DOI:
10.1039/C5RA02311D
(Paper)
RSC Adv., 2015,
5, 42233-42241
Lanthanum breaks the balance between osteogenesis and adipogenesis of mesenchymal stem cells through phosphorylation of Smad1/5/8†
Received
6th February 2015
, Accepted 5th May 2015
First published on 5th May 2015
Abstract
In the presence of lanthanum (La), the balance between osteogenesis and adipogenesis was broken in mouse mesenchymal stem cells (MSCs) in vitro, resulting in osteogenic induction and adipogenic inhibition, which were verified by alkaline phosphatase (ALP) activity and calcium secretion, and oil red O staining assays, respectively. The molecular data revealed that La up-regulated the expression levels of osteogenesis-associated genes and proteins, while it inhibited the expression of adipogenesis-associated genes and proteins. Furthermore, the mechanism of La on bone metabolism was investigated by screening 84 genes involved in differentiation of MSCs. The results showed that Smad1/5/8, a key protein involved in the bone morphogenetic protein (BMP) signaling pathway, was significantly phosphorylated under the exposure of La, leading to the activation of the BMP signaling pathway with subsequent up-regulation of the osteogenic genes and the down-regulation of the adipogenic genes.
1. Introduction
Lanthanum (La) belongs to the lanthanide series, has been recognized as a “bone-seeking” element because its physicochemical properties are similar to calcium.1,2 Growing evidence suggests that La might influence bone minerals through entering the crystal lattice of hydroxyapatite or depositing on the crystal surface. Lanthanum-contained titanium (Ti) alloys and apatites have been demonstrated to have attractive performance and bioactivity, especially with respect to the viability of cells such as osteoblasts, and they are therefore considered to be promising materials for bone implants.3 It has been reported that sheet-shaped lanthanum-doping hexagonal mesoporous silica (La-HMS) successfully promoted the osteogenesis of mouse MSCs by releasing of La3+ and SiO44−.4 Wang et al. reported that La tended to deposit in bone and enhanced osteoblastic differentiation by promoting osteocalcin secretion and matrix mineralization.5 Lanthanum carbonate (FOSRENOL) has been approved by the US Food and Drug Administration (FDA) for the treatment of hyperphosphatemia in renal failure and dialysis patients.6,7 Recent follow-up data showed that long-term use of lanthanum carbonate was safe and that it even suppressed bone abnormalities in these patients, a finding which was further confirmed in an animal model of osteoporosis.8 It was reported that lanthanum had positive effects on proliferation, differentiation, and osteogenic activity of osteoblasts within a certain concentration range.9–11 In addition, lanthanum carbonate had an impact on osteoclast suppression, by which the osteogenic differentiation was induced and phosphate retention was decreased.12,13 To date, the molecular mechanisms of La' effects on bone metabolism remain largely unknown.
Mesenchymal stem cells (MSCs) are multipotent stromal cells that can differentiate into a variety of mesoderm cell types, such as osteoblasts, adipocytes, and chondrocytes, etc. Generally, there appears to be a reciprocal relationship between osteoblasts and adipocytes in MSCs. Therefore, a hypothesis has been proposed that an increase in the number of adipocytes occurs at the cost of osteoblasts in osteopenic disorders.14 Thus, lineage determination between osteoblasts and adipocytes may be a critical step in the regulatory pathways of osteogenesis. Furthermore, the experimental data suggest that medullary adipocytes are secretory cells that may influence hematopoiesis and osteogenesis. Benayahu et al. reported that the conditional medium harvested from mouse stromal preadipocytes decreased the ALP activity of a mouse stromal osteoblastic cell line.15 Ailhaud G et al. reported that adipocytes could synthesize and release a variety of peptide and nonpeptide compounds or secrete cytokines such as tumor necrosis factor-α (TNF-α), which could stimulate bone resorption.16 So it was reported that there might be a therapeutic opportunity to either prevent or treat osteopenic disorders by inhibiting adipogenesis with a concomitant increase in osteogenesis of MSCs.17 Until now, whether and how La has a potential effect on bone metabolism by modulating osteogenic and adipogenic differentiation of MSCs have not been reported.
In the present study, we investigated the effects of La on the osteogenic and adipogenic differentiation of MSCs and the associated molecular mechanisms. The cellular effects of La on the proliferation, osteogenic differentiation, adipogenic differentiation, and mineralization of MSCs were evaluated by 3-(4,5-dimethyl-2-thiazolyl)-2,5-diphenyl-2H tetrazolium bromide (MTT), ALP activity, oil red O staining and alizarin red staining (ARS) assays, respectively. On the basis of the cellular effects, Q-PCR and western blot analysis were designed to elucidate the interactional mechanism between La and MSCs by determining the expression of genes and proteins related to pathways. More importantly, we evaluated the treatment efficacy of La on the phosphorylation of Smad1/5/8. We found that the increased p-Smad1/5/8 greatly activated the BMP signaling pathway, which accelerated the osteogenic differentiation of MSCs, meanwhile, inhibited the adipogenic differentiation in the presence of La.
2. Experimental section
2.1 Mouse-derived mesenchymal stem cells isolation and culture
MSCs were extracted from femora and tibia bone marrow from 4–6 weeks specific pathogen free (SPF) Kunming (KM) female mice as previously described.18 KM female mice were obtained from Medical Laboratory Animal Center of Hebei Province. All animals have been received permission from the Institutional Animal Care and Use Committee. The isolated MSCs were incubated in Dulbecco's modified eagle medium (DMEM, Gibco) with 10% fetal bovine serum (FBS, Gibco), 100 U mL−1 penicillin, and 100 μg mL−1 streptomycin (Life technologies) for 3 days in a humidified atmosphere of 5% CO2 in air at 37 °C, then suspended cells were removed by changing the medium.
2.2 Cell viability assay
The MSCs viability was investigated by MTT (Sigma-Aldrich) according to the protocol in previous work.19 In brief, cells were seeded at a density of 4 × 103 cells per well in a 96 well plate and incubated overnight. Subsequently, fresh medium containing lanthanum(III) chloride heptahydrate (purity 99.999%, Sigma-Aldrich) at different concentrations (0, 0.0001, 0.001, 0.01, 0.1, and 1 μM) was added to replace the culture medium for 48 and 72 h. After incubation, 20 μL of MTT (5.0 mg mL−1 in 1× phosphate buffered saline (PBS)) was added and incubated for another 4 h at 37 °C. The supernatant was removed, and dimethyl sulfoxide (DMSO, Sigma-Aldrich) was added to each well for 10 min to dissolve formazan crystals. The optical density (OD) was measured using a microplate reader (Biorad model680, USA) at 570 nm. The MSCs viability (%) was expressed as a percentage of [ODsample − ODblank]/[ODcontrol − ODblank] × 100.
2.3 Alkaline phosphatase activity assay
MSCs were seeded in 48 well plates at a density of 5 × 106 cells per well and then treated with osteogenic supplements (OS) (5.0 mM β-glycerophosphate, 50 μg mL−1 ascorbic acid and 0.1 μM dexamethasone, Sigma-Aldrich) and a series of dilutions of LaCl3 (final concentration 0.0001, 0.001, 0.01, 0.1, and 1 μM). MSCs treated with only OS were used as control group. NaF (1.0 μM, Sigma, USA), which can promote osteogenetic differentiation of MSCs, was used as a positive control. After incubation, ALP was measured on days 7, 10, and 21 according to the protocol described previously.20 All results were normalized by protein content.
2.4 Alizarin red S staining
5 × 106 MSCs were seeded into each well of a 48 well tissue culture plate, cultured for 3 days, and then changed to medium containing OS and LaCl3 (final concentration 0.0001, 0.001, 0.01, 0.1, 1, and 10 μM) for 14, 18 and 22 days. Mineralized matrix formation was evaluated using a previously described method.21 To quantify the extent of matrix mineralization, ARS-stained nodules were incubated in 10%(w/v) cetylpyridiumchloride for 10 min and the OD value was measured at 570 nm by a microplate reader.
2.5 Oil red O staining
MSCs (1 × 107 cells per well) were seeded in 48 well tissue culture plates exposed to LaCl3 (final concentration 0.0001, 0.001, 0.01, 0.1, and 1 μM) for 15, 18 and 21 days. The adipogenesis of MSCs were performed at 3 days post-confluence (designated as day 0) by the addition of DMEM (10% fetal bovine serum) containing 10 mg L−1 insulin, 1.00 × 10−7 mol L−1 dexamethasone, and 0.5 mM methyl-isobutylxanthine. After 3 days incubation, cells were grown in media containing only insulin and dexamethasone for another 12, 15, and 18 days. After 15–21 days incubation, the adipocytes differentiated from MSCs were stained by 0.6% (w/v) oil red O (Sigma-Aldrich) for 15 min and then quantified by dissolving the oil red O in isopropyl alcohol. The oil red O concentration in the extraction buffer was measured by a microplate reader at 510 nm. The adipogenic differentiation inhibition rate (%) was expressed as a percentage of [ODsample − ODblank]/[ODcontrol − ODblank] × 100.21
2.6 Quantitative real-time polymerase chain reaction
Following the 0.0001, 0.01, and 1 μM LaCl3 treatment in the presence of OS or AS for 4 days, total RNA was extracted from MSCs using Trizol reagent (Life technologies), followed by cDNA synthesis according to the TaKaRa protocol. All values were normalized to glyceraldehyde-3-phosphate dehydrogenase (GAPDH) levels by the CT (2−ΔΔCT) method.22 The sequences of primers are listed in Table 1. Reaction conditions and relative quantification have been performed as previously described.20,22
Table 1 qRT-PCR primers
Gene symbol |
Forward primer (5′-3′) |
Reverse primer (5′-3′) |
Runx-2 |
TTCTCCAACCCACGAATGCAC |
CAGGTACGTGTGGTAGTGAGT |
BMP-2 |
TGGCCCATTTAGAGGAGAACC |
AGGCATGATAGCCCGGAGG |
ALP |
GTTGCCAAGCTGGGAAGAACAC |
CCCACCCCGCTATTCCAAAC |
BSP |
GAATCCACATGCCTATTGC |
AGAACCCACTGACCCATT |
OCN |
GAACAGACTCCGGCGCTA |
AGGGAGGATCAAGTCCCG |
Col I |
AACATGACCAAAAACCAAAAGTG |
CATTGTTTCCTGTGTCTTCTGG |
ERα-1 |
GAATCCACATGCCTATTGC |
AGAACCCACTGACCCATT |
GAPDH |
GACTTCAACAGCAACTCCCAC |
TCCACCACCCTGTTGCTGTA |
PPARγ2 |
TGTGGGGATAAAGCATCAGGC |
CCGGCAGTTAAGATCACACCTAT |
C/EBPα |
GTGCTTCATGGAGCAAGCCAA |
TGTCGATGGAGTGCTCGTTCT |
C/EBPβ |
GCGCGAGCGCAACAACATCG |
CAGCACAGGCTGTTGACCATCATA |
C/EBPδ |
GAGCGTCCTACGCGCCAGTAC |
GATCACGGAGCTGTGCCGGTC |
2.7 RT2 Profiler PCR arrays for mechanism study
An RT2 Profiler PCR Array (Qiagen) containing 84 relevant pathway focused genes of differentiation of MSCs was performed using ABI 7300 Sequence Detection System (Applied Biosystems, USA) according to the manufacturer's protocol. PCR products were analyzed with PCR array data analysis web portal from the following address: http://www.SABiosciences.com/pcrarraydataanalysis.php. All values were normalized to GAPDH levels by the CT (2−ΔΔCT) method.22
2.8 Western blot analysis
MSCs (1 × 106 cells per well) were cultured overnight in 6 well plates in normal culture medium and then treated with 0.0001, 0.01, and 1 μM LaCl3 in the presence of OS or AS for 4 days, respectively. Subsequently, cells were washed with cold PBS and lysed in cold 50 mM Tris–HCl (pH 7.4), 10 mM ethylene diamine tetraacetie acid (EDTA), 4.3 M Urea and 1%Triton X-100 buffer. Equal amounts of protein from each sample were separated by 10% SDS-PAGE gel and then transferred onto nitrocellulose membranes. These membranes were then blocked with a buffer containing 5% bovine serum albumin in TBST solution (10 mM Tris–HCl pH 8.0, 150 mM NaCl, 0.05% Tween-20) for 2 h, and then incubated with primary antibodies (Santa Cruz Biotechnology) overnight at 4 °C. After a second washing with TBST, membranes were incubated with peroxidase-conjugated secondary antibodies and developed with a chemiluminescence detection kit (Thermo Fisher Scientific). β-Actin was used as a loading control. The OD of bands was quantified by LAS-1000 image analyzer (Fuji-Film) software.
2.9 Statistical analysis
Each sample was assayed in quadruplicate, with each experiment repeated at three times independently. Data were collected from three separate experiments and presented as means ± standard deviation (SD). Significant differences were determined by one-way ANOVA and Mann–Whitney U test. P values less than 0.05 were considered to indicate statistical differences.
3. Results
3.1 La promotes MSCs proliferation
MSCs were exposed to various concentrations of La, where low dose La caused significant increase of proliferation, showing a dose dependent stimulation of proliferation after short-term (1–3 days) incubation. As shown in Fig. 1, on day 2 and 3, La promoted MSCs proliferation at all concentrations. Especially, 8% and 15% proliferation rates were observed at 0.001 μM on day 2 and 3, respectively.
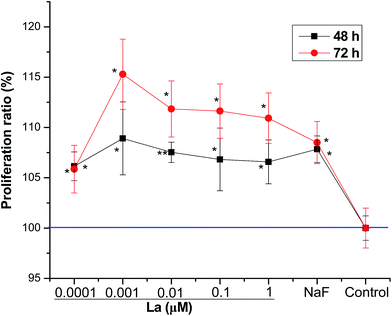 |
| Fig. 1 Effects of La on the proliferation of MSCs. MSCs were treated with various concentrations of La for 2 and 3 days. Data are presented as mean ± SD from a representative of three separate experiments performed in quadruplicate. *P < 0.05, **P < 0.01 compared with the control. | |
3.2 Effect of La on the ALP activity of MSCs
In osteogenic induction experiments, cultures of MSCs with OS were treated with different concentrations of La and collected at various time points for ALP activity assays. La treatment appeared to simulate osteogenic differentiation of MSCs on the basis of ALP activity. As shown in Fig. 2, low concentrations of La-treated cells exhibited significantly higher ALP activity compared to the untreated control cells at day 7 and 14. During the first 7 days, the ALP activities had a slight rise at concentrations of 0.0001, 0.001, and 0.01 μM but remained in low levels. However, there were no significant differences when cells treated with 0.1 and 1 μM La. Although a pick of ALP activity was observed on the day 10, no significant differences were observed after treatment of 0.0001, 0.001, 0.01, and 0.1 μM La. After that, the expression of ALP activity was promoted on day 21 after 0.0001, 0.001, 0.01, and 0.1 μM La treatments, showing dose-dependent manner.
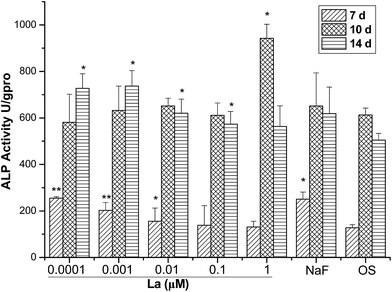 |
| Fig. 2 Effect of La on the ALP Activity of MSCs. ALP activity in La-treated MSCs was significantly higher than that of the control. Data are presented as mean ± SD from a representative of three separate experiments performed in quadruplicate. *P < 0.05, **P < 0.01 compared with the control. | |
3.3 Effect of La on the mineralization of MSCs
Mineralization of MSCs was determined by observation of the MSCs for mineral deposits and was confirmed by ARS staining for calcium, which is useful in evaluating osteogenesis at later phase of differentiation. As shown in Fig. 3A–D, there were more mineralized nodules in La-treated MSCs groups than in control group for 14 days. Quantification of mineralized nodules in secreted mineral matrix showed a maximum value of 1.8-fold increase in mineralization when MSCs were treated with 0.001 μM La on day 18 (Fig. 3E). This indicated the potential of La to induce bone formation.
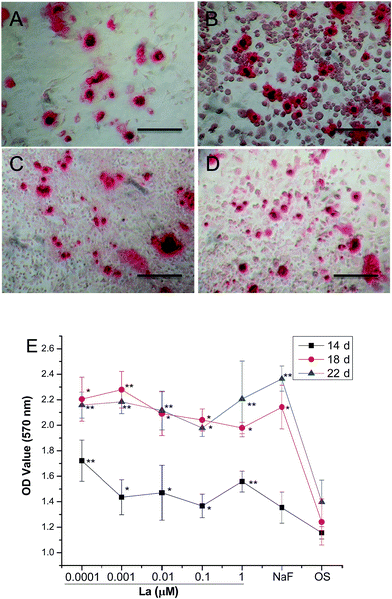 |
| Fig. 3 (A–D) ARS staining of osteogenic differentiation for 14 days; (A) cells treated with OS only; (B) cells treated with 0.01 μM La + OS; (C) cells treated with 0.1 μM La + OS; (D) cells treated with 1 μM La + OS, scale bar = 50 μm, original magnification = 100×; (E) time-dependent effect of La on the mineralization. Data are presented as mean ± SD from a representative of three separate experiments performed in quadruplicate. The statistical significance was calculated by the method of Mann–Whitney U test. *P < 0.05, **P < 0.01 compared with the OS group. | |
3.4 Effect of La on adipogenic differentiation of MSCs
Fig. 4A–D illustrates oil red O staining of adipogenic differentiation of MSCs after 18 days La treatment. MSCs were cultured with AS medium and the presence of lipid-rich droplets throughout the cytoplasm of differentiated cells colored red by oil red O staining. Majority of the cells were oil red O positive in the AS group and the number of positive stained cells was higher than that in La treatment. The intracellular lipid accumulation was determined by examination with light microscopy and absorbance measurement at 490 nm after oil red O staining. The quantitative results (Fig. 4E) revealed that the presence of La inhibited adipogenic differentiation of MSCs at most tested concentrations, but had no obvious dose- or time-dependent tendency. Generally, MSCs treated with La failed differentiate into adipocytes, that is, La broke the balance of adipogenesis and osteogenesis of MSCs.
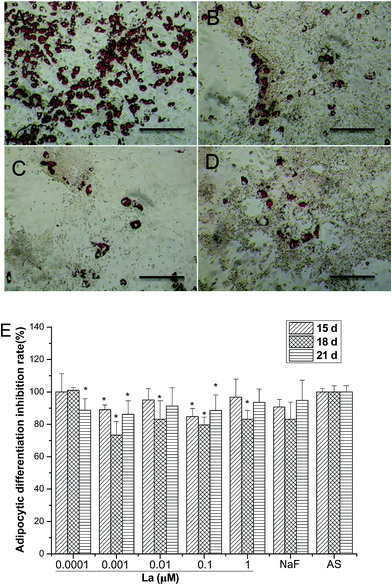 |
| Fig. 4 (A–D) Photographs of adipocytes stained by oil red O; (A) cells treated with AS only; (B) cells treated with AS+0.01 μM La; (C) cells treated with AS+0.1 μM La; (D) cells treated with AS+1 μM La, scale bar = 50 μm; (E) adipogenic differentiation rate was performed by quantification of oil red O content. Data are presented as mean ± SD from a representative of three separate experiments performed in quadruplicate. *P < 0.05 compared with the AS group. | |
3.5 Osteogenic differentiation-related genes expression analysis
To analyze expression of osteogenic differentiation-related genes, seven main differentiation markers were evaluated. As shown in Fig. 5, the expression of osteogenesis-related genes, such as runt-related transcription factor 2 (Runx2), BMP-2, ALP, osteocalcin (OCN), and estrogen receptor alpha (ERα) were significantly improved in MSCs treated with La compared to control. Specially, highest relative fold difference was shown by BMP-2, which indicated 13-fold difference in MSCs treated with 0.01 μM La compared to OS group. However, for the level of bone sialoprotein (BSP) and collagen I (COLI), there were no significant differences among the groups.
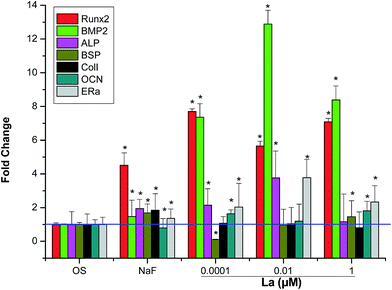 |
| Fig. 5 Osteogenic differentiation-related genes transcription determined by real time PCR. The osteogenesis-related genes were up-regulated after treatments of La. Data are presented as mean ± SD from a representative of three separate experiments performed in quadruplicate. *P < 0.05 compared with the control group. | |
3.6 Adipogenic differentiation-related genes expression analysis
Adipogenic differentiation-related genes expression has been extensively investigated using real time PCR. As shown in Fig. 6, several adipogenic-related genes (such as CCAAT/enhancer binding protein (C/EBP)α, C/EBPβ, C/EBPδ, and Peroxisome proliferator-activated receptor gamma (PPARγ2)) were significantly down-regulated in the La-treated (0.0001, 0.01, and 1 μM) MSCs for 4 days compared to AS group. Significant differences of adipogenic differentiation-related expression may imply that adipogenic differentiation of MSCs was suppressed by La treatment. Taken together, these results confirmed the hypothesis that the promotion of osteogenesis occurs concurrently with the inhibition of adipogenesis due to the reciprocal relationship between osteogenic and adipogenic differentiation of MSCs.
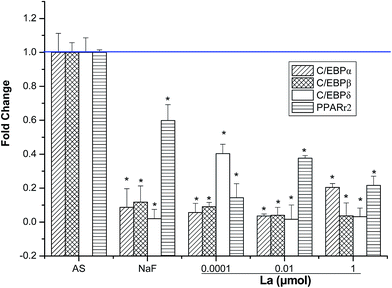 |
| Fig. 6 Adipogenic differentiation-related genes transcription determined by real time PCR. The adipogenesis-related genes were down-regulated after treatments of La. Data are presented as mean ± SD from a representative of three separate experiments performed in quadruplicate. *P < 0.05 compared with the control group. | |
3.7 La improves osteogenic proteins expression, but attenuates the adipogenic proteins expression
The level of proteins associated with osteogenesis of MSCs upon osteogenic induction was showed in Fig. 7A and B. The quantification of proteins expression was showed in Fig. 7B. The expression of BMP-2, Runx2, and OCN were up-regulated after 4 days La treatment (Fig. 7A and B), except the expression of Runx2 that treated with 1 μM La. A significant reduction expression of C/EBPα, C/EBPδ, and PPARγ in MSCs demonstrated intense inhibition of adipogenic differentiation by 4 days La treatment (Fig. 7C and D).
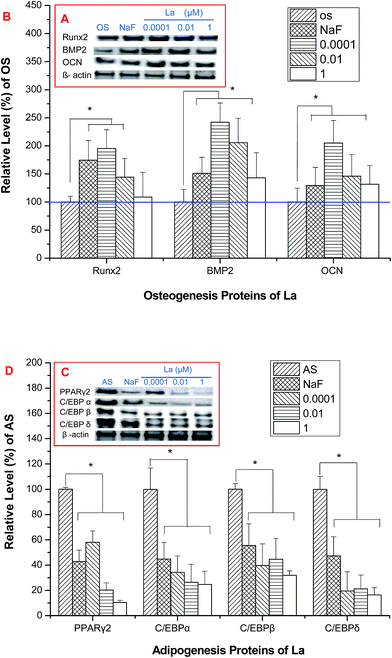 |
| Fig. 7 (A) Western blot analysis for BMP2, Runx2, and OCN proteins; (B) quantification of the blots for BMP2, Runx2, and OCN proteins; (C) western blot analysis for PPARγ2, C/EBPα, C/EBPβ and C/EBPδ proteins; (D) quantification of the blots for PPARγ2, C/EBPα, C/EBPβ and C/EBPδ proteins. Data are presented as mean ± SD from a representative of three separate experiments performed in quadruplicate. *P < 0.05 compared with the control. | |
3.8 La-induced Smad1/5/8 phosphorylation is regulated by BMP signaling pathway
MSCs treated with La were collected and screened for the expression of 84 genes linked to differentiation of MSCs. The data reflected that MSCs had a pattern of constitutive expression for the majority of osteogenic genes. As shown in Fig. 8, thirty-one genes of MSCs tested using RT2 Profiler™ PCR Array showed either up- or down-regulated expression after treatment with 0.0001 μM La. Of these, twenty-three related to osteogenic differentiation were up-regulated, such as BMP2, Runx2, and Col 1a1. On the other hand, some genes levels, including Gdf15, Gdf5, Gdf6, Gdf7, PPARγ, and Ins2 were down-regulated by treatment of La, in which PPARγ and Ins2 associated with adipogenesis of MSCs. 13 out of the 23 genes that were found to be highly expressed are involved in BMP signalling, such as Smad4, Bmp7, Bmp6, Bmp4, and Bmp2. On the other hand, the expression of Smurf1, Smurf2, Gdf7, Gdf6, Gdf5, and Gdf15 was down-regulated (see ESI, Fig. S1 and Tables S1–S3† for descriptions of all genes and their functions).
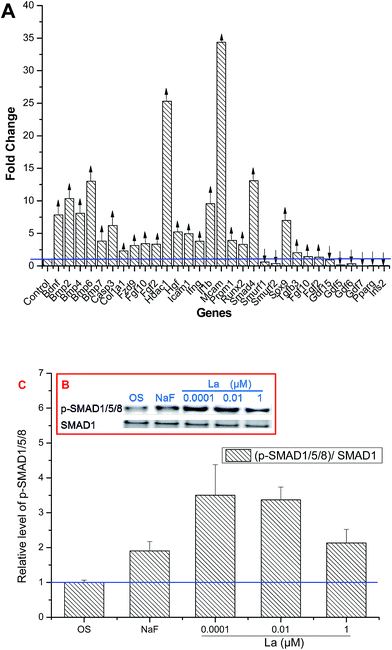 |
| Fig. 8 (A) Gene expression profiles in differentiation of MSCs. MSC had a pattern of constitutive expression for the majority of osteogenic genes; (B) a representative western blot result of p-Smad1/5/8 in MSCs in the presence or absence of 0.0001, 0.01, and 1 μM La; (C) quantification of the blots for p-Smad1/5/8/Smad1 protein. Data are presented as mean ± SD from a representative of three separate experiments performed in quadruplicate. *P < 0.05 compared with the control. | |
To examine whether the BMP pathway was involved in regulating of La-induced MSCs differentiation, western blot was used to detect this signaling pathway. Smad1/5/8, key proteins involved in BMP pathway, form a complex with Smad4 that subsequently regulates specific genes transcription. Since Smad-mediated genes transcription requires Smad phosphorylation, and then the levels of p-Smad1/5/8 was examined by western blot. Fig. 8B and C showed that Smad1/5/8 was efficiently phosphorylated in response to 0.0001 μM La treatment.
4. Discussion
With the application of La in the industry, agriculture and biomedical fields, a variety of biochemical and physiological effects of La have been studied. However, the potential effects and mechanism of La on MSCs homeostasis are not well elaborated. MSCs have great potential to differentiate into many different cells types, such as osteoblast, adipocyte, and chondrocyte. MSCs maintain their homeostasis through a comprehensive signaling network. Any perturbation of this system will influence cell function and behavior.23 In this study, the proliferation of MSCs was promoted after 2 and 3 days La treatment at all concentrations (Fig. 1). Subsequently, the differentiation balance of MSCs was disrupted by La, showing the osteogenic differentiation promotion and adipogenic differentiation inhibition. Both the early stage marker (ALP activity) and later stage marker (mineralization) of osteogenesis were significantly promoted in MSCs upon treatment of La (Fig. 2 and 3). Conversely, the adipogenic differentiation of MSCs was inhibited by La without obvious dose- or time-dependent tendency (Fig. 4). Furthermore, to analyse expression of osteogenic differentiation-related genes, seven main differentiation markers, including Runx2, BMP-2, ALP, OCN, ERα, were evaluated. La exhibited the up-regulation of all these osteogenic differentiation-related specific genes and proteins (Fig. 4 and 7A), which was consistent with the observed promotion effects of La on ALP activity (Fig. 2) and mineralized matrix nodule formation of MSCs (Fig. 3). A hypothesis proposed that there is a reciprocal relationship between the osteogenesis and adipogenesis of MSCs, it is expected that the promotion of osteogenic differentiation may result in the inhibition of adipogenic differentiation of MSCs. It has been reported that Runx2 determines the osteogenesis in MSCs, while PPARγ contributes to adipogenesis.19,21 Specific activation of PPARγ2 leads to suppression of the main transcription factors of osteogenesis, and also to increase conversion of MSCs into adipocytes.19,24 Adipogenic differentiation of MSCs starts with the transient expression of C/EBPβ and C/EBPδ, which activates C/EBPα and PPARγ. C/EBPα and PPARγ together coordinate the expression of adipogenic genes underlying the phenotype of terminally differentiated adipocytes.21 The expression of adipogenesis-associated genes and proteins is expected to be inhibited in the presence of La. In the present work, the expression of adipogenesis-associated genes and proteins was also down-regulated when MSCs were cultured in the presence of La (Fig. 6 and 7B), which is consistent with the observed inhibitory effects of La on adipogenic differentiation of MSCs in cellular level (Fig. 4). Taken together, these results confirmed the hypothesis that the promotion of osteogenesis occurs concurrently with the inhibition of adipogenesis due to the reciprocal relationship between osteogenic and adipogenic differentiation of MSCs.
A question was proposed that which species plays an important role in regulating the osteogenic and adipogenic differentiation of MSCs by La? It is generally known that pH 7.4 is a suitable condition for cell culture in vitro and the normal cell culture medium usually includes 0.91 mM PO43−, which is similar to the concentration of phosphate in blood. A growing of evidence suggested that “precipitation problem” was involved in cellular studies of the biological effects of multivalent metal cations.25 Li et al.26 reported that gadolinium ions formed gadolinium and phosphorous-containing nanoparticles or microparticles, which acted as a biologically active entity and deposited on the NIH3T3 cell surface in cell culture medium, resulting in cell cycle progression. The smaller gadolinium particles exhibited a stronger cell-cycle-promoting effect than the larger ones, but they shared the common signaling pathways. Furthermore, the particles may act as a gadolinium “buffer pool”, where Gd3+ ions were released to regulate cell cycle progression in NIH3T3 cells.26 Cerium, another rare earth element, was found to accumulate in the cardiac tissue and serum of patients, of which the biologically active species might be the precipitate of hydroxide or insoluble salts according to their chemical properties.20 La also belongs to the family of rare earth elements, and may have the high possibility to form precipitates at higher concentration under physiological conditions. Therefore, we deduced that La might also generate lanthanum-containing nanoparticles or microparticles to form a “buffer pool”, where La3+ ions were released to modulate the osteogenic and adipogenic differentiation of MSCs.
An increasing evidence indicated that the Smad-dependent BMP signaling pathway has potent effects on osteogenic differentiation of MSCs.27–29 BMPs play a major role in skeletal development and bone formation, and disruptions in BMP signaling cause a variety of skeletal and extraskeletal anomalies. Our previous study showed that Ce and Tb enhanced the osteogenic differentiation of MSCs via activation of TGF-β/BMP signaling pathway.20,22 Therefore, we hypothesized that La might promote osteogenic differentiation of MSCs through a Smad-dependent BMP signaling pathway. Fig. 8A showed that La up-regulated 23 marker genes that control the osteogenic differentiation of MSCs, which was observed by RT2 Profiler™ PCR Array. Interestingly, most of these genes were involved in BMP signaling pathway. Multiple BMPs, including BMP-2, BMP-4, BMP-6, and BMP-7, promote osteogenic differentiation of MSCs both in vitro and in vivo via the phosphorylation of Smad1/5/8, which translocate to the nucleus, bind to target genes, and regulate the transcription. The data shown in Fig. 8B and C indicated that the expression of p-Smad1/5/8 was promoted after treating with La, leading to subsequent up-regulation of the expression of osteogenic differentiation-associated genes. On the other hand, the over-expressed E3 ubiquitin ligases, such as Smurf1 and Smurf2, decreased the expression of Smads and BMPs. In this study, the expression of Smurf1 and Smurf2 was significantly down-regulated in MSCs after La treatment, indicating the subsequent inhibition of proteosomal degradation (Fig. 8A). Cell adhesion molecule (Mcam) belongs to the Ig superfamily, can be employed as a phenotypic marker for MSCs.30 Its higher expression on the cell surface may be linked to greater multipotency and differentiation potential.31,32 Histone deacetylase 1 (Hdac1) and intercellular adhesion molecule 1 (Icam1) are important process for osteogenesis. Lee et al.33 reported that Runx2 physically associated with Hdac1, implying that Runx2 might regulate its target gene expression by recruiting Hdac1 during osteogenic differentiation. In addition, it has been reported that the overexpression of Icam1 significantly enhanced the osteogenic differentiation.34 As shown in Fig. 8A, the expression of Mcam, Hdac1, and Icam1 showed an approximately 32.0-fold, 25.0-fold, and 5-fold increase when MSCs were treated with La, respectively. Hgf has potent anti-apoptotic effects on cells, Rodrigues et al. showed that short-term exposure of Hgf could promote the proliferation of MSCs.35 Additionally, the expression of Hgf showed about 5.0-fold increase when MSCs were treated with La. These data provided the evidence for the fact that La promotes proliferation of MSCs, which is consistent with the observed promotion effects of La on the proliferation of MSCs (Fig. 1). Gdfs are members of the TGF-β superfamily which regulate many aspects of development, including osteogenesis, chondrogenesis, and adipogenesis. It has been reported that the expression of Gdf5, Gdf6, and Gdf7 controls the chondrogenesis and increases proliferation of chondrocytes,36 Gdf15 plays an important role in regulating tenogenesis.37 In our study, the down-regulated expression of Gdf5, Gdf6, Gdf7, and Gdf15 suggested that MSCs treated with La failed to differentiate into chondrocytes and tenocytes (Fig. 8A).
Base on the above analysis, Fig. 9 showed a schematic model to illustrate how the La breaks the balance between osteogenesis and adipogenesis of MSCs through phosphorylating Smad1/5/8 to activate the BMP signaling pathway, which then induces Runx2 transcription to promote the progression of osteogenic differentiation. In response to BMP, Smad1/5/8 bind Smad4 to enhance its followed transcriptional activity, including osteogenesis-related genes Col I and BMP2 at early stages and ALP and OCN at later stages of differentiation.33,38 At the same time, the expression of p-Smad1/5/8 inhibits the adipogenesis in MSCs by suppressing the expression of C/EBPα and PPARγ2, thus driving MSCs to differentiate into osteoblasts.
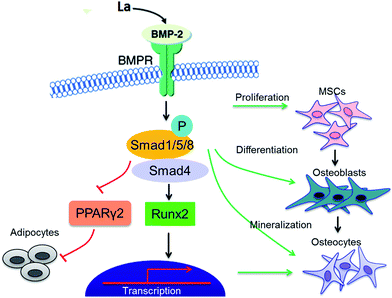 |
| Fig. 9 La breaks the balance between osteogenesis and adipogenesis of MSCs through phosphorylating Smad1/5/8 to activate the BMP signaling pathway. | |
5. Conclusion
In the present study, we have shown that La accelerated the proliferation and osteogenic differentiation of MSCs. La simulated the phosphorylation of Smad1/5/8, which bound Smad4 to accelerate the osteogenic differentiation of MSCs via activation of BMP signaling pathway. Subsequently, the levels of osteogenesis-related genes and proteins were up-regulated in MSCs in presence of La. On the other hand, the expression of a panel of adipogenic differentiation specific genes was found to be significantly down-regulated. From these results, we concluded that La-involved bone metabolism was modulated by phosphorylating Smad1/5/8, and then activated the BMP signaling pathway. These findings may be helpful for more rational application of La-based compounds in the future.
Acknowledgements
This research was supported by the National Natural Science Foundations of China (21271059 and 20971034), the Research Fund for the Doctoral Program of Higher Education of China (20111301110004, 20131301120004), Hebei Province “Hundred Talents Program” (BR2-202), Hebei Province “Three Three Three Talents Program” (A201401002), Key Basic Research Special Foundation of Science Technology Ministry of Hebei Province (14961302D).
References
- L. Jarup, Nephrol., Dial., Transplant., 2002, 17, 35 CrossRef CAS PubMed
. - F. Jalilehvand, D. Spangberg, P. Lindqvist-Reis, K. Hermansson, I. Persson and M. Sandstrom, J. Am. Chem. Soc., 2001, 123, 431 CrossRef CAS PubMed
. - D. G. Guo, A. H. Wang, Y. Han and K. W. Xu, Acta Biomater., 2009, 5, 3512 CrossRef CAS PubMed
. - F. Feyerabend, C. Siemers, R. Willumeit and J. Rosler, J. Biomed. Mater. Res., Part A, 2009, 90, 931 CrossRef PubMed
. - X. Wang, L. Yuan, J. Huang, T. L. Zhang and K. Wang, J. Cell. Biochem., 2008, 105, 1307 CrossRef CAS PubMed
. - S. S. Chiang, J. B. Chen and W. C. Yang, Clin. Nephrol., 2005, 63, 461 CrossRef CAS
. - F. Albaaj and A. J. Hutchison, Int. J. Clin. Pract., 2005, 59, 1091 CrossRef CAS PubMed
. - H. H. Malluche, G. A. Siami, C. Swanepoel, G. H. Wang, H. Mawad, S. Confer, M. Smith, R. D. Pratt and M. C. Monier-Faugere, Clin. Nephrol., 2008, 70, 284 CAS
. - X. Wang, J. Huang, T. Zhang and K. Wang, Prog. Nat. Sci., 2009, 19, 331 CrossRef CAS PubMed
. - D. D. Liu, J. C. Zhang, G. F. Wang, X. L. Liu, S. X. Wang and M. S. Yang, Biol. Trace Elem. Res., 2012, 150, 433 CrossRef PubMed
. - J. C. Zhang, J. Sun, G. Q. Gu, X. H. Hao, D. D. Liu, Y. P. Li, X. Y. Qin and S. X. Wang, J. Rare Earths, 2012, 30, 90 CrossRef CAS
. - C. Yu, B. Chen, A. Javed, T. T. Zhao, K. Z. Yu and R. Wang, J. Transl. Med., 2013, 11, 308 CrossRef PubMed
. - C. Ergun, H. Liu and T. J. Webster, J. Biomed. Mater. Res., Part A, 2009, 89, 727 CrossRef PubMed
. - M. E. Nuttall and J. M. Gimble, Bone, 2000, 27, 177 CrossRef CAS
. - D. Benayahu, D. Zipori and S. Wientroub, Biochem. Biophys. Res. Commun., 1993, 197, 1245 CrossRef CAS PubMed
. - G. Ailhaud, P. Grimaldi and R. Negrel, Annu. Rev. Nutr., 1992, 12, 207 CrossRef CAS PubMed
. - K. Tamama, C. K. Sen and A. Wells, Stem Cells Dev., 2008, 17, 897 CrossRef CAS PubMed
. - D. D. Liu, C. Q. Yi, D. W. Zhang, J. C. Zhang and M. S. Yang, ACS Nano, 2010, 4, 2185 CrossRef CAS PubMed
. - D. D. Liu, C. Q. Yi, C. C. Fong, W. K. Yu and M. Y. Yang, Nanomedicine: NBM, 2014, 10, 1153 CrossRef CAS PubMed
. - D. D. Liu, J. C. Zhang, Q. Zhang, S. X. Wang and M. Y. Yang, J. Cell. Biochem., 2013, 114, 1105 CrossRef CAS PubMed
. - D. D. Liu, C. Q. Yi, C. C. Fong, J. C. Zhang and M. Y. Yang, ACS Nano, 2010, 4, 6439 CrossRef PubMed
. - D. D. Liu, K. Ge, Y. Jin, J. Sun, S. X. Wang and M. Y. Yang, J. Biol. Inorg. Chem., 2014, 19, 879 CrossRef CAS PubMed
. - Q. X. Mu, G. Q. Du, T. S. Chen, B. Zhang and B. Yan, ACS Nano, 2009, 3, 1139 CrossRef CAS PubMed
. - P. Bianco, M. Riminucci, S. Gronthos and P. G. Robey, Stem Cell., 2001, 19, 180 CrossRef CAS PubMed
. - S. Sanyal, P. Marckmann, S. Scherer and J. L. Abraham, Nephrol., Dial., Transplant., 2001, 26, 3616–3626 CrossRef PubMed
. - J. X. Li, J. C. Liu, K. Wang and X. G. Yang, J. Biol. Inorg. Chem., 2010, 15, 547 CrossRef CAS PubMed
. - G. Chen, C. Deng and Y. P. Li, Int. J. Biol. Sci., 2012, 8, 272 CrossRef CAS PubMed
. - W. Huang, S. Yang, J. Shao and Y. P. Li, Front. Biosci., 2007, 12, 3068 CrossRef CAS PubMed
. - X. Guo and X. F. Wang, Cell Res., 2009, 19, 71 CrossRef CAS PubMed
. - N. Espagnolle, F. Guilloton, F. Deschaseaux, M. Gadelorge, L. Sensébé and P. Bourin, J. Cell. Mol. Med., 2014, 18, 104 CrossRef CAS PubMed
. - D. T. Covas, R. A. Panepucci, A. M. Fontes, W. A. Silva, M. D. Orellana, M. C. C. Freitas, L. Neder, A. R. D. Santos, L. C. Peres, M. C. Jamur and M. A. Zago, Exp. Hematol., 2008, 36, 642 CrossRef CAS PubMed
. - K. C. Russell, D. G. Phinney, M. R. Lacey, B. L. Barrilleaux, K. E. Meyertholen and K. C. O'Connor, Stem Cell., 2010, 28, 788 CrossRef CAS PubMed
. - M. H. Lee, Y. J. Kim, H. J. Kim, H. D. Park, A. R. Kang, H. M. Kyung, J. H. Sung, J. M. Wozney, H. J. Kim and H. M. Ryoo, J. Biol. Chem., 2003, 278, 34387 CrossRef CAS PubMed
. - X. Fu, B. Han, S. Cai, Y. Lei, T. Sun and Z. Sheng, Wound Repair Regen., 2009, 17, 185 CrossRef PubMed
. - M. Rodrigues, L. G. Griffith and A. Wells, Stem Cell Res. Ther., 2010, 1, 32 CrossRef PubMed
. - S. Y. Byeong, P. Robert, A. O. Dmitri, Y. Isaac, M. Yuji, R. B. Richard and M. L. Karen, Development, 2006, 133, 4667 CrossRef PubMed
. - M. Snjezana, M. Sanja, K. Veronika, B. Nikolina, J. R. Jasminka, B. Fran, B. Drago, S. Petra, G. Lovorka, L. Boris and V. Slobodan, J. Histochem. Cytochem., 2004, 52, 1159 CrossRef PubMed
. - H. Sowa, H. Kaji, L. Canaff, G. N. Hendy, T. Tsukamoto, T. Yamaguchi, K. Miyazono, T. Sugimoto and K. Chihara, J. Biol. Chem., 2003, 278, 21058 CrossRef CAS PubMed
.
Footnote |
† Electronic supplementary information (ESI) available. See DOI: 10.1039/c5ra02311d |
|
This journal is © The Royal Society of Chemistry 2015 |