosion, extraterrestrial life, microbial cell numbers and activity, individual chemical compounds, and for detection of electroactive microorganisms.13,14 BOD, VFA, and toxicity are highly relevant parameters in the water sector.
2.1 BOD sensors
BOD is a measure of the concentration of biodegradable organic compounds in water. It is an important water quality parameter and wastewater treatment plants typically have strict limits on effluent concentrations. The currently accepted standard method for BOD measurements is cumbersome, has high uncertainty and takes 5 days.15 There is a need for online sensors that are reliable and easy to use. Several types of BOD sensors are described in the literature. These include dissolved oxygen sensors with an immobilized layer of microorganisms between the solution and the oxygen-sensing electrode, bioreactor systems in which the BOD is determined using respirometry, and sensors based on luminescent bacteria, redox mediators, or pH shifts caused by microbial metabolism.16 All these sensors have different drawbacks. Sensors based on immobilized cells on oxygen electrodes typically have a short operational stability of only a few weeks, partly because of growth in the immobilized layer.16,17 Sensors based on luminescence or pH shifts are less studied and have used pure cultures of bacteria,18,19 which limits the applicability for real wastewater having a complex composition of organic matter. Bioreactor-type sensors appear to be the most robust alternative and some systems are commercially available. However, the systems are relatively large, sometimes consisting of several tanks in series.16
Microbial electrochemical cells have several features that make them attractive as BOD sensors. A mixed microbial community with metabolic potential to degrade a wide variety of organics can be enriched on biological anodes. The performance of biological anodes in MFCs has been shown to have long-term stability (>5 years).20 The systems can be small-scale and rapid, online monitoring is possible when the BOD concentration is correlated with the generated current.21 An MFC-type BOD sensor was developed already in the 1970s. The bacterium Clostridium butyricum was immobilized on a platinum anode, which was connected to an aerated carbon cathode. The system produced current which could be correlated to the concentration of glucose–glutamic acid in a solution.22 However, wastewater and natural water contain a mix of organic compounds and therefore a mixed microbial community is needed to provide the metabolic capacity of converting these organics into electrical current. Kim et al.20 developed a two-compartment MFC containing graphite felt electrodes separated by a cation exchange membrane. A microbial community was enriched on the anode, which was fed with wastewater from a starch processing plant. The cathode was fed with an aerated phosphate buffer. The electric charge generated in the batch-fed system was correlated to BOD concentration. The response time varied from 30 min to 10 h for BOD concentrations ranging from 2.6 to 206 mg L−1 and the sensor was in stable operation for over five year.20 A similar sensor with a response time of 45 min for measurements between 80 and 150 mg L−1 BOD was also developed.23 Following these pioneering studies, several researchers have worked to simplify sensor design and improve performance. Instead of using batch-fed systems and measuring electric charge, continuously-fed MFCs in which the magnitude of the current is correlated with BOD concentration were developed.21 This allowed faster response time and changes in BOD concentrations could be detected in a matter of minutes.24 However, such sensors would typically have a smaller measurement range than sensors based on charge measurements because of the Monod-type relationship between BOD concentration and current (Fig. 2). A simplified sensor configuration was tested by Di Lorenzo et al.25 They used a single-chamber MFC with wet-proofed carbon paper coated with platinum as air-cathode. The single-chamber configuration meant that an aerated catholyte no longer had to be fed to the cathode compartment. A sensor operating with potentiostatic control of the cell potential was developed by Modin et al.26 The current and power output of MFCs is often limited by the cathode surface area.27 Thus, potentiostatic control would ensure that the microorganisms converting BOD into current at the anode are able to work at their maximum rate irrespective of the size or performance of the cathode.
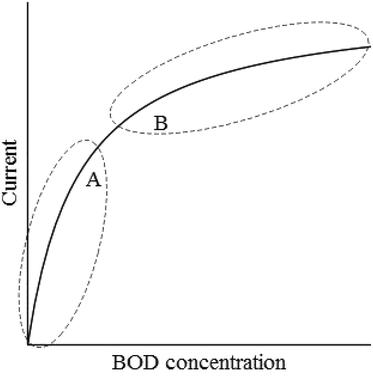 |
| Fig. 2 Conceptual figure showing Monod-type relationship between current and BOD concentration. (A) Region with good linear correlation where current measurements can be used to predict BOD concentration. (B) Saturated region where changes in BOD concentration only have minor effect on current generation. | |
A drawback, which METs share with other BOD sensor technologies, is that the measurement principle is fundamentally different from the conventional BOD5 test. Wastewater effluent quality regulations are based on BOD5, so treatment plants always have to measure this parameter. Thus, although BOD sensors could provide useful information for wastewater treatment plants, they cannot replace conventional BOD5 measurements unless regulations are changed. There is a desire in the water sector to establish alternative standard measurement methods for biodegradable organics.15 Such methods should be reliable and simple to use.
MET-based BOD sensors still suffer from a number of limitations that limit their widespread use. The conversion of organic compounds into electrical current at biological anodes is typically much lower than 100%, especially with real wastewater,28 in which methanogens typically compete with electroactive microorganisms for substrate.29 In MFC-type sensors, intrusion of oxygen from the cathode to the biological anode could also lead to aerobic oxidation of substrate. The consumption of organic compounds by non-electroactive microorganisms can affect the output from a BOD sensor and must therefore be controlled or monitored over time. Sensors should be designed and operated in ways that promote the activities of the electroactive microorganisms and limit the activities of other functional groups. A high anode surface area to volume ratio and hydraulic conditions that flush out suspended growth could be ways to minimize the space available for non-electroactive microorganisms. Furthermore, controlling the anode (or cell) potential instead of simply operating the sensor as an MFC with an external resistor, could be a way of ensuring that the electroactive microorganisms can grow at their maximum rate.26 Operational strategies such as having periods of starvation can also be used to inhibit methanogenesis.29 Operating sensors as MECs with anaerobic cathodes could be a way of preventing problems with oxygen intrusion. However, in that case it should be noted that hydrogen generated at the cathode likely contributes to current generation if it reaches the anode. Another question is if it is possible to store MET-based sensors during periods on non-use.30 Previous research has shown that storage using refrigeration and freezing is possible but can lead to reduced current generation and changes in the microbial community composition on the electrodes.31 Embedding the electroactive microorganisms in a gel matrix on the electrode could also be a way of improving storage of bioelectrodes.30
2.2 VFA sensors
Monitoring VFAs is of particular importance in anaerobic digesters as their accumulation can lead to pH drops and reactor failure. Typically, VFAs are measured offline using e.g. high pressure liquid chromatography or gas chromatography. Online techniques include pH titration, which can give information about the total VFA concentration,32 and headspace gas chromatography, which can detect individual VFAs but requires expensive equipment.33 Microbial electrochemical systems have also been investigated as sensors for VFAs. Acetate is a common substrate in MFC studies and other VFAs have previously also been shown to be converted into electrical current by biological anodes.34,35 Kaur et al.36 enriched MFCs on acetate, propionate, and butyrate. They analyzed the response using cyclic voltammetry and found correlations between peak current and VFA concentration. Interestingly, the MFCs enriched on acetate and propionate only showed electrochemical response when fed with their respective substrate while the MFC enriched on butyrate showed response with all substrates. This suggests that it may be possible to design microbial electrochemical sensors that are specific to acetate or propionate. This is particularly relevant considering that the ratio between propionate and acetate concentration is a key process parameter, whose sudden change may be used as an early-warning indication of anaerobic digester upsets. It should be noted that maintaining electroactive microbial communities specific to acetate or propionate in an anaerobic digester is probably very difficult. Using membranes that protect pre-enriched biofilms from invasion of other species but allow transfer of substrates could be a way of maintaining the substrate-specificity of the sensor. However, it is unlikely that MET-based VFA sensors will be as specific and accurate as gas- or liquid chromatography.
2.3 Toxicity sensors
Methods to rapidly detect toxic substances are important in the water sector. For example, toxic shocks in the influent wastewater can be detrimental to the biological treatment processes at a plant and toxic substances in raw water sources can threaten drinking water supplies. Kim et al.37 used a MFC to detect toxicity in real and synthetic wastewater. The current generation dropped rapidly when the MFC was exposed to an organophosphate insecticide, Pb, Hg, or polychlorinated biphenyl. MFC-based sensors have also been used to detect the toxic response caused by acidity,38 Ni, bentazon, sodium dodecyl sulfate, ferricyanide,39 and formaldehyde.40 Instead of simply looking at the drop in current as an indicator of toxicity, Stein et al.39 fitted polarization curves to kinetic models to analyze the type of inhibition taking place. Patil et al.41 investigated the effect of several toxic substances (two antibiotics, one disinfectant, four metals) on current generation in MFCs. They concluded that the electroactive biofilms were very resistant to toxins at concentrations similar to or a magnitude higher than the typical levels in wastewater. Instead, MFCs based on planktonic cells and anthraquinone-2-sulfonate as soluble redox mediators showed clear reduction in current generation when toxins were added.41
3.
In situ bioremediation
Clean-up of contaminated sites is important to protect groundwater and surface water resources. In Europe, there is an estimated 2.5 million contaminated sites; 340
000 of these are likely highly contaminated and require remediation. Excavation and landfilling has been a common remediation technique.42 However, in situ bioremediation is increasingly being recognized as one of the most effective and sustainable treatment technologies for the cleanup of contaminated subsurface environments (i.e., soils, sediments, and groundwater).43
3.1 Main challenges of conventional approaches
Bioremediation, which relies on the capability of naturally occurring microorganisms to destroy or transform, through enzymatic catalysis, organic and/or inorganic contaminants into harmless (or less dangerous) end products, has so far been successfully applied to treat a wide and ever-increasing variety of contaminants including petroleum hydrocarbons, chlorinated solvents, pesticides, and heavy metals. Unfortunately, under naturally occurring conditions microbial biodegradation processes proceed at low rates, being typically limited by the scarce bioavailability of metabolic electron acceptors, donors, and/or nutrients. To overcome these limitations, current engineered bioremediation approaches typically involve the continual addition of chemical amendments into the subsurface, such as oxygen (or oxygen-releasing chemicals), hydrogen (or hydrogen-releasing chemicals), fermentable organic substrates, and/or nutrients. Although this approach has gained a large consensus worldwide, it suffers from a number of limitations, which have so far markedly challenged the application of in situ bioremediation in the field.44
The main challenges, as highlighted and discussed in a number of recent review papers,45,46 include difficulties (i) to monitor and control subsurface processes, (ii) to efficiently supply substrate and nutrients, and (iii) to stimulate complete degradation pathways. For bioremediation to take place, it is important to maintain intimate contact between the degrading microorganisms, the contaminants, and the supplied substrates. However, it is difficult to monitor the spatial distribution of these components in a subsurface environment. To stimulate degradation processes, it is also important to supply the correct amount of substrate. Currently, this is typically done according to empirical criteria without taking the actual microbial activity into account. Addition of gaseous substrates is challenging because they are poorly water soluble and subsurface gas sparging can lead to undesired stripping of volatile contaminants, explosion hazards, and accumulation of gas bubbles which reduce aquifer permeability. Addition of solid or slow-release organic substrates is also challenging because it typically results in the accumulation of undesired products and in the stimulation of unwanted chemical and biological parasite reactions. Finally, it is difficult to achieve complete biodegradation (and detoxification) of certain types of contaminants (e.g., PCB, chlorinated solvents) or complex mixtures thereof, which require both oxidizing and reducing conditions.
Over the last years, many research efforts have been undertaken to overcome some of these limitations and important progress has certainly been made. Along this line, the application of culture-independent, molecular biology techniques which now allow tracking, in a relatively easy way, the abundance and spatial distribution of key degrading microorganism and/or functional catabolic genes, seem especially promising for augmenting current techniques for assaying biodegradation potential of a given site.47 As an example, a recent study demonstrated how the combined application of three methods namely CARD-FISH (CAtalysed Reporter Deposition Fluorescence In Situ Hybridization), quantitative PCR (qPCR), and Reverse Transcription qPCR (RT-qPCR) to groundwater samples from a chlorinated solvent groundwater plume proved the existence of a well-established dechlorinating microbial community able to use contaminants as substrates for their metabolic activity and indicated the occurrence of reductive dechlorination processes taking place at the site.48
Furthermore, it is also worth noting that the decreasing costs of next-generation sequencing (NGS) now permit a larger scale application of metagenomics and even metatranscriptomics protocols, which enable rapidly scanning of the pollution-associated microbiome for microbes harboring specific degradative capabilities and identifying bioremediation approaches which result in optimal degradative gene expression.49 Additionally, the application of in situ microcosms,50 compound specific isotope analysis (CSIA)51 and other improved site characterization techniques and mathematical models52 will likely contribute improving the reliability and robustness of in situ bioremediation technologies in the future.
3.2 Key advantages of bio-electro-remediation processes
Only very recently, METs started to be considered for (in situ) subsurface bioremediation purposes, a specific application niche which was not examined previously for these systems.53 To a certain extent, this finding is surprising, especially in consideration of the fact that a number of purely electrochemical remediation technologies (e.g., based on electrokinetic mobilization of contaminants), which rely on electrode arrangements and operational conditions similar to those in METs, are already available in the market and proved not only to be effective but also competitive from an economical standpoint.
With reference to remediation applications, METs have been proposed as a platform technology to stimulate both the oxidative degradation of reduced contaminants (e.g., petroleum hydrocarbons, lower chlorinated solvents) and the reductive transformation of oxidized contaminants (e.g., chlorinated solvents, sulfate, nitrate, Cr6+).53 A schematic overview of possible degradation pathways for some of the most relevant subsurface contaminants is depicted in Fig. 3.
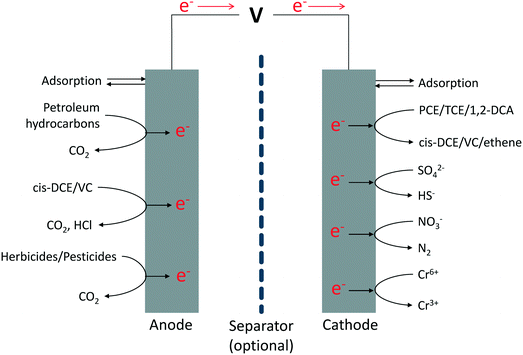 |
| Fig. 3 Schematic representations of possible reaction pathways taking place at cathodes and anodes for some of the most relevant classes of subsurface contaminants. Microorganisms may catalyze the reactions either directly or indirectly. In this latter case, microorganisms exploit redox mediators generated at the electrode(s) (e.g., H2 or O2) or naturally available (e.g., humic acids, sulfur species). | |
Notably, unlike conventional bioremediation approaches that only provide one redox condition, METs can also provide simultaneously (though spatially separated) reducing (at the cathode) and oxidizing (at the anode) conditions, which can be integrated within a single treatment sequence, thus enabling the complete degradation (and detoxification) of contaminants with specific characteristics as well as complex mixtures thereof.
Besides that, the most noticeable feature of METs for in situ bioremediation is that the electrodes, properly deployed within the contaminated matrix (soil, sediment, groundwater) can serve as virtually inexhaustible electron acceptors or donors for contaminant degradation, thus completely eliminating the need for the external addition of chemical amendments.
Another key feature is that, the “energy level” of the electron donor/acceptor can be set to the desired value with a potentiostat (to control individual electrodes' potential) or a power source (to control the potential difference between the anode and cathode), thereby providing a unique tool for increasing the selectivity of the target reaction while minimizing undesired side reactions. In a lab-scale system, this strategy was for instance exploited to minimize the competition for available electrons between dehalorespiring bacteria and methanogens.54 Indeed, when a graphite cathode was polarized at a relatively high potential values (i.e., −250 mV vs. SHE), which prevented electrolytic hydrogen generation, nearly 100% of the electric current flowing in the circuit was channeled to the reductive dechlorination process, while methanogenesis was outcompeted. By contrast, when the cathode was maintained at potentials more negative than −450 mV vs. SHE, which resulted in copious electrolytic hydrogen generation, most of the electric current was ultimately consumed in the competitive process of methane generation.54
It is worth noting that since the electrode(s) may also serve as a support for microbial growth, MET allows easy co-localization of the electron donor/acceptor and the degrading microorganisms. In addition to that, since many organic contaminants (e.g., petroleum hydrocarbons, chlorinated solvents, metals) can be adsorbed on the surface of carbon-based electrodes, their resulting concentration becomes favorably high in a highly reactive zone where also the biocatalysts and the electron donor/acceptor are simultaneously present.
3.3 Direct vs. indirect e-transfer: implications for in situ bioremediation
Extracellular electron transfer (EET) mechanisms play a pivotal role in any MET, including those for bioremediation applications. In general, EET between microbes and electrodes (both serving as cathodes and anodes) can be direct (i.e., through a viable electrically-conductive biofilm laying in contact with the electrode surface) or indirect (i.e. mediated by soluble redox shuttles).55,56 In the case of MET for wastewater treatment direct EET is typically considered to be advantageous and was reported to ensure higher current densities and coulombic efficiencies.57–59 In the case of bioremediation indirect EET may have some advantages over direct EET, particularly due to the fact that redox mediators, such as humic acids, iron and sulfur species, are ubiquitous in subsurface environments.60 As an example, a recent study demonstrated that a poised anode could indirectly accelerate petroleum hydrocarbons oxidation in marine sediments by stimulating the metabolism of sulfate reducing bacteria through the scavenging of toxic sulfide.61 Along this line, a key feature of indirect EET is that the diffusion-based shuttling of the redox mediator allows extending the radius of influence of the electrode and accordingly the size of the biologically reactive zone in the subsurface.
Another key example of indirect EET is the stimulation of reductive and oxidative degradation pathways by H2 and O2, serving as redox shuttles, generated at cathodes and anodes, respectively via water electrolysis. Indeed, in spite of the fact that H2 is the main electron donor in the reductive transformation of a variety of contaminants (e.g., chlorinated solvents, sulfate, nitrate), direct H2 sparging into the groundwater is technically and economically impractical due to the poor efficiency of the process and to safety issues. For this reason, injection of H2-releasing fermentable substrates is most often the preferred approach. Alternatively, to overcome limitations inherent to direct H2 injection, more complicated and expensive delivery systems have been proposed, such as those based on the use of gas-permeable hollow membranes,62 even though their practical and economical viability remains uncertain. By contrast, in situ H2 generation via electrodes may be advantageous due to the possibility to reach very high concentrations (even above saturation) in the proximity of the electrode, to fine-tune (by simply fixing the applied voltage or current) the rate of H2 generation and, by so doing, increase the efficiency of H2 utilization in the desired biodegradation reaction while minimization explosion hazards and the stripping of volatile contaminants. Along this line, in a recent lab-scale study, water electrolysis was successfully exploited to drive the sequential reductive-oxidative dechlorination of a groundwater containing PCE.63 One possible limitation of this approach is the need for using ad hoc electrode materials to drive water electrolysis at sufficiently low applied voltages. Indeed, though intrinsically rather inexpensive, carbon based electrodes are unfortunately characterized by high overpotentials for both hydrogen and oxygen evolution reactions. More specifically, as far as oxygen generation is concerned, titanium electrodes coated with mixed metal oxides (i.e., dimensionally stable anodes, DSA) may represent an interesting, yet more expensive, alternative to carbon-based anodes. One interesting feature of DSA is that the composition of the metal oxide coating can be tailored so as to minimize competing reactions such as chloride oxidation to chorine which, in certain environments (e.g., seawater), may adversely affect process performance. Recently, the long-term viability of electrolysis-driven biodegradation of crude oil in marine sediments has been demonstrated.64 By contrast, exploitation of indirect EET make it more difficult to correlate the electric current with contaminants biodegradation, thus limiting the possibility to use MET also for monitoring and biosensing purposes.
Recent studies have revealed that besides engaging in EET with solid-state electrodes, an ever-increasing number of microbes can also exchange metabolic electrons with neighboring microbes, via a process often referred to as direct interspecies electron transfer (DIET).65 This newly discovered syntrophic partnership, which can also be promoted by electrically conductive minerals (e.g., magnetite) has been identified to play a crucial role in the anaerobic degradation of a variety of organic substrates including ubiquitous soil and groundwater pollutants such as the chlorinated solvents.66,67 Future research efforts aiming at the identification of factors and conditions mostly influencing DIET will also certainly contribute boosting microbe-electrode interactions in current microbial electrochemical systems.
4. Metal removal and recovery
Treatment of metal-containing wastewater is important to prevent environmental contamination, which could threaten raw water sources. It is also desirable to recover valuable metals from wastewater as they are essential raw materials in modern society. Base metals such as aluminum and steel are used in large quantities in e.g. constructions, cars, and airplanes. Copper is used in e.g. wires, electronics, and motors. Special metals such as cobalt, indium, platinum-group metals, and rare-earth elements play important roles in a wide range of appliances. For example, a mobile phone can contain over 40 different elements. New low-carbon technologies such a photovoltaics, batteries, and fuel cells are also heavily reliant on special metals.68
4.1 Metals in wastewater
There are many types of metal-containing wastewater. Industrial wastewater from e.g. electroplating and surface treatment processes, printed circuit board manufacturing, wood processing, inorganic pigment manufacturing, petroleum refining, and photographic operations contain a variety of metals.69 Acid mine drainage, which is generated when sulfide-bearing rocks are exposed to oxygen and water, is a well-known problem in the mining industry and can contain several different metals.70 Metal-containing leachates can also be generated during washing of solid waste incineration fly ash.71
There is a variety of techniques for removing metals from wastewater including chemical precipitation, adsorption, ion-exchange, membrane separation, electrodialysis, and photocatalysis. Electrochemical recovery by reduction of metal ions on a cathode is particularly interesting because of the possibility of recovering specific metals in elemental form. Electrochemical processes are used in the metallurgical industry and for recovery of metals from highly concentrated waste streams. High metal concentrations are typically needed to have sufficient process efficiency and economy.72 However, for recovery of metals from dilute waste streams, MET could potentially be important.
4.2 Microbial electrochemical technologies for metal recovery
Several types of microbial electrochemical reactor configurations have been investigated for metal recovery. The most common configuration consists of a microbial anode where organic compounds or sulfide is oxidized and an abiotic cathode where metal ions are reduced to solid metal (Fig. 4). Such systems combine treatment of organic wastewater at the anode with metal recovery at the cathode. The first reactor was developed for Cu recovery.73 As Cu2+/Cu has a high reduction potential (0.34 V vs. the standard hydrogen electrode, SHE) compared to the normal operating potential of biological anodes (−0.2 to 0 V), the system could be operated as a MFC with simultaneous Cu recovery and generation of electrical energy. High removal efficiencies (>99.88%) were achieved from a 1 g L−1 Cu2+ solution and pure Cu could be obtained on the cathode.73 Several studies have investigated Cu recovery in MFCs and Cu concentrations ranging from about 0.05 to 6 g L−1 have typical been used.74–78 Reactors with biological anodes and abiotic cathodes have also been used to recover Au, Ag, Ni, Pb, Cd, and Zn.79–82 For metals with low reduction potentials (e.g. Ni, Pb, Cd, and Zn), the systems have been operated as MECs with input of electrical energy. In a mixed solution containing Cu, Pb, Cd, and Zn, applied potentials ranging from 0 V to about 1.7 V were used to extract the metals sequentially.82 For recovery of Ni, a voltage of 0.5–1.1 V was applied and removal efficiencies of up to 99 ± 0.6% were achieved from a 50 mg L−1 solution.81
 |
| Fig. 4 Illustration of a microbial electrochemical reactor with a microbial anode oxidizing organics and an abiotic cathode reducing metal ions. | |
For some metals, reduction at the cathode results in the formation of soluble ions or precipitates. A MFC was used to leach Co2+ from lithium cobalt oxides in spent lithium batteries.83 In another study, a MFC was used to reduce VO2+ to VO2+, which is less toxic and can be recovered by precipitation.84 In some cases the cathode has been used to generate an intermediate that reacts with the metal. Cr(VI) was reduced to Cr(III) with H2O2 as the reductant generated by O2 reduction on a cathode in a MFC.85 Hydroxide ion generated by O2 reduction in a MFC was used to precipitate Co(OH)2.86
Microorganisms could also serve as catalysts for metal reduction reactions on cathodes; however, such systems are much less studied than METs with abiotic cathodes. Gregory and Lovley87 showed that the bacterium Geobacter sulfurreducens as well as enrichments from a uranium-contaminated aquifer could reduce U(VI) to U(IV) precipitates using a cathode as electron donor. A microbial cathode has also been used to reduce Cr(VI) to Cr(III) in a MFC.88 The addition of bacteria to electrochemical cells have also been shown to affect the reaction thermodynamics of Fe3+ and AuCl4−.89
5. Other applications
In addition to the three applications of METs that we have highlighted in this paper, there are many other potential applications that are being investigated. Several of these are also highly relevant for the water sector. For example, METs have been investigated for nitrogen (N) and phosphorous (P) recovery from wastewater and urine.90–92 Wastewater treatment plants are typically designed to remove N and P. At the same time, N and P are plant nutrients and needed as fertilizers. Thus, technologies that can both remove and recover N and P from wastewater are desirable. METs could also use organic compounds in the wastewater to power production of chemicals such as H2O2 and alkali.93,94 It may be challenging for such systems to compete with established large-scale production processes when it comes to product purity and cost. However, small-scale systems designed to cover onsite chemical needs would likely have lower requirements for product purity and the installations could be a way for wastewater treatment plants to become more environmentally-friendly.95,96 Microbial desalination cells are systems in which the microbial oxidation of organics at the anode powers electrodialysis.97 Such systems could potentially be used as pretreatment for reverse osmosis systems in the production of potable water from sea water.98
There is a number of possible applications of METs outside the water sector. Several systems have been investigated as power supplies. For example, MFCs have been used to enhance the power output from reverse electrodialysis stacks, which harvest electrical energy from fresh water/sea water gradient.99 Benthic MFCs, in which the anode is buried in the sediments and the cathode is placed in the overlaying water column, could be used to power monitoring equipment in rivers, lakes, and the ocean.100 In the plant-MFC, the anode is placed in the soil. Organic compounds excreted by the root of plants (rhizodeposits) are converted into electric power by the MFC.101 Another application of microbial anodes buried in soils or sediments could be to mitigate methane emissions from such environments.102
Recently, many researchers focus on using METs for production of chemicals. Microbial electrosynthesis103 refers to the reduction of low-value carbon (e.g. carbon dioxide) to high-value chemicals on biological cathodes. Carbon dioxide has been reduced to methane, acetate, ethanol and butyrate.103–105 Reduction of acetate to ethanol106 and chain elongation of acetate to caproate have also been achieved.107 Electro-fermentation refers to the use of electrochemical cells to drive fermentation processes.108,109 Electrodes can influence microbial metabolic pathways by creating either more oxidizing (anode) or reducing (cathode) conditions. For example, Zhou et al.110 improved 1,3-propanediol production from glycerol by a mixed culture using a biocathode poised at −0.9 V vs. SHE. In anaerobic digestion, the presence of a poised cathode has been shown to improve methane production.111 The electrode may both have served as a growth support material and as electron donor for the microorganisms in the reactor. Electrochemical cells can also be used to extract products. Andersen et al.112 used an electrochemical cell operated with controlled current and an anion exchange membrane to extract VFAs from a fermentation process. The H2 generated at the cathode also steered the fermentation towards production of more reduced products. Electro-fermentation was recently reviewed by Schievano et al.109
6. Outlook
METs have a wide range of potential applications in the water sector and in other fields. However, several technical challenges must be overcome and it is not clear which of the technologies can become economically and technically viable in full-scale. In this paper we have highlighted three technologies that appear promising for practical application.
Sensors
Using microbial electrochemical reactors as BOD sensors was one of the first proposed applications when the research field took off in Korea about 15 years ago20 and a MFC-based sensor has been commercialized.113 Sensors can be small and do not suffer from the scale-up challenges that plague many other applications of microbial electrochemistry. Installation of sensors would be a very small capital investment. The possibility of online BOD measurements is highly attractive to wastewater treatment operators. Currently, there is no widely accepted alternative to the conventional 5 day test. Thus, there is need that microbial electrochemical sensors could fulfill. Moreover, a BOD sensor would contribute to the main goal of wastewater treatment – to meet discharge limits. In addition to monitoring influent and effluent BOD from a plant, it could potentially be used for control of the activated sludge process. In modern activated sludge systems, several operational parameters such as aeration rates in different zones of the tank and solids retention time can be controlled in real-time. The information provided by an online BOD sensor could together with other parameters be used to steer the process towards optimized energy efficiency and treatment performance. However, for BOD sensors to gain widespread acceptance, a sensor design that is simple to use and minimizes problems with competition for organic compounds between electroactive microorganisms and other functional groups should be developed. Microbial electrochemical sensors could also be used to monitor VFA accumulation in anaerobic digesters and toxicity in water. The toxicity application may be the most challenging because of the resistance of electroactive biofilm to toxins in low concentrations.41
In situ bioremediation
Application of METs in the field of subsurface remediation is recently catalyzing considerable attention and several laboratory-scale studies have demonstrated its viability for stimulating the oxidative and/or reductive transformation of harmful (soil/sediment and groundwater) contaminants. Overall, the great promise of MET is to allow increasing control over biodegradation processes taking place in the subsurface while ensuring a sustainable bioremediation strategy. Along this line, electrical power, possibly generated on-site from renewable sources (e.g., using solar panels) would be virtually the only “reagent” needed to drive the bioremediation process and the main operational cost. Clearly, electric power (P) consumption depends on the electric current flowing in the circuit (I) and the voltage applied between the electrodes (P = I × V). In general, in the context of subsurface remediation, the electrical current needed to ensure high contaminant degradation efficiencies can be relatively low (in the range of few A per m2 electrode surface) due to the fact that the contamination levels are also typically low (from μg L−1 to mg L−1); as far as the voltage is concerned, values up to a few volts per centimeter are commonly applied in full-scale electrokinetic remediation systems.114,115 Taken as a whole it is anticipated that existing design and operation protocols developed for purely electrochemical remediation technologies, which already proved to be technically and economically sustainable and effective, will possibly contribute driving the transition of MET-based remediation from the laboratory to the market. Extensive field-testing is required in the coming year to verify the actual long-term viability of the proposed approaches under highly representative environmental conditions.
Metal removal and recovery
A major advantage of using METs for recovery of metals compared to e.g. generation of energy is the high economic value of the product. A simple calculation shows that a MFC recovering copper at a cell potential of 0 V would generate about 80 times higher economic value than a MFC producing electrical energy at a cell potential of 0.5 V (assuming a copper price of 3.5 € per kg and an electricity price of 0.1 € per kW h). There is a wide range of aqueous streams containing metals and treatment is necessary to prevent environmental contamination. Furthermore, considering the importance of metals in society and that mining is dominated by only a few countries, new technologies for recovery of metals from secondary sources are becoming increasingly important.116 Here, microbial electrochemistry could meet a large societal need. Thus far, most studies have used microbial anodes to power metal reduction at abiotic cathodes. If we compare such a system with conventional abiotic electrolysis, the advantages of the microbial system include low energy consumption for metal recovery, the use of low-cost anodes (as opposed to dimensionally-stable anodes), and the possibility to selectively extract copper and other metals from a complex metal mix.82 However, there are also drawbacks with the microbial system. A suitable organics-containing anode feed should be readily available, a membrane will likely be needed to keep anode- and cathode feeds separate and prevent inhibition of the electroactive microorganisms, and the current density will be lower compared to an abiotic system, which will result in lower metal recovery rates and/or larger systems. In a study on abiotic electrochemical recovery of Cu from industrial wastewater, current densities of 23–70 A m−2 were used.117 These current densities could be delivered by biological anodes under optimal conditions118 suggesting that a METs could be competitive when compared with abiotic electrolysis for Cu recovery, especially considering that a MET can recover Cu without electrical energy consumption while >7 kW h kg−1 Cu was required in the abiotic system.117 It is still unclear for which waste streams and metals a microbial electrochemical system will be a better choice than an abiotic system or other techniques such as precipitation and adsorption. It will probably be highly site-specific. The use of microorganisms to catalyze metal transformations in electrochemical reactors has still received relatively little attention, except for detoxification of water.87,88 However, microorganisms can also reduce metal ions to nanoparticles, which are valuable in a wide range of technologies.119 Microbial electrochemistry could potentially be used for fine-tuning the redox potential of microbial cells and steer the production of nanoparticles with certain characteristics.
In conclusion, microbial electrochemistry is a platform technology that offers a myriad of potential applications. Sensors, in situ bioremediation, and microbial electrometallurgy are three applications that could be of particular importance for the water sector. The BOD sensor is striking as an application with high potential for widespread practical application in the short term. It does not require high capital costs, does not suffer from tough competitions from other technologies, has been demonstrated in several scientific studies, and meets a need in the water sector.
Acknowledgements
O. M. was funded by the Swedish Research Council (VR, 2012-5167) and a Marie Curie career integration grant (bioanode). F. A. acknowledges MIUR (ERANETMED Project WE-MET) for financial support.
References
- B. E. Logan, H. Hamelers, R. Rozendal, U. Schröder, J. Keller, S. Freguia, P. Aelterman, W. Verstraete and K. Rabaey, Environ. Sci. Technol., 2006, 40, 5181–5192 CrossRef CAS PubMed.
- A. Escapa, R. Mateos, E. J. Martínez and J. Blanes, Renewable Sustainable Energy Rev., 2016, 55, 942–956 CrossRef CAS.
- A. Escapa, X. Gómez, B. Tartakovsky and A. Morán, Int. J. Hydrogen Energy, 2012, 37, 18641–18653 CrossRef CAS.
- O. Modin and D. J. Gustavsson, Water Sci. Technol., 2014, 69, 1359–1372 CrossRef CAS PubMed.
- Y. Fan, S.-K. Han and H. Liu, Energy Environ. Sci., 2012, 5, 8273–8280 CAS.
- A. Escapa, M. I. San-Martin, R. Mateos and A. Moran, Bioresour. Technol., 2015, 180, 72–78 CrossRef CAS PubMed.
- R. A. Rozendal, H. V. Hamelers, K. Rabaey, J. Keller and C. J. Buisman, Trends Biotechnol., 2008, 26, 450–459 CrossRef CAS PubMed.
- A. K. Marcus, C. I. Torres and B. E. Rittmann, Bioresour. Technol., 2011, 102, 253–262 CrossRef CAS PubMed.
- W.-F. Liu and S.-A. Cheng, J. Zhejiang Univ., Sci., A, 2014, 15, 841–861 CrossRef CAS.
- S. Freguia, K. Rabaey, Z. Yuan and J. Keller, Environ. Sci. Technol., 2008, 42, 7937–7943 CrossRef CAS PubMed.
- P. Parameswaran, C. I. Torres, H. S. Lee, R. Krajmalnik-Brown and B. E. Rittmann, Biotechnol. Bioeng., 2009, 103, 513–523 CrossRef CAS PubMed.
- W. He, M. J. Wallack, K. Y. Kim, X. Zhang, W. Yang, X. Zhu, Y. Feng and B. E. Logan, Water Res., 2016, 105, 351–360 CrossRef CAS PubMed.
- X. C. Abrevaya, N. J. Sacco, M. C. Bonetto, A. Hilding-Ohlsson and E. Corton, Biosens. Bioelectron., 2015, 63, 591–601 CrossRef CAS PubMed.
- H. Yang, M. Zhou, M. Liu, W. Yang and T. Gu, Biotechnol. Lett., 2015, 37, 2357–2364 CrossRef CAS PubMed.
-
G. Tchobanoglous, F. L. Burton, H. D. Stensel and Metcalf & Eddy Inc., Wastewater Engineering, Treatment and Reuse 4th Ed., McGraw-Hill, New York, USA, 4th edn, 2004 Search PubMed.
- S. Jouanneau, L. Recoules, M. J. Durand, A. Boukabache, V. Picot, Y. Primault, A. Lakel, M. Sengelin, B. Barillon and G. Thouand, Water Res., 2014, 49, 62–82 CrossRef CAS PubMed.
- J. Liu and B. Mattiasson, Water Res., 2002, 36, 3786–3802 CrossRef CAS PubMed.
- T. Sakaguchi, K. Kitagawa, T. Ando, Y. Murakami, Y. Morita, A. Yamamura, K. Yokoyama and E. Tamiya, Biosens. Bioelectron., 2003, 19, 115–121 CrossRef CAS PubMed.
- Y. Murakami, T. Kikuchi, A. Yamamura, T. Sakaguchi, K. Yokoyama, Y. Ito, M. Takiue, H. Uchida, T. Katsube and E. Tamiya, Sens. Actuators, B, 1998, 53, 163–172 CrossRef CAS.
- B. H. Kim, I. S. Chang, G. C. Gil, H. S. Park and H. J. Kim, Biotechnol. Lett., 2003, 25, 541–545 CrossRef CAS PubMed.
- I. S. Chang, J. K. Jang, G. C. Gil, M. Kim, H. J. Kim, B. W. Cho and B. H. Kim, Biosens. Bioelectron., 2004, 19, 607–613 CrossRef CAS PubMed.
- I. Karube, T. Matsunaga, S. Mitsuda and S. Suzuki, Biotechnol. Bioeng., 1977, 19, 1535–1547 CrossRef CAS PubMed.
- M. Kim, S. M. Youn, S. H. Shin, J. G. Jang, S. H. Han, M. S. Hyun, G. M. Gadd and H. J. Kim, J. Environ. Monit., 2003, 5, 640–643 RSC.
- H. Moon, I. S. Chang, K. H. Kang, J. K. Jang and B. H. Kim, Biotechnol. Lett., 2004, 26, 1717–1721 CrossRef CAS PubMed.
- M. Di Lorenzo, T. P. Curtis, I. M. Head, S. B. Velasquez-Orta, S. B. Velasquez-Orta and K. Scott, Water Sci. Technol., 2009, 60, 2879–2887 CrossRef CAS PubMed.
- O. Modin and B.-M. Wilén, Water Res., 2012, 46, 6113–6120 CrossRef CAS PubMed.
- B. E. Logan, M. J. Wallack, K.-Y. Kim, W. He, Y. Feng and P. E. Saikaly, Environ. Sci. Technol. Lett., 2015, 2, 206–214 CrossRef CAS.
- Y. Ahn and B. E. Logan, Bioresour. Technol., 2010, 101, 469–475 CrossRef CAS PubMed.
- A. Kaur, H. C. Boghani, I. Michie, R. M. Dinsdale, A. J. Guwy and G. C. Premier, Bioresour. Technol., 2014, 173, 75–81 CrossRef CAS PubMed.
- J. B. A. Arends, Microb. Biotechnol., 2017 DOI:10.1111/1751-7915.12590.
- S. Saheb Alam, F. Persson, B.-M. Wilén, M. Hermansson and O. Modin, Sci. Rep., 2015, 5, 18433 CrossRef CAS PubMed.
- H. Feitkenhauer, J. von Sachs and U. Meyer, Water Res., 2002, 36, 212–218 CrossRef CAS PubMed.
- K. Boe, D. J. Batstone and I. Angelidaki, Biotechnol. Bioeng., 2007, 96, 712–721 CrossRef CAS PubMed.
- S. Freguia, E. H. Teh, N. Boon, K. M. Leung, J. Keller and K. Rabaey, Bioresour. Technol., 2010, 101, 1233–1238 CrossRef CAS PubMed.
- H. Liu, S. Cheng and B. E. Logan, Environ. Sci. Technol., 2005, 39, 658–662 CrossRef CAS PubMed.
- A. Kaur, J. R. Kim, I. Michie, R. M. Dinsdale, A. J. Guwy and G. C. Premier, Biosens. Bioelectron., 2013, 47, 50–55 CrossRef CAS PubMed.
- M. Kim, M. Sik Hyun, G. M. Gadd and H. Joo Kim, J. Environ. Monit., 2007, 9, 1323–1328 RSC.
- Y. J. Shen, O. Lefebvre, Z. Tan and H. Y. Ng, Water Sci. Technol., 2012, 65, 1223–1228 CrossRef CAS PubMed.
- N. E. Stein, H. V. Hamelers, G. van Straten and K. J. Keesman, Biosensors, 2012, 2, 255–268 CrossRef CAS PubMed.
- D. Davila, J. P. Esquivel, N. Sabate and J. Mas, Biosens. Bioelectron., 2011, 26, 2426–2430 CrossRef CAS PubMed.
- S. Patil, F. Harnisch and U. Schroder, ChemPhysChem, 2010, 11, 2834–2837 CrossRef CAS PubMed.
-
EEA, Progress in Management of Contaminated Sites, European Environment Agency, Copenhagen, Denmark, 2014 Search PubMed.
- K. Watanabe, Curr. Opin.
Biotechnol., 2001, 12, 237–241 CrossRef CAS PubMed.
- M. Majone, R. Verdini, F. Aulenta, S. Rossetti, V. Tandoi, N. Kalogerakis, S. Agathos, S. Puig, G. Zanaroli and F. Fava, New Biotechnol., 2015, 32, 133–146 CrossRef CAS PubMed.
- J.-L. Ramos, S. Marqués, P. van Dillewijn, M. Espinosa-Urgel, A. Segura, E. Duque, T. Krell, M.-I. Ramos-González, S. Bursakov, A. Roca, J. Solano, M. Fernádez, J. L. Niqui, P. Pizarro-Tobias and R.-M. Wittich, Trends Biotechnol., 2011, 29, 641–647 CrossRef CAS PubMed.
- M. Megharaj, B. Ramakrishnan, K. Venkateswarlu, N. Sethunathan and R. Naidu, Environ. Int., 2011, 37, 1362–1375 CrossRef CAS PubMed.
- D. R. Lovley, Nat. Rev. Microbiol., 2003, 1, 35–44 CrossRef CAS PubMed.
- B. Matturro, F. Aulenta, M. Majone, M. P. Papini, V. Tandoi and S. Rossetti, New Biotechnol., 2012, 30, 23–32 CrossRef CAS PubMed.
- L. M. Czaplicki and C. K. Gunsch, J. Environ. Eng., 2016, 142(10) DOI:10.1061/(ASCE)EE.1943-7870.0001141.
- C. Schurig, C. W. Mueller, C. Hoschen, A. Prager, E. Kothe, H. Beck, A. Miltner and M. Kastner, Appl. Microbiol. Biotechnol., 2015, 99, 957–968 CrossRef CAS PubMed.
- M. Elsner, M. A. Jochmann, T. B. Hofstetter, D. Hunkeler, A. Bernstein, T. C. Schmidt and A. Schimmelmann, Anal. Bioanal. Chem., 2012, 403, 2471–2491 CrossRef CAS PubMed.
- R. U. Meckenstock, M. Elsner, C. Griebler, T. Lueders, C. Stumpp, J. Aamand, S. N. Agathos, H. J. Albrechtsen, L. Bastiaens, P. L. Bjerg, N. Boon, W. Dejonghe, W. E. Huang, S. I. Schmidt, E. Smolders, S. R. Sorensen, D. Springael and B. M. van Breukelen, Environ. Sci. Technol., 2015, 49, 7073–7081 CrossRef CAS PubMed.
- H. Wang, H. Luo, P. H. Fallgren, S. Jin and Z. J. Ren, Biotechnol. Adv., 2015, 33, 317–334 CrossRef CAS PubMed.
- F. Aulenta, L. Tocca, R. Verdini, P. Reale and M. Majone, Environ. Sci. Technol., 2011, 45, 8444–8451 CrossRef CAS PubMed.
- D. R. Lovley, Geobiology, 2008, 6, 225–231 CrossRef CAS PubMed.
- M. Rosenbaum, F. Aulenta, M. Villano and L. T. Angenent, Bioresour. Technol., 2011, 102, 324–333 CrossRef CAS PubMed.
- D. R. Lovley, Curr. Opin. Biotechnol., 2008, 19, 564–571 CrossRef CAS PubMed.
- D. R. Lovley, Curr. Opin. Biotechnol., 2006, 17, 327–332 CrossRef CAS PubMed.
- L. Lu and Z. Y. J. Ren, Bioresour. Technol., 2016, 215, 254–264 CrossRef CAS PubMed.
- L. Klupfel, A. Piepenbrock, A. Kappler and M. Sander, Nat. Geosci., 2014, 7, 195–200 CrossRef.
- M. Daghio, E. Vaiopoulou, S. A. Patil, A. Suarez-Suarez, I. M. Head, A. Franzetti and K. Rabaey, Appl. Environ. Microbiol., 2016, 82, 297–307 CrossRef CAS PubMed.
- X. Ma, P. J. Novak, M. J. Semmens, L. W. Clapp and R. M. Hozalski, Water Res., 2006, 40, 1155–1166 CrossRef CAS PubMed.
- S. T. Lohner, D. Becker, K. M. Mangold and A. Tiehm, Environ. Sci. Technol., 2011, 45, 6491–6497 CrossRef CAS PubMed.
- M. Bellagamba, C. Cruz Viggi, N. Ademollo, S. Rossetti and F. Aulenta, New Biotechnol., 2016 DOI:10.1016/j.nbt.2016.03.003.
- Z. M. Summers, H. E. Fogarty, C. Leang, A. E. Franks, N. S. Malvankar and D. R. Lovley, Science, 2010, 330, 1413–1415 CrossRef CAS PubMed.
- F. Aulenta, S. Fazi, M. Majone and S. Rossetti, Process Biochem., 2014, 49, 2235–2240 CrossRef CAS.
- F. Aulenta, S. Rossetti, S. Amalfitano, M. Majone and V. Tandoi, ChemSusChem, 2013, 6, 433–436 CrossRef CAS PubMed.
-
M. A. Reuter, C. Hudson, A. van Shaik, K. Heiskanen, C. Meskers and C. Hagelüken, Metal recycling: Opportunities, limits, infrastructure, UNEP, 2013 Search PubMed.
- M. A. Barakat, Arabian J. Chem., 2011, 4, 361–377 CrossRef CAS.
- A. Akcil and S. Koldas, J. Cleaner Prod., 2006, 14, 1139–1145 CrossRef.
- B. Van der Bruggen, G. Vogels, P. Van Herck and C. Vandecasteele, J. Hazard. Mater., 1998, 57, 127–144 CrossRef CAS.
-
L. Figueroa and C. Wolkersdorfer, Electrochemical recovery of metals in mining influenced water: state of the art, An interdisciplinary response to mine water challenges – Proceedings of the 12th IMWA conference, China University of Mining and Technology Press, 2014, ISBN: 978-7-5646-2437-8 Search PubMed.
- A. ter Heijne, F. Liu, R. van der Weijden, J. Weijma, C. J. N. Buisman and H. V. M. Hamelers, Environ. Sci. Technol., 2010, 44, 4376–4381 CrossRef CAS PubMed.
- H.-C. Tao, M. Liang, W. Li, L.-J. Zhang, J.-R. Ni and W.-M. Wu, J. Hazard. Mater., 2011, 189, 186–192 CrossRef CAS PubMed.
- Z. An, H. Zhang, Q. Wen, Z. Chen and M. Du, Desalination, 2014, 346, 115–121 CrossRef CAS.
- P. Rodenas Motos, A. Ter Heijne, R. van der Weijden, M. Saakes, C. J. Buisman and T. H. Sleutels, Front. Microbiol., 2015, 6, 527 Search PubMed.
- S. A. Cheng, B. S. Wang and Y. H. Wang, Bioresour. Technol., 2013, 147, 332–337 CrossRef CAS PubMed.
- D. Wu, L. Huang, X. Quan and G. Li Puma, J. Power Sources, 2016, 307, 705–714 CrossRef CAS.
- C. Choi and N. Hu, Bioresour. Technol., 2013, 133, 589–598 CrossRef CAS PubMed.
- C. Choi and Y. Cui, Bioresour. Technol., 2012, 107, 522–525 CrossRef CAS PubMed.
- B. Qin, H. Luo, G. Liu, R. Zhang, S. Chen, Y. Hou and Y. Luo, Bioresour. Technol., 2012, 121, 458–461 CrossRef CAS PubMed.
- O. Modin, X. Wang, X. Wu, S. Rauch and K. K. Fedje, J. Hazard. Mater., 2012, 235–236, 291–297 CrossRef CAS PubMed.
- L. Huang, T. Li, C. Liu, X. Quan, L. Chen, A. Wang and G. Chen, Bioresour. Technol., 2013, 128, 539–546 CrossRef CAS PubMed.
- B. Zhang, H. Zhao, C. Shi, S. Zhou and J. Ni, J. Chem. Technol. Biotechnol., 2009, 84, 1780–1786 CrossRef CAS.
- L. Liu, Y. Yuan, F. B. Li and C. H. Feng, Bioresour. Technol., 2011, 102, 2468–2473 CrossRef CAS PubMed.
- L. Huang, Y. Liu, L. Yu, X. Quan and G. Chen, J. Cleaner Prod., 2015, 86, 441–446 CrossRef CAS.
- K. B. Gregory and D. R. Lovley, Environ. Sci. Technol., 2005, 39, 8943–8947 CrossRef CAS PubMed.
- M. Tandukar, S. J. Huber, T. Onodera and S. G. Pavlostathis, Environ. Sci. Technol., 2009, 43, 8159–8165 CrossRef CAS PubMed.
- J. Varia, A. Zegeye, S. Roy, S. Yahaya and S. Bull, Biochem. Eng. J., 2014, 85, 101–109 CrossRef CAS.
- X. Wu and O. Modin, Bioresour. Technol., 2013, 146, 530–536 CrossRef CAS PubMed.
- P. Kuntke, K. M. Smiech, H. Bruning, G. Zeeman, M. Saakes, T. H. Sleutels, H. V. Hamelers and C. J. Buisman, Water Res., 2012, 46, 2627–2636 CrossRef CAS PubMed.
- R. D. Cusick and B. E. Logan, Bioresour. Technol., 2012, 107, 110–115 CrossRef CAS PubMed.
- R. A. Rozendal, E. Leone, J. Keller and K. Rabaey, Electrochem. Commun., 2009, 11, 1752–1755 CrossRef CAS.
- K. Rabaey, S. Butzer, S. Brown, J. Keller and R. A. Rozendal, Environ. Sci. Technol., 2010, 44, 4315–4321 CrossRef CAS PubMed.
- O. Modin and K. Fukushi, Environ. Technol., 2013, 34, 2737–2742 CrossRef CAS PubMed.
- O. Modin, K. Fukushi, K. Rabaey, R. A. Rozendal and K. Yamamoto, Water Res., 2011, 45, 2691–2699 CrossRef CAS PubMed.
- X. Cao, X. Huang, P. Liang, K. Xiao, Y. Zhou, X. Zhang and B. E. Logan, Environ. Sci. Technol., 2009, 43, 7148–7152 CrossRef CAS PubMed.
- M. Mehanna, T. Saito, J. Yan, M. Hickner, X. Cao, X. Huang and B. E. Logan, Energy Environ. Sci., 2010, 3, 1114–1120 CAS.
- Y. Kim and B. E. Logan, Environ. Sci. Technol., 2011, 45, 5834–5839 CrossRef CAS PubMed.
- L. M. Tender, S. A. Gray, E. Groveman, D. A. Lowy, P. Kauffman, J. Melhado, R. C. Tyce, D. Flynn, R. Petrecca and J. Dobarro, J. Power Sources, 2008, 179, 571–575 CrossRef CAS.
- D. P. B. T. B. Strik, H. V. M. Hamelers, J. F. H. Snel and C. J. N. Buisman, Int. J. Energy Res., 2008, 32, 870–876 CrossRef CAS.
- E. S. Friedman, L. E. McPhillips, J. J. Werner, A. C. Poole, R. E. Ley, M. T. Walter and L. T. Angenent, Front. Microbiol., 2015, 6, 1523 Search PubMed.
- K. P. Nevin, T. L. Woodard, A. E. Franks, Z. M. Summers and D. R. Lovley, mBio, 2010, 1, 1–4 CrossRef PubMed.
- S. Cheng, D. Xing, D. F. Call and B. E. Logan, Environ. Sci. Technol., 2009, 43, 3953–3958 CrossRef CAS PubMed.
- S. Bajracharya, K. Vanbroekhoven, C. J. Buisman, D. Pant and D. P. Strik, Environ. Sci. Pollut. Res., 2016, 23(22), 22292–22308 CrossRef CAS PubMed.
- K. J. J. Steinbusch, H. V. M. Hamelers, J. D. Schaap, C. Kampman and C. J. N. Buisman, Environ. Sci. Technol., 2010, 44, 513–517 CrossRef CAS PubMed.
- M. C. A. A. Van Eerten-Jansen, A. Ter Heijne, T. I. M. Grootscholten, K. J. J. Steinbusch, T. H. J. A. Sleutels, H. V. M. Hamelers and C. J. N. Buisman, ACS Sustainable Chem. Eng., 2013, 1, 513–518 CrossRef CAS.
- K. Rabaey and R. A. Rozendal, Nat. Rev. Microbiol., 2010, 8, 706–716 CrossRef CAS PubMed.
- A. Schievano, T. Pepe Sciarria, K. Vanbroekhoven, H. De Wever, S. Puig, S. J. Andersen, K. Rabaey and D. Pant, Trends Biotechnol., 2016, 34, 866–878 CrossRef CAS PubMed.
- M. Zhou, J. Chen, S. Freguia, K. Rabaey and J. Keller, Environ. Sci. Technol., 2013, 47, 11199–11205 CrossRef CAS PubMed.
- K. Sasaki, D. Sasaki, M. Morita, S. Hirano, N. Matsumoto, N. Ohmura and Y. Igarashi, Bioresour. Technol., 2010, 101, 3415–3422 CrossRef CAS PubMed.
- S. J. Andersen, P. Candry, T. Basadre, W. C. Khor, H. Roume, E. Hernandez-Sanabria, M. Coma and K. Rabaey, Biotechnol. Biofuels, 2015, 8, 221 CrossRef PubMed.
- Korbi, BOD Analyzer: HABS series, http://korbi.en.ecplaza.net/bod-analyzer-habs-series--34392-157398.html, 2016).
- S. V. Ho, C. Athmer, P. W. Sheridan, B. M. Hughes, R. Orth, D. McKenzie, P. H. Brodsky, A. M. Shapiro, T. M. Sivavec, J. Salvo, D. Schultz, R. Landis, R. Griffith and S. Shoemaker, Environ. Sci. Technol., 1999, 33, 1092–1099 CrossRef CAS.
- S. V. Ho, P. W. Sheridan, C. J. Athmer, M. A. Heitkamp, J. M. Brackin, D. Weber and P. H. Brodsky, Environ. Sci. Technol., 1995, 29, 2528–2534 CrossRef CAS PubMed.
- A. Bloodworth, Nature, 2014, 505, 19–20 CrossRef PubMed.
- T.-C. Chen, R. Priambodo, R.-L. Huang and Y.-H. Huang, J. Waste Manage., 2013, 2013, 1–6 CrossRef.
- S. Chen, H. Hou, F. Harnisch, S. A. Patil, A. A. Carmona-Martinez, S. Agarwal, Y. Zhang, S. Sinha-Ray, A. L. Yarin, A. Greiner and U. Schröder, Energy Environ. Sci., 2011, 4, 1417–1421 CAS.
- K. B. Narayanan and N. Sakthivel, Adv. Colloid Interface Sci., 2010, 156, 1–13 CrossRef CAS PubMed.
|
This journal is © The Royal Society of Chemistry 2017 |