DOI:
10.1039/C5RA15261E
(Paper)
RSC Adv., 2015,
5, 78215-78228
Glycosylation improves the functional characteristics of chlorogenic acid–lactoferrin conjugate
Received
31st July 2015
, Accepted 7th September 2015
First published on 8th September 2015
Abstract
In the present study, a chlorogenic acid (CA)–lactoferrin (LF) conjugate prepared via alkali treatment was glycoslated with glucose (Glc) or polydextrose (PD) by the Maillard reaction. Formation of the covalent CA–LF–Glc/PD ternary conjugates was confirmed by matrix-assisted laser desorption/ionization time-of-flight mass spectrometry (MALDI-TOF-MS), Fourier transform infrared (FTIR) spectroscopy, differential scanning calorimetry (DSC) and fluorescence analyses. The results showed that the grafting ratio between the CA–LF conjugate and Glc/PD was 40.50% for the CA–LF–Glc ternary conjugate and 11.72% for the CA–LF–PD ternary conjugate. Conjugating CA and Glc/PD onto LF changed the conformation of the protein, leading to a reduction in the α-helix content and an increase in the unordered structure. The thermal stability of the CA–LF conjugate was remarkably improved by the Maillard-type conjugation. According to AFM and DLS results, the ternary conjugates showed coarser structures and bigger particle sizes than their mixtures. The reducing power was increased from 206.91 μmol Trolox g−1 of CA–LF conjugate to 255.76 and 273.25 μmol Trolox g−1 sample, respectively, in CA–LF–Glc and CA–LF–PD ternary conjugates, indicating the glycosylation was an effective way to improve the antioxidant activity of the CA–LF conjugate. Moreover, the ternary conjugates were employed to encapsulate β-carotene as a model biologically active macromolecule, and they could enhance the physicochemical stability of β-carotene emulsions. This work presented a simple and general approach to the preparation of polyphenol–protein–polysaccharide conjugates that could be potentially employed as food-grade biomacromolecules in the food and pharmaceutical industries.
Introduction
Proteins are widely utilized to enhance functional properties of food products, such as emulsification, gelling and foaming attributes.1 However, the applications of food proteins are still limited, because they are generally unstable against heating, organic solvents and proteolytic attack.2 If proteins can be transformed into stable forms, their applications will be prominently broadened in the food industry.
Various protein modification technologies were extensively investigated, there were considerable evidences to suggest that covalent bonding of proteins with polysaccharides and/or polyphenols is an effective approach to overcome their instability under the unfavourable conditions and even induce a beneficial synergistic effect on the functional properties of the proteins.1–3 Besides, conjugated proteins can be applied in the encapsulation and delivery system to provide an effective protection for bioactive compounds against thermo- or photodegradation and maximize the delivery efficiency.4,5
In recent years, the conjugation of proteins with polysaccharides through spontaneous Maillard reaction has attracted significant attention. Maillard reaction is a promising method because it can alter the functionality of proteins without the presence of chemical reagents.1,6 In the Maillard reaction, a protein and a polysaccharide are linked through a covalent bond between the amino group of the protein, especially the ε-amino group of lysine residues and the terminal reducing group of the carbonyl of the polysaccharide.1,7 The formation of protein–polysaccharide conjugates by controlled dry heating seems to be the most effective method for the production of emulsifiers for food products.2,8 Maillard reaction significantly increases food protein solubility, heat stability, emulsifying property, foaming property, antioxidant activity and antimicrobial property.1,9–11 On the other hand, the conjugates formed by glycosylation also have potential applications in controlled release systems and nanoencapsulation.12,13 Maillard-type protein–polysaccharide conjugates, which are natural, non-toxic and have improved functional properties, could be predictable to have extensive potential for application in the food and health industries.14
Phenolic compounds represent the largest group of secondary plant metabolites and are important food components with a large range of structures and functions.15 Naturally occurring polyphenols are known to have numerous biological activities, in particular, they have recently received attention as antioxidants. Conjugating antioxidant molecules with proteins forms new functionalized materials combined the properties of both molecules. In recent investigations it has been shown that binding phenolic compounds to proteins leads to significant improvement in physicochemical properties and activities of proteins.15,16 Phenolic compounds can covalently react with proteins in the form of a phenolic radical or quinone via enzymatic or non-enzymatic oxidation.17–19 The non-enzymatic oxidation method has been applied extensively, and the results showed that phenolic oxidation products generated under alkaline condition could react with amino groups, thiol groups and tryptophan residues of the protein.20 As a consequence, the modification induced the cross-linking of proteins and phenolic compounds and altered the physicochemical properties of proteins, such as the solubility, surface hydrophobicity, and emulsification, in addition, the covalent conjugation delivered proteins with enhanced antioxidant activity.21,22
However, to the best of our knowledge, there is no information so far concerning the characteristics of the polyphenol–protein–carbohydrate covalent complex. Based on the studies mentioned above, the ternary conjugation of protein, carbohydrate and polyphenol might be an attractive method to develop new food grade materials with better physicochemical properties by drawing advantages of these three components. In addition, the covalent reaction between carbohydrate and protein–polyphenol conjugate might be an encouraging way for the functionality modification of the biological macromolecule.
This work reports a rapid and eco-friendly procedure for the covalent complexing of polyphenol–protein molecules and glucose (Glc)/polydextrose (PD) by controlling pH and dry-heating process. Lactoferrin (LF), a mammalian cationic iron-binding glycoprotein belonging to the transferrin family, is raising great interest in food and medicinal researches owing to its health benefits.23,24 Because of its biodegradability and biocompatibility in physiological environment, LF was chosen as a polymer backbone to be functionalized with chlorogenic acid (CA) and Glc/PD to obtain new biomacromolecules. The objective of this study is to (i) create and characterize ternary conjugates obtained via Maillard reaction between CA–LF conjugate and Glc as well as PD, respectively; (ii) evaluate the thermal stability and antioxidative properties of the conjugates compared to the native protein; (iii) apply the ternary conjugates to encapsulate β-carotene and evaluate the influence of processing and storage conditions on the physicochemical stability of β-carotene emulsions.
Experimental
Materials
Lactoferrin (LF) was obtained from Hilmar Ingredients (Hilmar, Calif., USA), the product contained 1.2% moisture, 0.3% ash and 99% protein, of which 96.1% was LF. Chlorogenic acid (CA, purity ≥99%) was purchased from BSZH Science Company (Beijing, China). Glucose (Glc, purity ≥99.0%) was purchased from Beijing Chemical Reagent (Beijing, China). Polydextrose (PD, type III, E12003) was supplied by Henan Tailijie Bioceth Co., Ltd. (Henan, China). Trolox (6-hydroxy-2,5,7,8-tetramethylchroman-2-carboxylic acid) was obtained from Sigma-Aldrich Chemie GmbH (Steinheim, Germany). β-Carotene suspension (30% by mass β-carotene in sunflower oil) was supplied by Xinchang Pharmaceutical Co., Ltd. (Zhejiang, China). Medium-chain triglyceride (MCT) oil was provided by Lonza Inc. (Allendale, NJ, USA). Standard β-carotene (purity >95%) was purchased from Sigma-Aldrich (St. Louis, Missouri, USA). Dialysis bag (molecular weight cut-off 12–14 kDa) was provided by Biodee Biotechnology Co., Ltd (Beijing, China). All other chemicals were of analytical grade, unless otherwise stated.
Synthesis of CA–LF conjugate
The CA–LF conjugate was synthesized according to the method of Rawel et al.25 with slight modification. Briefly, 1.0 g LF was dissolved in 50 mL of distilled water and stirred overnight to ensure complete dispersion and dissolution, and then the pH value of the protein solution was adjusted to 9.0 with 0.1 M NaOH. To prepare CA–LF conjugate, 0.25 g CA was dissolved in 50 mL of distilled water and the pH was adjusted to 9.0. Then the protein solution was mixed with the CA solution under continuous stirring (120 rpm). To prevent microbial growth during sample preparation, sodium azide (0.02%, w/w) was added. After 24 h continuous stirring at room temperature with free exposure to air, the samples were dialyzed for 48 h against water to remove free CA. Thereafter, the resulting solution was frozen and dried with Alpha 1-2 D Plus freeze-drying apparatus (Marin Christ, Germany) to obtain porous solids.
Synthesis of CA–LF–Glc/PD conjugates
LF, CA–LF conjugate and Glc/PD were respectively dissolved in distilled water at a concentration of 20 mg mL−1 and stirred overnight at 25 °C. Then CA–LF conjugate solution was mixed with Glc and PD solutions (1
:
1, w/w), respectively. Each sample was adjusted to pH 7.0 and then frozen-dried. The resultant powders were incubated at 60 °C/79% relative humidity (RH) in the presence of saturated KBr solution, for a period of 24 hours.26 Unheated LF, CA–LF conjugate and mixtures of CA–LF conjugate and Glc/PD were prepared under the same condition, and served as controls.
Measurement of UV absorbance and browning extent
The UV-Vis analysis of the samples was performed using a spectrophotometer (UV-1800, Shimadzu, Kyoto, Japan). Samples were dissolved in distilled water (pH 7.0) to a final concentration of 0.25 mg mL−1 and each sample was scanned with the wavelength ranging from 200 nm to 450 nm. The UV absorbance and browning extent of the samples were measured according to the method of Ajandouz et al.27 The solutions of different samples were appropriately diluted and the absorbance was measured at 294 and 420 nm with distilled water as blank references, respectively.
Analysis for the degree of graft
Degree of graft (DG) was indirectly determined from analysis of contents of free amino groups in the samples, following the ortho-phthaldialdehyde (OPA) method28 with minor modification. The OPA reagent was prepared freshly before used by mixing the following reagents: 40 mg of OPA (dissolved in 1 mL of methanol), 25 mL of 0.1 M sodium borate buffer (pH 9.85), 100 μL of β-mercaptoethanol, and 2.5 mL of 20% (w/v) sodium dodecyl sulfate in deionized water. The mixture was diluted to 50 mL with deionized water. Then 4 mL of OPA reagent and 200 μL of protein solution (4 mg mL−1) were mixed thoroughly and then reacted in a 35 °C water bath for 2 min. After that, the absorbance at 340 nm was measured using a double beam spectrophotometer. The content of free amino groups was calculated by using the calibration curve of L-leucine as a standard.
Degree of graft (DG) of ternary conjugates was calculated from the loss in free amino groups compared to the mixtures of CA–LF conjugate and Glc/PD as follows:
where
C0 is the content of free amino groups of the mixtures of CA–LF conjugate and Glc/PD,
Ct is the content of free amino groups of the conjugates.
Matrix-assisted laser desorption/ionization time-of-flight mass spectrometry (MALDI-TOF-MS) analysis
MALDI-TOF-MS measurements were performed on Autoflex-II TOF/TOF mass spectrometer (Bruker Daltonics, Billerica, MA, USA). Sinapinic acid was selected as the matrix and dissolved to saturation in a 1
:
1 mixture of 0.1% trifluoroacetic acid (TFA) and acetonitrile (50%, v/v). Briefly, 1 mg of the samples was dissolved in 1 mL of distilled H2O, then 0.5 μL of these solutions was brought on to the target and covered with 0.5 μL matrix. After crystallization by air-drying, the samples were analysed by MALDI-TOF-MS. The mass spectra were recorded in the reflector mode with an acceleration voltage of 20 kV and an effective flight path of 200 cm, and external calibration was obtained using bovine serum albumin.
Fourier transform infrared (FT-IR) spectroscopy
The infrared spectra of the samples were obtained with the potassium bromide pellet method. The dried samples were ground into powders, pressed into pellets and measured by a Spectrum 100 Fourier transform spectrophotometer (Perkin-Elmer, UK) in 400–4000 cm−1 range, at a resolution of 4 cm−1. KBr was used as a reference. For each measurement, 11 scans were taken. The data were processed using Origin 9.0 (OriginLab, Northampton, USA).
Circular dichroism spectra
Far-UV circular dichroism spectra of the samples were recorded in the range 190–250 nm with 0.1 mg mL−1 sample by a Chirascan spectrometer (Applied Photophysics Ltd, UK) using a quartz cylindrical cell in 1 mm path length. Ellipticity was recorded at a speed of 100 nm min−1, 0.2 nm resolution, 20 accumulations and 2.0 nm bandwidth. The collected data were analyzed using Dichroweb (Circular Dichroism Website http://dichroweb.cryst.bbk.ac.uk).29,30
Fluorescence spectroscopy
Fluorescence steady state measurements were performed on a fluorescence spectrophotometer (Varian Instruments, Walnut Creek, CA, USA). Scanning parameters for all measurements were optimized with slit width 20 nm for excitation and 10 nm for emission. The concentration of the samples was 1 mg mL−1. The excitation wavelength was set at 295 nm to selectively excite the tryptophan residues and the emission spectrum was collected between 320 and 380 nm.
Differential scanning calorimetry (DSC) measurement
Calorimetric analyses were performed using a DSC-60 thermal analysis system (Shimadzu, Tokyo, Japan). In a standard procedure, about 5.0 mg of samples was placed inside an aluminum pan and sealed tightly by a perforated aluminum lid, heated from 30 to 120 °C at a constant rate of 10 °C min−1 with a constant purging of dry nitrogen at a rate of 30 mL min−1. An empty aluminum pan was used as a reference. The peak temperature of denaturation was computed using the universal analysis software from each thermal curve.
Atomic force microscopy (AFM) measurements
AFM measurements of native LF, CA–LF conjugate and CA–LF–Glc/PD ternary conjugates/mixtures were performed by using a Nanoscope IIIa system (Digital Instruments, Veeco, Santa Barbara, California, USA) operating in tapping mode. Samples were diluted to a total protein concentration of 10 mg L−1 using deionized water, and aliquots (2 μL) of the diluted sample were placed onto a freshly cleaved mica sheet that was fixed on an iron disk (Bruker Corp., Santa Barbara, California, USA). After air-drying for more than 2 h, AFM images were collected at a scanning rate of 1.0 Hz under ambient condition. Image analysis was conducted by using Digital Nanoscope software (version 5.30r3, Digital Instruments, Veeco, Santa Barbara, California, USA). For each experiment, at least five different areas were randomly chosen for imaging. The height and phase images were simultaneously recoded during AFM imaging.
Antioxidant activities
ABTS˙+ scavenging activity. The ABTS˙+ scavenging activity was evaluated according to Siddhuraju et al.31 A stock solution of ABTS˙+ (7 mM) was prepared by diluting 10 mg of ABTS with 2.6 mL of potassium persulfate solution (2.45 mM). Then the mixture was kept in the dark for 12–16 h at room temperature before used. Thereafter, the ABTS working solution was diluted with distilled water to an absorbance of 0.70 ± 0.02 at 734 nm. Then 1 mL of the sample (0.5 mg mL−1) and 3 mL of ABTS solution were mixed, incubated at room temperature for 1 h, and the absorbance at 734 nm was then measured using a spectrophotometer. The scavenging activities of samples were measured as the decrease of the absorbance and expressed as percent scavenging of ABTS˙+.
Reducing power. The ability of samples to reduce iron(III) was determined according to Yildirim et al.32 Specifically, 1 mL of the sample was mixed with 2.5 mL of 0.2 M sodium phosphate buffer (pH 6.6), and the reaction was initiated by addition of 1% (w/v) potassium ferricyanide. The mixtures were incubated at 50 °C for 20 min. After that, 2.5 mL of 10% (w/v) trichloroacetic acid was added and the mixture was centrifuged at 3000 × g for 10 min. Finally, 2.5 mL of the supernatant was mixed with 0.5 mL of distilled water and 0.1 mL of FeCl3 (0.1%, w/v), followed by the measurement of absorbance at 700 nm using a spectrophotometer. The reducing power was calculated on basis of the Trolox calibration curve, which was carried out by the method mentioned above, and expressed as μmol Trolox equivalents per g sample.
Preparation of β-carotene emulsions
LF, CA–LF conjugate, and CA–LF–Glc/PD mixtures and conjugates were first dispersed in distilled water at a protein concentration of 0.7 wt%, separately. And then the solutions were stirred overnight to ensure complete dispersion and dissolution, while sodium azide (0.02% w/v) was added to prevent microbial growth. β-Carotene emulsions were prepared according to a previous study33 with slight modification. Briefly, β-carotene (0.1 wt% in the final emulsion) was first dissolved in medium chain triacylglycerol (MCT) oil (5 wt% in the final emulsion) at 140 °C for 30 seconds, and then mixed with different aqueous phase by using an Ultra-Turrax (Model T25, IKA-Works, Inc., Cincinnati, Ohio, USA) at a speed of 10
000 rpm for 10 min to form coarse emulsions, which were further homogenized using a Niro-Soavi Panda two-stage valve homogenizer (Parma, Italy) for three cycles at 60 MPa. The final emulsions were immediately cooled down to 25 °C and sampled to measure the particle characteristics. The residual emulsions were transferred into screw-capped brown bottles and stored at 4 °C in the dark.
Measurement of droplet size and ζ-potential
Particle size and size distribution were determined by dynamic light scattering (DLS) using a Zetasizer Nano-ZS90 (Malvern Instruments, Worcestershire, UK) at a fixed angle of 90°. The ternary conjugates were determined at a total protein concentration of 1 mg mL−1, and β-carotene emulsions were diluted (1
:
500) with deionized water prior to analysis to minimize multiple scattering effects. The ζ-potential was determined by measuring the direction and velocity of droplet movement in a well-defined electric field. The data were collected from at least 10 sequential reading per sample after 60 s of equilibration, and the data were calculated by the instrument using the Smoluchowski model. All measurements were performed in triplicate.
Measurement of physicochemical stability of β-carotene emulsions
Thermal stability. The β-carotene emulsions were incubated at boiling water (100 °C) for 5 min, and then cooled down and stored for 1 week. The droplet sizes were measured to evaluate the stability of β-carotene emulsions.
Freeze–thaw stability. The β-carotene emulsions (5 mL) were transferred into cryogenic test tubes, which were incubated in a −18 °C freezer for 20 hours and then thawed by placing them in a water bath at 30 °C for 2 hours.34 The droplet sizes were evaluated before and after the freeze–thaw treatments.
Low pH stability. The pH of β-carotene emulsions was adjusted to 3.0 and 6.0 using 0.1 M HCl or NaOH, and then the sizes were measured to evaluate the stability of β-carotene emulsions.
NaCl stability. NaCl was dissolved in deionized water to prepare 0.8 mol L−1 NaCl solution. The emulsions were mixed with the same volume of NaCl solution. The mixtures were shaken fully and placed at 25 °C for 6 h and the droplet sizes were determined.
Stability of β-carotene in emulsions. Emulsion samples were diluted with deionized water (1
:
4) and then transferred into screw-capped brown bottles flushed with nitrogen. Each emulsion sample was stored at 25 °C and 37 °C respectively in dark immediately after the dilution. β-Carotene content in the emulsion was determined according to Yuan et al.35 during the storage.
Statistical analysis
All experiments were performed in triplicate and the results were expressed as mean value ± standard deviation in this study. Data were analyzed by the software package SPSS 18.0 (SPSS Inc., Chicago, USA). Statistical differences were determined by One-way analysis of variance (ANOVA) with Duncan's post hoc test, and differences were considered to be significant with p < 0.05.
Results and discussion
Physicochemical properties of ternary conjugates
Changes in UV-vis absorbance and browning intensity. UV-vis spectra of LF, CA–LF, and CA–LF–Glc/PD mixtures and conjugates were compared (Fig. 1a). CA has an absorbance maximum at 324 nm (data not shown), which was absent in the LF control, but presented as a shoulder in the spectrum of CA–LF conjugate. It can be observed that the ternary conjugates and mixtures showed different absorptions in the UV-vis spectra, although they had similar shapes. The absorbance of the ternary conjugates increased significantly compared to the mixtures, and the peak of the ternary conjugates had a maximum absorbance that appeared in the range of 260–300 nm, which is characteristic of melanoidins.36 These results indicated that the glycosylation of CA–LF conjugate could enhance its absorption in the ultraviolet region.
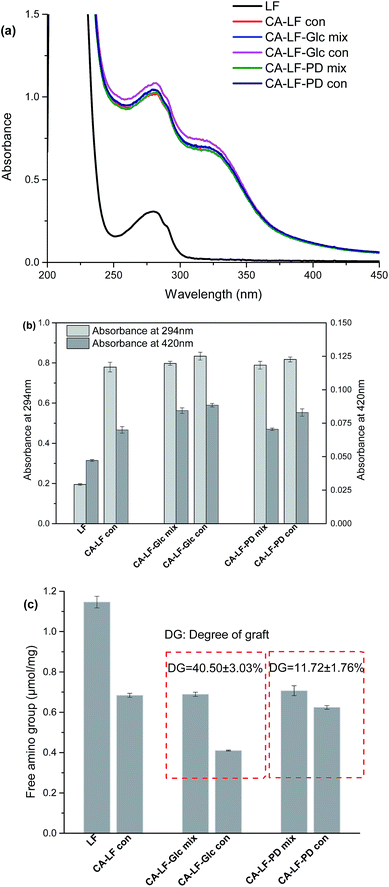 |
| Fig. 1 UV-Vis spectra (a), UV absorbance at 294 and 420 nm (b) and the content of free amino groups (c) of LF, CA–LF conjugate, CA–LF–Glc/PD mixtures and conjugates. | |
The colour of conjugated products was a direct and easy indication of Maillard reaction progress. Ultraviolet absorbance (A294 nm) and brown colour (A420 nm) are typical indicators of colourless intermediate compounds and final browning compounds, respectively.27 The changes of A294 and A420 of ternary conjugates were shown in Fig. 1b. It could be found that both A294 and A420 of LF significantly increased after its conjugation with CA, this was mainly owed to the formation of protein–polyphenol covalent complexes. Sabir et al.37 demonstrated that under neutral and alkaline conditions, sunflower protein solutions developed dark green and brown colours as a result of the covalent bonding with oxidation products of CA. Meanwhile, compared with CA–LF–Glc/PD mixture, CA–LF–Glc/PD ternary conjugates showed increased absorbance values of A294 and A420, which were characteristic indexes of the formation of intermediate compounds of the Maillard reaction. In addition, the A294/A420 absorbance ratio was indicative of the polymerization extent.27 Via calculating from the data in Fig. 1b, CA–LF–Glc conjugate exhibited the higher level of polymerization than CA–LF–PD conjugate. Therefore, these results analyses corroborated the occurrence of the Maillard reaction and confirmed that brown polymers were generated at the advanced stage.
Changes of free Amino groups' content. When evaluating the formation of protein–polyphenol conjugates, the estimation of modified amino acids is a critical method to analyze the extent of phenolic compound bound,15 which is also commonly used to determine the progress of Maillard reaction, since it occurs by covalent attachment of carbonyl group in reducing sugars with free amino groups in proteins to form Schiff base.38 In this study, the primary amino group availability in the protein was investigated using the OPA method. The amount of free amino groups in LF was reduced by 40.32% after the covalent binding between LF and CA, as the analysis was conducted in presence of 1% SDS (which destroys non-covalent protein interactions), we can suppose that the derivatization occurred through covalent binding. With dry-heating treatment for 24 h, the content of free amino groups in the CA–LF–Glc and CA–LF–PD conjugates was significantly reduced. As shown in Fig. 1c, the DG was 40.5% for CA–LF–Glc conjugate, and 11.72% for CA–LF–PD conjugate, indicating Maillard reaction between CA–LF conjugate and Glc occurred to a higher extent. These results interpreted that the Maillard reaction took place differently between CA–LF conjugate and two types of carbohydrates, the largely incomplete reaction with PD might be attributed to the steric hindrance. Because PD is an oligomer of Glc,39 not all lysines are accessible, this steric hindrance was increased with the conjugation of PD molecules to LF.
Confirmation of CA–LF–Glc/PD conjugates by MALDI-TOF-MS
MALDI-TOF MS is a high sensitivity technique that provides detailed structural information about the individual molecules contained in a polymer sample, it can potentially provide quantitative information required for determination of the average molecular mass and molecular mass distribution of a polymer.40 Analysis of molecular masses of native LF, control LF (heat-treated LF), native CA–LF conjugate, control CA–LF conjugate (heat-treated CA–LF conjugate) and CA–LF–Glc/PD ternary conjugates by MALDI-TOF MS was conducted to confirm the occurrence of the reaction described above and to investigate the extent of the glycosylation. The mass spectra of the samples were presented in Fig. 2. The mass of native LF showed a peak at 84
011.15, which was in agreement with its sequence. The spectrum profile of the control LF was very similar to that of the native LF, suggesting that dry heating almost did not cause any change in the molecular weight profile of LF. In case of native CA–LF conjugate, there was an increase in the molecular weight of 1058 corresponding to 3 CA molecules bound to 1 LF molecule. Moreover, it could be clearly observed from Fig. 2 that the dry heating induced substantial aggregation in the CA–LF conjugates, probably as a consequence of the covalent bonding of CA–LF conjugate with the exposed amino acids. Furthermore, the reaction of CA–LF conjugate with Glc and PD resulted in the higher-molecular mass species, which might have been caused by the introduction of Glc/PD to the CA–LF conjugate and the subsequent increase in the molecular mass. It was interesting to note that the mass of CA–LF–Glc conjugate was higher than that of CA–LF–PD conjugate, though Glc is a small molecule compared with PD, implying that the greater extent of reactivity between CA–LF conjugate and Glc. This phenomenon was attributed to the difference in structures of Glc and PD. PD was initially produced by vacuum-melt condensation of Glc, which is a highly branched indigestible Glc polymer with an average degree of polymerisation of ten or twelve Glc molecules.41,42 Thus, at the same mass ratio between protein and the carbohydrates in the Maillard reaction, Glc had higher reaction activity than PD for more C-terminals molecules. In addition, it was generally admitted that the sugar reactivity was directly to the proportion of the active carbonyl species in the solution, Glc was characterized by a more accessible carbonyl function, which was probably another explanation why it was more reactive.43
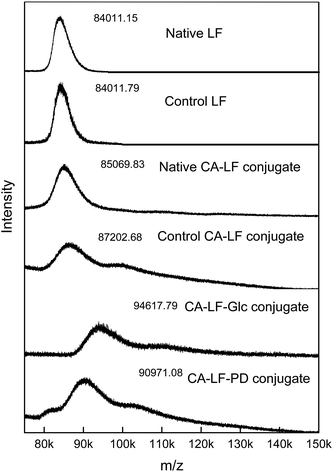 |
| Fig. 2 MALDI-TOF-MS analysis of native/control LF, native/control CA–LF conjugates and CA–LF–Glc/PD conjugates. | |
Generally, polyphenols can be oxidized in an alkaline solution to its corresponding quinone, and then being a reactive electrophilic intermediate, could readily undergo attack by nucleophiles such as lysine, cysteine and tryptophan moieties in a protein chain, leading to the formation of cross-linked protein polymers.20 According to Folin–Ciocalteu method (data not shown), the amount of CA covalently bound to LF was 27.26 mg g−1 CA–LF conjugates, with a reaction percentage yield of 13.63%.
In this study, the available amino group of the CA–LF conjugate could then react with the reducing end of the carbohydrate. The proposed scheme of the formation of ternary conjugates was shown in Fig. 3, CA was readily oxidized to its respective quinone, the CA quinone may form a dimer or polymer in a side reaction, and then reacted with amino or sulfhydryl side chains of LF, to generate covalent C–N or C–S bonds with the phenolic ring, with restructuring of hydroquinone. The latter might be reoxidized and bind a second protein, therefore it comes to cross-links of protein molecules, resulting in the polymerization. The available amine groups of the resulting polymer can react with the carbonyl group of Glc or PD, to form an N-glycosylamine with the release of one water molecule.44 The N-glycosylamine undergoes an irreversible rearrangement generating the Amadori product, which may be altered by oxidation, fragmentation, dehydration, and free radical reactions, resulting in the formation of CA–LF–Glc/PD conjugates.
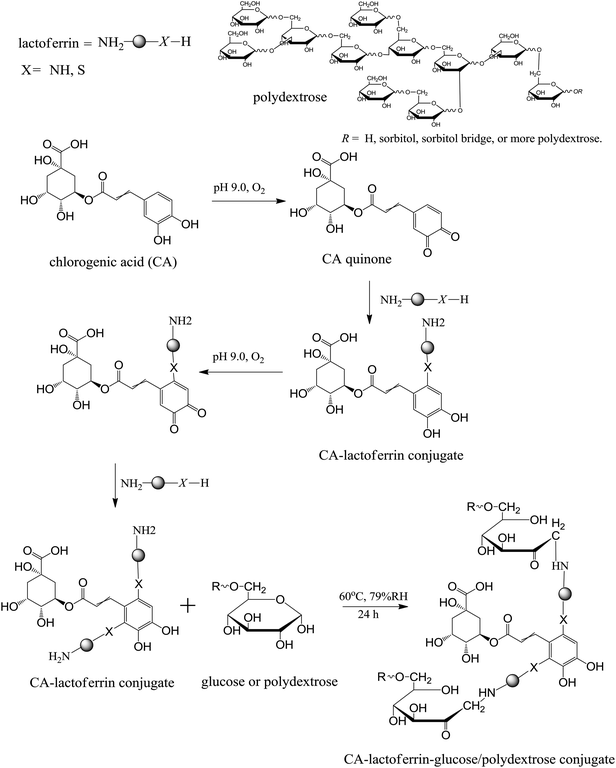 |
| Fig. 3 Proposed reactions of LF, CA and glucose/polydextrose. The quinone derivatives may be monomer, dimer, or polymer. | |
Structural characterization of CA–LF–Glc/PD ternary conjugates
FTIR analysis. The chemical structures of the ternary conjugates were characterized by FTIR measurement. Fig. 4 showed the infrared spectral changes of LF, CA–LF conjugate and CA–LF–Glc/PD conjugates. FTIR profiles revealed that some pronounced differences among native LF, CA and CA–LF conjugate (Fig. 4a), and distinguished CA–LF–Glc/PD conjugates from the CA–LF–Glc/PD mixtures (Fig. 4b). CA has three characteristic peaks around 3350, 3467 and 3618 cm−1, probably due to the vibration of the O–H linkage of phenolic hydroxyl groups, and two large peaks around 1686 and 1639 cm−1, probably due to the carbonyl stretching of CA, while those peaks were not found in CA–LF conjugate at all. In the FTIR measurement of LF, the assignments of representative peaks have been already clarified, the characteristic absorption bands at around 3300 cm−1, 1651 cm−1, and 1533 cm−1 were assigned to amide A (representative of N–H stretching coupled with hydrogen bonding), amide I (representative of stretching/hydrogen bonding coupled with COO–) and amide II (representative of C–N stretching coupled with NH bending modes), respectively.18 When CA was bound to LF, we could find that there was more or less spectral shifting for the protein amide A and amide I band, suggesting that the interaction of CA with skeletal N–H, C–O or COO– group of LF molecules most likely caused the conformational change of the protein. In addition, it could be found that there was a noticeable change in amide II, attributed to skeletal C–N and C
C vibrations, which might be due to the oxidation of CA and formation of more complicated dimeric-structures in the covalent reaction process.45
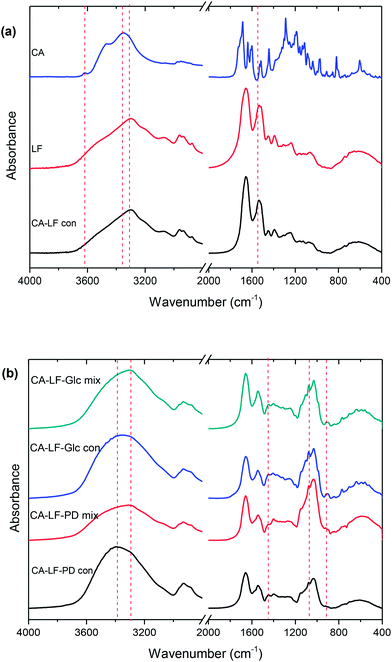 |
| Fig. 4 FTIR spectra of LF, CA, CA–LF conjugate (a) and CA–LF–Glc/PD mixtures and conjugates (b). | |
It might be predictable that Maillard reaction between CA–LF conjugate and carbohydrates could lead to loss of functional groups including NH2, especially from lysine, while their amount associated with Maillard products such as the Amadori compound (C
O) and Schiff base (C
N) may be increased, these chemical changes would lead to some alterations in the FTIR spectrum.46 For carbohydrates, a series of overlapping peaks located in the region of 1180–953 cm−1 resulted from vibration modes such as the stretching of C–C and C–O and the bending mode of C–H bonds, these absorptions were weak in the spectra of most proteins.47 Fig. 4b showed the infrared spectral changes of CA–LF–Glc/PD mixtures/conjugates. Compared with the CA–LF–Glc/PD mixtures, there were obvious red shifts at amide A in the spectra of CA–LF–Glc/PD conjugates (especially for CA–LF–PD conjugate with a shift of 90 nm), the absorption band located at 3300–3400 cm−1 could be attributed to the –NH2 and –OH stretch vibration,48 suggesting the modification of LF amino groups in these regions. Regions of 1651 and 1533 cm−1, denoted as C
O and C–N stretching from amide I and II, were modified by the Maillard reaction, because the intensity of CA–LF–Glc/PD conjugates decreased compared to CA–LF–Glc/PD mixtures. In addition, there were some variations in the peaks of CA–LF–Glc/PD conjugates at around 1445–1448 cm−1 attributes to CH2 stretching, and 1050–950 cm−1 corresponds to side-chain vibrations from the protein regions,49 indicating the alteration of protein structure.
Secondary structures analysis. Circular dichroism spectra are remarkably sensitive to the secondary structure of proteins.50 In this study, far-UV circular dichroism spectra were used to characterize the secondary structures of LF in the mixtures and conjugates, the estimated secondary structures were shown in Table 1. As indicated in Fig. 5a, the far-UV of the native LF displayed a negative minimum at 209 and a maximum at 190–195 nm, which is typical of α class protein. The conjugation of LF with CA caused a decrease in band intensity at all wavelengths in the far-UV spectrum, without any significant shift of the peaks. However, the change of the intensity resulted from a decrease in α-helix and an increase in unordered structure (Table 1), similar to that observed by Rawel et al.,25 who revealed that covalent interaction of CA with bovine serum albumin caused a decrease in α-helix structure, with a parallel increase in unordered structure. It was reported that covalent binding of polyphenols with different protein chains could result in full or partial unfolding or denaturation of the protein chains by altering their secondary and tertiary structures,51 hydrophobic interactions between polyphenol and pralines residues,25 electrostatic effects and hydrogen bonds51 could influence protein α-helical intermediate formation.
Table 1 Changes of secondary structures of LF, CA–LF conjugate and CA–LF–Glc/PD mixtures/conjugates
Samples |
α-Helix |
β-Sheet |
β-Turns |
Unordered |
LF |
0.550 |
0.086 |
0.151 |
0.257 |
CA–LF con |
0.385 |
0.086 |
0.226 |
0.320 |
CA–LF–Glc mix |
0.561 |
0.084 |
0.126 |
0.248 |
CA–LF–Glc con |
0.386 |
0.093 |
0.211 |
0.334 |
CA–LF–PD mix |
0.527 |
0.090 |
0.159 |
0.271 |
CA–LF–PD con |
0.336 |
0.087 |
0.248 |
0.356 |
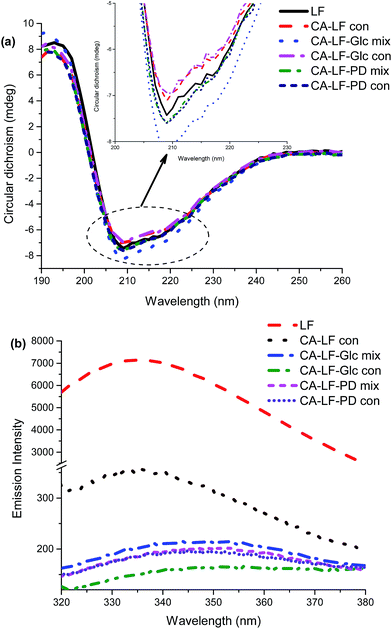 |
| Fig. 5 Far-UV CD spectra (a) and fluorescence spectra (b) of LF, CA–LF conjugate, CA–LF–Glc/PD mixtures and conjugates. | |
In the case of Maillard reaction products, compared with CA–LF–Glc/PD mixtures, the CA–LF–Glc/PD conjugates extremely lost the α-helix structure with a concomitant increase in turns and unordered proportions. These results indicated that the secondary structures of CA–LF conjugate might be changed by Maillard reaction procedure. Some researchers52,53 reported that the Maillard reactions between proteins and carbohydrates could affect the secondary structures of proteins, which was not only due to the interaction of biopolymers but also to the heat denaturation of proteins during the treatment. In this study, when Glc/PD were added to CA–LF conjugate, the hydrogen bonds could be formed, which probably had an intermolecular interaction among the neighbouring proteins and led to the increased amount of α-helix and reduced the amount of intermolecular unordered structure. After the CA–LF–Glc/PD mixtures were heated, the hydrogen bonds between Glc/PD and CA–LF conjugate were reduced, the conjugation of CA–LF and Glc/PD could possibly impair the intermolecular interaction, which would finally lead to a reduction of α-helix.54
Emission fluorescence spectroscopy analysis. LF was considered to have intrinsic fluorescence because of tryptophan and tyrosine residues. At 295 nm wavelength, tryptophan residue was excited and the quenching could take place when the quencher was sufficiently close to it.55 A possibility of the reaction between CA and tryptophan was also studied by Wang et al.33 The fluorescence emission spectra of LF, CA–LF conjugate and CA–LF–Glc/PD ternary conjugates were shown in Fig. 5b. When the excitation was at 295 nm, LF exhibited a fluorescence maximum emission (λmax) at 336 nm. Pronounced quenching effects were observed in case of CA–LF conjugate, which presumably involved a Forster type fluorescence resonance energy transfer (FRET). In addition, both the CA–LF–Glc/PD mixtures and conjugates showed decreased maximum emissions and marked red shifts of the λmax relative to the CA–LF conjugate. These findings proved that tryptophan residues in the ternary mixtures and conjugates were largely surrounded to a hydrophobic environment and the protein might have more compact tertiary conformations compared to native LF and CA–LF conjugate.53 It is believed that the conjugate layer of carbohydrates could efficiently reduce the fluorescence intensity,56 the fluorescence intensity of CA–LF–Glc conjugate was remarkably lower than that of CA–LF–Glc mixture, which implied the former had a thicker layer and formed a shielding effect.28
DSC analysis. Thermal characterization of native LF, CA–LF conjugate and CA–LF–Glc/PD mixtures/conjugates was also performed by recording the DSC thermograms, the characteristic parameters were summarized in Table 2. Native LF was observed to generate two endothermic peaks at 68.4 and 89.1 °C, which was corresponded to the two denaturation temperatures (Td). These peaks were attributed to the difference in the heat sensitivity of the two lobes of LF, since the C lobe seemed more compact than the N lobe in the iron-saturated protein.57 However, CA–LF conjugate showed one endothermic peak, at a higher (p < 0.05) temperature than native LF (compared with the first Td), indicating the modification of CA enhanced thermal stability of LF. In addition, the covalently modified LF required less energy to unfold, since the ΔH was decreased (p < 0.05) when compared with native LF. These results were in agreement with the findings reported by Liu et al.,18 who demonstrated that the modification with CA by radical polymerization could increase the denaturation temperature of LF.
Table 2 Denaturation temperature (Td) and enthalpy change (ΔH) of LF, CA–LF conjugate and CA–LF–Glc/PD mixtures/conjugates at pH 7.0
Samples |
Tonset |
Td |
ΔH (mJ mg−1) |
LF |
63.57 ± 0.12 (88.34 ± 0.15) |
68.43 ± 0.23 (89.14 ± 0.17) |
−9.26 ± 0.03 (−0.12 ± 0.01) |
CA–LF con |
64.14 ± 0.22 |
71.61 ± 0.21 |
−2.87 ± 0.06 |
CA–LF–Glc mix |
68.53 ± 0.19 |
68.72 ± 0.09 |
−3.00 ± 0.04 |
CA–LF–Glc con |
69.87 ± 0.13 |
72.13 ± 0.14 |
−1.36 ± 0.07 |
CA–LF–PD mix |
68.10 ± 0.23 |
74.03 ± 0.20 |
−3.43 ± 0.08 |
CA–LF–PD con |
71.60 ± 0.16 |
76.04 ± 0.08 |
−1.66 ± 0.03 |
In general, the glycation could lead to an increase of Td and a decrease of ΔH, indicating the improved thermal stability or tertiary conformational stability of proteins.7 In this study, Td values of CA–LF–Glc/PD mixtures/conjugates were significantly higher (p < 0.05) than that of native LF, and CA–LF–Glc/PD conjugates have higher (p < 0.05) values of Td than that of CA–LF conjugate. Thus, the thermal stability of CA–LF conjugate was remarkably improved by Maillard-type conjugation. Furthermore, the ΔH values of CA–LF–Glc/PD conjugates were decreased (p < 0.05) compared with CA–LF–Glc/PD mixtures, a lower ΔH value suggested that less energy was required for the denaturation, which might be attributed to the partial denaturation of CA–LF conjugate in the Maillard reaction. These findings indicated a protective effect of the glycation on the thermal denaturation of LF.
Particle size, ζ-potential and morphology analysis. DLS has been proved to be an effective technique for analyzing the size and size distribution of polymers, proteins and nanoparticles in suspension, based on the Brownian motion of spherical particles.58 AFM observation and DLS analysis were performed in order to determine the particles morphology and dimensions of the conjugates (Fig. 6). Native LF, a globular protein, was a small globule of non-uniform size (6.5 nm), with an average ζ-potential of about +25.7 mV. For CA–LF conjugate, the AFM image showed regular globular shape with smooth surface, and the average diameter was about 10.10 nm, slightly bigger than that of native LF. However, the ζ-potential of CA–LF conjugate was about −7.73 mV, this is because covalent modification of the protein with CA could change its isoelectric point (PI) to a lower pH value,25 the CA–LF conjugate carried negative charge when the pH of the solution was higher than the PI, the absolute ζ-potential value of CA–LF conjugate was smaller than that of LF, this phenomenon could be explained by the fact that CA conjugated to LF lowered the electrophoresis mobility. Meanwhile, the particles of the ternary mixtures were partial irregular in shape, with a mean size of 13.47 ± 0.28 nm, resulting in a polydispersed distribution. Compared with CA–LF–Glc/PD mixtures, the ternary conjugates showed coarser structure and bigger particles size, the greater hydrodynamic size of the conjugate particles indicated the formation of higher molecular weight polymers. Besides, the conjugating reaction was not homogeneous, the spherical structure was almost disappeared in the ternary conjugates, and therefore, it suggested that the conjugates might have core–shell structure, the CA–LF conjugate molecules conjugating with Glc/PD located on the surface of the core, while those without conjugation located inside the core. It is important to note that the ζ-potential values of the conjugates were different from those of the mixtures, CA–LF–Glc conjugate exhibited the highest ζ-potential among the conjugates, indicating the best stability based on electrostatic repulsion.
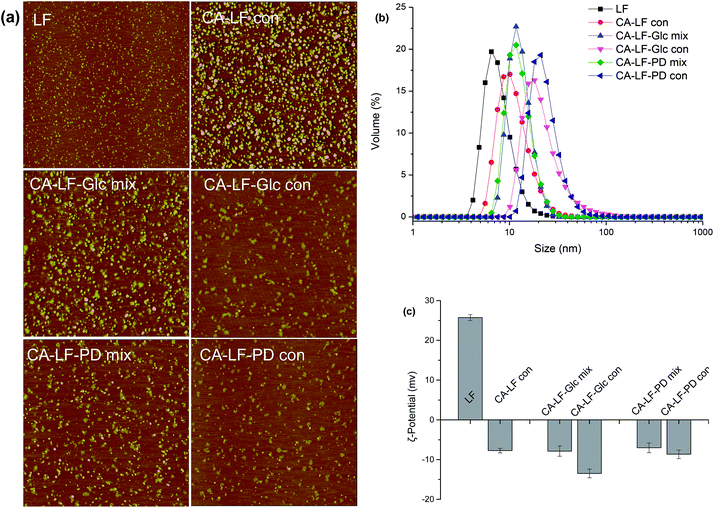 |
| Fig. 6 Atomic force microscopy images (2.0 × 2.0 μm) (a), particle size distribution (b) and ζ-potential (c) of LF, CA–LF conjugate, CA–LF–Glc/PD mixtures and conjugates in solutions. | |
Antioxidant activities of the conjugates
Some Maillard reaction products (MRPs) have been shown to contribute greatly to the shelf-life of heat-treated foods because of their antioxidant, antiallergenic, antimicrobial and cytotoxic properties, many studies were focused on the high antioxidant capacity of MRPs in model systems and foods such as beer, coffee and bakery products.59 The ABTS˙+ scavenging activity and the reducing power of LF, CA, CA–LF conjugate and CA–LF–Glc/PD mixtures/conjugates were shown in Fig. 7a and b, respectively. It could be found that native LF showed little ABTS˙+ scavenging activity and reducing power, however, the CA–LF conjugate exhibited higher (p < 0.05) antioxidant activity, with 2.73-fold ABTS˙+ radical scavenging activity and 1.86-fold reducing power of LF. These results indicated that the covalent incorporation of CA onto LF could strongly scavenge the radicals and formed more stable products, and finally terminated the radical chain reaction.
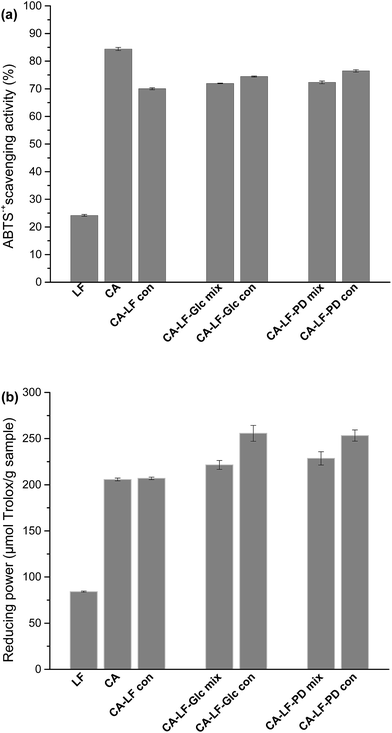 |
| Fig. 7 Antioxidant activities of LF, CA, CA–LF conjugate, CA–LF–Glc/PD mixtures and conjugates (a) ABTS˙+ scavenging activity, (b) reducing power. | |
With regard to the antioxidant activity of chlorogenic acid, the ABTS˙+ scavenging activity was 84.41% and the reducing power was 205.72 μmol Trolox/g CA–LF conjugate (an equivalent amount of free CA). As a consequence of the covalent binding between CA and LF, the ABTS˙+ scavenging activity of CA was significantly (p < 0.05) decreased, which may be attributed to the polymerization mechanism in Fig. 3. In addition, the steric hindrance might be partly responsible for the loss of the antioxidative ability of the covalently bound phenolic compound.60
Compared with unheated CA–LF–Glc/PD mixtures, CA–LF–Glc/PD conjugates presented enhanced antioxidant capacities. The ABTS˙+ scavenging activity and reducing power of MRPs derived from Glc and PD had no significant differences but were higher than those of the corresponding mixtures. It is supposed that the antioxidant effect was related to the development of intermediate reductone compounds, which were reported to break the radical chain by donation of a hydrogen atom, and were regarded as terminators of free radical chain reactions.61 By comparing the reducing power and radical-scavenging activity of the ternary conjugates and those of CA–LF–Glc/PD mixtures, it could be found that the antioxidant activity of the protein was greatly improved by the modification of CA and Glc/PD. Therefore, CA–LF–Glc/PD ternary conjugates might be used as effective antioxidants to prevent lipid oxidation in food products.
Stability of β-carotene emulsions stabilized by CA–LF–Glc/PD conjugates under different processing conditions
The purpose of these experiments was to investigate the emulsifying properties of CA–LF–Glc/PD conjugates and examine the effect of environmental stresses (thermal processing, freeze–thaw cycling, low pH and high ionic strength) on the droplet sizes of β-carotene emulsions. The droplet sizes of original and heated emulsions were presented in Fig. 8a. Compared with the conjugates, the droplet sizes of original emulsions stabilized by the mixtures of CA–LF conjugate and Glc/PD were larger, which indicated that the non-covalent carbohydrate molecules tended to enhance droplet flocculation phenomena through depletion effects. After heating, the droplet sizes of the emulsions stabilized by CA–LF–Glc/PD conjugates were significantly smaller than those by the mixtures, CA–LF–Glc conjugate exhibited better stability than CA–LF–PD conjugate.
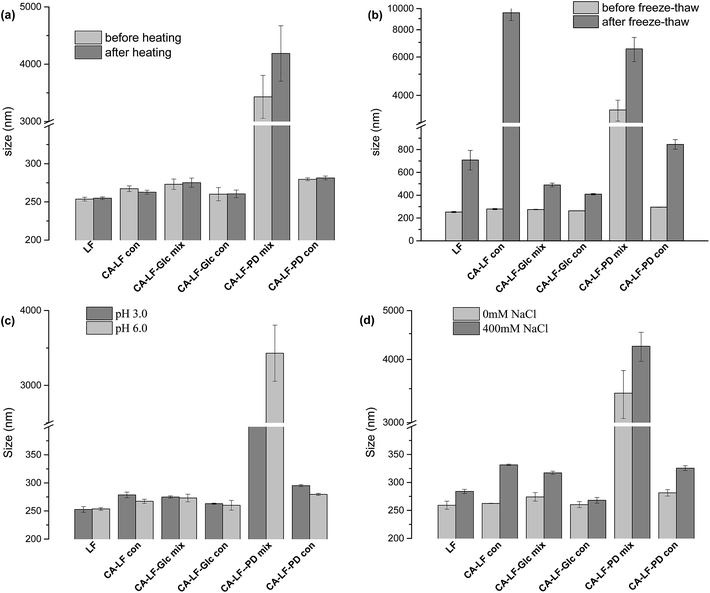 |
| Fig. 8 The effect of processing condition (a) 100 °C for 5 min, (b) freeze–thaw treatment, (c) low pH and (d) high ionic strength on the particle size of β-carotene emulsions stabilized by LF, CA–LF conjugate, CA–LF–Glc/PD mixtures and conjugates. | |
The influence of freeze–thaw cycling on droplet size of emulsions was shown in Fig. 8b, In comparison with the mixtures stabilized emulsions, CA–LF–Glc/PD conjugates stabilized emulsions had smaller sizes, demonstrating a better freeze–thaw stability, which might be attributed to the formation of a thick interfacial coating surrounding the oil droplets that resisted rupture during freezing and prevented the droplet aggregation.
Since acidic pH and high ionic strength are often found in food systems, the mean droplet sizes of emulsions were measured at both acidic and high ionic strength environment to evaluate the potential applications of these conjugates in food industry. Fig. 8c showed the droplet sizes of emulsions at pH 3.0 and 6.0. The droplet sizes of CA–LF–Glc mixture/conjugate stabilized emulsions at pH 3.0 were similar to those of the initial emulsions (pH 6.0), in ternary conjugates-stabilized emulsions they were smaller than those in the mixtures at pH 3.0, which indicated that the conjugates were efficient in forming stable emulsions under acidic condition. Fig. 8d showed droplet sizes of emulsions containing electrolyte (400 mM NaCl). Due to the electrostatic screening and ion binding effects reduced the electrostatic repulsion between the oil droplets, the emulsions stabilized by LF and the CA–LF conjugate were unstable to droplet aggregation in the presence of NaCl, but the CA–LF–Glc conjugate stabilized emulsion was stable against the aggregation, and average droplet sizes of the emulsions stabilized by CA–LF–Glc/PD conjugates were significantly (p < 0.05) smaller than those stabilized by CA–LF–Glc/PD mixtures, which interpreted clearly that the structure or thickness of interfacial membrane formed by Maillard-type conjugates was highly effective in stabilizing O/W emulsions. These results were in agreement with Zhu et al.,62 who stated that covalent bonds produced between whey protein isolate (WPI) and dextran in Maillard-type conjugate was very stable against the change in pH, temperature, and ionic strength, and the emulsifying properties was improved over the native WPI due to thick steric barrier, increased oil droplet surface hydrophilicity by polysaccharide or adsorptive ability by the unfolded structure of protein, and decreased non-covalent interaction.
Chemical stability of β-carotene in emulsions stabilized by CA–LF–Glc/PD conjugates under storage condition
Due to the highly unsaturated chemical structure, β-carotene is prone to degradation during processing and storage. Conjugating antioxidants (such as EGCG, CA) with a protein or polysaccharide as an emulsifier could be an effective way to retard β-carotene degradation in emulsions.33,63,64 The influence of CA–LF–Glc/PD conjugates on the chemical stability of β-carotene in emulsions was examined. Fig. 9 showed the degradation profile of β-carotene in emulsions stabilized with LF, CA–LF conjugate and CA–LF–Glc/PD mixtures/conjugates as a function of storage time.
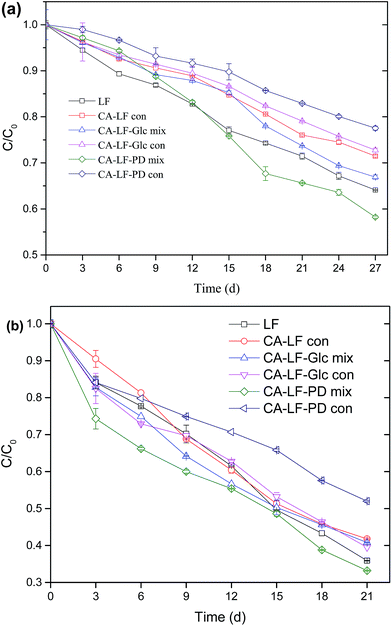 |
| Fig. 9 β-Carotene degradation as a function of storage time at (a) 25 °C and (b) 37 °C in emulsions stabilized by LF, CA–LF conjugate, CA–LF–Glc/PD mixtures and conjugates. | |
After the storage at 25 °C and 37 °C for a period of time, obvious losses of β-carotene were observed in emulsions stabilized by native LF and CA–LF–PD mixture, whereas the degradation of β-carotene in emulsions stabilized by CA–LF–PD conjugate was obviously retarded. After 3 weeks of the storage at 37 °C, the losses of β-carotene in the emulsions prepared with CA–LF–PD mixture and LF were 66.8% and 64.1%, respectively, but about 48.0% for CA–LF–PD conjugate stabilized emulsion. These results were attributed to that the conjugate could form a thicker interfacial layer in emulsion droplets than the protein alone, which could impact the diffusion of oxygen, free radicals, and pro-oxidants at oil–water interface. In addition, Maillard-type conjugates exhibited better antioxidant potential than the mixtures and the protein alone, which could also scavenge free radicals and consequently avoided the oxidation and degradation of β-carotene.63,65
Conclusions
CA–LF–Glc/PD ternary conjugates were successfully prepared using a naturally occurring Maillard reaction between CA-modified LF and Glc as well as PD under mild condition. Formation of ternary conjugates was confirmed by MALDI-TOF-MS, FTIR spectroscopy, DSC and fluorescence analyses. The covalent processes significantly altered the physicochemical and structural properties of LF and the conjugates were of high-antioxidative, heat-resistant and colloidally stable properties. By applying the conjugates as emulsifiers, they were shown to be effective to enhance physicochemical stability of β-carotene emulsions under processing and storage conditions. This study demonstrates that CA–LF–Glc/PD conjugates are suitable biomacromolecules for the protection and delivery of bioactive compounds in food and pharmaceutical industries.
Abbreviations
LF | Lactoferrin |
CA | Chlorogenic acid |
Glc | Glucose |
PD | Polydextrose |
CA–LF con | Chlorogenic acid–lactoferrin conjugate |
CA–LF–Glc con | Chlorogenic acid–lactoferrin–glucose ternary conjugate |
CA–LF–Glc mix | Mixture of lactoferrin–chlorogenic acid conjugate and glucose |
CA–LF–PD con | Chlorogenic acid–lactoferrin–polydextrose ternary conjugate |
CA–LF–PD mix | Mixture of lactoferrin–chlorogenic acid conjugate and polydextrose |
MALDI-TOF-MS | Matrix-assisted laser desorption/ionization time-of-flight mass spectrometry |
FTIR | Fourier transform infrared spectroscopy |
DSC | Differential scanning calorimetry |
AFM | Atomic force microscopy |
DLS | Dynamic light scattering |
PI | Isoelectric point |
Td | Denaturation temperatures |
DG | Degree of graft |
Acknowledgements
We thank Dr Yanxia Jia of Chinese Academy of Sciences for assistance in the AFM analysis. Financial support from the National Natural Science Foundation of China (No. 31371835) is gratefully acknowledged.
Notes and references
- C. M. Oliver, L. D. Melton and R. A. Stanley, Crit. Rev. Food Sci. Nutr., 2006, 46, 337–350 CrossRef CAS PubMed.
- A. K. I. O. Kato, Food Sci. Technol. Res., 2002, 8, 193–199 CrossRef CAS.
- L. Jakobek, Food Chem., 2015, 175, 556–567 CrossRef CAS PubMed.
- D. Xu, X. Wang, J. Jiang, F. Yuan and Y. Gao, Food Hydrocolloids, 2012, 28, 258–266 CrossRef CAS PubMed.
- H. Zhou, X. Sun, L. Zhang, P. Zhang, J. Li and Y. N. Liu, Langmuir, 2012, 28, 14553–14561 CrossRef CAS PubMed.
- C. Li, F. Liu, Y. Gong, Y. Wang, H. Xu, F. Yuan and Y. Gao, LWT--Food Sci. Technol., 2014, 57(2), 612–617 CrossRef CAS PubMed.
- J. Liu, Q. Ru and Y. Ding, Food Res. Int., 2012, 49, 170–183 CrossRef CAS PubMed.
- U. Einhorn-Stoll, M. Ulbrich, S. Sever and H. Kunzek, Food Hydrocolloids, 2005, 19, 329–340 CrossRef CAS PubMed.
- L. N. Vhangani and J. van Wyk, Food Chem., 2013, 137, 92–98 CrossRef CAS PubMed.
- Q. Liu, B. Kong, J. Han, C. Sun and P. Li, Food Struct., 2014, 1, 145–154 CrossRef PubMed.
- Y. Wang, F. Liu, C. Liang, F. Yuan and Y. Gao, J. Sci. Food Agric., 2014, 94, 2986–2991 CrossRef CAS PubMed.
- W. Deng, J. Li, P. Yao, F. He and C. Huang, Macromol. Biosci., 2010, 10, 1224–1234 CrossRef CAS PubMed.
- G. Markman and Y. D. Livney, Food Funct., 2012, 3, 262–270 CAS.
- M. Akhtar and E. Dickinson, Colloids Surf., B, 2003, 31, 125–132 CrossRef CAS.
- S. Rohn, Food Res. Int., 2014, 65, 13–19 CrossRef CAS PubMed.
- T. Ozdal, E. Capanoglu and F. Altay, Food Res. Int., 2013, 51, 954–970 CrossRef CAS PubMed.
- S. V. Prigent, A. G. Voragen, A. J. Visser, G. A. van Koningsveld and H. Gruppen, J. Sci. Food Agric., 2007, 87, 2502–2510 CrossRef CAS PubMed.
- F. Liu, C. Sun, W. Yang, F. Yuan and Y. Gao, RSC Adv., 2015, 5, 15641–15651 RSC.
- Z. Wei, W. Yang, R. Fan, F. Yuan and Y. Gao, Food Hydrocolloids, 2015, 45, 337–350 CrossRef CAS PubMed.
- J. Kroll, H. M. Rawel and S. Rohn, Food Sci. Technol. Res., 2003, 9, 205–218 CrossRef CAS.
- M. Ali, T. Homann, M. Khalil, H. P. Kruse and H. Rawel, J. Agric. Food Chem., 2013, 61, 6911–6920 CrossRef CAS PubMed.
- X. Wang, J. Zhang, F. Lei, C. Liang, F. Yuan and Y. Gao, Food Chem., 2014, 150, 341–347 CrossRef CAS PubMed.
- P. P. Ward, S. Uribe-Luna and O. M. Conneely, Biochem. Cell Biol., 2002, 80, 95–102 CrossRef CAS.
- N. Orsi, BioMetals, 2004, 17, 189–196 CrossRef CAS.
- H. M. Rawel, S. Rohn, H. P. Kruse and J. Kroll, Food Chem., 2002, 78, 443–455 CrossRef CAS.
- A. M. Moscovici, Y. Joubran, V. Briard-Bion, A. Mackie, D. Dupont and U. Lesmes, Food Funct., 2014, 5, 1898–1908 CAS.
- E. H. Ajandouz, L. S. Tchiakpe, F. D. Ore, A. Benajiba and A. Puigserver, J. Food Sci., 2001, 66, 926–931 CrossRef CAS PubMed.
- M. S. Vigo, L. S. Malec, R. G. Gomez and R. A. Llosa, Food Chem., 1992, 44(5), 363–365 CrossRef CAS.
- A. Lobley, L. Whitmore and B. A. Wallace, Bioinformatics, 2002, 18, 211–212 CrossRef CAS PubMed.
- L. Whitmore and B. A. Wallace, Nucleic Acids Res., 2004, 32, W668–W673 CrossRef CAS PubMed.
- P. Siddhuraju, Food Chem., 2006, 99, 149–157 CrossRef CAS PubMed.
- A. Yildirim, A. Mavi and A. A. Kara, J. Agric. Food Chem., 2001, 49, 4083–4089 CrossRef CAS PubMed.
- X. Wang, F. Liu, L. Liu, Z. Wei, F. Yuan and Y. Gao, Food Chem., 2015, 173, 564–568 CrossRef CAS PubMed.
- J. Zhao, J. Xiang, T. Wei, F. Yuan and Y. Gao, Food Res. Int., 2014, 66, 216–227 CrossRef CAS PubMed.
- Y. Yuan, Y. Gao, J. Zhao and L. Mao, Food Res. Int., 2008, 41, 61–68 CrossRef CAS PubMed.
- J. S. Kim and Y. S. Lee, Food Chem., 2009, 116, 846–853 CrossRef CAS PubMed.
- M. A. Sabir, F. W. Sosulski and A. J. Finlayson, J. Agric. Food Chem., 1974, 22, 575–578 CrossRef CAS.
- M. J. Spotti, M. J. Perduca, A. Piagentini, L. G. Santiago, A. C. Rubiolo and C. R. Carrara, Food Hydrocolloids, 2013, 31, 26–32 CrossRef CAS PubMed.
- S. J. Lahtinen, K. Knoblock, A. Drakoularakou, M. Jacob, J. Stowell, G. R. Gibson and A. C. Ouwehand, Biosci., Biotechnol., Biochem., 2010, 74, 2016–2021 CrossRef CAS PubMed.
- P. Rizzarelli and S. Carroccio, Anal. Chim. Acta, 2014, 808, 18–43 CrossRef CAS PubMed.
- O. Almrhag, P. George, A. Bannikova, L. Katopo, D. Chaudhary and S. Kasapis, Food Chem., 2012, 134, 1938–1946 CrossRef CAS PubMed.
- H. Wang, Y. Shi and G. Le, Carbohydr. Polym., 2014, 113, 225–230 CrossRef CAS PubMed.
- D. Laroque, C. Inisan, C. Berger, É. Vouland, L. Dufossé and F. Guérard, Food Chem., 2008, 111, 1032–1042 CrossRef CAS PubMed.
- L. Jiménez-Castaño, M. Villamiel and R. López-Fandiño, Food Hydrocolloids, 2007, 21, 433–443 CrossRef PubMed.
- S. V. Prigent, A. G. Voragen, F. Li, A. J. Visser, G. A. van Koningsveld and H. Gruppen, J. Sci. Food Agric., 2008, 88, 1748–1754 CrossRef CAS PubMed.
- M. M. Hashemi, M. Aminlari and M. Moosavinasab, LWT--Food Sci. Technol., 2014, 57, 594–602 CrossRef CAS PubMed.
- W. Q. Wang, Y. H. Bao and Y. Chen, Food Chem., 2013, 139, 355–361 CrossRef CAS PubMed.
- D. Bhattacharya, S. K. Sahu, I. Banerjee, M. Das, D. Mishra, T. K. Maiti and P. Pramanik, J. Nanopart. Res., 2011, 13, 4173–4188 CrossRef CAS.
- F. L. Gu, J. M. Kim, S. Abbas, X. M. Zhang, S. Q. Xia and Z. X. Chen, Food Chem., 2010, 120, 505–511 CrossRef CAS PubMed.
- K. X. Zhu, X. H. Sun, Z. C. Chen, W. Peng, H. F. Qian and H. M. Zhou, Food Chem., 2010, 123, 1163–1169 CrossRef CAS PubMed.
- C. Yin, L. Yang, H. Zhao and C. P. Li, Food Res. Int., 2014, 64, 855–863 CrossRef CAS PubMed.
- Y. Sun, S. Hayakawa and K. Izumori, J. Agric. Food Chem., 2004, 52, 1293–1299 CrossRef CAS PubMed.
- Y. Liu, G. Zhao, M. Zhao, J. Ren and B. Yang, Food Chem., 2012, 131, 901–906 CrossRef CAS PubMed.
- C. Mangavel, J. Barbot, Y. Popineau and J. Guéguen, J. Agric. Food Chem., 2001, 49, 867–872 CrossRef CAS PubMed.
- W. Yang, F. Liu, C. Xu, F. Yuan and Y. Gao, Food Res. Int., 2014, 64, 141–149 CrossRef CAS PubMed.
- S. Xia, Y. Li, Q. Xia, X. Zhang and Q. Huang, Food Hydrocolloids, 2015, 43, 228–235 CrossRef CAS PubMed.
- C. Bengoechea, I. Peinado and D. J. McClements, Food Hydrocolloids, 2011, 25, 1354–1360 CrossRef CAS PubMed.
- X. Miao, L. Ling and X. Shuai, Chem. Commun., 2011, 47, 4192–4194 RSC.
- J. M. Silván, J. van de Lagemaat, A. Olano and M. D. del Castillo, J. Pharm. Biomed. Anal., 2006, 41, 1543–1551 CrossRef PubMed.
- S. Rohn, H. M. Rawel and J. Kroll, J. Agric. Food Chem., 2004, 52, 4725–4729 CrossRef CAS PubMed.
- M. Y. Shon, T. H. Kim and N. J. Sung, Food Chem., 2003, 82, 593–597 CrossRef CAS.
- D. Zhu, S. Damodaran and J. A. Lucey, J. Agric. Food Chem., 2010, 58, 2988–2994 CrossRef CAS PubMed.
- F. Lei, F. Liu, F. Yuan and Y. Gao, Food Hydrocolloids, 2014, 39, 163–170 CrossRef CAS PubMed.
- F. Liu, D. Wang, C. Sun and Y. Gao, Food Hydrocolloids, 2016, 52, 661–669 CrossRef CAS PubMed.
- S. Drusch, S. Berg, M. Scampicchio, Y. Serfert, V. Somoza and S. Mannino, Food Hydrocolloids, 2009, 23, 942–948 CrossRef CAS PubMed.
|
This journal is © The Royal Society of Chemistry 2015 |