DOI:
10.1039/C5RA06754E
(Review Article)
RSC Adv., 2015,
5, 58371-58392
Methods and approaches of utilizing ionic liquids as gas sensing materials
Received
15th April 2015
, Accepted 15th June 2015
First published on 16th June 2015
Abstract
Gas monitoring is of increasing significance for a broad range of applications in the fields of environmental and civil infrastructures, climate and energy, health and safety, industry and commerce. Even though there are many gas detection devices and systems available, the increasing needs for better detection technologies that not only satisfy the high analytical standards but also meet additional device requirements (e.g., being robust to survive under field conditions, low cost, small, smart, more mobile), demand continuous efforts in developing new methods and approaches for gas detection. Ionic Liquids (ILs) have attracted a tremendous interest as potential sensing materials for the gas sensor development. Being composed entirely of ions and with a broad structural and functional diversity, i.e., bifunctional (organic/inorganic), biphasic (solid/liquid) and dual-property (solvent/electrolyte), they have the complementing attributes and the required variability to allow a systematic design process across many sensing components to enhance sensing capability especially for miniaturized sensor system implementation. The emphasis of this review is to describe molecular design and control of IL interface materials to provide selective and reproducible response and to synergistically integrate IL sensing materials with low cost and low power electrochemical, piezoelectric/QCM and optical transducers to address many gas detection challenges (e.g., sensitivity, selectivity, reproducibility, speed, stability, cost, sensor miniaturization, and robustness). We further show examples to justify the importance of understanding the mechanisms and principles of physicochemical and electrochemical reactions in ILs and then link those concepts to developing new sensing methods and approaches. By doing this, we hope to stimulate further research towards the fundamental understanding of the sensing mechanisms and new sensor system development and integration, using simple sensing designs and flexible sensor structures both in terms of scientific operation and user interface that can be miniaturized and interfaced with modern wireless monitoring technologies to achieve specifications heretofore unavailable on current markets for the next generation of gas sensor applications.
| Abdul Rehman received his PhD in Analytical and Food Chemistry (University of Vienna, Austria) under the supervision of Professor Franz Dickert. Afterwards, he worked as a postdoctoral associate first in Professor Mark Meyerhoff’s group (University of Michigan, Ann Arbor) researching on biocompatible polymers for in vivo blood sensors and devices, and then in Professor Xiangqun Zeng’s group (Oakland University) investigating the integration of various sensor platforms for critical real world applications. He also worked as a visiting assistant professor at Oakland University and recently joined the KFUPM as a faculty member. His research interests include material/interface designs for various sensing protocols, related data analysis algorithms, and the integration into prototypes and devices. |
| Xiangqun Zeng received her PhD in Electrochemistry and Surface Chemistry (State University of New York, Buffalo) in 1997 under the supervision of Professor Stanley Bruckenstein and worked in the same lab for one year as a postdoctoral associate. She worked at the University of Wisconsin, Oshkosh, as an assistant professor (1998–2001) and then moved to Oakland University where she is a full professor now. Her lab is interested in studying fundamental and applied interfacial phenomena, particularly the design and control of molecular characters and the characterization of dynamic reactions at electrode interfaces for chemical and biosensor applications. |
1. Introduction
Monitoring concentrations of different gases and gathering detailed chemical information about local environments is critical for a lot of applications, both in the industrial world and in our daily lives.1–4 In general, these measurements are needed for monitoring health and safety hazards5–11 (e.g., detection of toxic and explosive gases), process controls12–14 (e.g., industrial combustion processes), environmental monitoring15,16 (e.g., automobiles and in-home air quality), or forensics and medical diagnostics17–19 (e.g., breath analysis for disease detection). For all these scenarios, in order to have any practical value, the desired chemical information needs to be acquired on-site and in real-time without having delays associated with transferring samples to centralized laboratories. Moreover, as continuous monitoring is an essential requirement, the concerns of cost-effectiveness and user-friendliness hinder the use of high-tech analytical instruments (e.g., GC-MS) for such applications. This situation has attracted an appreciable number of research and development activities in the field of gas sensors3,20–23 with demands for reliability and robustness of the proposed systems. As a result of these explorations, many implementable sensors24 for gases such as CO2, O2, water vapors, and VOCs are already available. However, these sensors are particularly useful for detections in controlled environments with relatively few interfering species. For more diverse and dynamic environments (e.g., an airport security checkpoint with a multitude of vapors in the air), we need much more precise and reliable gas sensors in order to meet the always emerging requirements in such complex environments.
In the context of this distinctive increasing demand for time-efficiently monitoring various gaseous components, the gas sensing market is expanding into a number of areas related to human healthcare and environment, security and sustainable economy. However, the technological priorities have remained associated with cost and reliability, which should be the salient features of any prototype development activity. The gas sensing market is estimated to be $6.3 billion with a 10% growth rate for new technologies. The major share of this market is focused on the development of gas sensing instruments and new or improved gas sensors, as indicated in Fig. 1. A lesser growth rate in existing technologies (i.e., 4%) is representative of the challenges that this market is facing in terms of operation in complex backgrounds, the ability to identify abnormal variations, and most importantly, the stability of the sensing materials. Moreover, the revenue generated by portable instruments is 70.2% which continues to increase in comparison to the fixed ones having a revenue of 29.8%. Hence, the motivation in the present research directions is to move towards sensors that have the potential to offer advantages over existing sensors for volume, cost, compactness, power consumption and responsiveness, and thus have the potential to open up new application areas, displacing existing technologies or creating new markets. The feasibility of displacing existing sensor devices and systems, however, depends on the balance between investment costs and performance or functionality gains.
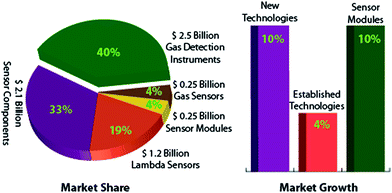 |
| Fig. 1 Fragmentation of the gas sensing market in terms of the generated revenue and expected growth for different technologies. | |
1.1 Analytical challenge
As described earlier, there is broad diversity in the analytical needs in terms of species and concentrations of the gaseous analytes under different environmental conditions. Our environment is inherently made up of gases which are roughly 78% N2, 21% O2, and 1% other trace gases including CO2. The detection and monitoring of these gases is important for many environmental standardization issues. Additionally, the anthropogenic processes have led to a multitude of modifications to this natural combination, and the new compositions can have detrimental effects on the overall quality of the resulting atmosphere. The most important alteration sources for such adverse effects include: (i) industrial processes generating toxic gases such as NOx, CO, CO2, SO2, NH3, and phosphene; (ii) domestic and household emissions of VOCs, e.g., acetone, benzene, methanol, phenol, methylene chloride, and chloroform; (iii) greenhouse gases coming both from natural (e.g., wetlands, termites, and the oceans) and human sources (e.g., landfills, livestock farming, as well as the production, transportation and use of fossil fuels for energy) causing methane and CO2 emissions; and (iv) chemical warfare agents like mustard gas, and other volatile explosives. Moreover, there is an additional need for monitoring localized gas environments for medical diagnostic and personal safety purposes. This diversity of needs and the associated challenges are precisely summarized in Table 1. Besides this diversity in a variety of analytes, for many of these applications the measurements have to cover a broad range of their regulated exposure limits spanning several orders of concentration magnitudes whilst being different for different analytes. This diversity in both the type and concentration of the target analytes is crucial for any further implementable action. Further complicating the situation are the chemical and physical interference parameters, the most abundant and most detrimental of which for the sensor performance are humidity and temperature. At room temperature and a 100% relative humidity (RH), the water concentration is ∼30
000 ppm which implies that a 100 ppm detection capability of a sensor has to overcome 300 times of humidity overload in terms of interference.25 Many other chemical interferents such as industrial solvents and household vaporizable chemicals further add to that interference-overload on the sensing capability of gas sensors.26 Therefore, system must have the ability to withstand all these detection challenges, besides satisfying the analytical requirements of the sensor performance in terms of sensitivity, selectivity, stability, reproducibility, response time, and the cost. In order to address the requirements of an enhanced analytical performance, a logical direction could be the development of new and smart materials in which the mechanisms of detection are supportive towards more and more specificity. However, these materials still need to be generic in terms of their applications, for being utilizable in multi-analyte detections, and multi-transduction modes.
Table 1 Summary of different areas of gas sensing with the target gases in each area and related challenges for the sensor devices
Area of application |
Sensing categories |
Gas targets |
Market barriers |
Atmospheric gases |
Environmental standardization |
CO2, O2, CO, N2 |
Long term operation, interference issues |
Greenhouse gases |
Health and safety standards for environment and workplace |
CO2, CH4 |
Multi-sensor technology required for long term operation, and tackle interference issues |
Industrial safety & industrial process control |
Flammable gas leaks |
HCs (CH4) |
Low concentrations, reliability and stability demands |
Toxic gases |
VOCs, SO2, NOx |
Specificity |
Energy fuel |
H2 |
Selectivity |
Asphyxiates and oxygen |
O2, CO2, N2, He |
Energy costs due to continuous operation |
Food quality and storage |
Amines, ethylene, alcohols, food aromas |
Matrix complexity |
Bioreactors and landfill |
O2, NH3, CO2, CH4, H2, VOCs, acetate vapors |
Multi-sensor technology required |
Domestic & household emissions |
Cabin air quality |
CO, VOCs |
Cost, lack of awareness |
Exhaust gases |
HCs, O2, CO, CO2, NOx |
Extreme environment workability, cost |
BTEX |
Benzene, toluene, ethylbenzene, xylene |
Versatile detection demands for each target |
Outdoor emissions |
CO2, NOx |
Cost and size |
Indoor air quality |
O3, NOx, formaldehyde, cleaning solvents |
Long term operation, reliability |
Refrigerants and intensive farming |
NH3, CFCs |
Cost, difficult temperature environment |
Asthma at home |
O3, NOx, bioparticulates |
No scheme available for bioparticles, cost and prevalence |
Nuisance odors |
VOCs, CH4 |
Reliability and stability |
Homeland security |
Explosives, chemical and biological attack |
TATP, HMTD, nitro-explosives, bio-hazardous chemicals |
Small size and efficient sampling required, selectivity to avoid false alarms |
Medical diagnostics & personal safety |
Domestic CO |
CO, O2 |
Cost, self-validation |
Fire and flammables |
CH4, NOX, SOX, ethylene, acetylene, HCl, Cl2 |
Identification of targets |
Breath and gut analysis |
CO2, VOC (e.g., ethane) |
Lack of comprehensive studies for marker specificity |
1.2 Goals and scope of this review
Due to the challenges described above, gas sensing technologies focusing on sensing materials, sensing mechanisms, sensing transducers, and sensing devices is a hot topic, thereby producing several reviews on the subject every year. These reviews particularly cover the sensing designs,27 integration into miniaturized and wireless sensing platforms,4 and the diversity in sensing materials (e.g., polymers, nanomaterials, and metal oxides).3,23,28,29 Sensing materials are very important since the choices of the sensing material often determine the sensing mechanisms, transducers and the ultimate design of the sensing devices. Ionic liquids (ILs) are one of the interesting materials that attracted significant recent research attention in many fields of science, and particularly in sensor science because of their diversified nature. We have recently reviewed their overall sensing capabilities as well as their contribution in robust chemical sensor developments.30 Despite these efforts, we believe that there is a need to comprehensively review the mechanistic understanding of these new materials that can lead to new gas sensing principles and protocols. Thus, this review is covering (i) the analytical needs of gas sensing technologies; (ii) gas sensing transducers and protocols when an IL is used as a component for sensing; (iii) sensing mechanisms based on IL materials with particular emphasis on the methodologies that provide a selective and reproducible response without the use of arrays; and (iv) finally commenting on orthogonal sensing based on multi-transducers with multiple response mechanisms. By discussing this subject matter, the goal is to stimulate research that can link the principles and mechanisms of sensing using ILs. In principle, the approach should be to properly combine the sensor components (i.e., sensing material, transducer, and signal generation/processing). Furthermore, the sensing criteria have to be carefully devised to include target concentration ranges, interferences, physical parameters, and sensor reliability. Another goal is to highlight the achievements in the field, demonstrate possible applications, and critically analyze the non-satisfactory performance and possible solutions.
2. Gas sensing attributes of ionic liquids
ILs have several attributes that make them desirable for gas sensing applications. As shown in Fig. 2, most ILs have organic cations (e.g., imidazolium, pyridinium, pyrrolidinium, phosphonium, and ammonium) whereas the anions could be inorganic (e.g., Cl−, PF6−, and BF4−) as well as organic (e.g., trifluoromethylsulfonate [CF3SO3]−, bis(trifluoromethylsulfonyl)imide [(CF3SO2)2N]− (NTf2), and trifluoroethanoate [CF3CO2]−). Thus, they can be considered as bifunctional materials, in which both their organic and inorganic attributes can be exploited for new sensor designs. The relatively large size of one or both ions and low symmetry account for many unique properties of IL materials.31 For instance, ILs have a negligible vapor pressure, wide potential window, high thermal stability and high viscosity. These characteristics make them stable materials for sensor designs. Although they are liquid at room temperature, their high viscosities enable them to be casted on solid supports to form thin, reproducible films making them behave as solid polymer-like matrices. Therefore, they can be considered as biphasic materials, i.e., solid/liquid. Moreover, they show good conductivity which is desirable for electrochemical processes and they show high solubility for a broad range of gases and VOCs, enabling many solvation interactions of the target molecules to provide both sensitivity and selectivity. Thus, they are also dual-property, acting as electrolytes and solvents at the same time. Due to such duality in phase and function, there is a tremendous opportunity to design orthogonal sensors by integrating multimodal transduction mechanisms (e.g., electrochemical quartz crystal microbalance (EQCM) and opto-electrochemical sensors) to provide enhanced reliability and internal validation of the detection outcome. The remarkable dissolution properties for organic and inorganic substances enable analyte pre-concentration and sensitivity enhancement for the detection whereas the molecular non-covalent interactions between ILs and the analytes contribute by increasing reversibility. All these properties (e.g., melting point, dielectric constant, viscosity, polarity, and water miscibility) can be further tailored by combining different cations with suitable anions. Furthermore, their miscibility with other solvents can also be controlled by the variation of cations and anions. These characteristics thus make them novel solvents and electrolytes, holding great promise for many studies on gas sensing.
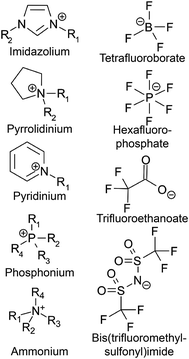 |
| Fig. 2 Names and structures of the most commonly employed cations and anions in different IL applications. | |
The use of ILs in different analytical applications is, however, determined by their intrinsic properties as well as the environmental circumstances of the targeted application. Water interference is the most important of these environmental factors32 that can drastically alter the physicochemical properties (e.g., viscosity, conductivity, and diffusivity) of ILs, consequently affecting the sensor responses. It has been shown that an increased water content of IL leads to a decrease in viscosity.33,34 Viscosity values are related to conductivity since a decrease in viscosity enhances the mass transport of the analyte to the electrode surface.35 A decrease in viscosity and subsequent increase in conductivity may appear to be advantageous. However, an increase in water content results in narrowing the electrochemical window of an IL.32,34 The narrowing of the electrochemical window occurs for both the cathodic and anodic limits which is likely due to water electrolysis.36 With this perspective, studies have been carried out to examine which ILs have the greatest water uptake, and hydrophobic anions that are immiscible with water have been identified.37 By selecting these hydrophobic IL coatings, the potential interference of water can be avoided. For electrochemical processes, proton donation from water could modify the redox mechanisms (e.g., O2 to superoxide) and affect the peak currents and peak positions. By selecting those redox processes for quantification where protons have no influence will be simplest to address water interference. Furthermore, the interplay between water and the redox analytes can be used to calibrate the water interference. Alternatively, trace amounts of water present in ILs enable some beneficial aqueous redox reactions of some gaseous analytes to occur at controlled rates, which are being explored in our lab to develop new IL based gas sensors. In this way, water is transforming from its status of interfering species to a valuable reactant, and thus IL-based gas sensing is moving towards the goal of real world sensing.
One important feature of ILs is that the combination of a variety of cations and anions provides an enormous number of ILs along with the possibility of facile custom synthesis. It is estimated that there could be up to 1018 ILs available.38 Thus, there is a large pool of possible ILs, from which ILs can be designed or selected for specific applications. Additionally, ILs are excellent solvents allowing them to be incorporated into conventional gas sensing matrixes, such as polymers, biopolymers, sol–gels, carbon nanotubes (CNTs), and metal nanoparticles,39–41 to form stable composite materials. These composite materials merge the multifunctional properties of both constituents involved in preparing the composites. For example, an IL/CNT based composite, with highly electroactive nanotubes and a liquid electrolyte, can be utilized for a wide variety of electrochemical gas sensing applications.
3. Gas sensor designs and developments
A gas sensor is defined as a miniaturized analytical device that can quantitatively determine the concentration of a family of gases or a specific gas in a given environment. The sensor typically relies on one or more sensing mechanisms to produce a signal that detects the presence and/or concentration of the target gas. The sensor system typically refers to a device that integrates the sensor detection with associated electronics and readout devices (e.g., transducers) allowing a user to directly obtain information on the analyte. As depicted in Fig. 3, a typical gas sensor comprises three major components: (i) a transducer that converts the presence of a gas analyte to some form of readable signal, (ii) a sensing film which is usually deposited onto the transducer allowing the detection and quantification of the gas analyte through a specific interaction mechanism, and (iii) signal processors and readout devices (e.g., data acquisition, lodging, and a processing unit) that can convert the sensor’s raw signal to usable information. Many amperometric gas sensors also use a permselective membrane in the sensor design. The membrane is located in closest proximity to the working electrode having the property of being highly permeable to the gas analyte but not to the other interfering electroactive species. Numerous research activities have been devoted to the data processing part of the sensor system.42,43 Besides, the engineering aspects of remote sensing44 and the energy harvesting processes45,46 for the automated sensor system also attract significant attention from scientists and engineers. Sensor calibration is another important area of research, but to keep the chemistry focus of this review article, we will concentrate on the first two components, i.e., the transducer and the sensing material with sensing/interaction mechanisms. The transducer can be a separate component or the sensing material by itself can act as the transducer. However, this is the component that has to detect the change in the local micro-environment (at the interface or in the bulk of the sensing film) as a result of change in target concentrations in the macro-environment of the sensor system. Thus, it deserves the central position in the development of any sensor application. On the other hand, the material component of the sensor most often takes up the responsibility of providing the required sensitivity, selectivity, speed and reproducibility. Therefore, this area of sensor research has been explored the most. In the following sections, the mechanisms using different transducers (methods) and the designs of different sensing materials/interaction mechanisms have been described, in particular in relation to IL-based gas sensing.
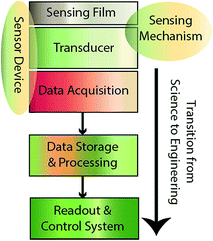 |
| Fig. 3 System layers of a typical gas sensing device showing the transition from science to engineering protocols. | |
4. Transducers for IL based gas sensing
ILs’ unique bifunctional (organic/inorganic), biphasic (solid/liquid), and dual-property (solvent/electrolyte) nature offers a broad range of sensing transducers to be employed in IL-based gas sensing. Among them, electrochemical transducers, thickness shear mode (TSM) resonators (i.e., quartz crystal microbalance (QCM)), surface acoustic wave (SAW) resonators, and optical transducers are the most explored. The rationalization behind the choice of transducer is directly related to the distinctive properties of ILs, as shown in Fig. 4. The dissolution power, weaker van der Waals interactions with the analyte and solid/liquid biphases of ILs are supportive towards the adsorption or dissolution of analytes on/into the ILs thin films and can be utilized for acoustic transducers. The bifunctionality of ILs as solvent and electrolyte leverages the design of membrane-free electrochemical sensors. On the other hand, the ease of incorporating task specific functions and solubility for both organic and inorganic compounds enables a wide range of optical sensing approaches to be designed and implemented. The selection and relative performance of the transducers for any particular detection application require the synergistic coupling of the intrinsic properties of the specific transducer (e.g., transducing or response mechanisms) with the chemical and/or physical properties of the sensing material which often determines the sensitivity, stability and dynamic range of the response in varying detection environments. Finally, the sensor fabrication, sensor manufacturing process, and readout are also important to be integrated into the overall sensor engineering process.
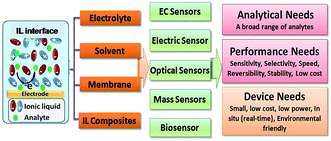 |
| Fig. 4 Demonstration of the IL interface and the links between its multiphasic character for various transducers and sensor developments thereby addressing numerous challenges. | |
4.1 Electrochemical transducers
The common electrochemical methods including potentiometry, voltammetry and impedance spectroscopy have all been applied in the IL-based sensor development. Among them, voltammetry based on the measurement of a redox current and impedance spectroscopy based on the measurement of the impedance/capacitance at the electrode/electrolyte interface, are especially suitable for the IL-based gas sensor development. Both methods require the use of electrolytes. Notably, a majority of the electrochemical gas sensors developed to date employ aqueous-based electrolytes. Another type of electrolyte is the non-aqueous electrolyte (e.g., salts that could be dissolved in organic solvents such as lithium perchlorate, organic compounds with a charged ion center such as tetra-n-butylammonium perchlorate, or polyelectrolytes such as perfluorosulfonate47,48 and Nafion ionomers).49,50 In contrast to battery research, such as on Li ion batteries, which can be designed as a completely closed system, the electrochemical sensor cell cannot be completely sealed, as it needs to be accessible to the ambient environment to detect the gaseous molecules. Aqueous electrolytes and most traditional non-aqueous electrolytes suffer from solvent volatility leading to the exhaustion of solvent in the electrochemical cells. Because of their non-volatility and much higher ionic conductivity, ILs have the ability to replace almost, if not all, conventional electrolytes in many types of electrochemical sensors.1,30,51–53 IL-based electrochemical sensors7,30,52–57 are also well suited for miniaturization and can be fabricated with very low cost. Different from adsorption based transducers such as a surface acoustic wave devices, arrays of amperometric and impedance transducers allow a secondary perturbation (e.g., potential) that enhances the selectivity and increases the analytical information content without increasing the number of physical sensor elements. However, the challenge is still the same, to achieve better sensing performance parameters, i.e., sensitivity, selectivity, reproducibility, response time, and cost. Among these parameters, the cost is often taken care of by employing low cost, low power transducers and by miniaturization. Sensitivity and selectivity are extremely important, however, these are mostly dealt with at the material selection and design stage of the development. A significant number of successful sensors in the lab phase fail to be implemented in the real world because of reproducibility issues, and thus the reproducibility has to be treated as a most important parameter in any sensor development. The response time is critical too, in order to achieve the appropriate action in real time. In the following, we will discuss different IL sensing approaches addressing these challenges.
4.1.1 IL gas sensing based on voltammetry. Voltammetry is an electrochemical method that provides qualitative and quantitative information on the analytes, obtained by measuring the current as a function of the applied potential. When the current is monitored at a fixed potential, the method is often called amperometry. In experiments where ohmic drop (iR drop) may be high, an amperometric gas sensor typically consists of working, reference and counter electrodes connected through an electrolyte (the desired redox reaction occurs at the working electrode, the counter electrode establishes the current path, and the reference electrode provides a stable reference electrode potential).A gas-permeable membrane is located proximal to the working electrode. The gas analyte passes through the membrane, and is detected at the working electrode–electrolyte interface. For amperometric sensors, it is not necessary to use a conventional three-electrode system if the currents encountered are in the nA range or lower. Amperometry can be used to detect those gas species that can undergo redox transitions at the working electrode/electrolyte interface in an electrochemical cell. Most commercially available amperometric sensors for gas detection (e.g., of O2, CO, SO2, H2S, NO2, and Cl2) currently employ conventional solvents (e.g., H2SO4–H2O mixtures, or organic solvents such as acetonitrile or propylene carbonate) which cannot survive drastic temperature changes or extremely dry or humid conditions. The “lifetime” of a sensor is often determined by how quickly the electrolyte dries up and the solvent often has to be replaced every few days under the most extreme conditions. Another often overlooked problem is the reference electrode. In an aqueous system, a Ag/AgCl reference electrode is often used but a number of species in an aqueous sample can dissolve the AgCl deposit off the electrode by forming soluble Ag(I) complexes. In ILs, a quasi-reference electrode such as Ag or Pt wire is often used. They are stable in ILs and are shown to maintain a stable reference electrode potential avoiding the problems of traditional reference electrodes (e.g., Ag/AgCl, and Hg2Cl2/Hg). ILs possess negligible volatility and a high chemical stability, making them desired electrolytic media in robust gas sensors for the potential application under more extreme operating conditions (e.g., at up to 300 °C), with little possibility of solvent evaporation or degradation. These unique properties of ILs, combined with the intrinsic conductivity (no need for a supporting electrolyte), wide potential window (to investigate compounds that are not combinable with amperometric detection otherwise, due to the absence of redox activity in conventional electrolytes) and in some cases increased gas solubility in ILs, make them ideal electrolyte media for developing gas sensors. In genral, the redox reaction occurring at the electrode/electrolyte interface such as Ox + ne−→ Red, involve three major steps: (i) the mass transport step (diffusion, diffusion-convection, migration of Ox from the bulk solution to the electrode surface), (ii) the heterogeneous electron transfer (charge transfer) step at the electrode surface, and (iii) chemical reactions preceding or following the electron transfer. These could be homogeneous processes (e.g., chemical reactions in solution) or heterogeneous processes at the electrode surface (e.g., adsorption and desorption, nucleation, crystal growth, surface diffusion). ILs as sensing materials could affect all of these steps. In addition, for the sensor development, where ILs are used as both electrolytes and solvents, there is the requirement that the analytes need to be soluble in the ILs, and the ILs can facilitate redox mechanisms that are desirable for sensors. It is also important that a steady-state current can be obtained under constant analyte conditions. Since the magnitude of the current is often limited by the inherent sluggishness of one or more reactions called rate limiting steps, it is very important to understand the electrode/electrolyte interfacial processes, and understanding these processes will enable the selection of the electrode potential, current density, solvent, supporting electrolyte, solution pH, temperature, deliberate modifications of the electrode surface, addition of other reagents etc., to meet the requirements of a specific gas sensor application. Furthermore, for an electrochemical gas sensor to work under real world conditions and for miniaturized electrochemical gas sensors, it will be important to consider all the reactions in an electrochemical cell and to determine the rate limiting steps for the entire electrochemical cell reactions including those at the counter electrode.
4.1.2 Simplicity of redox reactions in ILs for gas sensing. The preferred mechanisms for sensing are reversible and simple redox reactions which allow simple sensor designs and enhance the reproducibility of the sensor performance. ILs have been shown to enable many reactions to be simple and reversible, which otherwise are complicated in conventional electrolytes. The oxygen reduction reaction (ORR) is a classical example in this regard and has been studied by many different groups.58–63 In ILs, ORR is a one-electron reversible reduction process (O2+ e− → O2˙−) instead of a complicated four electron reduction process in aqueous electrolytes (O2 + 4H+ + 4e− → 2H2O). Because of the simple and reversible one-electron reduction of oxygen to form superoxide radicals which are stable in several ILs, this reaction was demonstrated in the development of a robust oxygen gas sensor.53 Compared with a conventional Clark cell design based on an aqueous supporting electrolyte for oxygen detection, the IL-based sensor achieved substantial improvements in performance and stability. A limit of detection for oxygen as low as 0.05 vol
%, linearity over an oxygen partial pressure between 0% and 20%, and a steady-state response time of 2 min were demonstrated, with a stable analytical response shown over the examined period of 90 days with no obvious fouling of the electrode surface.
4.1.3 New redox reactions in ILs for gas sensing. Although, IL electrolytes provide partial selectivity, the primary selectivity of an IL-based voltammetric sensor comes from the redox properties of the analyte observed at the interface, whereby the electrical current is generated by the electron transfer reactions of an analyte at an electrode at a specific potential.2 It is known that many species have similar redox potentials in aqueous electrolytes and it is difficult to achieve absolute selectivity for an analyte detection using amperometric sensors. The unique properties of ILs enable many new electrode reactions to occur which can be explored to enhance the selectivity of IL-based electrochemical sensors. For example, we have shown a unique redox chemistry that occurs only at an IL–electrode interface and can be exploited to enhance the sensor performance, especially the sensitivity and selectivity.64 At a platinum electrode in NTf2-based ILs, methane is detected due to an electrooxidation process. Fig. 5 shows the amperometric response of a methane sensor in the IL [C4mpy][NTf2]. Methane can be oxidized in [C4mpy][NTf2] at a potential of ∼0.9 V and oxygen can be reduced to form a superoxide radical at −1.2 V. Both analytes can be selectively quantified by alternating the bias potential between 0.9 V to −1.2 V. Furthermore, the electrooxidation of methane produces CO which can be oxidized to CO2 by the superoxide radical when the oxygen reduction is included. In situ generated CO2 arising from the methane oxidation was shown to provide an excellent internal standard for the quantification of the electrochemical oxygen sensor signal. As the methane concentration is increased, the oxygen concentration decreases. Thus, the simultaneous quantification of both methane and oxygen in real time strengthens the reliability of the measurements by cross-validation of the two co-existing ambient gases within a single sample matrix and allows for the elimination of several types of random and systematic errors in the detection. Thus, in place of a sensor array, a single sensor can be employed for real time measurements. We have also validated this IL-based methane sensor employing both conventional solid macroelectrodes and flexible microfabricated electrodes using single- and double-potential step chronoamperometry with good sensing attributes such as high sensitivity, complete reversibility, long term stability (less than 0.3% potential shift over 90 days), rapid response and strong selectivity (selectivity coefficient ∼0.01% for CO2, NO, NO2, and SO2).
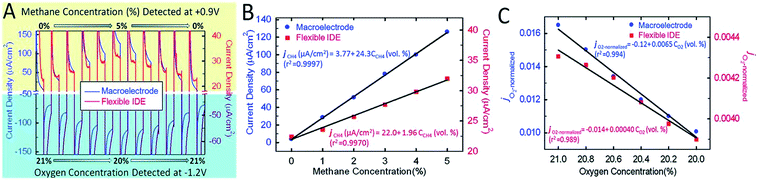 |
| Fig. 5 Response data of an IL sensor capable of detecting both oxygen and methane at two different bias potentials thereby showing a unique electrochemistry for IL systems. Data shown are (A) the real time response by varying the conditions, (B) the calibration curves for methane using a microelectrode and IDE, and (C) the calibration curves for oxygen using a microelectrode and IDE (ref. 64 – reproduced by permission of The Royal Society of Chemistry). | |
In another report,65 much more facile redox reactions have been identified for NOx for developing IL-based electrochemical sensors. Oxidation of NO2 occurred at less positive potentials in [C4mim][NTf2] and [C4mpy][NTf2] coated sensors than in conventional solvents. At the same time, oxidation of NO occurs roughly to the same degree and at almost the same anodic potential as that of NO2. Moreover, there was no significant difference between the peak potentials of NO2 and NO. These preliminary results obtained with this sensor proved that the oxidation reactions of NO2 and NO occur at very close potentials, thus allowing effective NOx determination with a single sensor. By contrast, other atmospheric components able to act as possible interfering species (CO, H2S, and SO2) were found to undergo oxidation only at much more positive potentials. Therefore, the sensor was able to detect NOx in a wide concentration range with reproducible and quantitative signals and a detection limit of 0.96 ppb v/v while having good selectivity. The reported IL NOx sensor is also suitable for measurements at reduced pressure and fairly high temperatures.
4.1.4 Multistep redox reactions in IL for gas sensing. In general, analytes with multi-step redox mechanisms are not desirable for the electrochemical sensor development due to the difficulties with quantifications based on a more complex sensor methodology. However, the unique redox chemistry in ILs provides a new possibility for the electrochemical sensor development, taking advantage of the redox reactions that have multiple step mechanisms. In such cases, the step that is most reversible is normally chosen for quantification purposes. This strategy can sometimes also be used to eliminate interference. For instance, an array of four IL-coated glassy carbon electrodes was characterized for the voltammetric detection of dinitrotoluene (DNT) and trinitrotoluene (TNT), and a correlation (with 100% classification accuracy) between the redox properties and the physicochemical parameters of the species involved was revealed (Fig. 6).66 Here, the interference of humidity was eliminated by calibrating with a redox process that does not involve protons, and thus a selective and quantitative response was obtained. The redox peak based on the redox reaction without the proton transfer thus had the most reversible character providing a more accurate quantification. Gas phase analysis showed strong redox signals for TNT and DNT and demonstrated that ILs serve as a preconcentrator to improve the sensitivity of very low vapor pressure analytes. In another report,67 the electrochemical behavior of highly toxic methylamine gas in several ILs has been investigated at a Pt microelectrode using CV. A broad oxidation wave at approximately 3.0 V, two reduction peaks, and another oxidation peak were observed indicating a complicated mechanism with ammonia as a likely by-product. The linear plots of the oxidation current vs. concentration however suggest that methylamine has a high solubility in ILs, thus generating a possibility to be detected using these media.
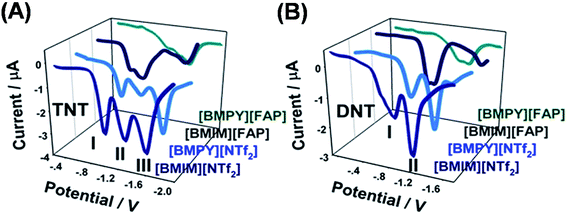 |
| Fig. 6 Square wave voltammetric response of 0.1 mM of TNT and DNT in four different ILs showing a multistep reaction, from which the most reproducible and interference-free peak can be chosen for quantification purposes. (Adapted with permission from ref. 66. Copyright (2012) American Chemical Society.) | |
The electrochemical reaction mechanisms of gases such as CO2, H2S, and Cl2 have been shown to be either irreversible or a multistep reaction. For example, the electrochemical reduction of CO2 in the IL [C4mim][Ac] was studied at a Pt microelectrode using cyclic voltammetry. CO2 undergoes a chemically irreversible one-electron reduction to the radical anion CO2˙− and subsequent follow-up chemistry including the interaction/complexation with IL. The behavior was found to be irreversible due to the strong absorption of CO2 in the IL, suggesting that this system may not be suitable for real-time sensing of CO2, however the high solubility (>1.5 M) of CO2 in [C4mim][Ac] suggests a method for sequestration of the greenhouse gas. We have also used voltammetry in ILs in combination with the QCM technique,68 proving that the CO2 reduction in ILs is irreversible and forms CO2˙− adsorbate at the electrode interface. In the presence of O2 and with increasing concentrations of CO2, the reduction of oxygen is switched from a one-electron process to an overall two-electron process, and forms adsorbed CO4˙− intermediate species. The mechanism of the reaction thus proposed is shown in Fig. 7. Such information about the mechanisms of the reaction is highly beneficial for the development of amperometric gas sensors. However, the most important consideration in such cases is that the products of the irreversible reaction do not poison the electrode or the electrolyte.
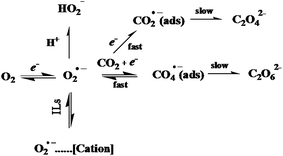 |
| Fig. 7 Mechanism of the reduction of CO2 in the presence of oxygen using IL electrolyte which can be supportive towards IL sensor developments. | |
4.1.5 Anomalous redox reactions in ILs. Studies focusing on understanding the reaction mechanisms and comparing the behavior to that in conventional electrolyte systems, have shown some notable differences and anomalous behavior in some IL electrolytes.69 It is extremely important to understand this anomalous behavior in ILs before they can be employed as direct replacements for conventional molecular solvents in sensor devices. Compton’s group,70 for example, has reported the two-electron reduction of 100% chlorine gas to chloride at a Pt microelectrode with a range of ILs ([C2mim][NTf2], [C4mim][NTf2], [C4mpy][NTf2], [C4mim][BF4], [C4mim][PF6], [C4mim][OTf], [N6,2,2,2][NTf2] and [C6mim][Cl]). The voltammetric behavior at various scan rates was highly unusual, with the limiting currents observed to decrease with increasing scan rates. This intriguing observation was assigned to a mechanism of adsorption of chlorine gas on the Pt electrode surface. The adsorbed chlorine itself cannot be reduced, but must undergo desorption before the electron transfer step. At slower scan rates with longer time scales, there is more desorption resulting in more surface available to chlorine for the electron-transfer reaction, thereby resulting in higher currents at slower scan rates. The large voltammetric currents observed suggest that Cl2 has a very high solubility (1–10 M) in ILs, making these solvents attractive for the purpose of Cl2 gas sensor development. The authors did not report the effect of varying concentrations of Cl2 on the current response, but this seems like the next logical step especially given the unusual adsorption mechanism. If the current changes linearly with concentration, ILs may prove to be a useful medium for robust Cl2 gas sensing.In a similar way, some notable differences were observed for the oxidation of toluene diisocyanate (2,4-TDI) in IL which occurred at a lower anodic potential than that in traditional acetonitrile-based electrolytes with no side reactions.71 In this case, the redox reaction is not that simple and reversible, yet the understanding of the redox chemistry thus revealed and the carefully chosen measurement conditions allowed the development of a robust amperometric sensor for 2,4-TDI detection. This was done by selecting the hydrophobic IL [C4mpy][NTf2] and by restricting the potential window to only include the oxidation processes. In this way, the sensor is capable to avoid the two most ubiquitous interferents under ambient conditions, i.e., humidity and oxygen, thereby enhancing the sensor performance and reliability for real world applications. 2,4-TDI detection was performed in both liquid and gas phases with detection limits of 9.32 ppm and 2.65 ppm, respectively. These values are comparable to the safety standards set by NIOSH. The so-developed sensor has a quick response time with good sensitivity and selectivity, and can be miniaturized for smart sensor protocols.
4.1.6 ILs as sensitivity enhancement components. The distinctive characteristics of the analyte redox properties in ILs were also demonstrated as a sensitivity-enhancing component of the amperometric sensor for the detection of ammonia by Ji et al.72 They observed that the electrochemical oxidation of ammonia in propylene carbonate was indirect, enabling the measurement of 10–100 ppm by measuring the peak current of the reaction between ammonia and hydroquinone as a function of the ammonia concentration, giving a sensitivity of 1.29 × 10−7 A ppm−1 and LOD of 5 ppm. On the other hand, the oxidation of ammonia has been shown to be direct in several ILs, suggesting that ammonia is initially oxidized to nitrogen and protons, which are transferred to an ammonia molecule, forming NH4+ via the protonation of the anion(s). NH4+ was then reduced at the electrode surface, forming hydrogen gas, which is then oxidized. In all the ILs studied, the cyclic voltammetry shows a broad oxidative peak, with two cathodic peaks and one anodic peak following the oxidation. The two cathodic peaks were identified as: (i) the reduction of the protonated anion(s), and (ii) the reduction of NH4+. The resultant anodic peak is likely associated with the oxidation of bulk hydrogen. Thus, the analytical ability of this sensor has been established showing that the limit of detection was about ten times better than that in propylene carbonate. Moreover, it is highlighted that ILs not only provide direct redox mechanisms for detection, but also appear to enhance the sensitivity and other analytical performance parameters by implementing these mechanisms.
4.1.7 Innovations in IL-based electrochemical gas sensor designs. ILs have a higher viscosity than those of most aqueous or non-aqueous electrolytes, which can result in a slow response time due to the slow mass transport processes in an electrochemical cell. Furthermore, the high viscosity of ILs often results in ion-pair formation which can result in a slow and less reproducible response.20 This process can limit their direct use in practical sensors because of the longer response time and lower sensitivity. Recent advances in amperometric gas sensors involving ILs has been reviewed by Rogers et al.,69 describing the electrochemistry of various gases including O2, CO2, H2, NH3, H2S, SO2, and NO2 in ILs, indicating that the viscosity can be the most important limiting factor in the sensor development. One approach to surpass this barrier is to employ thin IL layers, thus overcoming the slow diffusion often associated with viscous ILs. This may result in shorter response times, in the order of minutes or seconds, which is satisfactory for most gas sensor device requirements. Another approach to increase response speed and to circumvent the high viscosity of ILs is to explore new electrochemical sensor structures. We introduced a novel macro-electrochemical cell with a porous PTFE Teflon membrane (Fig. 8A) with the desired wettability of ILs that facilitates the diffusional transport through the pores and provides two levels of selectivity.53 Analytes entering from the back side were able to quickly reach the electrode/electrolyte interface without passing through the IL diffusion barrier, reducing the response time to mere seconds.53 To further reduce the response time, we designed a planar electrode (Fig. 9) with a structure joining the gas, liquid and solid phase at the site of the electrode. It features a micro-fabricated porous gold electrode on a porous PTFE thin film and an IL thin layer of [C4mpy][NTf2] as the electrolyte. [C4mpy][NTf2] has a high contact angle on the PTFE membrane so that the pores (typical diameter 10 μm) are slightly wetted by IL but it has a low contact angle on Au and wets well on Au, allowing the fast transport of gases to the electrode–electrolyte interface. In addition, variation of the IL structures can be used to fine-tune the sensor sensitivity. Fig. 8B shows the current responses for three NTf2-based ILs and illustrates a strong dependence of the signals on the choice of cation, for this particular application. High sensitivity, complete reversibility, long term stability (less than 0.3% potential shift over 90 days), rapid response and strong selectivity (selectivity coefficient ∼0.01% for CO2, NO, NO2, SO2) of this IL oxygen sensor were also the benefits of this new sensor design. As discussed in later sections, microelectrodes fabricated on a silicon chip can be another important advancement in circumventing the viscosity effect, also shown by Compton’s group.56 The use of small (micron-sized) working electrodes can minimize any iR/Ohmic drop limitations that are often associated with voltammetry in highly resistive ILs on larger electrodes (larger currents). To improve the current density and to overcome the iR/Ohmic drop at the interface, a common attribute in viscous and resistive ILs, small sized working electrodes and eventually “lab-on-a-chip” type systems are being investigated.21,73,74
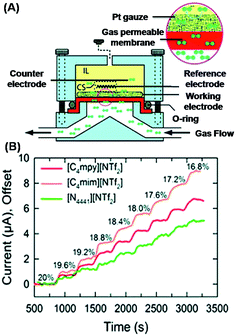 |
| Fig. 8 (A) Backflow sensor design allowing gas molecules to directly reach the interface without passing through a viscosity barrier. (B) Concentration-dependent electrochemical response for oxygen with three different IL systems. (Reprinted with permission from ref. 53. Copyright (2011) American Chemical Society.) | |
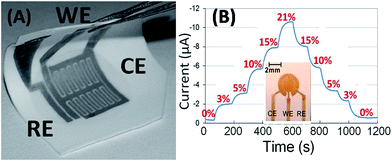 |
| Fig. 9 (A) Image of a flexible Pt-interdigited electrode (IDE) made on a Teflon gas-permeable membrane via micro-fabrication techniques; (B) amperometric sensing of oxygen using a micro-fabricated concentric ring disk Au-electrode (shown in the inset) on porous Teflon (ref. 64 – reproduced by permission of The Royal Society of Chemistry). | |
4.1.8 Innovations in electrode designs for IL gas sensing. ILs provide new opportunities not only for the electrochemical sensor design but also for miniaturization by innovations in the electrode geometry and substrate flexibility. Micro-fabrication technologies, in particular the thin film deposition of microelectrodes and formation of micro-fluidic channels, have been widely applied to analytical systems.75,76 Electrode arrays, both macro- and micro-scale, are now commonly fabricated using thin film metal deposition and photolithography.5,77–79 For example, Fig. 9 shows an interdigited electrode (IDE) fabricated using thin film deposition of both platinum and gold on porous Teflon which were used to obtain sensor data for the measurement of oxygen concentrations.64 These miniaturized sensors yield a similar sensitivity compared to results shown before for the oxygen detection.53 As the fabrication of these electrodes relies on photolithography, the size and geometry of the electrode can easily be varied to meet the needs of the sensor interfaces, and different electrode configurations can be utilized within the same array to improve the analytical performance.The IL-based methane sensor discussed earlier was also characterized by these microelectrodes and compared using both a conventional solid macroelectrode and a flexible planar microfabricated electrode, as shown in Fig. 5. Both single- and double-potential step chronoamperometry experiments were performed. As shown in Fig. 5, the flexible IDE electrode provided equally quantitative results but with a relatively lower sensitivity. This is likely due to the vapor deposited platinum having a lower catalytic activity than that of a solid platinum electrode. In both sensor configurations, we were able to obtain reversible signals with long term stability (less than 0.3% potential shift over 90 days), rapid response and good selectivity (selectivity coefficient ∼0.01% for CO2, NO, NO2, and SO2).
4.2 Impedance-based gas sensing approaches
Although the voltammetric approaches can be used for reproducible sensor developments, still in many cases it is not easy to establish a reversible or feasible reaction mechanism for the target analyte detection. The products from the redox reactions often generate changes at the electrode interfaces which can reduce the sensor lifetime. Electrochemical impedance spectroscopy (EIS) involves the application of a sinusoidal electrochemical perturbation (potential or current) over a wide range of frequencies, allowing the measurement of impedance changes in the form of double-layer capacitance and charge-transfer conductance which originate from the change of polarization of the IL–electrode interface. EIS is an alternative approach to promote reversible sensing mechanisms since there is no redox reaction, thereby making no product and avoiding any fouling or poisoning of the electrode or electrolyte. This mechanism is also non-invasive, providing better reversibility for sensing which has led to long sensor life times. Thus, EIS-based sensors not only provide orthogonal detection modes to amperometric sensors, but they also permit self-monitoring of the sensor’s stability and automated calibration for drift mechanisms. At room temperature, ILs have a high viscosity. As a consequence, the diffusion and conductivity of ions are normally lower in ILs than those in aqueous electrolyte solutions. Thus, they can be simultaneously regarded as a solid and liquid interface, making them ideally suited for applications that require a thin or intensely concentrated layer of ionic charge, such as the capacitance sensor development. Compared with molten salts at room temperature, the ions contained in ILs are often large, flexible, highly polarizable, and chemically complex with a number of interionic forces (such as dispersion forces, dipole–dipole interactions, hydrogen bonding, and pi-stacking forces) in addition to the Coulombic forces. Moreover, adsorption of anions and/or cations is likely to happen at the interfaces with ILs. Varying the applied potential can result in the rearrangement of adsorbed ions which allows the modification of the IL double layer capacitance. When exposed to an analyte, especially small gaseous molecules, the molecular interaction events that occur at the IL interface can lead to polarization reactions at the interface via redox reactions, as well as binding or dissolution of the analyte in ILs. For example, the analyte can displace or rearrange the IL order in the double layer toward a new orientation. Removing analyte allows the IL double layer to return to its original orientation. Based on these principles, an innovative capacitance sensor has been applied for methane detection,8 measuring the change of the surface charge at IL–electrified metal interfaces. The adsorption of anions and/or cations is likely to happen at the interfaces whereas the specific adsorption depends upon the chemistry of the ions and the applied potential. The IL [C4mpy][NTf2], which has a double layer structure favoring small gas molecule adsorption, can sensitively and selectively measure concentrations of CH4 (Fig. 10) using EIS with a −0.3 V DC bias. Fig. 10 also clearly shows that the sensor returns to its baseline value when the analyte is removed, demonstrating that the reaction is fully reversible with less than 0.1% drift. The selectivity comes from the unique highly ordered arrangement of ions in the innermost layer of the IL–electrode interface which is potential-dependent. The degree of the ordered structure at the IL–electrode interface can be tuned by the applied bias (e.g., −0.3 V for CH4) and by the unique molecular structure of the IL ions. The high viscosity of ILs that is usually considered a limitation to practical electrochemical applications due to the slow rate of mass transport, is an advantage in capacitance measurements80 due to a more ordered and concentrated double layer. The experimentally measured response time is often the sum of the gas sampling time related to the test set-up and the sensor response time. The overall response times t90 of NO2, CH4 and SO2 were only 5.1, 6.4 and 23.6 s, respectively, when tested using electrochemical impedance spectroscopy (EIS).
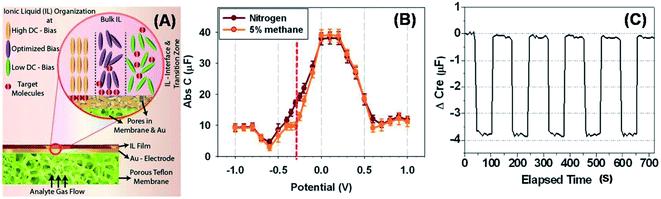 |
| Fig. 10 (A) Schematic diagram of the IL–electrified metal interface sensor response mechanism indicating that an optimized bias potential is required for the gases to adsorb at the interface. (B) Capacitance–potential curves for the exposure of nitrogen or 5% methane at a frequency of 1 Hz and a carefully chosen potential range (−0.8 V to 0.8 V) where no Faradaic processes exist for the gases tested. The response of the two gases is exactly similar except at a specific optimized DC bias (−0.3 V in this case) where the adsorption of methane occurs. (C) Capacitance measured over five cycles of alternate exposure to 5% methane and nitrogen (as the baseline gas) indicating that the response is fully reversible. (Reprinted with permission from ref. 8. Copyright [2013], The Electrochemical Society.) | |
4.3 Electrochemical gas sensing approaches based on disposable devices
Even if there is an unavailability of a simple and reversible redox reaction, the mechanism is too complex to identify one quantification parameter, or the reaction products are present which can hinder the reproducible use of the developed sensor, as might be the case with the above detailed methods, the IL materials still present an opportunity to design electrochemical gas sensors on disposable platforms due to the possibility of the low cost production of ILs at mass-scale. Although this opportunity is not usually conceived for solving the above problems, it rather, for a more critical need, enables the implementation of the electrochemical sensors in much more remote areas and third world countries where the availability of even the simplest technical equipment is scarce and the end users are limited in their experience. Moreover, such sensors can be cost efficient as well using inexpensive materials (e.g., paper) in the fabrication. ILs are also stable and relatively low cost when they are mass-produced. One of the first examples in this regard is a sensitive and fast-responding membrane free amperometric gas sensor consisting of a small filter paper foil soaked with an IL, with three electrodes screen-printed on it with carbon ink.21 By carefully controlling the preparation procedure, a very close contact between IL and the carbon electrode is achieved, allowing gaseous analytes to undergo charge transfer as soon as they reach the electrode. Thus, the adverse effect on recorded currents of slow steps such as analyte diffusion and dissolution in a solvent is avoided. To evaluate the performance of this device, 1-butanethiol vapors were adopted as the model analyte. The results obtained were quite remarkable for a disposable sensor (detection limit: 0.5 μM, dynamic range: 2–200 μM, both referring to solution concentrations; correlation coefficient: 0.998, repeatability: ±7% RSD, and long-term stability: 9%). A similar strategy is utilized by this group for the detection of acidic vapors such as phenol as model analytes,74 but with a shift towards task specificity in the IL selection. This time, the inexpensive paper support was soaked by an acetate anion based IL, thus having a basic character. The results obtained showed that the detection of these acidic analytes can be performed at a significantly lowered oxidation potential which otherwise is quite high for the selected target. In this way, overlap with the medium discharge is avoided, and the possible adverse effects of interfering species are minimized. The sensor performance was also comparable to that in a previous report by the same group. Toniolo et al.81 also reported a paper-supported amperometric sniffer for monitoring volatile amines (VAs) released from fish samples, in order to gain indication of their state of turning spoiled. The performance of this sensor was preliminarily tested on synthetic samples of trimethylamine (TMA), dimethylamine (DMA), methylamine (MA) and ammonia (i.e., the main species responsible for the typical flavor of spoiled fish), detecting only TMA, DMA and MA without interference from NH3 whose oxidation occurred beyond the solvent discharge. This detection of sole TMA, DMA and MA as a whole turned out to be well suited for the rapid assessment of fish spoilage, since during storage the enhanced release of these amines is largely predominant over that of NH3. This approach was then applied to the detection of VAs released from real fish samples with a substantially satisfactory agreement with data obtained by routinely adapted approaches.
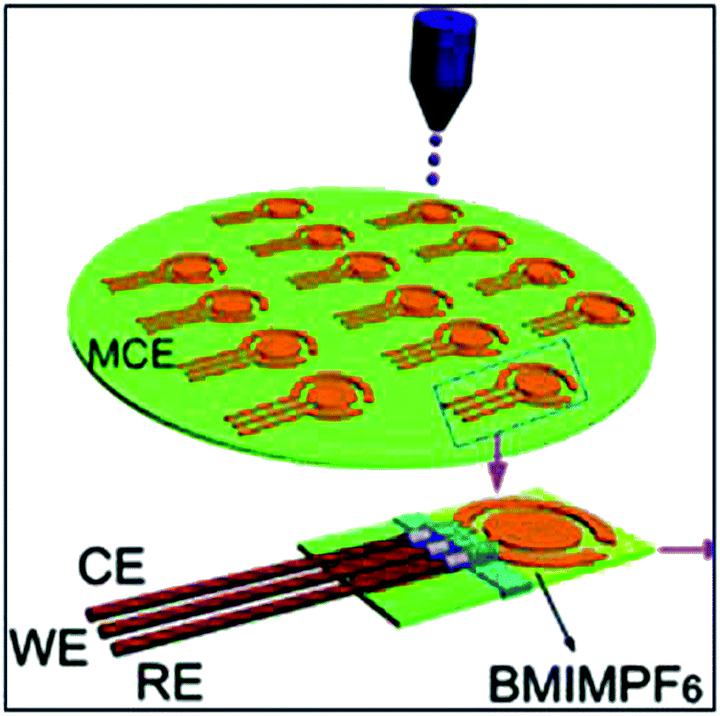 |
| Fig. 11 An example of using IL systems and disposable electrodes made on: a cellulose membrane by inkjet-printing of gold nanoparticles. (Reprinted with permission from ref. 73. Copyright (2012) American Chemical Society.) | |
Another step towards disposable sensors is to utilize commercially available electrodes and sensors which can be cost effective using batch production technologies. This has been done by Gebicki et al.82 for the detection of VOCs using different ILs as the electrolyte (e.g., [C4mim][N(CN)2], [C8mim][BF4], and [C4mim][NTf2]). The sensors implemented for this study were designed by the DropSens Company in Spain in the form of stripes with the electrodes screen printed on a ceramic substrate. The working and counter electrodes were made of a deposited gold layer, whereas the reference electrode was made of a deposited silver layer. The presented prototype for measuring benzaldehyde, methyl benzoate and acetophenone in an argon atmosphere was characterized by a sensitivity from 71 to 191 nA ppm−1, whereas the limit of detection of these analytes was at a level of 1.4–5.7 ppm (v/v). However, these parameters again depended on the analyte measured as well as on the electrolyte employed, confirming the previously obtained results. The developed prototype was also shown to have a good reproducible character as the sensor was operated for 2 months yielding a relative standard deviation below 8%. This indicates that even the commercial sensors can be employed for obtaining an enhanced performance if stable and reproducible redox chemistry has been identified and IL materials are utilized. In order to mass-produce such sensors, a simple approach of inkjet-printing gold nanoparticle (GNP) patterns with the self-catalytic growth of these patterns into conducting layers on cellulose membranes, has been introduced73 and presented for electrochemical sensing of oxygen using IL electrolytes. Using this approach, hundreds of self-designed gold arrays can be fabricated in hours using an inexpensive inkjet printer. The resulting paper-based gold electrode arrays have several unique properties as thin-film sensor platforms. The porous nature of these new electrodes also allowed the addition of electrolytes and analytes from the back side of the cellulose membrane and controllably produced large three-phase electrolyte/electrode/gas interfaces at the front side of the electrode. The sensor looked like a piece of paper but possessed high sensitivity for O2 in a linear range from 0.054 to 0.177 v/v%, along with a low detection limit of 0.0075% and a short response time of less than 10 s, foreseeing its promising application in developing cost-effective and environmentally friendly paper-based electrochemical gas sensors.
4.4 IL modified electrodes
Although, the use of ILs as electrolytes and solvents can be a performance enhancing factor for the electrochemical gas sensors based on voltammetric and impedance readouts in terms of selectivity and reproducibility, yet another new direction of using the IL materials as sensing interfaces is to employ them as a component for developing modified electrodes, and in many innovative ways to produce good responses for analyte detection in real-world samples (Fig. 12). Surface modified electrodes are electrodes with deliberately immobilized materials so that the electrodes display chemical, biological and other properties of the immobilized molecules. The properties of the surface modified electrodes are closely associated with the physical and chemical properties of the immobilized molecules as well as the immobilization processes on the substrate electrodes. ILs are good solvents to be miscible with many other materials and they are not volatile allowing many new composite materials to be made. Task specificity of ILs renders high selectivity, if employed in a modification. Many of the sensing performance parameters are enhanced up to certain extents, even if ILs are only used as an inert solvent. The low melting points and low tendency to crystallize, realized by the combination of a large, usually asymmetric cation and a smaller anion, make ILs good candidates for this modification that can lead to a very diverse set of applications in the sensing field. These modifications can be done in many different ways; however, the choice of modification is entirely based on the application requirements.
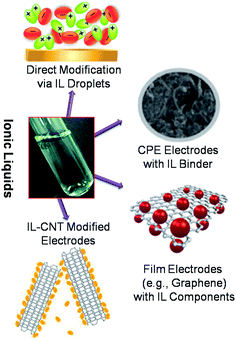 |
| Fig. 12 Schematic of most commonly employed electrode modification protocols utilizing ILs as one of the component. | |
Among many different types of electrode modifications possible for sensor applications, electrodes modified with IL droplets or films are the most commonly employed. In this method, electrodes are prepared by the direct deposition of IL on the electrode surface83 or from its diluted solution in volatile solvent.84 These two procedures may provide different geometries of the deposit. A thin liquid film geometry is most important for sensors which can be enforced by surface coverage with porous Teflon membranes. The preparation of an IL-modified electrode in situ by adsorption of IL from an aqueous solution has also been reported.85 The appropriate selection of electrode substrate and IL is important in this case to obtain a stable liquid deposit in contact with the aqueous solution. Although a lot of research on IL-modified electrodes started from the electrode substrate covered by droplets or a liquid film, in the absence of other film components, now the focus has been shifted to develop modified electrodes with organic or inorganic polymers, nanoparticles, nanotubes and other micro- or nano-objects as other components. Their complexity ranges from simple ones such as a polymer film plasticized with IL to multicomponent films. In many of these cases of direct modification, ILs, especially the imidazolium based ones, can also be appended to demonstrate functionalities which are related to their immobilization onto the electrodes to generate different types of modified electrodes. These functionalities include self-assemblies of IL on the electrode surface, covalent bonding of IL to the electrode surface, IL functions that can be used for the preparation of IL-based polymer films (PILs), and IL used for the functionalization of conductive elements of the film. When the electrode is ready, the counterions are electrostatically attracted to the positively charged functionalities and they can be exchanged after immobilization on the electrode surface. Such small modifications can be highly suitable for incorporating the desired characteristics into the electrode materials. However most importantly, the electrodes modified by any of these methods can then be utilized for various sensing applications. For example, Ng et al.86 employed a nanocomposite gel consisting of a three-dimensional graphene material and IL [C4mim][PF6] for the amperometric detection of nitric oxide (NO). A linear response of current vs. concentration over the range 1–16 μM NO was observed, with a fast response time of less than 4 seconds and a low detection limit of 16 nM. The improved response of this modified electrode is attributed to the porous graphene material that has a high specific surface area and superior conductivity which generated a 3-D graphene/IL nanocomposite to provide a novel platform for sensitive NO detection. Composites of IL materials can also be used for electrode modifications. For instance, an amperometric NO sensor based on poly(eosin b)–IL composite (PEB–IL) films has been described.87 In this work, conducting PEB was firstly electrodeposited on the surface of a glassy carbon electrode, and then a composite of [C6mim][PF6] and didodecyl dimethyl ammonium bromide (DDAB) was coated on the PEB film. The resulting PEB–IL composite has the advantages of a fast response and high selectivity for the determination of NO. The results showed that the PEB–IL composite modified electrode exhibited an excellent anti-interference ability and a wide linear relationship over a NO concentration range of 3.6 × 10−8 to 1.3 × 10−4 mol L−1 with a low detection limit of 1.8 × 10−8 mol L−1 (S/N = 3). This sensor was successfully applied to monitor NO release in rat kidneys signifying its real life performance which can have potential applications in many heart and kidney related conditions.
The high viscosity of ILs can also be employed to enhance the performance of the modified electrodes, for example, as a binder in carbon paste electrodes which can then also be used as sensing materials. In most cases, hydrophobic ILs are used for IL-based carbon paste electrodes (ILCPE). In contrast to classic CPEs, their binder is composed of charged species and exhibits ionic conductivity which enhances the electrochemical sensing capabilities of these modified electrodes. Typically, ILCPEs are prepared as classic CPEs by mixing or grinding graphite particles with IL and placing the mixture in the cavity of the polymer or glass tube. After polishing, the electrode is ready to use. The IL/graphite particles ratio has to be optimized from the point of view of not only mechanical stability but also capacitive current, resistance and specific electrode process, and a 7
:
3 graphite to IL ratio has become a popular composition. Imidazolium-type ILs also tend to form physical gels when ground with SWCNTs by physical cross-linking of the nanotube bundles, mediated by local molecular ordering of ILs. Recently, ILs have been found to be efficient binders in the preparation of carbon composite/carbon paste electrodes (CPEs). They are prepared by mixing or grinding graphite particles with IL, followed by the transfer of this mixture into a cavity of a polymer. Higher currents (both faradaic and capacitive) are often observed at ILCPEs compared to those of traditional CPEs. This is believed to be due to the larger electroactive area for electron transfer in the ILCPEs due to the conductive IL medium. In traditional oil-based CPEs, the electron transfer can only take place at the carbon–aqueous electrolyte interface. Other reasons for the higher currents may also be explained by the changes in the paste morphology, better solubility of polar analytes in IL (compared to the binder) or the presence of an additional interface where transfer across the liquid–liquid interface can occur. Due to these attributes of IL–carbon pastes, they have been employed for the highly selective sensing of explosive TNT by Guo et al.88 IL [C4mim][PF6] was combined with three-dimensional graphene to make an IL–graphene paste electrode with a large surface area, low background current and pronounced mesoporosity. A linear relationship was observed between the peak current, using absorptive stripping voltammetry, and the concentration from 2 to 1000 ppb, with a low detection limit of 0.5 ppb for TNT. This performance was superior to those demonstrated by IL–CNT and IL–graphite composites.
4.5 Mass sensing transducers: QCM and SAW devices for IL sensing
ILs provide unique properties required for a chemical sensor using a mass sensing transducer such as QCM and SAW devices. QCM and SAW transducers are often made of quartz crystals or other piezoelectric materials with associated metal electrodes that are in contact with the sensing films. The mechanism of operation of these sensors involves analyte induced changes in the mass, viscosity, elasticity, and even the conductivity of the sensing film and the correlation of these changes with the changes in the frequency, dissipation, and damping resistance of the sensor. In contrast to electrochemical sensors, the high viscosity of ILs is beneficial to these sensors since they can be cast into thin films. Being highly volatile, solvents are seldom used as sensing elements. By contrast, ILs have no significant volatility, enabling chemical processes to be carried out with essentially zero emission of toxic organic solvents and their utilization as recognition elements. In these applications, ILs behave simultaneously as both solid and liquid interfaces, thus overcoming the issues associated with the interchangeable use of solid and liquid phases and the requirement of solvents to generate these phases. Depending on the physical phenomenon involved in the solvation of analytes in ILs, a coating of IL can swell, shrink, or undergo a viscosity change. The solvation in ILs is controlled by the variable contributions of adsorption–desorption and partition phenomena depending upon IL and analyte properties to furnish additional selectivity, thus opening up excellent opportunities to design different arrays of chemically selective IL films. Such characteristics make these ILs suitable for the detection of analytes in gas environments via QCM, SAW, and even MEMS transducers. The major outcome in all these cases, however, is the reversibility of gas sensing as the mechanism is absorption based and is mostly relying on weaker chemical interactions. The sensitivity and selectivity, on the other hand, has to be achieved by the selection or design of ILs, or using a combination of them in the form of sensor arrays.
Dai and co-workers89 first developed a sensor for organic vapors based on QCM using ILs as sensing materials. When the analytes were dissolved in an IL, the viscosity of this IL changed rapidly which generated a frequency shift of the QCM device. The response of the QCM depended on the nature of both the analyte and IL. Some of these ILs also have strong affinities for selected chemical species. Sensor arrays comprising multiple of these sensors, each with a different IL coating, thus can not only detect target analytes but also help to identify chemical speciation. In most of these cases, ILs are solely used as sensing films which interact with the target analytes through ion and dipole forces, hydrogen bonding, and van der Waals interactions on various time and space scales. All these interactions depend upon variations in the ionic composition and structure of ILs, thereby generating a criterion for the selective incorporation and interaction of analytes. In addition, the great solvation ability of ILs enables the rapid and reversible incorporation of gases, as shown in the work done by our group for detecting different explosive gases.7 We have also shown that the very different solubilities of ILs for specific reactant gases create a membrane-like selectively to enhance or suppress (preconcentrate) the transport of specific reactants, and provide a mechanism for the selectivity.53 Using this principle, we have successfully classified four volatile organic compounds (benzene, hexane, methylene chloride and ethanol) via seven IL film-based mass sensors, where the unique selectivity of the ILs results from the strong hydrogen bond basicity and significant capacity for dipole-type interactions with the analyte.22,90 A similar strategy was implemented for the detection of organic acid vapors using [C4mim][C1] coated on QCM.91 The experimental results indicated that the sensor showed a fast response, excellent sensitivity and good reproducibility towards organic acids. There was a good linear relationship between the frequency response and the testing concentration of these organic acid vapors.
Expanding this approach to real life applications, an array of quartz crystals coated with seven different ILs is proposed for the analysis of flavors by QCM measurements.92 The selected ILs all contained imidazolium or phosphonium cations, differing from one another in the length and branching of the alkyl groups. The array was at first applied to the analysis of 31 VOCs chosen as representative components of a wide variety of food flavors. Multivariate data analysis using principal component analysis (PCA) led to separated clusters for these different chemical categories. To further prove the good performance of the developed electronic nose, it was applied to the analysis of headspace from cinnamon samples belonging to different botanical varieties (Cinnamon zeylanicum and Cinnamon cassia) indicating that the responses recorded on different stocks of samples of both varieties could be fully discriminated.
4.5.1 High temperature mass sensing via ILs. ILs possess a high thermal stability.93 Most ILs show typical decomposition temperatures of 350+ °C. This remarkable thermal stability has important implications in the use of ILs for high temperature sensing.9,94 For example, a polar IL, [P14,6,6,6][DBS], was prepared via the alcohol-to-alkyl halide conversion method and coated onto QCM from its ethanol solution. This sensor was studied for the exposure of both polar (ethanol, dichloromethane) and nonpolar (heptane, benzene) vapors even at 200 °C, showing a linear response pattern and clear signals, as shown in Fig. 13. As thermodynamically expected, the sensor signal decreased with increasing temperature, but a 5% detection limit was still achieved, which is encouraging because most solid surfaces are unable to adsorb vapors at temperatures that much higher than their boiling points. In addition, it showed an excellent reversibility for adsorption–desorption processes, requiring no experimental manipulation for sensor recycling. The data for the damping resistance showed that physicochemical parameters such as Henry’s constant can be more accurately determined at higher temperatures because of the lowered viscosity of the ILs. In another study,95 gel beads containing ionic liquid were prepared by suspension polymerization with diameters of 100–2000 μm and were applied as hydrogen sensing materials. The sensor utilizing this material has a nice flexibility and high heat endurance. The weight and shape of the gel beads were maintained not only under high temperature conditions but also under vacuum conditions. The resulting hydrogen microsensor was able to detect a 2% hydrogen concentration. It has a high grade of gas selectivity and can detect hydrogen gas in the range of the explosion limit. These results indicate that IL materials can be employed for the detection in harsh, otherwise unmanageable environments.
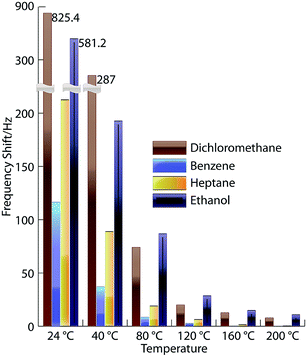 |
| Fig. 13 Responses shown by IL-sensors from ambient to elevated temperatures for different VOCs. (Reprinted with permission from ref. 30. Copyright (2012) American Chemical Society.) | |
4.5.2 IL composites for mass sensing approach. For IL-based mass-sensors, to minimize the bulk properties (e.g., viscoelastic properties) change generated signals, the sensing films on the electrodes have to be thin which are usually formed by employing the methods of electrode modification. The effectiveness of most thin film-based sensors relies on the high sensitivity and specificity of the detection interface. The obvious approach to increase the sensitivity of QCM sensors is to increase the thickness/amount of the sensing material. Pure ILs inherently possess certain limitations in this regard, especially for low molecular weight analytes, where a sensitivity enhancement by thicker IL films can substantially influence the reproducibility through temperature-controlled variations in the IL-layer thickness and the spreading-out effects. The non-rigidity of thicker films makes Sauerbrey’s equation invalid in addition to the slower responses, requiring a strategy to achieve higher IL-loading while maintaining the IL-film integrity. Thin films made from ILs would perform well as sensor interfaces and provide additional control over the selectivity and sensitivity when interacting with the analytes in the gas phase. Most organic solvents or vapors are soluble in ILs. Therefore, the partition process will very quickly reach equilibrium after the sensor is exposed to the vapors. For these situations, IL composite films are usually employed. Several approaches have been developed for fabricating IL-composite sensing films. In one such example, ILs are trapped as nanodroplets into the cylindrical cavities of a solid alumina matrix, thus avoiding liquid wetting and softness.96 Although this matrix can hold more IL than a planar gold surface, the detection limits were yet not low enough for the selected analytes. By contrast, conducting polymers (CPs) and polyelectrolytes have charges, which make them ideal template materials to make IL-composite films. The proof of concept was provided for the example of methane,6 a highly inflammable gas with a very low molecular weight, which is hence an evident candidate for a higher sensitivity in mass sensing devices, requiring an abundant total absorption/partition into the films. An ideal template for this purpose should be a porous and stable scaffold that can be modified to generate the required surface area and wettability for IL immobilization. Polyaniline (PAN) can provide this dimensionality. Four different oxidation states of PAN including the doped and undoped ones were tested for the analytical response with an IL, e.g., [C2mim][CS], which can form hydrogen bonds through the sulfonate group, however, the doped PAN showed the highest sensitivity being highly charged and facilitating the IL wettability through electrostatic interactions, in addition to hydrogen bonding. This increased the IL surface area exposed to the analyte. The IL distribution into the nanosized channels of the PAN film helped to increase the response owing to the increase in IL film coverage. UV-vis and FT-IR data in this case confirmed97 the formation of hydrogen bonds between camphorsulfonate and the nitrogen sites of protic acid-doped PAN. These bonds force the anions to align along the polymer backbone in a comb-like manner so as to enhance the long-range π-orbital conjugation. The interacting methane molecules can fit into these comb spaces and thus an enhanced sensitivity was observed. The enthalpy and entropy of the dissolution were shown to be higher than those in pure IL or PAN which further supported the existence of methane inside the composite by generating a more ordered structure. Molecular mechanics simulations agreed with these results as well. This example and others indicate that CPs often have fairly rigid structures with tunable porosity and charge states, which can promote the rational development of the CP/IL interface alongside the IL-controlled parameters. The chemical selectivity can be provided by varying the oxidation states of CP and the ionic structure of IL.Besides, many useful approaches are available for the IL-composite formation for their use in QCM sensors, as was the case with electrochemical sensing applications. The most widely used techniques include direct mixing, casting, physical adsorption, electrodeposition, layer-by-layer assemblies, and sol–gel encapsulation. Physical adsorption of IL onto a solid support is most often used to prepare the sensing films, which is based on binding forces including ionic interactions, hydrogen bonds, van der Waals forces, hydrophobic interactions, and so on. However, the more sophisticated methods for this immobilization are electrodeposition, sol–gel encapsulation, and layer by layer assembly. In electrodeposition, a clean electrode is immersed in a bathing solution containing the supporting electroactive material and corresponding IL which are then electrochemically deposited onto the electrodes.98,99 Electrodeposition of metal nanoparticles onto IL/CNT is another well studied technique to prepare AuNP/IL/CNT nanocomposite-based sensors.100 In sol–gel encapsulation, an IL–silica sol is synthesized which is then mixed with different types of interesting molecules to form the films,101 whereas in the layer-by-layer method a sequential deposition of multiple layers is achieved,102 one being the IL and the other one the supporting material, onto the electrode surface by electrostatic, van der Waals, hydrogen bonding, and charge transfer interactions. From all these approaches, benefits and weaknesses can be identified for any of them; however, the selection is highly related to the particular application in which the approach is going to be implemented.
4.6 IL-based optical approaches for gas sensing
Although the solvation capability of the IL films plays the role of interacting with the target analytes both in the electrochemical and mass sensing formats, thereby having a partition coefficient and defining the sensitivity and selectivity of the resulting sensor, their roles as solvating species can yet be best described with the examples of optical sensing. Here, ILs enable many important optical substances to be dissolved and to form stabilized optical matrices.103–106 The optical sensing mechanism probably has the highest face value for commercial sensing, as most of the time it involves the change in some color pattern (readable by the end user without technical knowledge) after interaction of the target with the sensing film; though there are many sensor developments where the change in color pattern is too minute or complex to be detected visibly, or the change involves some non-visible part of the electromagnetic spectrum thereby requiring analytical tools/instruments for detection and further analysis. Moreover, the use of ILs provides even more stability to the resulting optical matrix thereby enhancing the reliability and reproducibility of sensing.
4.6.1 IL matrices and composites for optical gas sensing. The IL-based colorimetric sensors are usually fabricated using color responsive dyes that are integrated into ILs and change their color based on intermolecular interactions, such as acid–base, dipole–dipole, and π–π interactions. IL-based optoelectronic sensors have shown a higher chemical selectivity and sensitivity compared to those of other types of sensors.107–109 For instance, Oter et al. reported110 an optical CO2 sensor using ILs ([C4mim][BF4] or [C4mim][Br]) as the matrix with 8-hydroxypyrene-1,3,6-trisulfonic acid trisodium salt (HPTS). The detection of CO2 is based on the fluorescence signal change of HPTS when pairing with CO2. More recently, the same group reported111 that the IL modification of an ethyl cellulose matrix extended the detection range to 0–100% pCO2. In comparison to conventional solid matrix or liquid optical sensors, which have leaching problems, short life times because of the evaporation of solvents, and poisoning issues with ambient air and interfering gases, IL-based optical sensors showed a much better performance due to the ILs’ distinctive properties and better CO2 adsorption than that of polymeric materials. For these optical sensors, different IL composites have again been tested to enhance the different sensing parameters. In one such report,112 the feasibility of being able to control the selectivity of solid-state ruthenium complex-based electrochemiluminescence (ECL) sensors is examined by selecting ILs with appropriate hydrophobicity in a sol–gel titania/Nafion composite film. Four different kinds of IL with different hydrophobicity were incorporated into the sol–gel films, and the sequence of the ECL intensities for different hydrophobic analytes was observed. It was found that this sequence was closely related to the sequence of hydrophobicity of the ILs. By contrast, the observed ECL sequence for hydrophilic analytes was opposite to the sequence of hydrophobicity of the ILs. Based on these signals, the final sensor was able to give a remarkable detection limit (S/N = 3) of 0.48 nM for tripropylamine. Similar ECL sensor designs have also been reported113 for the detection of SO2 with remarkable outcomes in terms of sensing capability (e.g., ppb level of detection).
4.6.2 IL structural diversity for optical sensing approach. The IL diversity in terms of anion and cation combination has also provided further selectivity in the optical protocols. Only by a simple counter-ion exchange of ILs, a rapid, facile, and efficient strategy was developed to create a cross-reactive sensor array with a dynamic tunable feature,114 which can then be used to construct a florescence sensor array for the identification and classification of nitroaromatics and explosive mimics. The so-generated cross-reactive array had diversiform properties, such as different micro-environments, diverse molecular interactions, and distinctive physicochemical properties, to obtain a distinct fingerprint of explosives. It was found that the LUMO–HOMO energies, excitation and emission spectra, fluorescent life times as well as the sensing capability of the synthesized IL could be significantly influenced and tuned by coupling different counter-anions, indicating that from one single IL sensor element, a large sensor element pool with rich diversities could be conceivably created by a simple exchange process of counter-anions. Moreover, the reversible counter-ion exchange capability of IL also provides a plenty of room and useful means to post-modify the sensing properties of individual element for further correcting or optimizing the sensing performance of the formed sensor array. The reversible anion exchange ability further endowed the sensor array with a dynamic tunable feature as well as a good controllability and practicality for real-world applications. In addition to these cross-reactive arrays, specific colorimetric ILs have also been synthesized from readily available pH indicator dyes. For example, a series of 12 different chemosensory ILs were synthesized115 by pairing anionic pH indicator dyes with the trihexyl(tetradecyl)phosphonium ([P6,6,6,14]) cation via an ion exchange reaction. The incorporation of the [P6,6,6,14] cation imparted hydrophobic characteristics to these ILs, and the induced hydrophobicity led to the desired low solubility in aqueous solutions, as well as eliminated the need for a specialized hydrophobic matrix/substrate for immobilization. These sensor arrays were then used to analyze pH values of aqueous solutions as well as for the detection of acidic and basic vapors. Using cotton threads as a matrix led to the development of an even more flexible, low volume, and lightweight array to estimate pH and detect a variety of vapors. These wearable arrays may possibly be incorporated into bandages, sweatbands, diapers, and similar systems. Overall, these IL-based sensor arrays should provide a new research direction in the development of advanced colorimetric sensor arrays for the detection and identification of a range of analytes relevant to many different applications.
4.6.3 Innovative IL chemistries for optical detection. A unique contribution that IL materials have provided to the sensor science is new and innovative chemistries of reactions due to their biphasic nature and inorganic/organic characteristics. These new chemistries are not left behind in the development of new optical detection technologies. The most important and most comprehensively studied one is the stability of the superoxide radical in ILs. The same basic principle has been utilized to develop a CO2 gas sensor.116 It has been shown that in the absence of stable superoxide in a conventional system, the oxygen–luminol reaction was unable to produce the ECL signal in an electrolyte-free N,N-dimethylformamide (DMF)–dipropylamine (DPA) co-solution. However, when CO2 was introduced to the system, it induced the formation of a carbamate IL by reacting with DPA. Because of the presence of this IL in the system, the superoxide radical became stable thereby generating an ECL signal for luminol. This system was used as a sensing mechanism for CO2 demonstrating good performance parameters such as high safety, high selectivity, a wide linear response range, and good sensitivity. The gas sensor was found to have a linear response range from 100 ppm to 100 v/v% and a detection limit of 80 ppm. Besides showing innovative chemistries, IL also provide stability and other performance parameters which was shown in a ratiometric CO2 sensor,111 based on the spectrophotometric signal change of bromothymol blue (BTB). Effects of ILs with different anionic and cationic parts on the sensor signal intensity were tested. For this sensor, the sensing agents were produced both in form of nanofibrous materials and continuous thin films. The nanofibers were fabricated by the electrospinning technique. The ratiometric response to CO2 was monitored at 396 and 640 nm. The offered sensing material can be used for the quantitative determination of CO2 in a concentration range of 0.0–100.0% pCO2. With respect to continuous thin films, the electrospun nanofibers offered an enhanced sensitivity extending to a 98% relative signal change, lower detection limits and shorter response times. BTB also satisfies best the requirement of a color change distinguishable with the naked eye when encapsulated in nanofibers. ILs not only provided enhanced long-term stabilities in this system but also facilitated the fabrication of nanofibers providing an electrical conductivity in the polymer by the effect of ionic groups.
4.6.4 Photo-responsive ILs. Another straightforward method for IL-based colorimetric sensors is the synthesis of luminescent ILs, which can be obtained by integrating photochromic cations or anions into ILs and can enable the direct detection of targeted reactions. Pina et al. recently reported, for example, the successful synthesis of photochromic intrinsic ILs using the photochromic methyl orange anion,117 taking advantage of its light response together with the properties specific to ILs. Deng and co-workers also synthesized a new class of azobenzene-based ILs, which showed a distinct and reversible solvent-dependent photoresponsive conductivity.118 It is interesting to see that sometimes even the same photochromic modified IL could show different properties with isomers.119–121 The modification of ILs by a photochromic group which will present a reversible photochromic behavior122 and can exhibit lower melting points, will be an ideal possibility for the gas sensor development.
4.7 Task specific approach for IL sensing
The interplay of physical forces and interactions between ILs and gases results in many sensor applications as described earlier, however the most important limitations in this regard can be the sensitivity and selectivity. Improvement in sensitivity can be brought about by increasing the solvation power of ILs which has recently been proposed using task specific ILs which can take up higher concentrations of simple gases, such as CO2, SO2, H2 and O2.123,124 Presently, this field of research is expanding because of the need of CO2 and SO2 capture and absorption studies.125 Davis and co-workers have done the pioneering work in this regard by designing a special task-specific IL that can capture CO2 specifically by chemisorption.126 The imidazolium cation in this IL contains an amine functionality, which forms a carbamate upon the addition of CO2. In this way, a maximum CO2 uptake of 0.5 mol per each mole of IL was reached within 3 h. The process can be reversed by heating to 80–100 °C under vacuum which can be an important step towards the detection and then regeneration of the sensor by heating. The same research group used combinatorial click chemistry to generate an array of ILs127 based on a bifunctional anion containing an amine and a sulfonate group, in combination with an ammonium cation. All these ILs have gas capture abilities and thus can be used for sensors.
Although, the generic combinations of IL with other electrode materials as shown in the case of NO sensing have proven quite useful for sensing applications,86 the results are yet again generic in most cases with much less selectivity than required. Therefore, the focus is now shifting to use more task-specific ILs also in the case of electrode modifications. Lu et al.128 have shown that a task-specific IL in combination with bismuth oxide (Bi2O3) can be used for the electrochemical detection of heavy metal oxides including cadmium oxide (CdO), copper oxide (CuO) and lead oxide (PbO). The IL contained [NTf2]− anions and tetraalkylammonium cations with one carbon chain functionalized with a carboxylic acid group. The presence of the acid group allowed for solubilization of the metal oxides in the IL. The IL was coated as a thin layer onto a surface containing three indium tin oxide (ITO) printed electrodes and acted as the selective solubilization medium and electrolyte. It is envisioned that more analyte species may begin to be detected by employing newly-synthesized ILs with specific functional groups.
4.8 IL gas sensors with multi-transduction modes
Despite the availability of such a large library of IL materials and their composites, existing and intended gas sensor applications need more sophisticated methodologies to overcome the posed complications in gas sensing. For example, a gas sensor system can be calibrated to quantitate up to three individual gases in a mixture with interferents using the available transducers and methodologies. Starting from this calibrated state, the quality of the performance degrades due to: (i) the presence of analytes and interferents above the calibrated levels, (ii) temperature fluctuations, (iii) contamination and poisoning of the sensor surface, (iv) moisture or mechanical damage, and (v) ageing of the sensing material and transducer. All these end-user factors result in a poor selectivity and reversibility of the sensor response which are often conflicting to each other. Full reversibility is only achievable using weak interactions of the film while the selectivity/specificity come from stronger interactions. In order to address this problem, either the design of new sensing materials or the development of sensor arrays is the direction to look for. However, more recently the development of a multi-transducer sensor and sensor arrays is gaining importance. Examples of such hyphenated devices are shown in Fig. 14 and include chemiresistor–capacitor, cantilever–capacitor–calorimeter,129 electrochemical-QCM,68,130 optical-QCM,131 FET-QCM,132 and opto-electronic sensors.133 Even multiparameter detections have been demonstrated within the same transduction principle (e.g., capacitance–resistance)134,135 and using FET-temperature programmed sensors.136
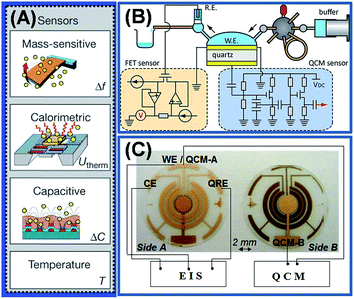 |
| Fig. 14 Examples of multimodal transduction approaches for the gas sensor development: (A) a single chip microsystem for smart gas sensing simultaneously using mass sensitive calorimetric and capacitive measurements, (B) a sensor design utilizing QCM and the field effect transistor (FET) approach, and (C) and integrated electrochemical QCM for simultaneous EIS and mass sensing measurements. (Adapted from ref. 129 and 132 with permissions from Nature publishing group and American Chemical Society respectively.) | |
A critical limitation in this regard is the sensing material and its capability of being used for the multiple transducers applied in the sensor system. The diverse nature and properties of ILs is a special gift in this regards, as it can be exploited for this multi-sensing approach. Electrochemical quartz crystal microbalance (EQCM) is a powerful analytical technique to demonstrate this potential, as IL can be used as an electrolyte and the sensing film at the same time. Fig. 14 shows an integrated EQCM electrode fabricated on a monolithic quartz wafer. AT-cut quartz crystals oscillating in a shear thickness mode exhibit extraordinary high sensitivity to potential-induced changes in mass at the electrode–electrolyte interface via change of its resonance frequency. In addition to that, the monitoring of the mechanical state of the viscoelastic coatings at quartz crystal electrode via a change in their shear storage and loss modulus is possible. Thus, its dynamic capability allows for real-time monitoring of minute mass changes during electrochemical reactions in the IL and the accompanying viscoelastic changes.137 Thus, a QCM used with electrochemistry methods such as EIS and CV enables real-time in situ mass changes in the nanogram range during the electrochemical perturbation of the electrode solution interfaces. Forzani et al.138 proposed a hybrid electrochemical-colorimetric sensing platform for the detection of explosive trinitrotoluene (TNT) vapors. A thin layer of IL [C4mim][PF6] was found to selectively pre-concentrate the explosive materials and quickly transport the analytes to the electrodes. The explosive vapors were detected by electrochemical (cyclic voltammetry) and colorimetric (absorbance change) methods at the working electrode. The observed currents and distinct color changes provide a fingerprint for the identification and quantification of TNT. The same group later employed a conducting polymer nanojunction that was sensitive only to the reduction products of TNT and was able to discriminate them from other redox-active interferents found in ambient air. The sensor simultaneously measured the current for the reduction of TNT and the resulting conductance change of a polymer (poly(ethylenedioxythiophene), PEDOT). A linear response (current vs. concentration) was observed at concentrations of 30 pM to 6 nM TNT and the sensor was capable of detecting extremely low levels of TNT within 1–2 minutes. Thus, it could be shown that IL materials are capable of bringing about plentiful new innovation in sensor technologies by this hyphenation approach.
5. Concluding remarks and future directions
By synergistically utilizing an IL interface as well as the molecular design and control of IL composites, many sensing platforms have been demonstrated which enable multiple gas detections with multiple-mode detection principles, addressing many gas sensing challenges, especially the sensitivity, selectivity, and reproducibility for real-world scenarios. In terms of resolution, however, these sensors are not competitive against high-end laboratory instruments (e.g., a GC × GC instrument equipped with TOF-MS can detect ∼1300 volatiles in breath at a signal/noise resolution of ∼100). Even the field deployable and microversions of these instruments (e.g., a cell phone sized gas analyzer can detect and quantify ∼10 volatiles at the ppb level) are advancing in this competition due to their power requirements similar to those of packaged sensor systems, and due to the advances in miniaturization of these instruments. Thus, the conventional arguments of low cost, low power and small size in favor of the sensors are not so compelling. However, a number of clear advantages (e.g., the smallness which enables wearable sensors to be developed; the mobility which enables continuous measurements performed by the sensor in comparison to periodic measurements done by instruments; the ability to design wireless sensors thereby enabling continuous tracking of gas emissions, interpret sensing data in real time, and provide in situ alerts and control services for a broad range of applications; low costs which enable disposable and wireless sensors to be developed for harsh environments by exposing only the detection part to the harsh environments and keeping the readout as well as the operating personnel protected from that environment; and the overall capability to address the new and emerging sensor markets by making the so-developed wearable and disposable sensors even utilizable by non-technical users) motivate us and other proponents of gas sensor technologies to continue advocating for it. However, in order to keep doing this, there is a critical need for innovative approaches and for identifying and analyzing the failure factors in each and every practical sensor development. For this purpose, three aspects of the sensor development should especially be focused on: (i) sensing materials should be developed based on new design concepts and new fabrication principles, (ii) a proper selection and possibly proper combinations of transduction principles should be favored to obtain the best response parameters and to suppress interferences, and (iii) signal processing should be reinforced to move towards complex signal resolutions from real-life data.
Especially for IL sensors, many fundamental aspects of physical chemistry and electrochemistry remain to be thoroughly explained, before they can promise to further improve their potential for gas sensing applications. For example, the fundamental electrochemical issues such as the structure of the double layer and diffusion layer at the electrode/IL interface, the speciation of solute ions such as metal ions and polymerized ions, transference numbers and how these are influenced by the speciation, dielectric properties and how these merge with the conduction properties as a function of frequency, surface chemistry and wetting phenomena of ILs at a three-phase interface, thermodynamic quantities such as chemical potentials of solutes in ILs and how these influence redox potentials, ion association and its effect on thermodynamic and transport properties, and interactions of ILs with solutes and interfaces, should be focused on. Studies on the thermal stability of ILs for engineering applications should analyze the IL with thoughts on evaporation or temperatures at which evaporation kinetics are known. Temperature-dependent properties and the high dependency of the results on the experimental factors need to be investigated by comparing different conditions. In addition, ILs have been shown as promising alternative solvents in variety of applications. However, only a few processes are commercialized due to the relatively high cost of ILs. The recovery and reuse of ILs play an important role in the commercialization of processes employing ILs. The understanding of the recyclability of ILs based on the available literature in various application fields is still in its infancy stage. Therefore, this aspect of research should be focused on for all practical applications.
Another area where we are lacking knowledge not only in terms of IL sensors, but rather all sensor systems, is field testing or the actual deployment of working prototypes. Initial experiments under controlled conditions are critical, however without further testing in real-world environments, most of the development in the field is just piling up as publications. This is why the number of sensors deployed in the field is rather limited. In order to speed up this commercialization process, more research is required for system designs and integration thereby engaging collaborations from diverse disciplines for prototype development and field testing.
Acknowledgements
X. Zeng likes to thank the following grants for IL-based gas sensor development: the National Institute of Occupational Safety and Health (1R21OH009099-01A1 and 1R01OH009644-01A1), Office of Naval Research (Grant N000141010734), National institute of Environmental Health (R01ES022302) and Michigan Initiative for Innovation and Entrepreneurship (MIIE). Dr Yongan Tang’s contribution in the initial stages of the manuscript preparation is acknowledged.
References
- J. R. Stetter, G. Korotcenkov, X. Zeng, Y. Tang and Y. Liu, Chemical Sensors Comprehensive Sensor Technologies, ed. G. Korotcenkov, Momentum Press, LLC, New York, 2011, vol. 5, ch. 1, pp. 1–89 Search PubMed.
- J. R. Stetter and J. Li, Chem. Rev., 2008, 108, 352–366 CrossRef CAS PubMed.
- T. Wagner, S. Haffer, C. Weinberger, D. Klaus and M. Tiemann, Chem. Soc. Rev., 2013, 42, 4036–4053 RSC.
- R. A. Potyrailo, C. Surman, N. Nagraj and A. Burns, Chem. Rev., 2011, 111, 7315–7354 CrossRef CAS PubMed.
- X. Jin, Y. Huang, A. Mason and X. Zeng, Anal. Chem., 2009, 81, 595–603 CrossRef CAS PubMed.
- X. Jin, L. Yu and X. Zeng, Sens. Actuators, B, 2008, 133, 526–532 CrossRef CAS PubMed.
- A. Rehman, A. Hamilton, A. Chung, G. A. Baker, Z. Wang and X. Q. Zeng, Anal. Chem., 2011, 83, 7823–7833 CrossRef CAS PubMed.
- Z. Wang, X. Y. Mu, M. Guo, Y. Huang, A. J. Mason and X. Q. Zeng, J. Electrochem. Soc., 2013, 160, B83–B89 CrossRef CAS PubMed.
- L. Yu, D. Garcia, R. Ren and X. Zeng, Chem. Commun., 2005, 2277–2279 RSC.
- L. Haitao, M. Xiaoyi, W. Zhe, G. Min, Z. Xiangqun and A. J. Mason, IEEE Sens. J., 2013, 13(10), 3976–3981 CrossRef.
- L. Haitao, M. Xiaoyi, W. Zhe, L. Xiaowen, G. Min, J. Rong, Z. Xiangqun and A. J. Mason, Proceedings of the Annual International Conference of the IEEE Engineering in Medicine and Biology Society, EMBS, 2012, pp. 503–506 Search PubMed.
- A. Fulmer, M. Mullen, C. Sun and P. K. Dutta, Micro- and Nanotechnology Sensors, Systems, and Applications VI, Proceedings of SPIE, 2014, vol. 9083, p. 90830W Search PubMed.
- Y. Liu, J. Parisi, X. Sun and Y. Lei, J. Mater. Chem. A, 2014, 2, 9919–9943 CAS.
- P. Lieberzeit, A. Rehman, B. Najafi and F. Dickert, Anal. Bioanal. Chem., 2008, 391, 2897–2903 CrossRef CAS PubMed.
- D. G. Rickerby and A. N. Skouloudis, Int. J. Nanotechnol., 2014, 11, 583–593 CrossRef CAS.
- M. Xiaoyi, W. Zhe, Z. Xiangqun and A. J. Mason, IEEE Sens. J., 2013, 13, 3976–3981 CrossRef.
- S. Li, J. Forensic Sci. Criminol., 2014, 1, 1 CrossRef.
- A. Wilson, J. Forensic Sci. Criminol., 2014, 1, S103 Search PubMed.
- M. Righettoni, A. Amann and S. E. Pratsinis, Mater. Today, 2015, 18, 163–171 CrossRef CAS PubMed.
- M. C. Buzzeo, C. Hardacre and R. G. Compton, Anal. Chem., 2004, 76, 4583–4588 CrossRef CAS PubMed.
- N. Dossi, R. Toniolo, A. Pizzariello, E. Carrilho, E. Piccin, S. Battiston and G. Bontempelli, Lab Chip, 2012, 12, 153–158 RSC.
- X. Jin, L. Yu, D. Garcia, R. X. Ren and X. Zeng, Anal. Chem., 2006, 78, 6980–6989 CrossRef CAS PubMed.
- S. Mao, G. Lu and J. Chen, J. Mater. Chem. A, 2014, 2, 5573–5579 CAS.
- N. Yamazoe, Sens. Actuators, B, 2005, 108, 2–14 CrossRef CAS PubMed.
- CRC Handbook of Chemistry and Physics, ed. D. R. Lide, CRC Press, Boca Raton, FL, 74th edn, 1993 Search PubMed.
- A. R. Hopkins and N. S. Lewis, Anal. Chem., 2001, 73, 884–892 CrossRef CAS.
- J. R. Stetter, W. R. Penrose and S. Yao, J. Electrochem. Soc., 2003, 150, S11–S16 CrossRef CAS PubMed.
- L. Rassaei, F. Marken, M. Sillanpää, M. Amiri, C. M. Cirtiu and M. Sillanpää, TrAC, Trends Anal. Chem., 2011, 30, 1704–1715 CrossRef CAS PubMed.
- P. A. Lieberzeit and F. L. Dickert, Anal. Bioanal. Chem., 2007, 387, 237–247 CrossRef CAS PubMed.
- A. Rehman and X. Zeng, Acc. Chem. Res., 2012, 45, 1667–1677 CrossRef CAS PubMed.
- I. Krossing, J. M. Slattery, C. Daguenet, P. J. Dyson, A. Oleinikova and H. Weingärtner, J. Am. Chem. Soc., 2006, 128, 13427–13434 CrossRef CAS PubMed.
- A. M. O’Mahony, D. S. Silvester, L. Aldous, C. Hardacre and R. G. Compton, J. Chem. Eng. Data, 2008, 53, 2884–2891 CrossRef.
- J. A. Widegren, A. Laesecke and J. W. Magee, Chem. Commun., 2005, 1610–1612, 10.1039/b417348a.
- S. Silvester Debbie and G. Compton Richard, Z. Phys. Chem., 2006, 220, 1247–1274 CrossRef.
- J. A. Widegren, E. M. Saurer, K. N. Marsh and J. W. Magee, J. Chem. Thermodyn., 2005, 37, 569–575 CrossRef CAS PubMed.
- U. Schroder, J. D. Wadhawan, R. G. Compton, F. Marken, P. A. Z. Suarez, C. S. Consorti, R. F. de Souza and J. Dupont, New J. Chem., 2000, 24, 1009–1015 RSC.
- N. V. Ignat’ev, U. Welz-Biermann, A. Kucheryna, G. Bissky and H. Willner, J. Fluorine Chem., 2005, 126, 1150–1159 CrossRef PubMed.
- A. J. Carmichael and K. R. Seddon, J. Phys. Org. Chem., 2000, 13, 591–595 CrossRef CAS.
- M. B. Turner, S. K. Spear, J. D. Holbrey and R. D. Rogers, Biomacromolecules, 2004, 5, 1379–1384 CrossRef CAS PubMed.
- F. Zhao, X. Wu, M. Wang, Y. Liu, L. Gao and S. Dong, Anal. Chem., 2004, 76, 4960–4967 CrossRef CAS PubMed.
- Y. Liu, M. Wang, J. Li, Z. Li, P. He, H. Liu and J. Li, Chem. Commun., 2005, 1778–1780, 10.1039/b417680d.
- R. Seifert, H. Keller and J. Matthes, Sens. Transducers J., 2015, 184(1), 1–10 CAS.
- P. Reimann and A. Schütze, Gas Sensing Fundamentals, Springer, 2014, pp. 67–107 Search PubMed.
- T. Slawomir, Meas. Sci. Technol., 2007, 18, R31 CrossRef.
- N. Karker, G. Dharmalingam and M. A. Carpenter, ACS Nano, 2014, 8, 10953–10962 CrossRef CAS PubMed.
- H. Zhang, Y. Yang, Y. Su, J. Chen, K. Adams, S. Lee, C. Hu and Z. L. Wang, Adv. Funct. Mater., 2014, 24, 1401–1407 CrossRef CAS PubMed.
- G. Bontempelli, N. Comisso, R. Toniolo and G. Schiavon, Electroanalysis, 1997, 9, 433–443 CrossRef CAS PubMed.
- J. A. Cox, K. S. Alber, C. A. Brockway, M. E. Tess and W. Gorski, Anal. Chem., 1995, 67, 993–998 CrossRef CAS.
- B. Barroso-Fernandez, M. T. Lee-Alvarez, C. J. Seliskar and W. R. Heineman, Anal. Chim. Acta, 1998, 370, 221–230 CrossRef CAS.
- S. Otsuki and K. Adachi, J. Appl. Polym. Sci., 1995, 56, 697–705 CrossRef CAS PubMed.
- C. Xiao, A. Rehman and X. Zeng, RSC Adv., 2015, 5, 31826–31836 RSC.
- M. J. A. Shiddiky and A. A. J. Torriero, Biosens. Bioelectron., 2011, 26, 1775–1787 CrossRef CAS PubMed.
- Z. Wang, P. Lin, G. A. Baker, J. Stetter and X. Zeng, Anal. Chem., 2011, 83, 7066–7073 CrossRef CAS PubMed.
- M. A. G. Zevenbergen, D. Wouters, V.-A. T. Dam, S. H. Brongersma and M. Crego-Calama, Anal. Chem., 2011, 83, 6300–6307 CrossRef CAS PubMed.
- A. D. Aguilar, E. S. Forzani, M. Leright, F. Tsow, A. Cagan, R. A. Iglesias, L. A. Nagahara, I. Amlani, R. Tsui and N. J. Tao, Nano Lett., 2010, 10, 380–384 CrossRef PubMed.
- X.-J. Huang, L. Aldous, A. M. O’Mahony, F. Javier del Campo and R. G. Compton, Anal. Chem., 2010, 82, 5238–5245 CrossRef CAS PubMed.
- A. A. J. Torriero and A. M. Bond, Electroanalytical Chemistry Research Trend, ed. K. Hayashi, Nova Science Publisher Inc., New York, 2009, pp. 1–63 Search PubMed.
- S. O’Toole, S. Pentlavalli and A. P. Doherty, J. Phys. Chem. B, 2007, 111, 9281–9287 CrossRef PubMed.
- M. T. Carter, C. L. Hussey, S. K. D. Strubinger and R. A. Osteryoung, Inorg. Chem., 1991, 30, 1149–1151 CrossRef CAS.
- A. S. Barnes, E. I. Rogers, I. Streeter, L. Aldous, C. Hardacre, G. G. Wildgoose and R. G. Compton, J. Phys. Chem. C, 2008, 112, 13709–13715 CAS.
- R. G. Evans, O. V. Klymenko, S. A. Saddoughi, C. Hardacre and R. G. Compton, J. Phys. Chem. B, 2004, 108, 7878–7886 CrossRef CAS.
- M. Hayyan, F. S. Mjalli, M. A. Hashim and I. M. AlNashef, Ind. Eng. Chem. Res., 2012, 51, 10546–10556 CrossRef CAS.
- Y. Katayama, H. Onodera, M. Yamagata and T. Miura, J. Electrochem. Soc., 2004, 151, A59–A63 CrossRef CAS PubMed.
- Z. Wang, M. Guo, G. A. Baker, J. R. Stetter, L. Lin, A. J. Mason and X. Zeng, Analyst, 2014, 139, 5140–5147 RSC.
- R. Toniolo, N. Dossi, A. Pizzariello, A. P. Doherty and G. Bontempelli, Electroanalysis, 2012, 24, 865–871 CrossRef CAS PubMed.
- C. Xiao, A. Rehman and X. Zeng, Anal. Chem., 2012, 84, 1416–1424 CrossRef CAS PubMed.
- K. Murugappan, C. Kang and D. S. Silvester, J. Phys. Chem. C, 2014, 118, 19232–19237 CAS.
- C. H. Xiao and X. Q. Zeng, J. Electrochem. Soc., 2013, 160, H749–H756 CrossRef CAS PubMed.
- L. E. Barrosse-Antle, A. M. Bond, R. G. Compton, A. M. O’Mahony, E. I. Rogers and D. S. Silvester, Chem.–Asian J., 2010, 5, 202–230 CrossRef CAS PubMed.
- X.-J. Huang, D. S. Silvester, I. Streeter, L. Aldous, C. Hardacre and R. G. Compton, J. Phys. Chem. C, 2008, 112, 19477–19483 CAS.
- L. Lin, A. Rehman and X. Zeng, manuscript in preparation.
- X. Ji, C. E. Banks, D. S. Silvester, L. Aldous, C. Hardacre and R. G. Compton, Electroanalysis, 2007, 19, 2194–2201 CrossRef CAS PubMed.
- C. Hu, X. Bai, Y. Wang, W. Jin, X. Zhang and S. Hu, Anal. Chem., 2012, 84, 3745–3750 CrossRef CAS PubMed.
- R. Toniolo, N. Dossi, A. Pizzariello, A. Casagrande and G. Bontempelli, Anal. Bioanal. Chem., 2013, 405, 3571–3577 CrossRef CAS PubMed.
- M. Schienle, C. Paulus, A. Frey, F. Hofmann, B. Holzapfl, P. Schindler-Bauer and R. Thewes, IEEE J. Solid-State Circuits, 2004, 39, 2438–2445 CrossRef.
- X. Zhu and C. H. Ahn, IEEE Sens. J., 2006, 6, 1280–1286 CrossRef CAS.
- L. Li, X. Liu, W. A. Qureshi and A. J. Mason, IEEE Transaction on Biomedical Circuits and Systems, 2011, 5, 439–448 CrossRef PubMed.
- N. Trombly and A. Mason, Electron. Lett., 2008, 44, 29–30 CrossRef.
- C. Yang, Y. Huang, B. L. Hassler, R. M. Worden and A. J. Mason, IEEE Transaction on Biomedical Circuits and Systems, 2009, 3, 160–168 CrossRef PubMed.
- T. Ohsaka, M. T. Alam, M. M. Islam and T. Okajima, Electrochem. Commun., 2007, 9, 2370–2374 CrossRef PubMed.
- R. Toniolo, N. Dossi, R. Svigely, S. Susmel, I. G. Casella and G. Bontempelli, Electroanalysis, 2014, 26, 1966–1974 CrossRef CAS PubMed.
- J. Gębicki, A. Kloskowski and W. Chrzanowski, Sens. Actuators, B, 2013, 177, 1173–1179 CrossRef PubMed.
- J. Niedziolka, E. Rozniecka, J. Stafiej, J. Sirieix-Plenet, L. Gaillon, D. di Caprio and M. Opallo, Chem. Commun., 2005, 2954–2956, 10.1039/b502194d.
- J. D. Wadhawan, U. Schröder, A. Neudeck, S. J. Wilkins, R. G. Compton, F. Marken, C. S. Consorti, R. F. de Souza and J. r. Dupont, J. Electroanal. Chem., 2000, 493, 75–83 CrossRef CAS.
- P. Yu, Y. Lin, L. Xiang, L. Su, J. Zhang and L. Mao, Langmuir, 2005, 21, 9000–9006 CrossRef CAS PubMed.
- S. R. Ng, C. X. Guo and C. M. Li, Electroanalysis, 2011, 23, 442–448 CrossRef CAS PubMed.
- Y. Peng, Y. Ji, D. Zheng and S. Hu, Sens. Actuators, B, 2009, 137, 656–661 CrossRef CAS PubMed.
- C. X. Guo, Z. S. Lu, Y. Lei and C. M. Li, Electrochem. Commun., 2010, 12, 1237–1240 CrossRef CAS PubMed.
- C. Liang, C.-Y. Yuan, R. J. Warmack, C. E. Barnes and S. Dai, Anal. Chem., 2002, 74, 2172–2176 CrossRef CAS.
- L. Yu, D. Garcia, R. B. Rex and X. Q. Zeng, Chem. Commun., 2005, 2277–2279 RSC.
- Y. Yan, X. Jiang, H. Zhou, L. Wu, Q. Wu, L. Zhang and L. Cai, Fresenius Environ. Bull., 2014, 23, 1198–1202 CAS.
- R. Toniolo, A. Pizzariello, N. Dossi, S. Lorenzon, O. Abollino and G. Bontempelli, Anal. Chem., 2013, 85, 7241–7247 CrossRef CAS PubMed.
- Z. Zhang and R. G. Reddy, EPD congress 2002, ed. P.R. Taylor, TMS, Warrendale, PA, 2002, p. 199 Search PubMed.
- X. Jin, L. Yu, D. Garcia, R. X. Ren and X. Zeng, Anal. Chem., 2006, 78, 6980–6989 CrossRef CAS PubMed.
- T. Yamauchi, T. Matsui, T. Nishiyama, K. Tsunashima, N. Tsubokawa and S. Harada, ECS Trans., 2013, 50, 231–236 CrossRef PubMed.
- I. Goubaidoulline, G. Vidrich and D. Johannsmann, Anal. Chem., 2004, 77, 615–619 CrossRef PubMed.
- L. Yu, X. Jin and X. Zeng, Langmuir, 2008, 24, 11631–11636 CrossRef CAS PubMed.
- X. Zeng, X. Li, L. Xing, X. Liu, S. Luo, W. Wei, B. Kong and Y. Li, Biosens. Bioelectron., 2009, 24, 2898–2903 CrossRef CAS PubMed.
- F. Xi, L. Liu, Q. Wu and X. Lin, Biosens. Bioelectron., 2008, 24, 29–34 CrossRef CAS PubMed.
- R. Gao and J. Zheng, Electrochem. Commun., 2009, 11, 608–611 CrossRef CAS PubMed.
- Y. Liu, L. Shi, M. Wang, Z. Li, H. Liu and J. Li, Green Chem., 2005, 7, 655–658 RSC.
- X. Wu, B. Zhao, P. Wu, H. Zhang and C. Cai, J. Phys. Chem. B, 2009, 113, 13365–13373 CrossRef CAS PubMed.
- C. Coll, R. Martinez-Manez, M. D. Marcos, F. Sancenon and J. Soto, Angew. Chem., Int. Ed., 2007, 46, 1675–1678 CrossRef CAS PubMed.
- C. Coll, J. V. Ros-Lis, R. Martinez-Manez, M. D. Marcos, F. Sancenon and J. Soto, J. Mater. Chem., 2010, 20, 1442–1451 RSC.
- S. Aydogdu, K. Ertekin, A. Suslu, M. Ozdemir, E. Celik and U. Cocen, J. Fluoresc., 2011, 21, 607–613 CrossRef CAS PubMed.
- S. Das, P. K. S. Magut, S. L. de Rooy, F. Hasan and I. M. Warner, RSC Adv., 2013, 3, 21054–21061 RSC.
- C. Zhang, D. P. Bailey and K. S. Suslick, J. Agric. Food Chem., 2006, 54, 4925–4931 CrossRef CAS PubMed.
- L. A. Feng, C. J. Musto, J. W. Kemling, S. H. Lim, W. X. Zhong and K. S. Suslick, Anal. Chem., 2010, 82, 9433–9440 CrossRef CAS PubMed.
- B. A. Suslick, L. Feng and K. S. Suslick, Anal. Chem., 2010, 82, 2067–2073 CrossRef CAS PubMed.
- O. Oter, K. Ertekin, D. Topkaya and S. Alp, Anal. Bioanal. Chem., 2006, 386, 1225–1234 CrossRef CAS PubMed.
- O. Oter, K. Ertekin and S. Derinkuyu, Talanta, 2008, 76, 557–563 CrossRef CAS PubMed.
- J. Jang and W.-Y. Lee, J. Electroanal. Chem., 2015, 736, 55–60 CrossRef CAS PubMed.
- L. Chen, Y. Zhang, S. Ren, D. Huang, C. Zhou, Y. Chi and G. Chen, Analyst, 2013, 138, 7006–7011 RSC.
- W. Zhu, W. Li, H. Yang, Y. Jiang, C. Wang, Y. Chen and G. Li, Chemistry, 2013, 19, 11603–11612 CrossRef CAS PubMed.
- W. I. S. Galpothdeniya, K. S. McCarter, S. L. De Rooy, B. P. Regmi, S. Das, F. Hasan, A. Tagge and I. M. Warner, RSC Adv., 2014, 4, 7225–7234 RSC.
- L. Chen, D. Huang, S. Ren, Y. Chi and G. Chen, Anal. Chem., 2011, 83, 6862–6867 CrossRef CAS PubMed.
- L. C. Branco and F. Pina, Chem. Commun., 2009, 6204–6206, 10.1039/b907672g.
- S. G. Zhang, S. M. Liu, Q. H. Zhang and Y. Q. Deng, Chem. Commun., 2011, 47, 6641–6643 RSC.
- H. Tamura, Y. Shinohara and T. Arai, Chem. Lett., 2010, 39, 240–241 CrossRef CAS.
- H. Tamura, Y. Shinohara and T. Arai, Chem. Lett., 2011, 40, 129–131 CrossRef CAS.
- J. Avo, L. Cunha-Silva, J. C. Lima and A. J. Parola, Org. Lett., 2014, 16, 2582–2585 CrossRef CAS PubMed.
- V. W. W. Yam, J. K. W. Lee, C. C. Ko and N. Y. Zhu, J. Am. Chem. Soc., 2009, 131, 912–913 CrossRef CAS PubMed.
- J. L. Anthony, J. L. Anderson, E. J. Maginn and J. F. Brennecke, J. Phys. Chem. B, 2005, 109, 6366–6374 CrossRef CAS PubMed.
- P. J. Dyson, G. Laurenczy, C. A. Ohlin, J. Vallance and T. Welton, Chem. Commun., 2003, 2418–2419 RSC.
- A. Yokozeki and M. B. Shiflett, Energy Fuels, 2009, 23, 4701–4708 CrossRef CAS.
- E. D. Bates, R. D. Mayton, I. Ntai and J. H. Davis Jr, J. Am. Chem. Soc., 2002, 124, 926–927 CrossRef CAS PubMed.
- M. D. Soutullo, C. I. Odom, B. F. Wicker, C. N. Henderson, A. C. Stenson and J. H. Davis, Chem. Mater., 2007, 19, 3581–3583 CrossRef CAS.
- D. Lu, N. Shomali and A. Shen, Electrochem. Commun., 2010, 12, 1214–1217 CrossRef CAS PubMed.
- C. Hagleitner, A. Hierlemann, D. Lange, A. Kummer, N. Kerness, O. Brand and H. Baltes, Nature, 2001, 414, 293–296 CrossRef CAS PubMed.
- F. Ma, A. Rehman, H. Liu, J. Zhang, S. Zhu and X. Zeng, Anal. Chem., 2015, 87, 1560–1568 CrossRef CAS PubMed.
- S. Kazunari, U. Akihiro, H. Ryo, B. Akira, O. Yasuo, K. Keizo and K. Futao, Jpn. J. Appl. Phys., 2013, 52, 05DC20 CrossRef.
- T. Goda, Y. Maeda and Y. Miyahara, Anal. Chem., 2012, 84, 7308–7314 CrossRef CAS PubMed.
- J. R. Askim, M. Mahmoudi and K. S. Suslick, Chem. Soc. Rev., 2013, 42, 8649–8682 RSC.
- E. S. Snow and F. K. Perkins, Nano Lett., 2005, 5, 2414–2417 CrossRef CAS PubMed.
- S. Brahim, S. Colbern, R. Gump and L. Grigorian, J. Appl. Phys., 2008, 104, 024502 CrossRef PubMed.
- D. C. Meier, B. Raman and S. Semancik, Annu. Rev. Anal. Chem., 2009, 2, 463–484 CrossRef CAS PubMed.
- A. R. Hillman, J. Solid State Electrochem., 2011, 15, 1647–1660 CrossRef CAS PubMed.
- E. S. Forzani, D. Lu, M. J. Leright, A. D. Aguilar, F. Tsow, R. A. Iglesias, Q. Zhang, J. Lu, J. Li and N. Tao, J. Am. Chem. Soc., 2009, 131, 1390–1391 CrossRef CAS PubMed.
|
This journal is © The Royal Society of Chemistry 2015 |