DOI:
10.1039/D4SC00482E
(Perspective)
Chem. Sci., 2024,
15, 9016-9025
The impact of earth-abundant metals as a replacement for Pd in cross coupling reactions†
Received
21st January 2024
, Accepted 12th April 2024
First published on 16th April 2024
Abstract
Substitution of one metal catalyst for another is not as straightforward as simply justifying this change based on the availability and/or cost of the metals. Methodologies to properly assess options for reaction design, including multiple factors like a metal's availability, cost, or environmental indicators have not advanced at the pace needed, leaving decisions to be made along these lines more challenging. Isolated indicators can lead to conclusions being made in too hasty a fashion. Therefore, an extensive life cycle-like assessment was performed documenting that the commonly held view that methods using earth-abundant metals (and in this case study, Ni) are inherently green replacements for methods using palladium in cross-coupling reactions, and Suzuki–Miyaura couplings, in particular, is an incomplete analysis of the entire picture. This notion can be misleading, and unfortunately derives mainly from the standpoint of price, and to some degree, relative natural abundance associated with the impact of mining of each metal. A more accurate picture emerges when several additional reaction parameters involved in the compared couplings are considered. The analysis points to the major impact that use of organic solvents has in these couplings, while the metals themselves actually play subordinate roles in terms of CO2-release into the environment and hence, the overall carbon footprint (i.e., climate change). The conclusion is that a far more detailed analysis is required than that typically being utilized.
Introduction
In reading any paper these days in most journals containing transition metal-mediated synthetic organic chemistry claiming to reduce our dependence on “precious metal catalysis”; … well, as a search using SciFinder indicates, this is really about palladium; not platinum; not gold; palladium. What actually drives such statements?1 The answer is often the same: given the occurrence and relative amounts of Pd involved, the chemistry community is too dependent upon this one precious metal for metal-catalyzed couplings due to its limited availability. Hence, one usually interprets this to mean, in either the short2 or long term,3 that Pd must be replaced with “earth-abundant” metals such as Ni, Cu, and Co. Additional justification for switching to a base metal then shifts to the one parameter with which virtually all users can identify: cost. What is cited as proof that switching to earth-abundant metal catalysis makes unequivocal economic sense? Answer: catalog pricing, showing how much less costly each of these EAMs is compared with palladium.4 And while there are many exciting scientific advances emerging from development of new methodologies employing EAMs, the focus on cost, natural abundance, and occasionally the pollution originating from mining and refining, rather than the overall science associated with each metal is so pervasive that it has led to a long and (seemingly) impressive list of “supporting evidence”, further advancing these concepts. Thus, in addition to the many individual papers continuously promoting the notion palladium catalyzed methods are inherently inferior,1 there are now thematic issues noting collaborations highlighting earth-abundant metal usage (complete with tabular surveys comparing costs).5 The “short cuts” taken over the years have led to conclusions that may well be misguided, as documented herein, surrounding use of EAMs. Part of this equation may well be a reflection of an overall inability to accurately measure additional metrics or indicators like the environmental impact of such widespread processes, potentially pointing to severe deficiencies in education and tools in these particular aspects of green and sustainable chemistry.
What drives the interest to abandon methods using palladium or other platinum group metals (PGMs)?6 Could it really be just on the basis of the up-front cost differentials between Pd salts and those of cheaper metals? Is the available evidence so overwhelming that the move away from palladium is truly compelling, whether focusing on cost, relative abundance, or environmental issues associated with accessing each metal (i.e., mining)? Direct 1
:
1 comparison of palladium and nickel along the lines shown in Fig. 1 would, in fact, lead to this conclusion. However, these numbers reflect a 1
:
1 relationship comparing 1 kg of palladium with 1 kg of nickel and their environmental footprint for the preparation of virgin metal including the mining footprint. Looking at the reports comparing Ni-catalysis and Pd-catalysis, the same catalyst loadings or the same amount of solvents are usually assumed, neglecting the fact that palladium loadings often are considerably lower, for example. Therefore, how much stock can be placed in these numbers? Does any process chemist making anything at scale use as much Pd as is typically needed with Ni? The answers to these questions are, or should be, obvious. In other words, is it possible that most of the discussion surrounding replacement of palladium in synthesis by EAMs for environmental reasons could actually be misleading, and maybe improperly stated in terms of the bigger picture? There are those who have already started to ask the same question.7 So, is earth-abundant metal chemistry the path forward to a greener future? Again, the numbers populating Fig. 1 would appear to say so; the footprint for Pd, according to indicators used from the European Footprint (EF) model,8 is orders of magnitude greater than for Ni. But wait; is this conclusion the same after examining the many other variables associated with using each metal?
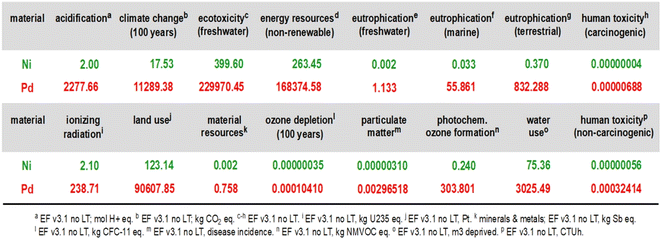 |
| Fig. 1 Direct, 1 : 1 comparison of environmental indicators for the production of 1 kg of virgin (i.e., freshly mined, from ore) Ni and Pd.9 | |
Firstly, what needs to be appreciated is that any discussion involving both PGMs and base metals (EAMs) have many variables that the chemistry community today is starting to evaluate to a greater extent. In the past and as mentioned before, due to the availability of limited data, consideration of variables such as price, natural abundance, and access unequivocally indicated that use of EAMs is highly preferred. An accurate, full picture did not emerge until recently when additional, important data can be taken into account. For example, use of lower loadings of Pd catalysts can reduce environmental differences between metals, and while such analyses often still favor base metals, different recovery techniques might help to reduce the gap even more. Secondly, as will be shown, use of different reaction media is actually the major determining factor.
To address conflicting arguments of availability, cost, environmental issues, endangered status, etc.; that is, to get at the reality of earth-abundant metal usage in organic synthesis, it seems timely to do an analysis that uses up-to-date information and indicators. Availability and cost, for example, are simple indicators and their usage is rather straightforward. Environmental metrics, however, are often limited to process mass intensity or other mass-based metrics. To better do justice to the environmental side of such an argument, life cycle inventory data should be included in a meaningful comparison. In other words, factors that are not normally considered in the analysis, including, e.g., solvent incineration as waste, for example. What about the actual amounts of metal needed, and the associated ligands? Or what about residual metal content in the products, especially regarding drugs where the regulatory authority (i.e., the FDA) imposes limits, typically requiring removal (taking valuable time and adding cost, not to mention generation of more waste) to meet their stringent standards?9 These, and several other factors (e.g., energy demands, as reaction temperatures are typically higher using Ni; functional group tolerance,10etc.) are rarely part of the calculus leading to a conclusion as to which type of catalysis should actually be pursued. The effects of these parameters on climate change and the environment loom large, and can no longer be ignored. Hence, these parameters can play important roles and suggest that, in their absence, an innate discrimination against Pd by the greater chemistry community may lead to an inaccurate conclusion.
Chemists have started to appreciate the value of utilizing, when possible, more extensive metrics associated with environmental issues. A life cycle assessment (LCA), while more time-consuming and costly, is now recognized as the premier approach to evaluating the “greenness” of any process.11 And while this may, today, be a reasonable approach mainly in industrial circles, nonetheless, an LCA is rapidly becoming the gold standard going forward. Nothing approaching this type of fact-finding mission yet exists for EAM usage, at least to our knowledge. Therefore, it is the intention of this perspective to provide more insight behind this alternative, Ni-based approach to group 10 metal-catalyzed cross coupling chemistry showcased using one example of each type of catalysis from literature cases. Isn't it time to find out whether using base metal catalysis as practiced today and encouraged for tomorrow makes sense under all circumstances, as one might believe reading todays literature, or do cases exist in which PGMs and their protocols factually offer more environmentally friendly alternatives? The conclusions that come forth from unbiased calculations is that the use of earth abundant (and also endangered)12 metals in place of existing cross coupling technologies that rely on (lower loadings of) palladium in alternative reaction media is NOT inevitably the best solution; not in terms of cost, nor in terms of the environment and overall impact on sustainability, and certainly not in terms of climate change.13 In other words, the use of Ni in organic solvents, as practiced today, might not be the best way forward and palladium can offer greener solutions. The important message of this perspective is to look at as much data as possible and not follow single metrics to make judgements as different scenarios can lead to different outcomes. And adopting this message, Ni-catalysis is not inherently more environmentally friendly than PGM-catalysis as one is often made to believe.
The data
To illustrate the point made earlier, we selected two literature reports focused on the most common, by far, group 10 metal-catalyzed reaction in the pharmaceutical industry: a Suzuki–Miyaura cross coupling, catalyzed by either Ni or Pd, both leading to the same product. Having the reports selected, we should also mention that while earth-abundant metal catalysis continues to be developed in this direction, with nickel leading the move away from use of palladium, other metals have made an appearance, such as cobalt14 and more recently, Fe.15 However, these reports are relatively few in number and do not in any way change the outcome of this study. The model reaction associated with the paper appearing in Organic Letters on this topic is representative of this goal (Scheme 1).16
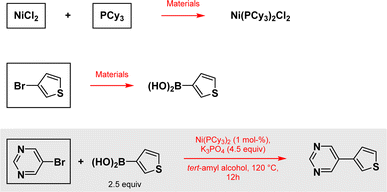 |
| Scheme 1 Reaction taken from the paper in Organic Letters and all other reactions considered for this example. Specific data according to ref. 17 are used for framed materials. Reagents, solvents, additives and all other materials used in the outlined reactions are also considered.16 | |
According to a recently published LCA-like method to determine the environmental footprint of reactions,17 we looked at the mass balance for preparation of the catalyst, including the preparation of the ligand, both coupling partners, including the preparation of the boronic acid from 3-bromothiophene, and the Suzuki–Miyaura reaction as reported, and normalized everything to the preparation of 1 kg of final product, 5-(thiophen-3-yl)pyrimidine, as functional unit, serving as reference basis for all calculations in this impact assessment (Scheme 1). Using this approach the consumption of energy is also included for the preparation of all material's while the energy consumption for the performed reactions themself are excluded. It is also worth noting, that the indicator for climate change (total) used in Fig. 3 contains the generated CO2-pollution for all material production back to a material's cradle, including the power consumption therein according to the cut-off model used in the ecoinvent database,18 as all other indicators do, and an estimated fixed value of CO2-pollution for a material's incineration. The other reaction, representative of an alternative technology relying on well-established ligated Pd catalysis derives from a study appearing in Science,19 where ppm levels of palladium catalysis are employed in the same coupling (Scheme 2). Here, the mass balance for the preparation of the surfactant, the preparation of the nanocatalyst and the needed SPhos ligand, the substrates as mentioned above, and the coupling reaction itself are all evaluated. Also as above, everything is normalized to the preparation of 1 kg of the coupling product.
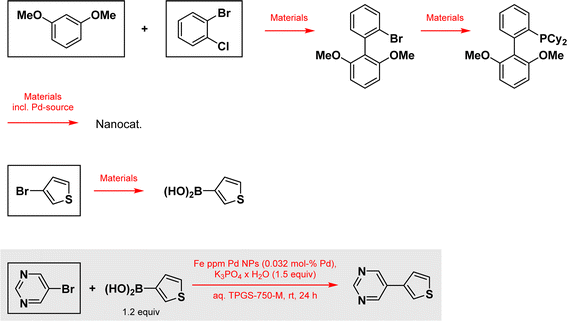 |
| Scheme 2 Suzuki–Miyaura coupling using ppm Pd taken from the paper in Science and all other reactions considered for this example. Specific data according to ref. 17 are used for framed materials. Reagents, solvents, additives and all other materials used in the outlined reactions are also considered.19 | |
These published literature (unoptimized) procedures indicate that in order to prepare 1 kg of heterobiaryl product using each process as described, 18.9 g of NiCl2 are needed at a cost of $17.40, while 0.50 g of (higher molecular weight) Pd(OAc)2 is needed costing $36.50. As will be shown, however, that while these numbers reflect what is surely anticipated (i.e., that using Ni is far less costly than is Pd), the more meaningful reaction parameter is that water (and not organic solvent) serves as the reaction medium.20 Couplings in water, where both the reaction partners and ligated catalysts are insoluble, is enabled by the (representative) designer surfactant TPGS-750-M present at the two weight percent level (i.e., 20 mg mL−1 of water).21 Nonetheless, as the data unequivocally confirm, factors such as use of a surfactant and many others, including the choice of metal, are of little-to-no consequence in terms of impact on cost, and notably, climate change and sustainability, and in general, the overall analysis (vide infra) looking at the selected examples.
Cost analyses
While nickel is much more “earth-abundant” relative to palladium,22 its price in any catalog and at any quantity should not even be a consideration in any analysis of its present or future prospects for replacing palladium as the premier PGM for catalysis in organic synthesis. The use of a Ni(II) salt (quantity-wise) represents an extremely small component of the overall picture, which includes not just the final Suzuki–Miyaura coupling but also the preparation of the nickel catalyst itself (Scheme 1 and Fig. 2), which is also the case for catalytic amounts of Pd(II)(OAc)2 (Scheme 2 and Fig. 2). While it is correct to state that the relative cost of Pd can be much greater than that of Ni,23 in terms of all the parameters involved in converting reaction partners to a targeted product over an entire sequence of several steps involved, both metals are insignificant. The metal costs remain neglectable in this case even when the amount of Pd catalyst is increased by a factor of ten; that is, to (unsustainable) loadings characteristic of many currently used couplings which, on occasion, may even be stoichiometric in palladium.24 Instead, what should be the focus of attention is the role that solvents play in determining categories of true consequence, such as toxicity, ionizing radiation, impact on ozone and water resources, and acidification, and most notably, their impact on climate change. That is, the total environmental footprint of both processes, rather than the footprint of metal usage alone, is significant by comparison (vide infra; Fig. 3).
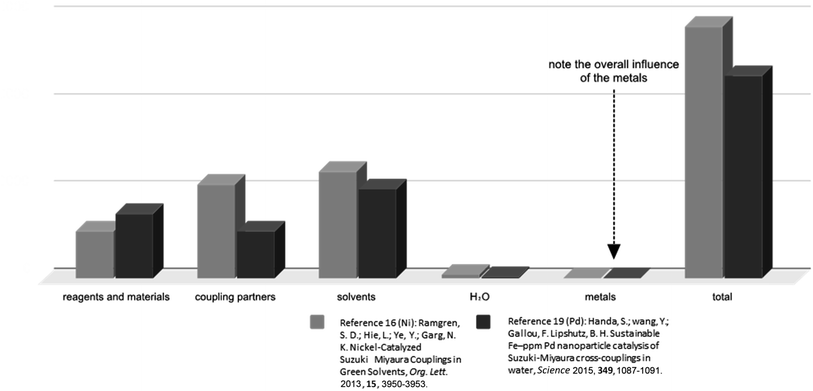 |
| Fig. 2 Relative costs associated with the numerous parameters involved for Suzuki–Miyaura couplings. As for the entire assessment, this cost calculation also includes the preparation of all materials needed for the Suzuki reactions presented in Schemes 1 and 2. This includes the costs to prepare the coupling reagents, the catalysts, the surfactant, and all other materials needed in these reactions. | |
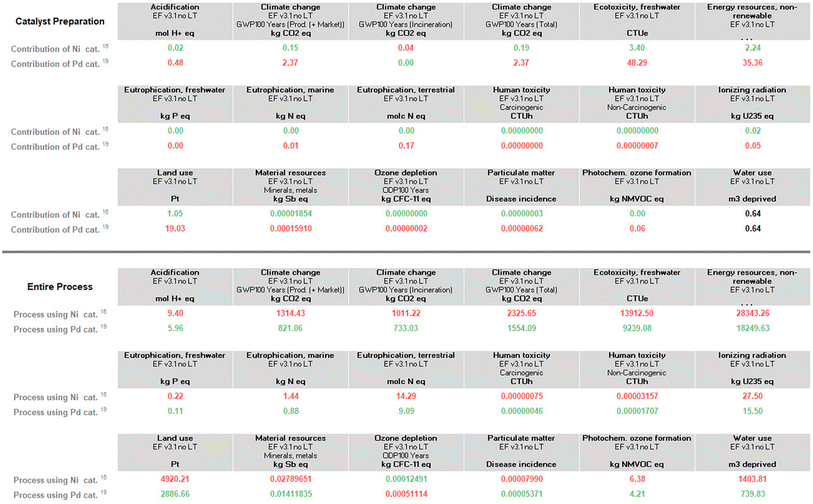 |
| Fig. 3 Environmental footprint for just metal usage in the entire process (top), and for the entire processes itself according to Schemes 1 and 2 (bottom). All indicators other than climate change represent the environmental burdens of material production (and market distribution). Climate change (total) contains an additional indicator for waste treatment (incineration). | |
The numbers in Fig. 3 (which have varying units and hence, cannot be directly compared, column-to-column) do point out that for the processes involved, all reactions presented in Schemes 1 and 2, where post-reaction handling and waste treatment for the final Suzuki–Miyaura coupling are (assumed) to be the same (extraction, purification via column chromatography, and incineration), palladium has, in fact, a slightly higher footprint than does nickel, if treated individually (top half). Looking at the Suzuki–Miyaura couplings as a whole and all needed steps upfront according to Schemes 1 and 2, however, where organic solvent usage is higher, as is substrate usage in Ni catalysis, the micellar catalysis approach in water is roughly 45–50% “greener” in terms of the total carbon footprint (bottom half; see “climate change (total)”, in particular).
These numbers get even more lopsided against use of EAM catalysis when the details associated with using water as the gross reaction medium in the Suzuki–Miyaura reaction are considered. For group 10 metal-catalyzed couplings leading to products that are usually solids (as routinely observed in the pharmaceutical industry). These, as noted previously,25 participate in molecule-by-molecule exchange between micellar arrays. This occurs through the water, where, being water-insoluble, they precipitate. In other words, a “recrystallization” takes place automatically, in which case the “workup” oftentimes involves nothing more than a simple filtration. While this difference in purification is obvious (i.e., no extraction and/or chromatography is needed), just what such a cost savings might be will vary and is not included in this analysis. Nonetheless, this will add further to the baseline differential in terms of total carbon footprint, already at ca. 45–50%.
The energy invested per reaction is yet another reaction variable that has not been included in the tabular survey illustrated in Fig. 1–3. In the given example relying on aqueous micellar catalysis (Scheme 2) the implication is that couplings are taking place at much higher concentrations that exist within the inner micellar cores,26 whereas traditional bond formations in organic solvents are impractical under these conditions (i.e., reactions cannot be stirred). This leads to milder reactions conditions, and aside from the obvious lower reaction temperatures and hence, less energy being invested, another important outgrowth is the impact of temperature on the resulting impurity profile. Cleaner reactions characteristic of chemistry in water translates as well into a time savings associated with product isolation/purification, which can also lead to benefits on total solvent use and overall cost. Thus, the savings in time, energy investment, and use of resources for product cleanup are also not quantified herein, and yet, clearly add to the case disfavoring use of EAMs in organic solvents.
And what about residual metals in the product of a Ni- or Pd-catalyzed coupling? All regulatory authorities make it very clear that only 20 and 10 ppm per day per dose is allowable for Ni and Pd, respectively.27 Using 1–5 mol percent nickel virtually guarantees that removal of residual nickel will be needed should a cross coupling en route to an API be used at a later stage of its synthesis. On the other hand, with lower Pd loadings associated with couplings run in water, levels of Pd found in an API are independent of when in the sequence the coupling is performed, since the products having residual levels of Pd above the FDA allowance are very rare.28 This consideration undoubtedly adds even more cost to the bottom line when using an EAM as catalyst.
How are safety issues to be quantified? Flammable and toxic organic solvents are routinely stored in large holding tanks on a manufacturing site. This raises questions as to, e.g., government-imposed limits placed on infrastructure, which might then dictate exactly where, how, and to what extent a target compound (i.e., quantity) can be made at a given location. Efficiency, and thus, economics are likely to be impacted if such restrictions exist (i.e., the economy of scale may be limited).
Then there is the question regarding waste generation and disposal. The rules that exist in any location throughout the world vary, and could certainly place additional limitations on production. Using aqueous media may ease many of these concerns, but each situation is likely to be unique. Nonetheless, what is clear from this analysis is that chemistry in water vs. use of petroleum-based organic solvents (or solvents obtained from any source, including biorenewables) is the key factor that determines the impact of the catalysis on climate change, and to a much lower extent the choice of metal, earth-abundant or otherwise. Water-based chemistry offers, in contrast, a high potential in this regard as already demonstrated at Novartis.29 Hence, the baseline differential of 45–50% is likely to be even greater when, on a case-by-case basis, all of these additional factors are considered. Indeed, a more likely number favoring use of Pd (or any metal) in water is far north of 50%. Having said this, we would like to acknowledge that the effect onto the indicator for climate change thorough the contribution of PGMs is often not representative for their actual effect on a process' pollution as resource depletion and other factors are excluded. However, we believe that the carbon footprint can be used to guide chemists.
Climate change and sustainability
A closer look at the data in Fig. 3 (both top and bottom) reveals that the total impact on climate change associated with the two processes is far from equal. The value of consequence for the Ni-catalyzed reaction stands at ca. 2326 kg CO2 per kg final product, while that for the Pd-catalyzed coupling is roughly 1554 kg CO2 per kg product. By contrast, the corresponding data associated with the metal-only footprint (Fig. 3, top) is 0.19 kg CO2 per kg product for Ni versus 2.4 for Pd. These values, again, illustrate the relatively small, almost trivial overall impact associated with the choice of metal selected for these Suzuki–Miyaura reactions. Looking at the other environmental indicators outlined, each with its unique physical unit, it becomes clear that the different categories cannot be compared with each other. To understand each category's relative impact and to get a single score for the process' overall “greenness”, the results in each category can be normalized and then weighted to afford unitless values generating a single numerical score (Fig. 4). We would like to note that reducing any assessment to a single score removes an important level of detail. The relative importance of each indicator can be seen as a function of priorities of a person assessing the process. Individual indicators allow for geographic or personal priorities to be considered. A single score also has its benefits. For example, it can be used in communications with stakeholders having different scientific backgrounds, or to compare impacts of multiple processes in a larger portfolio.
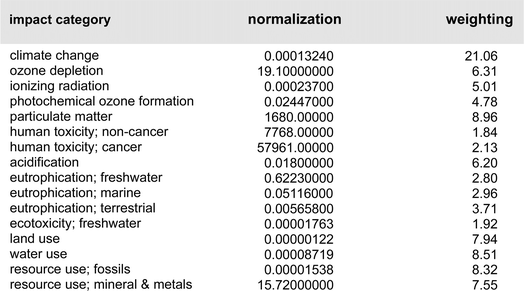 |
| Fig. 4 Normalization30 and weighting31 for the factors impacted by a metal-catalyzed coupling. | |
Applying normalization30 and weighting31 factors as outlined in Fig. 4, to the initial analysis that led to the data provided in Fig. 3, now leads to comparison values for the two methodologies (i.e., using Ni catalysis, as in the Organic Letters paper,16 and the lower loadings associated with the Pd-catalyzed technology in water, as described in the Science paper),19 shown in Fig. 5. The impact associated with each factor leads to the stand-alone conclusion for the reaction sequences investigated: climate change is the most important outcome, and substantially so. It is followed by resource use, fossils, then metals and minerals, and then by particulate matter formation. The sum total of all factors points to the net differential between the two synthetic approaches: 19.48 Eco-Points for Ni catalysis in organic solvents, and the preparation of the materials leading up to the Ni-catalyzed Suzuki reaction (Scheme 1), vs. 12.09 using Pd catalysis in water (Scheme 2),32 or a >60% change against Ni. Moreover, as noted above, these numbers represent a minimum differential, since several parameters are not easily included.
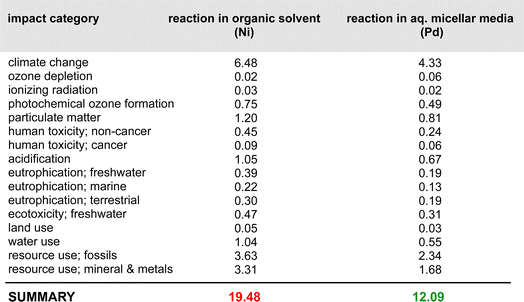 |
| Fig. 5 Relative weighting of factors involved in both Ni- and Pd-catalyzed Suzuki–Miyaura couplings and the steps leading up to them according to Schemes 1 and 2. | |
Conclusions
This discussion hopefully convinces the reader that the overall picture being put forth encouraging a switch to earth-abundant metals, and nickel, in particular, as a replacement for palladium in cross-couplings, and perhaps other types of processes, is not necessarily valid from an environmental perspective (as summarized in the spider diagram; Fig. 6, which is representative of a relative comparison). Using a straightforward LCA-like assessment17 that includes the many reaction variables and environmental issues involved clearly indicate that cost considerations, natural abundance, and/or the argument focused on the environmental impact of metal mining used so frequently in many literature reports have to be put into perspective; that is, the points made in all of these papers and symposia are oftentimes not the key determining factors. Rather, it may be ideal going forward to further develop EAM catalysis using water as the reaction medium.33 It should also be appreciated that not all Ni-based catalysis is aimed at this one goal; rather, there is a growing number of outstanding EAM-catalyzed technologies being developed for other purposes (e.g., photocatalysis, Csp3–Csp3 couplings, asymmetric synthesis, biocatalysis, etc.) many of which are not amenable to palladium catalysis.34 Hence, one overall conclusion might be that Ni or other non-noble catalysis (such as that based on Cu, or Co, etc.) for cross-coupling chemistry is better regarded as complementary to, and not a replacement for, Pd.35 Clearly, a more careful and extensive rather than superficial analysis including data-based environmental indicators along with additional factors such as the sustainable development goals (SDGs),36 as well as health and safety concerns, resource scarcity, and energy consumption must be taken into account prior to drawing conclusions. This recently developed tool for such an analysis at Novartis17 has helped in providing additional insight regarding various processes, and enabling opportunities for developing chemistry guided towards more impactful directions based on the use of better and broader environmental metrics being applied to this and other important topics. It is hoped, therefore, that this contribution will trigger the inclusion of such assessment in future efforts by the chemistry community reflecting greater accuracy and practicality. Indeed, looking to the future, where climate change is especially in focus, we may have no choice!
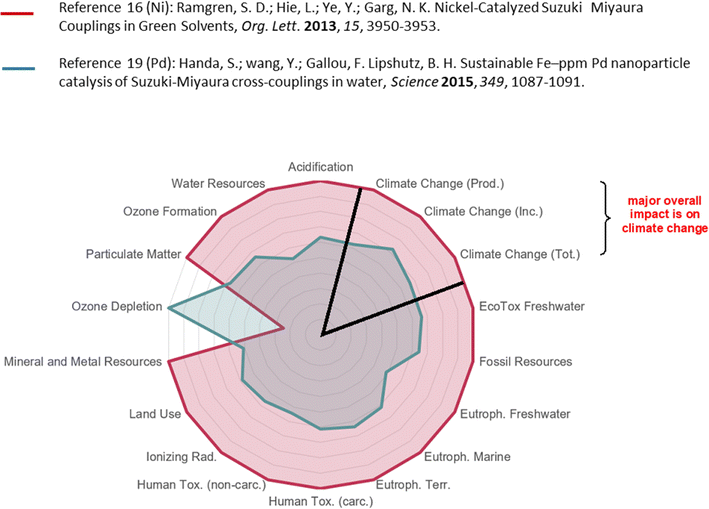 |
| Fig. 6 Pictorial summary of the overall environmental impact of the processes in Schemes 1 and 2 using Ni (in red) vs. Pd (in green). | |
Bottom line
The data obtained via this LCA-like analyses unequivocally document that by focusing on replacing palladium with earth-abundant metal (EAM) alternatives based solely on catalog pricing and availability is only a partial evaluation that can be of little consequence, especially from the overall standpoint of sustainability. That is, catalysis in organic solvents leads to a significant negative impact on climate change relative to any metal catalyst, precious or earth-abundant. Their use in water, however, translates into less environmentally costly processes.
Data availability
The data supporting this article have been uploaded as part of the ESI.†
Author contributions
All authors contributed in the design of the paper, and to the writing. The methodology was designed by MUL and FG, and calculations were done by MUL.
Conflicts of interest
There are no conflicts to declare.
Acknowledgements
Support for this contribution (to BHL) from the NSF in the form of CHE 21-52566 is warmly acknowledged. FG and MUL are grateful to Dr Andreas Knell and Dr Thomas Heinz for their trust and vision.
References
- See, as representative examples,
(a) (Review) B. A. Baviskar, P. V. Ajmire, D. S. Chumbhale, M. S. Khan, V. G. Kuchake, M. Singupuram and P. R. Laddha, Recent advances in nickel catalyzed Suzuki-Miyaura cross coupling reaction via C-O & C-N bond activation, Sustainable Chem. Pharm., 2023, 32, 100953 CrossRef CAS;
(b) (Using 1 mol% Ni) X. Guo, H. Dang, S. R. Wisniewski and E. M. Simmons, Nickel-Catalyzed Suzuki−Miyaura Cross-Coupling Facilitated by a Weak Amine Base with Water as a Cosolvent, Organometallics, 2022, 41, 1269–1274 CrossRef CAS;
(c) (Using 4.5 mol% Ni) M. J. Goldfogel, X. Guo, J. L. Melendez Matos, J. A. Gurak, M. V. Joannou, W. B. Moffat, E. M. Simmons and S. R. Wisniewski, Advancing Base-Metal Catalysis: Development of a Screening Method for Nickel-Catalyzed Suzuki−Miyaura Reactions of Pharmaceutically Relevant Heterocycles, Org. Process Res. Dev., 2022, 26, 785–794 CrossRef CAS;
(d) (Using 3 mol% Ni) M. M. Rahman, Q. Zhao, G. Meng and R. S. M. Szostak, [Ni(Np#)(η5-Cp)Cl]: Flexible, Sterically Bulky, Well-Defined, Highly Reactive Complex for Nickel-Catalyzed Cross-Coupling, Organometallics, 2022, 41, 2597–2604 CrossRef CAS PubMed;
(e) (Using 10 mol% Ni) L. Guo, W. Srimontree, C. Zhu, B. Maity, X. Liu, L. Cavallo and M. Rueping, Nickel-catalyzed Suzuki-Miyaura cross-couplings of aldehydes, Nat. Commun., 2019, 10, 1957 CrossRef PubMed.
-
(a) E. R. Schofield, On the Criticality of Palladium in Organic Synthesis: A Perspective, Johnson Matthey Technol. Rev., 2023, 67, 285–289 CrossRef CAS;
(b) See also: E. R. Schofield, The Continuing Importance of Platinum Group Metals, Johnson Matthey Technol. Rev., 2023, 67, 247–248 CrossRef.
- B. H. Lipshutz, On the Sustainability of Palladium in Organic Synthesis: A Perspective, Johnson Matthey Technol. Rev., 2023, 67, 278–284 CrossRef CAS.
- For example, consider PdCl2: https://www.google.com/search?client=safari&sca_esv=572890011&rls=en&q=palladium%20chloride%20price&ved=2ahUKEwjRp6T3_fCBAxVKHkQIHRX4CXYQmoICKAB6BAgIEA4&biw=2049&bih=1316&dpr=2, while for NiCl2: https://www.google.com/search?client=safari&rls=en&q=nickel+chloride+price&ie=UTF-8&oe=UTF-8.
-
(a) P. J. Chirik, K. M. Engle, E. M. Simmons and S. R. Wisniewski, Collaboration as a Key to Advance Capabilities for Earth-Abundant Metal Catalysis, Org. Process Res. Dev., 2023, 27, 1160–1184 CrossRef CAS;
(b) K. M. P. Wheelhouse, R. L. Webster and G. L. Beutner, Advances and Applications in Catalysis with Earth-Abundant Metals, Org. Process Res. Dev., 2023, 27, 1157–1159 CrossRef CAS.
- See: https://www.nsf.gov/pubs/2023/nsf23157/nsf23157.jsp, November 24, 2023, see also: January 15, 2024.
- A. A. Komarova and D. S. Perekalin, Noble Metal versus Abundant Metal Catalysts in Fine Organic Synthesis: Cost Comparison of C-H Activation Methods, Organometallics, 2023, 42, 1433–1438 CrossRef CAS.
- See: https://eplca.jrc.ec.europa.eu/EnvironmentalFootprint.html, accessed Jan, 15, 2024.
-
FDA, Q3D (R1) Elemental Impurities Guidance for Industry, 2022, 403, https://www.fda.gov/media/148474/download, accessed on Jan, 15, 2024 Search PubMed.
- A. K. Cooper, P. M. Burton and D. J. Nelson, Nickel versus Palladium in Cross-Coupling Catalysis: On the Role of Substrate Coordination to Zerovalent Metal Complexes, Synthesis, 2020, 52, 565–573 CrossRef CAS.
- For a discussion, see: https://learning.acsgcipr.org/life-cycle-impacts-and-environmental-fate-of-pharmaceuticals/examining-the-life-cycle/, November 24, 2023, see also the many contributions of Jessop; see: accessed January 18, 2024.
- For the ACS GCI Table of Endangered Metals, see https://www.acs.org/greenchemistry/research-innovation/endangered-elements.html.
- This is the case independent of what has been advanced by the GCI; see: https://www.acs.org/acs-webinars/library/abundant-metal-catalysis.html, November 24, 2023.
-
(a) R. R. Mills, D. Gygi, E. M. Simmons, S. R. Wisniewski, J. Kim and P. J. Chirik, Mechanistic Investigations of Phenoxyimine−Cobalt(II)-Catalyzed
C(sp2)−C(sp3) Suzuki−Miyaura Cross-Coupling, J. Am. Chem. Soc., 2023, 145, 17029–17041 CrossRef PubMed;
(b) S. B. Tailor, M. Manzotti, G. J. Smith, S. A. Davis and R. B. Bedford, Cobalt-Catalyzed Coupling of Aryl Chlorides with Aryl Boron Esters Activated by Alkoxides, ACS Catal., 2021, 11, 3856–3866 CrossRef CAS;
(c) J. M. Neely, M. J. Bezdek and P. J. Chirik, Insight into Transmetalation Enables Cobalt-Catalyzed Suzuki−Miyaura Cross Coupling, ACS Cent. Sci., 2016, 2, 935–942 CrossRef CAS.
- P. O. Peterson, M. V. Joannou, E. M. Simmons, S. R. Wisniewski, J. Kim and P. J. Chirik, Iron-Catalyzed C(sp2)−C(sp3) Suzuki−Miyaura Cross-Coupling Using an Alkoxide Base, ACS Catal., 2023, 13, 2443–2448 CrossRef CAS.
- S. D. Ramgren, L. Hie, Y. Ye and N. K. Garg, Nickel-Catalyzed Suzuki Miyaura Couplings in Green Solvents, Org. Lett., 2013, 15, 3950–3953 CrossRef CAS PubMed.
- M. U. Luescher and F. Gallou, Interactions of multiple metrics and environmental indicators to assess processes, detect environmental hotspots, and guide future development, Green Chem., 2024 10.1039/D4GC00302K.
- G. Wernet, C. Bauer, B. Steubing, J. Reinhard, E. Moreno-Ruiz and B. Weidema, Int. J. Life Cycle Assess., 2016, 21, 1218–1230 CrossRef.
- S. Handa, Y. Wang, F. Gallou and B. H. Lipshutz, Sustainable Fe–ppm Pd nanoparticle catalysis of Suzuki-Miyaura cross-couplings in water, Science, 2015, 349, 1087–1091 CrossRef CAS PubMed.
- For representative reviews, see:
(a) T. N. Ansari, F. Gallou and S. Handa, Palladium-catalyzed micellar cross-couplings: an outlook, Coord. Chem. Rev., 2023, 488, 215158 CrossRef CAS;
(b) F. Fabris, M. Illner, J.-U. Repke, A. Scarso and M. Schwarze, Is Micellar Catalysis Green Chemistry?, Molecules, 2023, 28, 4809 CrossRef CAS;
(c) E. Borrego, A. Caballero and P. J. Perez, Micellar Catalysis as a Tool for C−H Bond Functionalization toward C−C Bond Formation, Organometallics, 2022, 41, 3084–3098 CrossRef CAS;
(d) T. Lorenzetto, D. Frigatti, F. Fabris and A. Scarso, Nanoconfinement Effects of Micellar Media in Asymmetric Catalysis, Adv. Synth. Catal., 2022, 364, 1776–1797 CrossRef CAS.
- B. H. Lipshutz, S. Ghorai, A. R. Abela, R. Moser, T. Nishikata, C. Duplais, A. Krasovskiy, R. D. Gaston and R. C. Gadwood, TPGS-750-M: A Second-Generation Amphiphile for Metal-Catalyzed Cross-Couplings in Water at Room Temperature, J. Org. Chem., 2011, 76, 4379–4391 CrossRef CAS PubMed.
- See: https://www.angelo.edu/faculty/kboudrea/periodic/physical_abundances.htm, accessed November 24, 2023.
- See: https://www.google.com/search?client=safari&rls=en&q=price+of+palladium+versus+nickel&ie=UTF-8&oe=UTF-8, accessed November 24, 2023.
- M. R. Uehling, R. P. King, S. W. Krska, T. Cernak and S. L. Buchwald, Pharmaceutical diversification via palladium oxidative addition complexes, Science, 2019, 363, 405–408 CrossRef CAS.
- J. Epstein, J. J. Kaminski, N. Bodor, R. Enever and J. Sowa, Micellar acceleration of organophosphate hydrolysis by hydroximinomethylpyridinium type surfactants, J. Org. Chem., 1978, 43, 2816–2821 CrossRef CAS.
- For recent representative comparisons of conditions needed in several processes in organic solvents vs. those using aqueous micellar conditions, see:
(a) J. C. Caravez, M. J. Wong, R. D. Kavthe, B. S. Takale and B. H. Lipshutz, Pd-Catalyzed Carbonylations of Aryl/Heteroaryl Halides in Aqueous Micellar Media, ACS Catal., 2023, 13, 12383–12390 CrossRef CAS;
(b) K. S. Iyer, C. Nelson and B. H. Lipshutz, Facile, green, and functional group-tolerant reductions of carboxylic acids…in, or with, water, Green Chem., 2023, 25, 2663–2671 RSC;
(c) Y. Zhang, B. S. Takale, F. Gallou, J. Reilly and B. H. Lipshutz, Sustainable ppm level palladium-catalyzed aminations in nanoreactors under mild, aqueous conditions, Chem. Sci., 2019, 10, 10556–10561 RSC.
-
FDA, Q3D(R1) Elemental Impurities Guidance for Industry, see: https://www.fda.gov/media/135956/download, accessed November 24, 2023 Search PubMed.
- For a rare case that exceeds the FDA allowed level (13.5 ppm residual Pd), see J. Yu, K. S. Iyer and B. H. Lipshutz, An environmentally responsible synthesis of the antitumor agent lapatinib (Tykerb), Green Chem., 2022, 24, 3640–3643 RSC.
- C. Krell, M. Parmentier, L. Padeste, M. Buser, J. Hellsttern, S. Glowienke, R. Schreiber, R. Wickendick, L. Hueber, R. Haenggi, L. Sciascera, X. Zheng, A. Clarke and F. Gallou, Water waste from surfactant chemistry: risk or opportunity, Org. Proc. Res. Dev., 2021, 25, 900 CrossRef CAS; F. Gallou, N. A. Isley, A. Ganic, U. Onken and M. Parmentier, Surfactant technology applied toward an active pharmaceutical ingredient: more than a simple green chemistry advance, Green Chem., 2016, 18, 14–19 RSC.
-
European Commission, Joint Research Centre, R. Pant, S. Sala, E. Crennaet al., Global Normalisation Factors for the Environmental Footprint and Life Cycle Assessment, Publications Office, 2017, https://data.europa.eu/doi/10.2760/88930, accessed Jan, 10, 2024 Search PubMed.
-
European Commission, Joint Research Centre, A. Cerutti, R. Pant and S. Sala, Development of a Weighting Approach for the Environmental Footprint, Publications Office, 2018, https://data.europa.eu/doi/10.2760/945290, accessed: Jan, 10, 2024 Search PubMed.
-
N. Jungbluth, Description of Life Cycle Impact Assessment Methods, ESU-services Ltd., Schaffhausen, Switzerland, 2022, https://esu-services.ch/fileadmin/download/tender/ESU-Description-of-LCIAmethods.pdf, accessed Jan, 10, 2024 Search PubMed.
-
(a) S. Handa, E. D. Slack and B. H. Lipshutz, Nanonickel-Catalyzed Suzuki–Miyaura Cross-Couplings in Water, Angew. Chem., Int. Ed., 2015, 54, 11994–11998 CrossRef CAS;
(b) S. Handa, J. D. Smith, M. S. Hageman, M. Gonzalez and B. H. Lipshutz, Synergistic and Selective Copper/ppm Pd-Catalyzed Suzuki–Miyaura Couplings: In Water, Mild Conditions, with Recycling, ACS Catal., 2016, 6, 8179–8183 CrossRef CAS.
- See, as recent examples:
(a) M. C. Haibach, S. Shekhar, T. S. Ahmed and A. R. Ickes, Recent Advances in Nonprecious Metal Catalysis, Org. Process Res. Dev., 2023, 27, 423–447 CrossRef CAS;
(b) R. M. Bullock, J. G. Chen, L. Gagliardi, P. J. Chirik, O. K. Farha, C. H. Hendon, C. W. Jones, J. A. Keith, J. Klosin, S. D. Minteer, R. H. Morris, A. T. Radosevich, T. B. Rauchfuss, N. A. Strotman, A. Vojvodic, T. R. Ward, J. Y. Yang and Y. Surendranath, Using nature's blueprint to expand catalysis with Earth-abundant metals, Science, 2020, 369, 786 CrossRef.
- V. M. Chernyshev and V. P. Ananikov, Nickel and Palladium Catalysis: Stronger Demand than Ever, ACS Catal., 2022, 12, 1180–1200 CrossRef CAS.
- Sustainable Development Goals, see: https://www.undp.org/sustainable-development-goals, accessed January 19, 2024.
|
This journal is © The Royal Society of Chemistry 2024 |