DOI:
10.1039/D4QI01773K
(Review Article)
Inorg. Chem. Front., 2024,
11, 6694-6710
Tuning photoluminescence properties of Au clusters by surface modification and doping: lessons from case studies of icosahedral Au13
Received
15th July 2024
, Accepted 9th September 2024
First published on 9th September 2024
Abstract
The photoluminescence (PL) properties of monolayer-protected gold nanoclusters (Au MPCs) have been extensively studied due to their wide range of potential applications, such as photocatalysis, photosensitization, bioimaging, and tumor therapy. The key factor that determines the PL properties of Au MPCs is the Au core size, but other parameters such as surface modifications and doping also significantly affect the PL properties. To understand and highlight the importance of these secondary parameters on the PL properties, this review article focuses on the ubiquitous icosahedral Au13 core as a benchmark and addresses the question of how the PL properties are affected by surface modification with X-type (thiolates, alkynyls) and L-type (phosphines, N-heterocyclic carbenes) ligands and doping with heterometals (Ru, Rh, Ir, Ni, Pd, Pt, Ag, Cd). The PL emission energy of Au13 MPC can be varied in the wide range of 1.1–2.1 eV and PL quantum yields up to 70% can be achieved by appropriate surface modification and doping. Based on the publications available until June 2024, the following empirical rules are derived to tune the PL emission energy and increase the PL quantum yield: (1) The PL emission energy and PL quantum yield can be increased by using L-type ligands and/or doping with small group elements such as Ir, Pt, Ru, and Rh. (2) The PL quantum yield can be enhanced by maximizing the interaction between the ligands through π⋯π or C–H⋯π interaction, chemical bonding, and steric packing. The first rule follows the energy gap law and can be understood qualitatively by simple schemes based on the jellium model. The last rule is due to the suppression of non-radiative decay by the stiffening of the Au core. We hope that this review will help the audience to establish rational guidelines for designed PL properties.
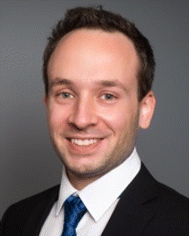 Dennis Alexander Buschmann | Dr. Dennis Alexander Buschmann studied nano-science at the Eberhard Karls Universität Tübingen, where he obtained his M.Sc. degree in 2017. He received his Dr. rer. nat. degree for research on organo-rare-earth-metal clusters in inorganic chemistry in 2022 under the supervision of Prof. Reiner Anwander. After that, he joined the research group of Prof. Tatsuya Tsukuda as a postdoctoral researcher at The University of Tokyo. His current research interests include the synthesis and photoluminescence properties of NHC-protected gold clusters. |
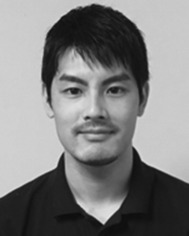 Haru Hirai | Dr. Haru Hirai received a Ph.D. degree in 2023 from The University of Tokyo under the supervision of Prof. Tatsuya Tsukuda. He is currently a postdoctoral researcher at the Department of Chemistry, The University of Tokyo. His research interests are mainly the atomically-precise synthesis and functionalization of ligand-protected gold-based clusters. |
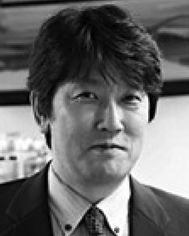 Tatsuya Tsukuda | Prof. Tatsuya Tsukuda received his Ph.D. degree in 1994 from The University of Tokyo under the supervision of Prof. Tamotsu Kondow. After postdoctoral research at RIKEN, he was appointed Assistant Professor at The University of Tokyo in 1994 and he became an Associate Professor at the Institute for Molecular Science in 2000. In 2007, he was promoted to Professor at the Catalysis Research Center of Hokkaido University, and in 2011 he moved to The University of Tokyo. His research interests cover the atomically precise synthesis and characterization of stabilized gold clusters and their catalytic applications. |
1. Introduction
For decades, monolayer-protected gold clusters (Au MPCs) have attracted materials science researchers for two main reasons: (1) they exhibit new physicochemical properties not found in the corresponding bulk or nanoparticles (NPs); (2) their properties can be tuned over a wide range by changing the number of constituent atoms.1–10 The origins for size-specific photophysical11–22 and catalytic23–29 properties are the unique atomic packing and quantized electronic structures of the Au cluster cores.
Photoluminescence (PL) is one of the size-specific properties of Au MPCs arising from direct consequences of quantized electronic structures.30 The Au NPs and bulk Au do not exhibit intense PL because non-radiative quenching via strong electron–phonon and electron–electron coupling dominates the relaxation processes of the photoexcited state: the PL quantum yield (QY) for bulk Au is reported to be 10−10%.31 In contrast, the energy gap between the quantized orbitals of Au MPCs exceeds the thermal energy so that they behave like conventional molecules and start to show superior PL properties.11–22 In this regard, Au MPCs are promising materials with superior and tailored PL properties because their electronic structures can be tuned not only by size, but also by shape and composition of the Au core and the nature of the protecting ligands. Their high chemical stability and relatively low cytotoxicity make Au MPCs an attractive tool for medicinal chemistry applications.32–37 PL emission can be tuned over a wide range, even into the near-infrared (NIR) region, which is important for deep tissue penetration. As a result, Au MPCs are frequently applied as bioimaging agents.38–41 Recently, Au MPCs have also been used in tumor therapy.42–44 Au MPCs are actively targeted to cancer cells by surface modification with tumor targeting agents, which are then used to destroy tumor cells via photothermal therapy and reactive oxygen species generated.
In addition, Au MPCs provide us with an ideal opportunity to develop rational design principles of the PL properties for the following reasons: (1) there is an increasing number of Au MPCs whose structures have been determined by single-crystal X-ray diffraction (SCXRD) analysis. Such structural information allows us to determine how the PL properties are affected by each structural parameter, (2) the electronic structure of Au MPCs is much simpler than that of other transition metals. The valence electrons in Au MPCs are accommodated in discrete orbitals labelled 1S, 1P, 1D, 2S, 2P, … distributed over a jellium-like potential (Fig. 1).45–53 The chemical formula [AuxMy]z (M: ligand) allows us to calculate the formal number of valence electrons (n*) according to the following equation proposed by Häkkinen:46
where
A and
B represent the number of valence electrons supplied by a single metal atom and the ligand M, respectively. Specifically,
A is 1 for Au, but we should apply suitable
A values, depending on the group in the periodic table as shown in
Table 1 when other elements are doped onto Au MPCs. Meanwhile,
B is 0 for L-type ligands, such as mono-/diphosphines (PR
3/d-PR
2) and mono-/bis-N-heterocyclic carbenes (NHC/bis-NHC), while it is −1 for X-type ligands, such as halide (X), thiolate (SR), and alkynyl (C
![[triple bond, length as m-dash]](https://www.rsc.org/images/entities/char_e002.gif)
CR) due to their electron-withdrawing nature
46 as shown in
Table 1.
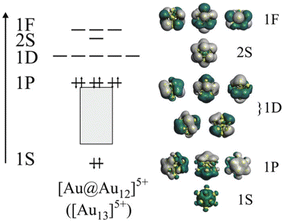 |
| Fig. 1 Schematic representation of the electronic structure of Au13 MPC including the relevant superatomic orbitals. Reprinted with permission from ref. 37. Copyright 2019 Royal Society of Chemistry. | |
Table 1 Formal charge state and preferential location of dopant
Group (element) |
Formal charge (A in eqn (1)) |
Doping location |
Group 8 (Ru) |
−2 |
Center |
Group 9 (Rh, Ir) |
–1 |
Center |
Group 10 (Ni, Pd, Pt) |
0 |
Center |
Group 11 (Cu, Ag) |
+1 |
Center/surface |
Group 12 (Cd, Hg) |
+2 |
Surface |
The “Au core size” is the primary factor that determines various physicochemical properties of Au MPCs. However, the properties of Au MPCs can also be affected by other parameters such as surface modifications and doping. To understand and highlight the importance of these secondary parameters on the PL properties, this review article focuses on the ubiquitous icosahedral Au13 core as a benchmark and addresses the question of how the PL properties are affected by surface modification with ligands (SR,54–58 C
CR,59,60 halogens (X), PR3/d-PR2
61,62 or NHC/bis-NHC63,64) and doping of heterometals (Ru, Rh, Ir, Ni, Pd, Pt, Cu, Ag, Cd, Hg).65–71Fig. 2 shows the geometric structures of the typical examples of Au13 MPCs determined by SCXRD: [Au25(SC2H4Ph)18]− (Fig. 2a),72,73 [Au25(C
CPh(CF3)2)18]− (Fig. 2b),74 [Au13(Ph2PC2H4PPh2)5Cl2]3+ (Fig. 2c),75 and [Au13(BzimBn)9Cl3]2+ (Fig. 2d).76 The interfacial structures around the Au13 core depend on the type of the ligands. In [Au25(SC2H4Ph)18]− and [Au25(C
CPh(CF3)2)18]− protected by X-type ligands, the icosahedral Au13 is capped by six bidentate units of Au2(SR)3 and Au2(C
CR)3, respectively (Fig. 2a and b).72–74 In [Au13(Ph2PC2H4PPh2)5Cl2]3+ and isostructural [Au13(bis-NHC)5X2]3+ protected by L-type ligands, the icosahedral Au13 is capped directly by five bidentate phosphines and NHCs, respectively (Fig. 2c).75,77,78 In [Au13(BzimBn)9Cl3]2+, the icosahedral Au13 is capped directly by nine monodentate NHC ligands and three halides (Fig. 2d).63 Despite the structural diversity in the ligand layers, the n* values calculated by eqn (1) are 8. According to the theoretical studies, the 1P and 1D superatomic orbitals correspond to the highest occupied molecular orbital (HOMO) and the lowest unoccupied molecular orbital (LUMO) (Fig. 1). These Au13 MPCs with a closed electron configuration (1S)2(1P)6 have been considered representative “superatoms”.45–53,72–78 The position of the dopant M in MAu12 MPCs depends on the element.79–92 Group 8–10 elements such as Pd and Pt exclusively occupy the central position (Fig. 2e), whereas group 12 elements such as Cd and Hg prefer the surface position (Fig. 2f). Nevertheless, the n* values calculated by eqn (1) are always 8 regardless of the dopants, indicating that MAu12 also has a closed electron configuration (1S)2(1P)6.
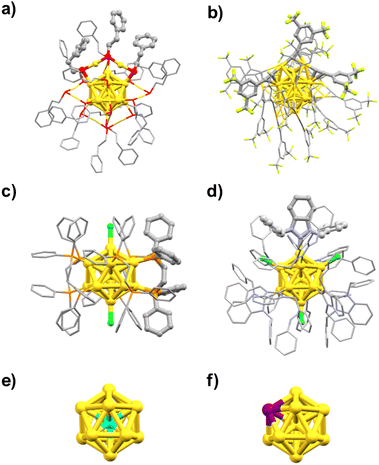 |
| Fig. 2 Molecular structures of (a) [Au25(SC2H4Ph)18]−, (b) [Au25(C CPh(CF3)2)18]−, (c) [Au13(Ph2PC2H4PPh2)5Cl2]3+, (d) [Au13(BzimBn)9Cl3]2+. Color code: Au = yellow, Cl = green, P = orange, N = blue, S = red, F = light green, C = dark grey. Only one ligand each is shown in ball-and-stick representation, other ligands are shown as a wireframe model for improved visualization. Preferential doping site of (e) M = Pd, Pt, Rh, Ir, Ru and (f) M = Cd, Hg in MAu12 core. | |
The photophysical processes of Au MPCs, like those of conventional fluorophores, can be described as follows. First, the photoexcited state generated by a vertical transition from the ground state (S0) relaxes to the vibrational ground state of the first electronically excited state (S1) according to Kasha's rule. Then, three relaxation pathways compete: (1) non-radiative internal conversion (IC) to the vibrational excited state of S0, (2) radiative decay to the vibrational states in the Franck–Condon region in the S0 state (fluorescence), (3) intersystem crossing (ISC) to the triplet state (T1) followed by radiative decay (phosphorescence) to the S0 state.93–95 In the presence of other molecules, deactivation of the T1 state can occur via energy transfer (ET) to oxygen or emitter molecules, followed by generation of singlet oxygen96–98 or upconverted fluorescence emission,99 respectively. Another deactivation pathway for photoexcited Au MPC is via electron transfer (eT), which can be applied to photoredox catalysis.91,100,101 In most cases, the radiative decay of small Au MPCs is dominated by phosphorescence rather than fluorescence due to the strong spin–orbit coupling due to the heavy atom effect. However, in contrast to the case of conventional organic emitters, the radiative decay mechanism of Au MPCs cannot be distinguished by the PL lifetime alone due to the fast ISC and vibrational quenching, resulting in phosphorescence lifetimes of Au MPCs in the range of 100 ns. Therefore, additional experimental evidence (e.g. by triplet state quenching or transient absorption spectroscopy) is required. An overview of the emission mechanisms in Au MPCs was recently provided by Jin.22
Emission energy (Eem) and QY are the key PL properties of Au MPCs that should be tuned for the application. In general, the Eem reflects the electronic structures of Au NCs, such as the energy gap (EHL) between HOMO and LUMO. The Eem is also affected by the relaxation in the electronically excited states, which is determined by the potential energy curves in the S1 and T1 states. The PLQY, on the other hand, is also related to the EHL according to the energy gap law: the rate of non-radiative IC quenching increases inversely proportional to the EHL.102,103 Similarly, the luminescence lifetime follows the energy gap law, and increasing the EHL usually leads to elongated PL lifetimes. Therefore, the EHL is a primary descriptor to characterize the PL properties. In other words, the PL properties can be tuned by controlling the EHL.
In this review article, we first show that the EHL values of Au13/MAu12 MPCs reported so far are distributed over a wide range of 1.2–2.3 eV, although they all share a common electron configuration of (1S)2(1P)6. These data indicate that the electronic structures of Au13 MPC can be finely tuned by the choice of protecting ligands and the dopants. Then, we summarize the PL properties (Eem and PLQY) of Au13/MAu12 MPCs and extract empirical rules on how to tune the Eem values and to enhance the PLQY by the surface modification and doping. In addition, we attempted to rationalize these empirical rules based on modified spherical jellium models. Finally, we discuss the challenges for the future development of Au-based MPCs with designed PL properties. We hope that this review article will help the audience to establish the rational guidelines for designed PL properties.
2. Ligand effects
This section will showcase the characteristic PL properties of Au13 MPCs protected by the respective ligands. Fig. 3 and 4 plot the Eem and PLQY values as a function of EHL, respectively. The data points for Au13 MPCs are indicated by different colors depending on the ligands: thiolates (blue), selenolate (purple), alkynyls (green), phosphines (red), and N-heterocyclic carbenes (orange). The EHL was determined either by the onset in the optical absorption spectrum or by a voltametric method, while the Eem was determined by the peak position in the PL spectrum. Thus, the estimated optical EHL values tend to underestimate the true values. All data presented were recorded under ambient atmosphere unless otherwise noted. In the following, the ligands are divided into two categories, X-type and L-type ligands.
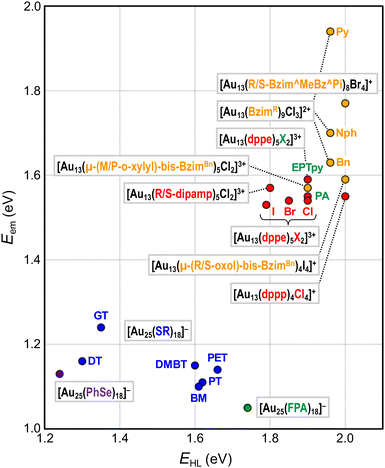 |
| Fig. 3 Overview of Eem as a function of EHL for Au13 MPCs. Color code: thiolate (blue), selenolate (purple), alkynyl (green), phosphine (red), NHC (orange). | |
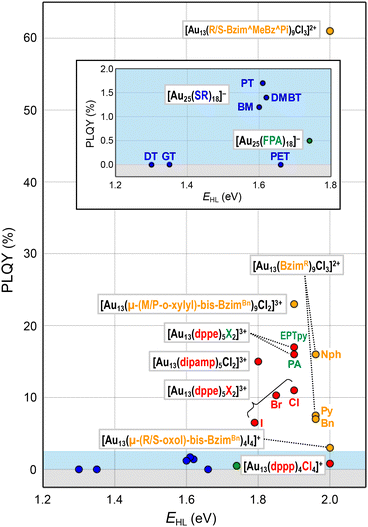 |
| Fig. 4 Overview of PLQYs as a function of EHL for Au13 MPCs protected by X-type ligands (inset) and L-type ligands. Color code: thiolate (blue), alkynyl (green), phosphine (red), NHC (orange). | |
2.1. X-type ligands
2.1.1. Thiolates.
The EHL and Eem values of Au13 protected by various thiolates (RS, Scheme 1) are in the range of 1.30–1.66 and 1.10–1.24 eV, respectively, depending on the structure of the R group (blue dots in Fig. 3). The PLQY was relatively small and distributed in the range of 0.1–1.7% (blue dots in Fig. 4). More detailed descriptions of the effects of the thiolate structure on the PL properties are given below.
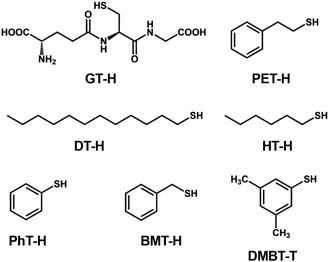 |
| Scheme 1 Structures and abbreviations of the RS ligands used to protect the Au13 core in section 2.1.1. | |
[Au
25
(GT)
18
]
− (GT-H = glutathione, Scheme 1): this cluster is the first member of the ubiquitous family of Au25(SR)18, reported by Negishi and Tsukuda in 2005.104,105 The EHL and Eem were 1.35 and 1.24 eV,94 respectively (Fig. 3), while the PLQY was only 2 × 10−3% (Fig. 4). The PL intensity of [Au25(GT)18]− was increased by ∼1.8 times in [Au25(GT)18−x(SPNA)x]−, where PNA represents a long-chain peptide nucleic acid with a high proportion of electron-rich N and O atoms.106 This enhancement was attributed to electron donation by electron-rich groups of SPNA (e.g. carboxy and amino groups) to the Au13 core.
[Au
25
(PET)
18
]
− (PET-H = phenylethylthiol, Scheme 1): this cluster is the first member of the Au25(SR)18 family whose structures were theoretically predicted by Grönbeck,107 and experimentally determined independently by Murray72 and Jin73 in 2008. The EHL and Eem were 1.66 and 1.14 eV, respectively, indicating a large Stokes shift (Fig. 3).108 Note that this EHL corresponds to the value at 0 K estimated from temperature-dependent optical spectra based on the Bose–Einstein model and is larger than that estimated from optical onset.104 The PLQY was only 1 × 10−2% (Fig. 4).106 [Au25(PET)18]− showed a fluorescence lifetime of 0.73 μs. Theoretical calculations by Aikens revealed that the PL is dominated by core-based transitions with a very small contribution from the outer Au atoms in the staple ligands.109
[Au
25
(DT)
18
]
− and [Au25(HT)18]− (DT-H = 1-dodecanethiol; HT-H = 1-hexanethiol, Scheme 1): the EHL and Eem of [Au25(DT)18]− were 1.3 and 1.16 eV, respectively (Fig. 3).110,111 Wu and Jin reported that the PLQYs of [Au25(DT)18]− and [Au25(HT)18]− were 5 × 10−3 and 2 × 10−3%, respectively (Fig. 4).106 The PLQY of [Au25(SR)18]− decreased in the order of SR = PET > DT > HT, which is parallel to the order of the ability of the ligands to donate electronic charge to the Au13 core. The PL lifetimes were 0.42 and 0.64 μs for [Au25(DT)18]− and [Au25(HT)18]−, respectively. Theoretical calculations revealed that core-based transitions as well as ligand-core interactions are essential for the PL behavior of [Au25(SR)18]− (R = H, CH3, C2H5, C3H7).109
[Au
25
(PhT)
18
]
−, [Au25(BMT)18]−, [Au25(DMBT)18]− (PhT-H = phenylthiol, BMT-H = benzylmethanethiol, DMBT-H = 3,5-dimethylbenzenethiol, Scheme 1): effects of aromatic thiolates on the optical properties of [Au25(SR)18]− have been reported by Jin.108 The EHL and Eem of [Au25(SR)18]− (SR = PhT, BMT, DMBT) were comparable to those of [Au25(PET)18]− (Fig. 3), indicating that the aromatic group does not significantly affect the electronic structures. [Au25(SR)18]− (SR = PhT, BMT, DMBT) showed PLQYs of 1.7, 1.5 and 1.3%, respectively (Fig. 4), significantly larger than that of [Au25(PET)18]−.
Typically, [Au25(SR)18]− exhibits an Eem in the NIR region and PLQYs of <2%. Overall, the geometric structure of the R group does not significantly affect the electronic structures of [Au25(SR)18]− in terms of the EHL and Eem, because the HOMO and LUMO are mostly localized in the common Au13 cores. In contrast, the electronic structure of the R group affects the PLQYs. In particular, R groups with electron-rich atoms (e.g., O, N) or functional groups enhance the PLQY by donating electronic charge to the Au13 core.
2.1.2. Selenolates.
[Au
25
(PhSe)
18
]
− (PhSe-H = phenylselenol): PhSe, an analogue of PhS, was used by the group of Wu to protect the Au13 core.112 Square-wave voltammetry of [Au25(PhSe)18]− revealed a EHL of 1.24 eV, which is significantly smaller than that of [Au25(PhT)18]− (1.6 eV). The Eem of [Au25(PhSe)18]− was 1.13 eV (purple dot in Fig. 3), comparable to that of [Au25(PET)18]− (1.14 eV), while the PLQY was not reported.
2.1.3. Alkynyls.
The molecular structures of the alkynyls used are shown in Scheme 2.
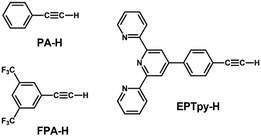 |
| Scheme 2 Structures and abbreviations of the alkynyl ligands used to protect the Au13 core in sections 2.1.3 and 2.2.1. | |
[Au
25
(FPA)
18
]
− (FPA-H = 3,5-bis(trifluoromethyl)phenyl-acetylene, Scheme 2): Wang synthesized [Au25(FPA)18]− by direct reduction of the precursor gold complex Au(I)-FPA.74 While the Au(I)-alkynyl staples have similar structures to Au(I)-SR staples (Fig. 2b), [Au25(FPA)18]− has a chiral D3 symmetry, due to the higher steric demand of alkynyl ligands than thiolates in [Au25(SR)18]−. The EHL and Eem of [Au25(FPA)18]− were 1.74 (determined electrochemically) and 1.05 eV, respectively (green dot in Fig. 3).87 The PLQY was 0.5% (green dot in Fig. 4).
2.2. L-type ligands
In contrast to the formation of short staple motifs in the protection by X-type ligands (Fig. 2a and b), L-type ligands such as phosphines and N-heterocyclic carbenes bind directly to the Au13 core together with auxiliary X-type ligands (Fig. 2c and d).
2.2.1. Phosphines.
With few exceptions such as [Au13(PPhMe2)10Cl2]3+,113 [Au13(PPh2Me)8Cl4]+,114 and [Au13(P(Oct)3)8Cl4]+,115 diphosphines (Scheme 3) have been conventionally used as protecting ligands of Au13. The EHL and Eem values of diphosphine-protected Au13 are in the range of 1.79–1.90 and 1.53–1.57 eV, respectively (red dots in Fig. 3). The PLQY was in the range of 6–17% (red dots in Fig. 4), which is significantly larger than those of Au13 protected by X-type ligands (section 2.1). This section focuses on the effects of diphosphine structures (Scheme 3) on the PL properties.
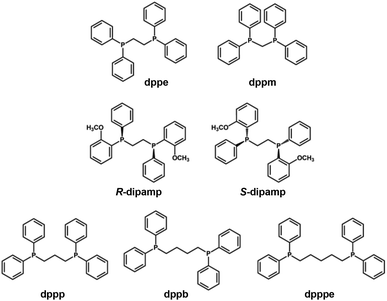 |
| Scheme 3 Structures and abbreviations of the diphosphine ligands used to protect the Au13 core in section 2.2.1. | |
[Au
13
(dppe)
5
X
2
]
3+
(dppe = 1,2-bis(diphenylphosphino)ethane, X = Cl, Br, I, Scheme 3): [Au13(dppe)5Cl2]3+ was synthesized by Konishi in 2010 using size focusing (“etching”) with HCl.75 The EHL and Eem were 1.9 and 1.55 eV, respectively (red dots in Fig. 3). The PLQY was 11% (red dot in Fig. 4), with a phosphorescence lifetime of 2.69 μs.116 The EHL of [Au13(dppe)5X2]3+ decreased in the order of X = Cl > Br > I from 1.90 to 1.79 eV, while the Eem values were comparable (1.53–1.54 eV) regardless of X.117 Accordingly, the PLQY decreased in the same order by 11% (Cl) > 10.3% (Br) > 6.5% (I) (red dots in Fig. 4).
[Au
13
(dppe)
5
(PA)
2
]
3+
(PA-H = phenylacetylene, Scheme 2): Konishi synthesized [Au13(dppe)5(PA)2]3+via a ligand exchange reaction of [Au13(dppe)5Cl2](PF6)3 with PA-H.116 The EHL of [Au13(dppe)5(PA)2]3+ (1.9 eV) was same as that of [Au13(dppe)5Cl2]+ (red dot in Fig. 3). DFT calculations showed that the HOMO of [Au13(dppe)5(PA)2]3+ is extended over the PA units. Likewise, HOMO−1, HOMO−2 and HOMO−3 with superatomic P-like character located on the Au13 core are distributed over the π system of PA. The interaction of the superatomic valence electrons of Au13 with the π-orbitals then leads to the generation of new and unique molecular orbitals. The Eem value of [Au13(dppe)5(PA)2]3+ (1.55 eV, red dot in Fig. 3) was also comparable to that of [Au13(dppe)5Cl2]+, the PLQY of the former (16%, red dot in Fig. 4) was higher than that of the latter (11%), which was attributed to a higher quenching effect of Cl in comparison to PA. This trend is consistent with the longer PL lifetime of the former (3.51 μs) compared to the latter (2.69 μs).
[Au
13
(dppe)
5
(EPTpy)
2
]
3+
(EPTpy-H = 4′-(4-ethynylphenyl)-2,2′:6′,2′′-terpyridine, Scheme 2): in 2023, our group successfully obtained [Au13(dppe)5(EPTpy)2]3+ with two terpyridyl moieties on opposite sides of the Au13 core.118 The EHL, Eem and PLQY of [Au13(dppe)5(EPTpy)2]3+ (1.9 eV, 1.59 eV, 17%) were comparable to those of [Au13(dppe)5(PA)2]3+ (1.9 eV, 1.55 eV, 16%). The PL of [Au13(dppe)5(EPTpy)2]3+ was completely quenched by coordination of Co2+, Ni2+ or Cu2+ at the terpyridyl moieties, but not by Zn2+.118 The PL lifetime of [Au13(dppe)5(EPTpy)2]3+ decreased from 3.66 μs to 0.02 μs upon coordination of Co2+, while it remained unchanged upon coordination of Zn2+ (3.41 μs). This PL quenching phenomenon was explained by efficient energy transfer from the photoexcited Au13 core to the d9 transition metal cations via electron exchange between them.
[Au
13
(R/S-dipamp)
5
Cl
2
]
3+
(R/S-dipamp = (R,R)/(S,S)-2-bis[(2-methoxyphenyl)phenylphosphino]ethane, Scheme 3): Yang reported the synthesis of enantiopure Au13 clusters [Au13(R-dipamp)5Cl2]3+ and [Au13(S-dipamp)5Cl2]3+ using the chiral diphosphine R/S-dipamp.119 The EHL of [Au13(R-dipamp)5Cl2]3+ (1.8 eV) was slightly smaller than that of [Au13(dppe)5Cl2]3+ (1.9 eV) (red dots in Fig. 3). Konishi showed that the Eem of [Au13(R-dipamp)5Cl2]3+ (1.57 eV) was comparable to that of [Au13(dppe)5Cl2]3+ (1.54 eV) (red dots in Fig. 3) and that the PLQY (15%) was slightly higher than that of [Au13(dppe)5Cl2]3+ (11%) (red dots in Fig. 4).120 This was attributed to an increased core rigidification, as the chiral R/S-dipamp ligands induced additional torsional strain on the icosahedral Au core. [Au13(R-dipamp)5Cl2]3+ and [Au13(S-dipamp)5Cl2]3+ exhibited circularly polarized luminescence (CPL) with emission maxima at 1.63 eV and emission anisotropy factors of 2.5 × 10−3 and 2.3 × 10−3, respectively.120
[Au
13
L
4
Cl
4
]
+
(L = Ph2P-(CH2)m-PPh2 with m = 3 (dppp), 4 (dppb), 5 (dpppe), Scheme 3): the chemical formula of Au13 protected by long bis(diphenylphosphino) ligands [Au13L4Cl4]+ (m = 3–5) differ from [Au13(dppe)5Cl2]3+ (m = 2).115 Konishi reasonably proposed that [Au13L4Cl4]+ adapted an icosahedral Au13 core, although SCXRD analysis was not possible. The absorption spectrum profiles of [Au13L4Cl4]+ (m = 3–5) were similar regardless of m, but were significantly different from that of [Au13(dppe)5Cl2]3+ (m = 2). While the EHL and Eem values of [Au13(dppp)4Cl4]+ (∼2.0 and 1.60 eV, red dots in Fig. 3) were similar to those of [Au13(dppe)5Cl2]3+ (∼2.0 and 1.55 eV, red dots in Fig. 4), the PLQY of [Au13(dppp)4Cl4]+ (0.8%) was significantly lower than that of [Au13(dppe)5Cl2]3+ (11%). This remarkable PL quenching suggests that diphosphines linked by longer alkyl chains cannot rigidify the Au13 and thus cannot suppress non-radiative decay. The PL properties of [Au13L4Cl4]+ (m = 4, 5) have not been reported.
Overall, the EHL and Eem values of diphosphine-protected Au13 are larger than those of Au13 protected by X-type ligands as shown in section 2.1. The PLQYs were significantly increased compared to those of thiolate-protected Au13. This trend is in parallel to the energy gap law between PLQY and EHL.102,103 The mechanical stress imposed by the bidentate ligation of diphosphines may contribute to the suppression of non-radiative decay by stiffening the Au13 core and to the emergence of CPL by distorting the Au13 core into a chiral structure. Alkynyls as X-type auxiliary ligands largely enhance the EHL by electronic conjugation, while the effect of halides as X-type auxiliary ligands is relatively small.
2.2.2. Arsines and stibines.
In contrast to monodentate phosphines, monodentate arsines and stibines can protect the icosahedral Au13 core.
[Au
13
(AsPh
3
)
8
Cl
4
]
+
: the [Au13(AsPh3)8Cl4]+ reported by Sun exhibited a phosphorescence of Eem = 1.55 eV,121 which is comparable to that of [Au13(dppe)5Cl2]3+ (1.54 eV), but smaller than that of [Au13(P(Oct)3)8Cl4]+ (Eem = 1.60 eV).115 No other PL properties, including the PL QY, have been reported.
[Au
13
(dpap)
5
Cl
2
]
3+
(dpap = Ph2As-(CH2)3-AsPh2): Konishi reported on the diarsine-protected cluster [Au13(dpap)5Cl2]3+.122 The EHL was ∼2.0 eV, while the Eem and PLQY were 1.63 eV and 7%, respectively. The Eem and PLQY were slightly larger and smaller than those of [Au13(dppe)5Cl2]3+ (1.55 eV and 11%, respectively). The difference in their electronic structures was attributed to subtle deformation of the Au13 icosahedron, rather than the coordinating atoms (As or P).
[Au
13
L
8
Cl
4
]
+
(L = SbPh3, Sb(p-tolyl)3): Leong reported that [Au13L8Cl4]+ exhibited both fluorescence at Eem = 1.68 eV and phosphorescence at Eem = 1.50 eV due to a heavy metal effect of Sb.123 This dual emission behavior of [Au13L8Cl4]+ was attributed to facilitated reverse ISC from the T1 to the S1 state.
Overall, the Eem values for the phosphorescence increased in the order of [Au13(SbPh3)8Cl4]+ < [Au13(AsPh3)8Cl4]+ < [Au13(P(Oct)3)8Cl4]+.
2.2.3. N-heterocyclic carbenes.
In contrast to the phosphine ligands, NHC ligands can stabilize the Au13 cores even in monodentate form via strong Au–C bonds.124 Further stabilization can be achieved by using bidentate NHC ligands. One of the practical advantages of NHCs is their structural designability for fine-tuning the PL properties of Au13 NCs. Therefore, the effects of the substituent group R at the wingtips and multidentate ligation on the PL properties have been systematically investigated. The molecular structures of the NHCs used are shown in Scheme 4. The EHL values of NHC-protected Au13 are nearly constant at ∼2.0 eV, while the Eem values vary over a wide range of 1.57–1.94 eV (orange dots in Fig. 3). The PLQYs are comparable to those of diphosphine-protected Au13 in most cases, but it can be as high as 61% when using a specific NHC (orange dots in Fig. 4). The increase in PLQY was attributed to the stiffening of the Au13 core by π⋯π or C–H⋯π interaction between the adjacent NHC ligands. More detailed descriptions of the effects of the NHC structures on the PL properties are given below.
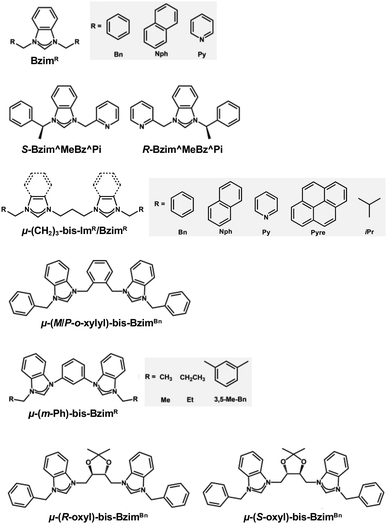 |
| Scheme 4 Structures and abbreviations of the NHC ligands used to protect the Au13 core in section 2.2.3. | |
[Au
13
(Bzim
R
)
9
Cl
3
]
2+
(BzimR = benzyl/naphthyl/pyridyl-benzimidazole for R = Bn, Nph, and Py, respectively, Scheme 4): [Au13(BzimR)9Cl3]2+ is an ideal benchmark system to study how the wingtips of NHC ligands affect the PL properties.76,77 While the EHL values were ∼2.0 eV independent of R, the Eem values were 1.63, 1.70 and 1.94 eV (orange dots in Fig. 3) with the PLQYs of 7, 16, and 7.5% (orange dots in Fig. 4), for R = Bn, Nph, and Py, respectively. The highest PLQY for R = NPh was explained by the stiffening of the Au13 core by the π⋯π interaction between the naphthyl wingtips.
[Au
13
(R/S-Bzim^MeBz^Pi)
8
Br
4
]
+
(R/S-Bzim^MeBz^Pi = R/S-pyridine-ylmethylbenzylbenzimidazole, Scheme 4): Zang reported the synthesis of enantiopure Au13 clusters [Au13(R-Bzim^MeBz^Pi)8Br4]+ and [Au13(S-Bzim^MeBz^Pi)8Br4]+ using chiral benzimidazole ligands.125 The EHL and Eem values were 2.00 and 1.77 eV, respectively (orange dot in Fig. 3). The PLQY was as high as 61% (orange dot in Fig. 4) with a PL lifetime of 3.07 μs. This extraordinary high PLQY was explained by the combination of two factors: rigidification of the Au13 core by multiple interligand interactions such as C–H⋯N, C–H⋯π, C–H⋯Au and π⋯π interactions and higher electron-donating capability of R/S-Bzim^MeBz^Pi than other NHC ligands. [Au13(R-Bzim^MeBz^Pi)8Br4]+ and [Au13(S-Bzim^MeBz^Pi)8Br4]+ exhibited CPL with an Eem of 1.63 eV and a dissymmetry factor of 2.65 × 10−3 in a frozen DCM solution.
[Au
13
(μ-(CH
2
)
3
-bis-Bzim
R
)
5
X
2
]
3+
(μ-(CH2)3-bis-BzimR = μ-propyl-di-benzyl/naphthyl/pyridyl/pyrenyl-bis-benzimidazole for R = Bn, Nph, Py, Pyre; X = Cl, Br, Scheme 4): although the PL properties of these MPCs could not be plotted in Fig. 3 and 4 due to the lack of the EHL values, the remarkable effects of the wingtips on the PL were observed. The Eem values of [Au13(μ-(CH2)3-bis-BzimBn)5Br2]3+ and [Au13(μ-(CH2)3-bis-BzimPy)5Br2]3+ were 1.91 and 1.94 eV, respectively, while their PLQYs were both 15%.126 Extending the π-conjugation of the R group further improved the PLQY. The PLQY increased from 38% for [Au13(μ-(CH2)3-bis-BzimNph)5Cl2]3+ to 56% for [Au13(μ-(CH2)3-bis-BzimPyre)5Cl2]3+, while their Eem values were both 1.75 eV. Consistent with this enhancement of the PLQY, their PL lifetimes were extended from 2.6 to 3.4 μs. This enhancement of the PLQY is associated with the stiffening of the Au13 core by stronger π⋯π interaction between the adjacent ligands. The PLQYs of [Au13(μ-(CH2)3-bis-BzimR)5X2]3+ were higher than those of [Au13(BzimBn)9Cl3]2+ (7%).77,126 This enhancement of PLQY was attributed to the suppression of non-radiative decay of the photoexcited state by restricting the motion of the Au13 core by the bidentate coordination of μ-(CH2)3-bis-BzimR.
[Au
13
(μ-(CH
2
)
3
-bis-Im
R
/Bzim
R
)
m
(tpp)
n
X
2
]
3+
(μ-(CH2)3-bis-ImR = μ-propyl-di-benzyl/iso-propyl-bis(benz)imidazole for R = Bn, iPr; tpp = PPh3; X = Cl, Br; (m, n) = (2, 4), (3, 3), Scheme 4): the Biffis group reported mixed NHC/phosphine protected Au13 clusters.127 All clusters showed the same Eem of 1.63 eV regardless of R or NHC/phosphine ratio, while the EHL of the clusters was not reported. The PLQYs were significantly different depending on the NHC/phosphine ratio. [Au13(μ-(CH2)3-bis-ImBn)2(tpp)4Cl2]3+ and [Au13(μ-(CH2)3-bis-BzimBn)2(tpp)4Cl2]3+ and showed QYs of 4 and 3%, respectively, while [Au13(μ-(CH2)3-bis-BzimBn)3(tpp)3Cl2]3+ showed a high PLQY of 44%. This was attributed to increased rigidity due to a higher number of NHC ligands. PL lifetimes of the clusters were not reported.
[Au
13
(μ-(M/P-o-xylyl)-bis-Bzim
Bn
)
5
Cl
2
]
3+
(μ-(M/P-o-xylyl)-bis-BzimBn = minus/plus-(μ-o-xylyl)-dibenzyl-bisbenzimidazole, Scheme 4): the EHL and Eem of [Au13(μ-(M/P-o-xylyl)-bis-BzimBn)5Cl2]3+ were 1.9 and 1.57 eV, respectively (orange dot in Fig. 3).78 The PLQY was 23% (orange dot in Fig. 4), which is higher than that of the monodentate counterpart [Au13(BzimBn)9Cl3]2+ (7%). This led to an increased core rigidification due to both bidentate coordination by the ligand system on the one hand, and the exertion of torsional strain on the Au core on the other hand. These effects prevented vibrational quenching of the excited state. Although CPL properties might be expected due to the ligand-induced torsion of the Au13 core, CPL spectra have not been reported.
[Au
13
(μ-(m-Ph)-bis-Bzim
R
)
5
Br
2
]
3+
(μ-(m-Ph)-bis-BzimR = μ-(m-phenylene)-di-methyl/ethyl/3,5-dimethylbenzyl-bis-benzimidazole for R = Me, Et, 3,5-Me2Bn, Scheme 4): [Au13(μ-(m-Ph)-bis-BzimR)5Br2]3+, reported by Mak, demonstrated the key role of the NHC wingtip R in the relaxation behavior of bis-NHC Au13.128 While the Eem value was nearly constant (1.75 eV) regardless of R, the PLQYs increased from 8 to 16% in the order of R = Me < Et < 3,5-Me2Bn, which was explained by an increased rigidification by the bulkier ligand systems. Counterintuitively, the PL lifetime decreased from 1.03 to 0.80 μs in the same order. The origin of the discrepancy between the PLQY and the PL lifetime is unknown.
[Au
13
(μ-(R/S-oxol)-bis-Bzim
Bn
)
4
I
4
]
+
(μ-(R/S-oxol)-bis-BzimBn = μ-(R/S-2,2-dimethyl-1,3-dioxolane)-dibenzyl-bis-benzimidazole, Scheme 4): the number of bis-NHC ligands (4) in this cluster is smaller than that in other Au13 NCs protected by bis-NHC ligands (5) due to the greater steric hindrance of the ligands.129 [Au13(μ-(R/S-oxol)-bis-BzimBn)4I4]+ showed an EHL of 2.0 eV and an Eem of 1.59 eV (orange dot in Fig. 3). The PLQY was 3% (orange dot in Fig. 4), which is significantly lower compared to the bis-NHC stabilized Au13 NCs mentioned above. This behavior was attributed to less efficient core stiffening due to a smaller number of NHC ligands and confinement of valence electrons to a smaller volume due to a larger number of X-type ligands.
2.3. Qualitative explanation of ligand effects on the PL properties
Overall, Fig. 3 shows that EHL values of the Au13 core can be tuned over a wide range of 1.2–2.0 eV by surface modification. There is a clear trend that EHL values for the Au13 NCs protected by L-type ligands (phosphines, NHCs) are much larger than those protected by X-type ligands (thiolates, alkynyls). We previously explained this trend based on the spherical jellium model (Fig. 5). The Au13 protected by X-type ligands is viewed as a formal Au135+ core protected by six electronegative surface units (e.g. [Au2(SR)3]−). In contrast, the Au13 protected by L-type ligands is viewed as a formal Au135+ core protected mostly by electroneutral ligands with few electronegative ligands (e.g. halogen, alkynyls). We make a simple assumption that the isotropic siege of the Au13 core by electroneutral ligands leads to the expansion of potential volume for the confinement of valence electrons (step ❶ in Fig. 5), and thereby stabilizing both HOMO and LUMO orbitals, corresponding to 1P and 1D superatomic orbitals, respectively (step ❷ in Fig. 5). If we assume that the 1P orbitals have a larger populated outer region of the core than the 1D orbitals, we can predict that the 1P orbitals are stabilized more than 1D orbitals when reducing the negative charge on the ligand layer by using L-type ligands.53 This qualitatively explains why the EHL values of Au13 protected by L-type ligands are obviously larger than those of Au13 protected by X-type ligands.
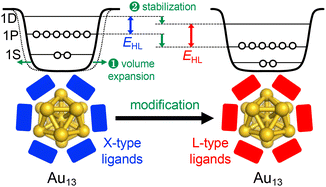 |
| Fig. 5 Schematic jellium potentials for the Au13(8e) core protected by X-type (left) and L-type (right) ligands. The blue and red rectangles represent X-type and L-type ligands, respectively. | |
Fig. 3 shows that the Eem values for the Au13 NCs protected by L-type ligands (1.5–1.9 eV) are much larger than those by X-type ligands (1.1–1.3 eV). This ligand effect is not only due to larger EHL values for the former, but also a smaller Stokes shift for the L-type ligation (0–0.4 eV) than X-type ligation (0.1–0.6 eV). The trend in the Stokes shift suggests that the photoexcited state of Au13 NC protected by X-type ligands undergoes larger relaxation, but the reason is not clear at this moment.
Fig. 4 illustrates that the PLQYs for the protection with L-type ligands are much larger than those with X-type ligands. The PL of Au13 protected by L-type ligands is basically assigned to phosphorescence from the T1 state because the excited lifetime is in the order of μs.95 The correlation between the EHL and PLQY is in line with the energy gap law, in which an expansion of the EHL can efficiently suppress the non-radiative decay process of photoexcited states.102,103 Practically, L-type ligands are suitable to enhance the PLQY. Further enhancement in PLQY is expected by proper design of the ligand structures to enhance the noncovalent interaction (π⋯π, C–H⋯π, and hydrogen bonding) between the adjacent ligands. The non-radiative decay of the photoexcited state can be suppressed by the rigidification of the Au13 core by these interligand interactions. Recently, Tsukuda demonstrated the importance of ligand packing on the PLQY. The PLQY of [IrAu12(dppe)5(PA)2]+ decreased from 53% to 25% upon the removal of one PA ligand by Brønsted acid treatment.130 In contrast, the PLQY of the resulting [IrAu12(dppe)5(PA)]2+ dramatically increased up to 70% by introducing an isocyano-adamantane to the vacant site. These results clearly indicated that dense packing in the ligand layers facilitate the rigidification of the Au13 core to improve the PLQY.
3. Doping effects
This section focuses on the effects of heterometal doping on the PL properties of Au13 MPCs. According to the energy gap law,102,103 broadening of the HOMO–LUMO energy gap is a guiding principle for improving the PLQY. Tuning the electronic structure by heterometal doping is an effective method to change the HOMO/LUMO levels of Au13 MPCs.65–71 So far, group 8–12 elements have been successfully doped into the icosahedral Au13 core. The formal charge and preferred dopant sites in Au13 are listed in Table 1. Doping a heterometal into the Au13 core modifies the depth and shape of the jellium potential, leading to an upshift or downshift of the superatomic S, P, and D orbitals, thereby changing the HOMO–LUMO gap of the Au13 MPC. Typical examples of the doping effects on the electronic structure and the PL properties are described below. Fig. 6 and 7 show the Eem and PLQY as a function of EHL, respectively. The data for doped Au13 MPCs are shown in lighter tones depending on the ligands while the data for undoped Au13 MPCs are shown in brighter tones. As described in the introduction, the total charge z of the doped Au13 MPC differs depending on the dopant group to keep the n* value at 8. Therefore, in the following, the z value is omitted for simplicity.
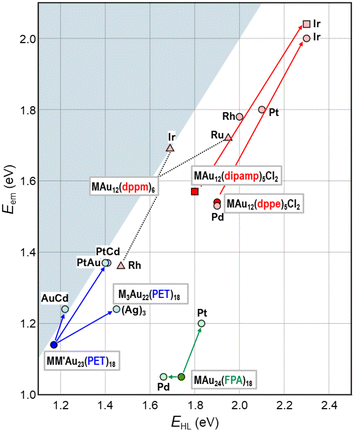 |
| Fig. 6 Overview of Eem as a function of EHL for doped Au MPCs. Color code for ligands: thiolate (blue), alkynyl (green), phosphine (red). The data for undoped Au13 MPCs (M, M′ = Au) are shown in brighter tones. The arrows indicate the change in Eem and EHL after doping with the respective heterometal. | |
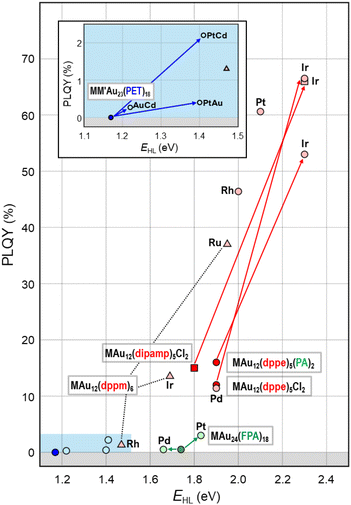 |
| Fig. 7 Overview of PLQY as a function of EHL for doped Au MPCs. Color code for ligands: thiolate (blue), alkynyl (green), phosphine (red). The data points for undoped Au13 MPCs (M, M′ = Au) are painted in brighter tones. The arrows indicate the change in PLQY and EHL after doping with the respective heterometal. | |
3.1. X-type ligands
MM′Au
23
(PET)
18
((M, M′) = (Pt, Au), (Au, Cd), (Pt, Cd)): multi-metal doping can synergistically enhance the PL properties.89 PtAu24(PET)18 had a Pt-centered icosahedral Pt@Au12 core.131 The EHL and Eem values of PtAu24(PET)18 having a PtAu12(8e) core were 1.40 and 1.37 eV, respectively (light blue dot in Fig. 6), which are significantly larger than those of undoped Au25(PET)18 (1.66 and 1.21 eV): the EHL value of 1.17 eV estimated from the optical onset was used for comparison rather than that at 0 K (1.66 eV) estimated from temperature-dependent optical spectra based on the Bose–Einstein model.108 The PLQY of PtAu24(PET)18 was 0.41% (light blue dots in Fig. 7), ∼9 times larger than that of Au25(PET)18 (0.048%),88 due to the increase in EHL. In contrast, the Cd dopant in CdAu24(PET)18 was located on the surface of the Au@Au11Cd core and the EHL and Eem values were 1.22 and 1.24 eV, respectively (light blue dot in Fig. 6),89 comparable to those of undoped Au25(PET)18. The PLQY of CdAu24(PET)18 (0.27%, light blue dot in Fig. 7) was ∼5 times higher than that of Au25(PET)18, due to a larger transition dipole moment from the photoexcited state to the ground state. Interestingly, the Pt- and Cd-codoped cluster PtCdAu23(PET)18 had an icosahedral Pt@Au11Cd core and has the EHL and Eem values of 1.41 and 1.37 eV, respectively (light blue dot in Fig. 6). The PLQY of PtCdAu23(PET)18 was 2.2%, 46 times higher than that of Au25(PET)18. Since the enhancement factor was expressed by the products of those by Pt (×9) and Cd (×5) doping, the PL enhancement was associated with the synergy of two independent mechanisms by Pt and Cd doping.
Ag
3
Au
22
(PET)
18
: in 2013, Kauffman et al. reported on Ag3Au22(PET)18, with all 3 Ag atoms occupying positions on the surface to form an Au@Au9Ag3 icosahedral core.132 Optical absorption spectroscopy revealed an increase in both EHL (1.45 eV) and Eem (1.24 eV) compared to undoped Au25 (light blue dot in Fig. 6). DFT calculations indicated that the shift was due to an increase in the LUMO energy level, while the HOMO energy level remained unaffected. The PLQYs of Ag3Au22(PET)18 were not reported.
Ag
x
Au
25−x
(DT)
18
(x ∼1, ∼4, ∼6, ∼7, ∼9): Negishi succeeded in increasing the number of Ag dopants to Au25(DT)18 with an accuracy of ±1 atom.111 There was an overall trend of increasing EHL and Eem with increasing Ag dopant content. In contrast, PLQY varied in the range of 1–4%, with no clear dependence on x observed.
MAu
24
(FPA)
18
(M = Au, Pd, Pt): the doping of one Pd atom on Au25(FPA)18 decreased the EHL value from 1.74 to 1.66 eV, while Pt doping increased the EHL value from 1.74 to 1.83 eV.87 The Eem value showed little change from 1.05 to 1.06 eV with Pd doping on Au25(FPA)18, while it increased to 1.20 eV with Pt doping (light green dots in Fig. 6). The Pt-doped cluster has the largest EHL and shows the highest PLQY of 3% in contrast to the undoped or Pd-doped clusters (0.5% each, light green dots in Fig. 7), which was due to a large increase in the EHL induced by Pt doping.
3.2. L-type ligands
MAu
12
(dppe)
5
Cl
2
(M = Au, Pt, Pd, Rh, Ir): doping Au13(dppe)5Cl2 with a group 9 (Rh, Ir) or group 10 (Pt, Pd) increased the EHL value in the order of 1.9 eV (M = Pd) ≤ 1.9 eV (Au) < 2.0 eV (Rh) < 2.1 eV (Pt) < 2.3 eV (Ir).91 In accordance with the increase in EHL, the Eem was shifted toward higher energies (light red dots in Fig. 6): 1.53 eV (M = Pd) < 1.54 eV (Au) < 1.78 eV (Rh) < 1.80 eV (Pt) < 2.00 eV (Ir), and correspondingly, the PLQY increased dramatically (light red dots in Fig. 7): 11% (M = Pd) ∼12% (Au) < 46% (Rh) < 61% (Pt) < 67% (Ir) under Ar atmosphere. The PL lifetime was also increased in the following order: 2.88 μs (M = Au) < 3.07 μs (Pd) < 5.71 μs (Pt) < 6.03 μs (Ir) < 6.96 μs (Rh), which can be explained by the energy gap law. In the presence of O2, the PLQYs for M = Rh and Ir were significantly reduced to 0.17 and 0.15% of the initial values, respectively, while that of the undoped clusters remained almost unaffected at 11%. This PL quenching by O2 indicates that phosphorescence is responsible for the PL of Rh- or Ir-doped clusters.
MAu
12
(dppe)
5
X
2
(M = Au, Ir; X = Br, I): the EHL and Eem values of IrAu12(dppe)5Br2 were 2.01 and 1.92 eV, while those of IrAu12(dppe)5I2 were 2.03 and 1.96 eV, respectively.100 These values are larger than those for the undoped counterparts (1.85, 1.54 eV) and (1.79, 1.54 eV). Accordingly, the PLQY of IrAu12(dppe)5Br2 and IrAu12(dppe)5I2 under Ar atmosphere remarkably increased to 75 and 77%, respectively, from those of the undoped counterparts (10.3 and 6.5%). These QYs of IrAu12(dppe)5X2 (X = Br, I) are among the highest in MAu12 NCs, but are not plotted in Fig. 6 and 7 because the doping effects were similar to those of Au13(dppe)5Cl2. The PL lifetimes of IrAu12(dppe)5X2 under Ar atmosphere were in the μs order (6.03, 4.90, and 4.15 μs), while they were reduced to 8.04, 7.16, and 6.65 ns for X = Cl, Br, and I, respectively, under air. The PL quenching of IrAu12(dppe)5X2 by O2 indicates that a phosphorescence from T1 state, populated by efficient ISC, is responsible for the radiative decay.
MAu
12
(dppe)
5
(PA)
2
(M = Au, Ir): the EHL and Eem of IrAu12(dppe)5(PA)2 were 2.3 eV and 2.02 eV, respectively.130 The PLQY increased from 16% for Au13(dppe)5(PA)2 to 53% for IrAu12(dppe)5(PA)2. Again, the data are not plotted in Fig. 6 and 7 for comparison as the doping effect was similar to that in MAu12(dppe)5Cl2.
MAu
12
(R/S-dipamp)
5
Cl
2
(M = Au, Ir): the EHL and Eem values of IrAu12(S-dipamp)5Cl2 were 2.3 and 2.04 eV, respectively (light red square in Fig. 6), which are significantly larger than those of undoped Au13(R/S-dipamp)5Cl2 were 1.9 and 1.57 eV, respectively.133 As expected from the increased EHL, IrAu12(S-dipamp)5Cl2 showed a significantly increased PLQY (66%) and PL lifetime (5.87 μs) than those of undoped Au13(R/S-dipamp)5Cl2 (15%, 3.19 μs) (light red square in Fig. 7). IrAu12(R/S-dipamp)5Cl2 exhibited CPL properties. Although Ir doping did not appreciably enhance the PL anisotropy factors, the brightness of the CPL of IrAu12(S-dipamp)5Cl2 was five times higher than that of undoped Au13(S-dipamp)5Cl2. This enhancement in CPL brightness is mainly due to that in the PLQY.
MAu
12
(dppm)
6
(M = Au, Ru, Rh, Ir): undoped Au13(dppm)6 had a significantly distorted Au13 core,134 whereas MAu12(dppm)6 (M = Ru, Rh, Ir) had an icosahedral M@Au12 core.90 The EHL value increased in the order of 1.47 eV (M = Rh) ≤ 1.69 eV (Ir) < 1.95 eV (Ru). Although Au13(dppm)6 was non-emissive, MAu12(dppm)6 exhibited PL behavior. Consistent with the increase in EHL, the Eem was shifted to higher energies (light red triangles in Fig. 6): 1.48 eV (M = Rh) < 1.70 eV (Ir) < 1.72 eV (Ru). The PLQY increased dramatically in the order of 1.3% (M = Rh) < 13.5% (Ir) < 37% (Ru) (light red triangles in Fig. 7). The PL lifetime of RuAu12(dppm)6 at room temperature decreased from 5.7 μs under Ar to 0.22 μs under ambient conditions, indicating that phosphorescence is responsible for the PL. Interestingly, the PL mechanism of RuAu12(dppm)6 changed to fluorescence at low temperature (<130 K). This temperature dependence was attributed to an energy barrier between the S1 and T1 states, which could not be overcome at low temperatures due to the lack of thermal energy.90
3.3. Qualitative explanation of doping effects on the PL properties
Overall, Fig. 6 shows that single heterometal atom doping can tune the Eem values of the Au13 core over the range of 1.1–2.1 eV, a wider range than that achievable by surface modification (Fig. 3). In contrast, the Stokes shift is not affected by heterometal doping, as evidenced by an almost equal increase in both Eem and EHL. Fig. 7 illustrates that the PLQY can be dramatically enhanced to as high as >70% by single heterometal atom doping. We can clearly see in Fig. 7 that the correlation between the PLQY and EHL follows the energy gap law:102,103 the PLQY increases with increase in EHL. Practically, doping of smaller group element (lower valence element) such as Ir is very effective to enhance the PLQY. Previously, we qualitatively explained this trend by a two-step spherical jellium model,53 which assumes that the host (Au) and dopant atoms provide different background potentials for the confinement of valence electrons. The potential provided by the dopant with lower valency is shallower than that by the Au host element, and vice versa (Table 1 and Fig. 8). When the smaller group dopant is located at the center of the icosahedral Au13 core, the central portion of the jellium potential is upshifted (step ❶ in Fig. 8). According to this simple criterion, the 1P orbitals are destabilized by doping a smaller group element in the same period or a smaller period element in the same group with smaller electronegativity. If we assume that the 1D orbitals has larger population near the core center than the 1P orbitals,135 we can predict that the 1D orbitals are destabilized more than 1P orbitals when upshifting the central portion of the jellium potential by doping a smaller period element (step ❷ in Fig. 8). This qualitatively explains why the EHL value of Ir@Au12 MPC is obviously larger than that of Au13 MPC.
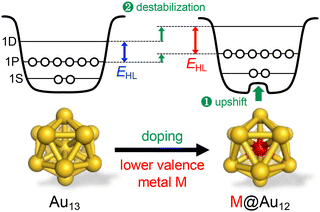 |
| Fig. 8 Two-step jellium potential for doping lower valent atoms to Au13. | |
4. Conclusion and perspective
In summary, we show that the PL emission energy of Au13 MPC can be varied in the range of 1.1–2.1 eV and PL quantum yields (PLQYs) as high as 70% can be realized by proper surface modification and heterometal doping. We can draw the following empirical rules to tune and improve the PL properties of Au13 MPC:
(1) The emission wavelength can be blue shifted by using L-type ligands (phosphines, N-heterocyclic carbenes) and/or doping smaller group element such as Ir, Pt, Ru, and Rh.
(2) The PLQY can be enhanced by using L-type ligands (phosphines, N-heterocyclic carbenes) and/or doping smaller group element such as Ir, Pt, Ru, and Rh.
(3) The PLQY can be enhanced by maximizing the interaction between the ligands via π⋯π or C–H⋯π interaction, chemical bonding, and steric packing.
(4) Circularly polarized luminescence (CPL) emerged by the structural deformation of the Au13 core by chiral ligands.
The first two rules are associated with the expansion of the energy gap between HOMO and LUMO and can be understood qualitatively with a simple scheme based on the jellium model (Fig. 5 and 8). However, a more sophisticated theoretical explanation is needed. The third rule is due to the suppression of non-radiative decay by rigidifying the Au core. When the proper interactions between ligands are at work, the independent ligands forming the protective layer act as if they were a single multidentate ligand, thereby limiting the freedom of movement of the Au13 core. When the Au13 core is in such a protective state, its vibrational frequency increases, reducing the density of vibrational states. As a result, its ability to act as a heat bath for radiation-free relaxation from electronically excited states is reduced. In order to enhance the brightness of CPL, not only PLQY, but also the dissymmetry factor should be increased by structural deformation by chiral ligands.
Given that the PL quantum yield is governed by the energy gap law,102,103 the obvious challenge is how to achieve high PL quantum yields in the long wavelength region. In particular, for bioimaging applications, there is a strong need for phosphors that emit efficiently in the NIR-I or NIR-II region, where the penetration into deep tissue is high. So far, Au13 clusters emitting in the NIR-II region have been limited to those protected by X-type ligands with very low PLQYs, as illustrated in the upper panel of Fig. 9.
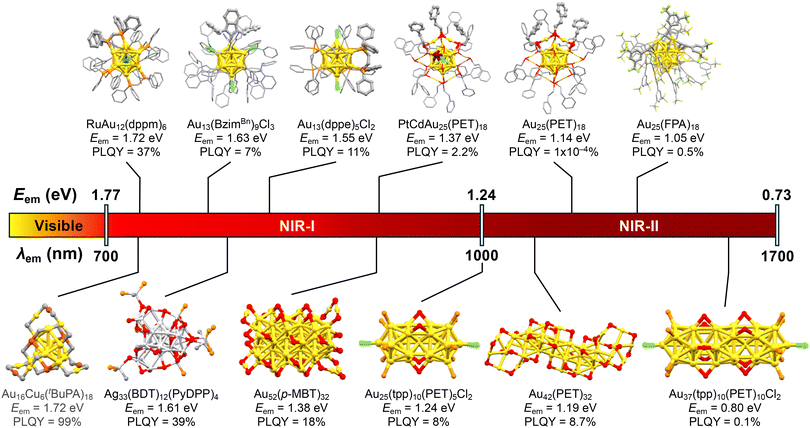 |
| Fig. 9 Examples of MPCs showing PL in the NIR I and II region. Color code: Au = yellow, Ag = silver, Ru = turquoise, Pt = light blue, Cd = purple, S = red, Cl = green, P = orange, N = blue, F = light green, C = dark gray. Ligand structures are omitted in the lower panel for simplicity. | |
However, the PL properties in the NIR-I or NIR-II region can be tuned and improved by controlling other structural parameters such as the nuclearity, morphology, and element of the metal core of MPCs. Actually, there are several examples of non-Au13 MPCs with superior PL properties in the NIR-I or NIR-II region, as listed in the lower panel of Fig. 9. [Au25(tpp)10(SCnH2n+1)5Cl2]2+ (n = 2–18) with a bi-icosahedral Au25 core136 had an Eem of 1.24 eV and PLQY of 0.1%.137 The PL of [Au25(tpp)10(SCnH2n+1)5Cl2]2+ was assigned to phosphorescence.138 [Au25(tpp)10(PET)5Cl2]+ had a PLQY of ∼8%,139 while [Au37(tpp)10(PET)10Cl2]+ with a tri-icosahedral Au37 core140 had an Eem and PLQY of 0.80 eV and 0.1%, respectively.139 Au42(PET)32 with a rod-like Au20 core with an aspect ratio of 6.2 also exhibited dual emission with Eem of 1.41 eV and 1.19 eV. These PLs correspond to fluorescence and phosphorescence with PLQYs of 3.2% and 8.7%, respectively.141 When this Au MPC was embedded in polystyrene films, the PLQY of phosphorescence increased to 20.3%, while the PLQY of fluorescence decreased to 1.1%. The PLQYs of Au52(SR)32 ranged from 4 to 18% depending on the steric demand of the ligands:142 Au52(p-MBT)32 (p-MBT: p-methylbenzenethiolate) had an Eem of 1.38 eV with a PLQY of 18%. Recently, Wang reported that Au16Cu6(tBuPA)18 (tBuPA = tBuPhC
C) exhibited remarkably bright PL in the NIR-I region.143 The Eem and PLQY were 1.72 eV and 95%, respectively, under ambient conditions. Impressively, the PLQY reached >99% under deaerated conditions. The PLQY of Au16Cu6(tBuPA)18 was significantly higher than that of undoped Au22(tBuPA)18 (9% under air), while the Eem value was comparable to that of undoped Au22(tBuPA)18 (Eem = 1.79 eV). The authors concluded that Cu doping slowed down the non-radiative decay by ∼60 times and accelerated the ISC process by ∼300 times. A NIR-emitting Ag MPC was reported by Nakashima.144 The modification of [Ag29(BDT)12(tpp)4]3− (BDT: 1,3-benzenedithiol) with Ag(I)-PyDPP complexes (PyDPP: pyridyl-diphenylphosphine) resulted in the formation of Ag33(BDT)12(PyDPP)4. This modification reduced the Eem from 1.82 to 1.61 eV and increased the PLQY from 1.4 to 39%. The authors proposed that the involvement of a triplet state is responsible for the intense PL. These examples suggest that bright PL in the NIR I and II regions can be realized by (1) increasing the Au core size, (2) doping more Ag and Cu into the Au core, and/or (3) making the morphology of Au MPCs anisotropic.
Data availability
No primary research results, software or code have been included and no new data were generated or analysed as part of this review.
Conflicts of interest
There are no conflicts to declare.
Acknowledgements
This work is affiliated with the Carbon to Metal Coating Institute at Queen's University and was partially funded by the NFRF-T program (#NFRFT-2020-00573). This research was supported financially by JST, CREST (grant no. JPMJCR20B2).
References
- R. L. Whetten, J. T. Khoury, M. M. Alvarez, S. Murthy, I. Vezmar, Z. L. Wang, P. W. Stephens, C. L. Cleveland, W. D. Luedtke and U. Landman, Nanocrystal Gold Molecules, Adv. Mater., 1996, 8, 428–433 CrossRef CAS.
- A. C. Templeton, W. P. Wuelfing and R. W. Murray, Monolayer-Protected Cluster Molecules, Acc. Chem. Res., 2000, 33, 27–36 CrossRef CAS PubMed.
- H. Qian, M. Zhu, Z. Wu and R. Jin, Quantum Sized Gold Nanoclusters with Atomic Precision, Acc. Chem. Res., 2012, 45, 1470–1479 CrossRef CAS PubMed.
- P. Maity, S. Xie, M. Yamauchi and T. Tsukuda, Stabilized Gold Clusters: From Isolation Toward Controlled Synthesis, Nanoscale, 2012, 4, 4027–4037 RSC.
- T. Tsukuda, Toward an Atomic-Level Understanding of Size-Specific Properties of Protected and Stabilized Gold Clusters, Bull. Chem. Soc. Jpn., 2012, 85, 151–168 CrossRef CAS.
-
T. Tsukuda and H. Häkkinen, Protected Metal Clusters: From Fundamentals to Applications, Elsevier, Amsterdam, 1st edn, 2015 Search PubMed.
- R. Jin, C. Zeng, M. Zhou and Y. Chen, Atomically Precise Colloidal Metal Nanoclusters and Nanoparticles: Fundamentals and Opportunities, Chem. Rev., 2016, 116, 10346–10413 CrossRef CAS PubMed.
- I. Chakraborty and T. Pradeep, Atomically Precise Clusters of Noble Metals: Emerging Link between Atoms and Nanoparticles, Chem. Rev., 2017, 117, 8208–8271 CrossRef CAS PubMed.
- T. Higaki, Q. Li, M. Zhou, S. Zhao, Y. Li, S. Li and R. Jin, Toward the Tailoring Chemistry of Metal Nanoclusters for Enhancing Functionalities, Acc. Chem. Res., 2018, 51, 2764–2773 CrossRef CAS PubMed.
- S. Li, N.-N. Li, X.-Y. Dong, S.-Q. Zang and T. C. W. Mak, Chemical Flexibility of Atomically Precise Metal Clusters, Chem. Rev., 2024, 124, 7262–7378 CrossRef CAS PubMed.
- S. Knoppe and T. Bürgi, Chirality in Thiolate-Protected Gold Clusters, Acc. Chem. Res., 2014, 47, 1318–1326 CrossRef CAS PubMed.
- N. Goswami, Q. Yao, Z. Luo, J. Li, T. Chen and J. Xie, Luminescent Metal Nanoclusters with Aggregation-Induced Emission, J. Phys. Chem. Lett., 2016, 7, 962–975 CrossRef CAS PubMed.
- A. Cantelli, G. Guidetti, J. Manzi, V. Caponetti and M. Montalti, Towards Ultra–Bright Gold Nanoclusters, Eur. J. Inorg. Chem., 2017, 5068–5084 CrossRef CAS.
- C. M. Aikens, Electronic and Geometric Structure, Optical Properties, and Excited State Behavior in Atomically Precise Thiolate-Stabilized Noble Metal Nanoclusters, Acc. Chem. Res., 2018, 51, 3065–3073 CrossRef CAS PubMed.
- X. Kang and M. Zhu, Tailoring the Photoluminescence of Atomically Precise Nanoclusters, Chem. Soc. Rev., 2019, 48, 2422–2457 RSC.
- H. Yu, B. Rao, W. Jiang, S. Yang and M. Zhu, The Photoluminescent Metal Nanoclusters with Atomic Precision, Coord. Chem. Rev., 2019, 378, 595–617 CrossRef CAS.
- Y. Zhu, J. Guo, X. Qiu, S. Zhao and Z. Tang, Optical Activity of Chiral Metal Nanoclusters, Acc. Mater. Res., 2021, 2, 21–35 CrossRef CAS.
- S. Qian, Z. Wang, Z. Zuo, X. Wang, Q. Wang and X. Yuan, Engineering Luminescent Metal Nanoclusters for Sensing Applications, Coord. Chem. Rev., 2022, 451, 214268 CrossRef CAS.
- J. Kong, W. Zhang, Y. Wu and M. Zhou, Optical Properties of Gold Nanoclusters Constructed from Au13 Units, Aggregate, 2022, 3, e207 CrossRef CAS.
- D. Cheng, R. Liu and K. Hu, Gold Nanoclusters: Photophysical Properties and Photocatalytic
Applications, Front. Chem., 2022, 10, 958626 CrossRef CAS PubMed.
- K. Bharti, J. K. Sahu and K. K. Sadhu, Origin of Luminescence Properties and Synthetic Methods for Gold- and Bimetallic Gold-Based Nanomaterials, Mater. Adv., 2022, 3, 5698–5724 RSC.
- Z. Liu, L. Luo and R. Jin, Visible to NIR–II Photoluminescence of Atomically Precise Gold Nanoclusters, Adv. Mater., 2023, 2309073 Search PubMed.
- G. Li and R. Jin, Atomically Precise Gold Nanoclusters as New Model Catalysts, Acc. Chem. Res., 2013, 46, 1749–1758 CrossRef CAS PubMed.
- Q. Tang, G. Hu, V. Fung and D.-E. Jiang, Insights into Interfaces, Stability, Electronic Properties, and Catalytic Activities of Atomically Precise Metal Nanoclusters from First Principles, Acc. Chem. Res., 2018, 51, 2793–2802 CrossRef CAS.
- J. Yan, B. K. Teo and N. Zheng, Surface Chemistry of Atomically Precise Coinage–Metal Nanoclusters: From Structural Control to Surface Reactivity and Catalysis, Acc. Chem. Res., 2018, 51, 3084–3093 CrossRef CAS.
- K. Kwak and D. Lee, Electrochemistry of Atomically Precise Metal Nanoclusters, Acc. Chem. Res., 2018, 52, 12–22 CrossRef PubMed.
- Y. Du, H. Sheng, D. Astruc and M. Zhu, Atomically Precise Noble Metal Nanoclusters as Efficient Catalysts: A Bridge between Structure and Properties, Chem. Rev., 2020, 120, 526–622 CrossRef CAS PubMed.
- R. Jin, G. Li, S. Sharma, Y. Li and X. Du, Toward Active-Site Tailoring in Heterogeneous Catalysis by Atomically Precise Metal Nanoclusters with Crystallographic Structures, Chem. Rev., 2021, 121, 567–648 CrossRef CAS PubMed.
- H. Seong and D. Lee, Atomically Precise Metal Nanoclusters for Energy Conversion, Bull. Korean Chem. Soc., 2024, 45, 435–450 CrossRef CAS.
- S. Link, A. Beeby, S. FitzGerald, M. A. El-Sayed, T. G. Schaaff and R. L. Whetten, Visible to Infrared Luminescence from a 28-Atom Gold Cluster, J. Phys. Chem. B, 2002, 106, 3410–3415 CrossRef CAS.
- A. Mooradian, Photoluminescence of Metals, Phys. Rev. Lett., 1969, 22, 185–187 CrossRef CAS.
- Y. Tao, M. Li, J. Ren and X. Qu, Metal Nanoclusters: Novel Probes for Diagnostic and Therapeutic Applications, Chem. Soc. Rev., 2015, 44, 8636–8663 RSC.
- H. Cui, Z.-S. Shao, Z. Song, Y.-B. Wang and H.-S. Wang, Development of Gold Nanoclusters: From Preparation to Applications in the Field of Biomedicine, J. Mater. Chem. C, 2020, 8, 14312–14333 RSC.
- H. Li, H. Li and A. Wan, Luminescent Gold Nanoclusters for In Vivo Tumor Imaging, Analyst, 2020, 145, 348–363 RSC.
- J. Tang, H. Shi, G. Ma, L. Luo and Z. Tang, Ultrasmall Au and Ag Nanoclusters for Biomedical Applications: A Review, Front. Bioeng. Biotechnol., 2020, 8, 1019 CrossRef PubMed.
- S. Zhu, X. Wang, Y. Cong and L. Li, Regulating the Optical Properties of Gold Nanoclusters for Biological Applications, ACS Omega, 2020, 5, 22702–22707 CrossRef CAS PubMed.
- S. M. van de Looij, E. R. Hebels, M. Viola, M. Hembury, S. Oliveira and T. Vermonden, Gold Nanoclusters: Imaging, Therapy, and Theranostic Roles in Biomedical Applications, Bioconjugate Chem., 2022, 33, 4–23 CrossRef CAS PubMed.
- C. Zhang, X. Gao, W. Chen, M. He, Y. Yu, G. Gao and T. Sun, Advances of Gold Nanoclusters for Bioimaging, iScience, 2022, 25, 105022 CrossRef CAS.
- X. He, S. Liu, X. Hu, X. Huang, H. Zhang and X. Mao, Precious Metal Clusters as Fundamental Agents in Bioimaging Usability, Front. Chem., 2023, 11, 1296036 CrossRef CAS PubMed.
- D. Mordini, A. Mavridi-Printezi, A. Menichetti, A. Cantelli, X. Li and M. Montalti, Luminescent Gold Nanoclusters for Bioimaging: Increasing the Ligand Complexity, Nanomaterials, 2023, 13, 648 CrossRef CAS.
- S. Ni, Y. Liu, S. Tong, S. Li and X. Song, Emerging NIR-II Luminescent Gold Nanoclusters for in vivo Bioimaging, J. Anal. Test., 2023, 7, 260–271 CrossRef.
- A. Cifuentes-Rius, V. G. Deepagan, J. Xie and N. H. Voelcker, Bright Future of Gold Nanoclusters in Theranostics, ACS Appl. Mater. Interfaces, 2021, 13, 49581–49588 CrossRef CAS PubMed.
- K. Sood and A. Shanavas, The Role of Gold Nanoclusters as Emerging Theranostic Agents for Cancer Management, Curr. Pathobiol. Rep., 2021, 9, 33–42 CrossRef CAS.
- H. Liu, L. Wang, Z. Xue and X. D. Zhang, Atomic Precise Gold Nanoclusters: Toward the Customize Synthesis, Precision Medicine, Part. Part. Syst. Charact., 2023, 40, 2300084 CrossRef CAS.
- H. Häkkinen, Atomic and Electronic Structure of Gold Clusters: Understanding Flakes, Cages and Superatoms from Simple Concepts, Chem. Soc. Rev., 2008, 37, 1847–1859 RSC.
- M. Walter, J. Akola, O. Lopez-Acevedo, P. D. Jadzinsky, G. Calero, C. J. Ackerson, R. L. Whetten, H. Grönbeck and H. Häkkinen, A Unified View of Ligand-Protected Gold Clusters as Superatom Complexes, Proc. Natl. Acad. Sci. U. S. A., 2008, 105, 9157–9162 CrossRef CAS PubMed.
- Z. Luo and A. W. Castleman, Special and General Superatoms, Acc. Chem. Res., 2014, 47, 2931–2940 CrossRef CAS PubMed.
- J.-I. Nishigaki, K. Koyasu and T. Tsukuda, Chemically Modified Gold Superatoms and Superatomic Molecules, Chem. Rec., 2014, 14, 897–909 CrossRef CAS PubMed.
- P. Jena and Q. Sun, Super Atomic Clusters: Design Rules and Potential for Building Blocks of Materials, Chem. Rev., 2018, 118, 5755–5870 CrossRef CAS PubMed.
- A. Muñoz-Castro, On the Ligand-Core Interaction in Ligand-Protected Gold Superatoms. Insights from Au25(XR)18 (X = S, Se, Te) via Relativistic DFT Calculations, Phys. Chem. Chem. Phys., 2019, 21, 13022–13029 RSC.
- H. Hirai, S. Ito, S. Takano, K. Koyasu and T. Tsukuda, Ligand-Protected Gold/Silver Superatoms: Current Status and Emerging Trends, Chem. Sci., 2020, 11, 12233–12248 RSC.
- T. Omoda, S. Takano and T. Tsukuda, Toward Controlling the Electronic Structures of Chemically Modified Superatoms of Gold and Silver, Small, 2021, 17, 2001439 CrossRef CAS PubMed.
- S. Takano and T. Tsukuda, Chemically Modified Gold/Silver Superatoms as Artificial Elements at Nanoscale: Design Principles and Synthesis Challenges, J. Am. Chem. Soc., 2021, 143, 1683–1698 CrossRef CAS PubMed.
- R. Jin, Quantum Sized, Thiolate-Protected Gold Nanoclusters, Nanoscale, 2010, 2, 343–362 RSC.
- W. Kurashige, Y. Niihori, S. Sharma and Y. Negishi, Precise Synthesis, Functionalization and Application of Thiolate-Protected Gold Clusters, Coord. Chem. Rev., 2016, 320–321, 238–250 CrossRef CAS.
- X. Kang, H. Chong and M. Zhu, Au25(SR)18: The Captain of the Great Nanocluster Ship, Nanoscale, 2018, 10, 10758–10834 RSC.
- Q. Yao, T. Chen, X. Yuan and J. Xie, Toward Total Synthesis of Thiolate-Protected Metal Nanoclusters, Acc. Chem. Res., 2018, 51, 1338–1348 CrossRef CAS PubMed.
- N. A. Sakthivel and A. Dass, Aromatic Thiolate-Protected Series of Gold Nanomolecules and a Contrary Structural Trend in Size Evolution, Acc. Chem. Res., 2018, 51, 1774–1783 CrossRef CAS PubMed.
- Z. Lei, X.-K. Wan, S.-F. Yuan, Z.-J. Guan and Q.-M. Wang, Alkynyl Approach toward the Protection of Metal Nanoclusters, Acc. Chem. Res., 2018, 51, 2465–2474 CrossRef CAS PubMed.
- M.-M. Zhang, X.-Y. Dong, Y.-J. Wang, S.-Q. Zang and T. C. Mak, Recent Progress in Functional Atom-Precise Coinage Metal Clusters Protected by Alkynyl Ligands, Coord. Chem. Rev., 2022, 453, 214315 CrossRef CAS.
-
K. Konishi, in Gold Clusters, Colloids and Nanoparticles I, ed. D. M. P. Mingos, Springer International Publishing, Cham, 2014, pp. 49–86 Search PubMed.
- R. H. Adnan, J. M. L. Madridejos, A. S. Alotabi, G. F. Metha and G. G. Andersson, A Review of State of the Art in Phosphine Ligated Gold Clusters and Application in Catalysis, Adv. Sci., 2022, 9, 2105692 CrossRef CAS PubMed.
- H. Shen, G. Tian, Z. Xu, L. Wang, Q. Wu, Y. Zhang, B. K. Teo and N. Zheng, N-heterocyclic Carbene Coordinated Metal Nanoparticles and Nanoclusters, Coord. Chem. Rev., 2022, 458, 214425 CrossRef CAS.
- E. L. Albright, T. I. Levchenko, V. K. Kulkarni, A. I. Sullivan, J. F. DeJesus, S. Malola, S. Takano, M. Nambo, K. Stamplecoskie, H. Häkkinen, T. Tsukuda and C. M. Crudden, N-Heterocyclic Carbene-Stabilized Atomically Precise Metal Nanoclusters, J. Am. Chem. Soc., 2024, 146, 5759–5780 CrossRef CAS PubMed.
- R. Jin and K. Nobusada, Doping and Alloying in Atomically Precise Gold Nanoparticles, Nano Res., 2014, 7, 285–300 CrossRef CAS.
- Z. Gan, N. Xia and Z. Wu, Discovery, Mechanism, and Application of Antigalvanic Reaction, Acc. Chem. Res., 2018, 51, 2774–2783 CrossRef CAS PubMed.
- S. Wang, Q. Li, X. Kang and M. Zhu, Customizing the Structure, Composition, and Properties of Alloy Nanoclusters by Metal Exchange, Acc. Chem. Res., 2018, 51, 2784–2792 CrossRef CAS PubMed.
- A. Ghosh, O. F. Mohammed and O. M. Bakr, Atomic-Level Doping of Metal Clusters, Acc. Chem. Res., 2018, 51, 3094–3103 CrossRef CAS PubMed.
- S. Hossain, Y. Niihori, L. V. Nair, B. Kumar, W. Kurashige and Y. Negishi, Alloy Clusters: Precise Synthesis and Mixing Effects, Acc. Chem. Res., 2018, 51, 3114–3124 CrossRef CAS PubMed.
- X. Kang, Y. Li, M. Zhu and R. Jin, Atomically Precise Alloy Nanoclusters: Syntheses, Structures, and Properties, Chem. Soc. Rev., 2020, 49, 6443–6514 RSC.
- T. Kawawaki, Y. Imai, D. Suzuki, S. Kato, I. Kobayashi, T. Suzuki, R. Kaneko, S. Hossain and Y. Negishi, Atomically Precise Alloy Nanoclusters, Chem. – Eur. J., 2020, 26, 16150–16193 CrossRef CAS PubMed.
- M. W. Heaven, A. Dass, P. S. White, K. M. Holt and R. W. Murray, Crystal Structure of the Gold Nanoparticle [N(C8H17)4][Au25(SCH2CH2Ph)18], J. Am. Chem. Soc., 2008, 130, 3754–3755 CrossRef CAS PubMed.
- M. Zhu, C. M. Aikens, F. J. Hollander, G. C. Schatz and R. Jin, Correlating the Crystal Structure of a Thiol-Protected Au25 Cluster and Optical Properties, J. Am. Chem. Soc., 2008, 130, 5883–5885 CrossRef CAS PubMed.
- J.-J. Li, Z.-J. Guan, Z. Lei, F. Hu and Q.-M. Wang, Same Magic Number but Different Arrangement: Alkynyl-Protected Au25 with D3 Symmetry, Angew. Chem., Int. Ed., 2019, 58, 1083–1087 CrossRef CAS PubMed.
- Y. Shichibu and K. Konishi, HCl–Induced Nuclearity Convergence in Diphosphine–Protected Ultrasmall Gold Clusters: A Novel Synthetic Route to “Magic–Number” Au13 Clusters, Small, 2010, 6, 1216–1220 CrossRef CAS PubMed.
- M. R. Narouz, S. Takano, P. A. Lummis, T. I. Levchenko, A. Nazemi, S. Kaappa, S. Malola, G. Yousefalizadeh, L. A. Calhoun, K. G. Stamplecoskie, H. Häkkinen, T. Tsukuda and C. M. Crudden, Robust, Highly Luminescent Au13 Superatoms Protected by N-Heterocyclic Carbenes, J. Am. Chem. Soc., 2019, 141, 14997–15002 CrossRef CAS PubMed.
- H. Shen, S. Xiang, Z. Xu, C. Liu, X. Li, C. Sun, S. Lin, B. K. Teo and N. Zheng, Superatomic Au13 Clusters Ligated by Different N-Heterocyclic Carbenes and their Ligand-Dependent Catalysis, Photoluminescence, and Proton Sensitivity, Nano Res., 2020, 13, 1908–1911 CrossRef CAS.
- H. Yi, K. M. Osten, T. I. Levchenko, A. J. Veinot, Y. Aramaki, T. Ooi, M. Nambo and C. M. Crudden, Synthesis and Enantioseparation of Chiral Au13 Nanoclusters Protected by bis-N-Heterocyclic Carbene Ligands, Chem. Sci., 2021, 12, 10436–10440 RSC.
- M. Laupp and J. Strähle, [(Ph3PAu)6(dppeAu2)(AuCl)4Pd], an Icosahedral Au12 Cluster with a Central Pd Atom, Angew. Chem., Int. Ed., 1994, 33, 207–209 CrossRef.
- S. L. Christensen, M. A. MacDonald, A. Chatt, P. Zhang, H. Qian and R. Jin, Dopant Locaton, Local Structure, and Electronic Properties of Au24Pt(SR)18 Nanoclusters, J. Phys. Chem. C, 2012, 116, 26932–26937 CrossRef CAS.
- C. Kumara, C. M. Aikens and A. Dass, X-ray Crystal Structure and, Theoretical Analysis of Au25−xAgx(SCH2CH2Ph)18− Alloy, J. Phys. Chem. Lett., 2014, 5, 461–466 CrossRef CAS PubMed.
- S. Yamazoe, W. Kurashige, K. Nobusada, Y. Negishi and T. Tsukuda, Preferential Location of Coinage Metal Dopants (M = Ag or Cu) in [Au25-xMx(SC2H4Ph)18]− (x∼1) as Determined by Extended X-ray Absorption Fine Structure and Density Functional Theory Calculations, J. Phys. Chem. C, 2014, 118, 25284–25290 CrossRef CAS.
- M. A. Tofanelli, T. W. Ni, B. D. Phillips and C. J. Ackerson, Crystal Structure of the PdAu24(SR)180 Superatom, Inorg. Chem., 2016, 55, 999–1001 CrossRef CAS PubMed.
- S. Tian, L. Liao, J. Yuan, C. Yao, J. Chen, J. Yang and Z. Wu, Structures and Magnetism of Mono-Pallidium and Mono-Platinum Doped Au25(PET)18 Nanoclusters, Chem. Commun., 2016, 52, 9873–9876 RSC.
- S. Sharma, S. Yamazoe, T. Ono, W. Kurashige, Y. Niihori, K. Nobusada, T. Tsukuda and Y. Negishi, Tuning the Electronic Structure of Thiolate-Protected 25-Atom Clusters by Co-Substitution with Metals Having Different Preferential Site, Dalton Trans., 2016, 45, 18064–18068 RSC.
- S. Takano, S. Ito and T. Tsukuda, Efficient and Selective Conversion of Phosphine-Protected (MAu8)2+ (M = Pd, Pt) Superatoms to Thiolate-Protected (MAu12)6+ or Alkynyl-Protected (MAu12)4+ Superatoms via Hydride Doping, J. Am. Chem. Soc., 2019, 141, 15994–16002 CrossRef CAS PubMed.
- W. Fei, S. Antonello, T. Dainese, A. Dolmella, M. Lahtinen, K. Rissanen, A. Venzo and F. Maran, Metal Doping of Au25(SR)18− Clusters: Insights and Hindsights, J. Am. Chem. Soc., 2019, 141, 16033–16045 CrossRef CAS PubMed.
- M. Suyama, S. Takano and T. Tsukuda, Synergistic Effects of Pt and Cd Codoping to Icosahedral Au13 Superatoms, J. Phys. Chem. C, 2020, 124, 23923–23929 CrossRef CAS.
- H. Hirai, S. Takano, T. Nakamura and T. Tsukuda, Understanding Doping Effects on Electronic Structures of Gold Superatoms: A Case Study of Diphosphine-Protected M@Au12 (M = Au, Pt, Ir), Inorg. Chem., 2020, 59, 17889–17895 CrossRef CAS PubMed.
- S. Takano, H. Hirai, T. Nakashima, T. Iwasa, T. Taketsugu and T. Tsukuda, Photoluminescence of Doped Superatoms M@Au12 (M = Ru, Rh, Ir) Homoleptically Capped by (Ph2)PCH2P(Ph2): Efficient Room-Temperature Phosphorescence from Ru@Au12, J. Am. Chem. Soc., 2021, 143, 10560–10564 CrossRef CAS PubMed.
- H. Hirai, S. Takano, T. Nakashima, T. Iwasa, T. Taketsugu and T. Tsukuda, Doping-Mediated Energy-Level Engineering of M@Au12 Superatoms (M = Pd, Pt, Rh, Ir) for Efficient Photoluminescence and Photocatalysis, Angew. Chem., Int. Ed., 2022, 61, e202207290 CrossRef CAS PubMed.
- S. Takano, E. Ito, T. Nakamura and T. Tsukuda, Effect of Group-10 Element M (Ni, Pd, Pt) on Electronic Structure of Icosahedral M@Au12 Cores of MAu24L18 (L = Alkynyl, Thiolate), J. Phys. Chem. C, 2023, 127, 4360–4366 CrossRef CAS.
- G. Yousefalizadeh and K. G. Stamplecoskie, A Single Model for the Excited-State Dynamics of Au18(SR)14 and Au25(SR)18 Clusters, J. Phys. Chem. A, 2018, 122, 7014–7022 CrossRef CAS PubMed.
- M. Zhou and Y. Song, Origins of Visible and Near-Infrared Emissions in [Au25(SR)18]− Nanoclusters, J. Phys. Chem. Lett., 2021, 12, 1514–1519 CrossRef CAS PubMed.
- K. Yoshida, D. Arima and M. Mitsui, Dissecting the Triplet-State Properties and Intersystem Crossing Mechanism of the Ligand-Protected Au13 Superatom, J. Phys. Chem. Lett., 2023, 14, 10967–10973 CrossRef CAS PubMed.
- H. Kawasaki, S. Kumar, G. Li, C. Zeng, D. R. Kauffman, J. Yoshimoto, Y. Iwasaki and R. Jin, Genration of Singlet Oxygen by Photoexcited Au25(SR)18 Clusters, Chem. Mater., 2014, 26, 2777–2788 CrossRef CAS.
- S. Wang, L. Tang, B. Cai, Z. Yin, Y. Li, L. Xiong, X. Kang, J. Xuan, Y. Pei and M. Zhu, Ligand Modification of Au25 Nanoclusters for Near-Infrared Photocatalytic Oxydative Functionalization, J. Am. Chem. Soc., 2022, 144, 3787–3792 CrossRef CAS PubMed.
- K. Isozaki, K. Iseri, R. Saito, K. Ueda and M. Nakamura, Dual Catalysis of Gold Nanoclusters: Photocatalytic Cross–Dehydrogenative Coupling by Cooperation of Superatomic Core and Molecularly Modified Staples, Angew. Chem., Int. Ed., 2024, 63, e202312135 CrossRef CAS PubMed.
- Y. Niihori, T. Kosaka and Y. Negishi, Triplet–Triplet Annihilation-Based Photon Upconversion Using Nanoparticles and Nanoclusters, Mater. Horiz., 2024, 11, 2304–2322 RSC.
- H. Hirai, S. Takano, S. Masuda and T. Tsukuda, Introducing Iodide Ligands on IrAu12 Cluster Enhances Phosphorescence Efficiency and Photoredox Activity, ChemElectroChem, 2024, 11, e202300669 CrossRef CAS.
- R. Saito, K. Isozaki, Y. Mizuhara and M. Nakamura, Synthesis of N2-type Superatomic Molecules, J. Am. Chem. Soc., 2024, 146, 20930–20936 CrossRef CAS PubMed.
- R. Englman and J. Jortner, The Energy Gap Law for Non-Radiative Decay in Large Molecules, J. Lumin., 1970, 1, 134–142 CrossRef.
- S. J. Jang, A Simple Generalization of the Energy Gap Law for Nonradiative Processes, J. Chem. Phys., 2021, 155, 164106 CrossRef CAS PubMed.
- Y. Negishi, K. Nobusada and T. Tsukuda, Glutathione-Protected Gold Clusters Revisited: Bridging the Gap between Gold(I)-Thiolate Complexes and Thiolate-Protected Gold Nanocrystals, J. Am. Chem. Soc., 2005, 127, 5261–5270 CrossRef CAS PubMed.
- J. F. Parker, C. A. Fields-Zinna and R. W. Murray, The Story of a Monodisperse Gold Nanoparticle: Au25L18, Acc. Chem. Res., 2010, 43, 1289–1296 CrossRef CAS PubMed.
- Z. Wu and R. Jin, On the Ligand's Role in the Fluorescence of Gold Nanoclusters, Nano Lett., 2010, 10, 2568–2573 CrossRef CAS PubMed.
- J. Akola, M. Walter, R. L. Whetten, H. Häkkinen and H. Grönbeck, On the Structure of Thiolate-Protected Au25, J. Am. Chem. Soc., 2008, 130, 3756–3757 CrossRef CAS PubMed.
- Z. Liu, Y. Li, E. Kahng, S. Xue, X. Du, S. Li and R. Jin, Tailoring the Electron–Phonon Interaction in Au25(SR)18 Nanoclusters via Ligand Engineering and Insight into Luminescence, ACS Nano, 2022, 16, 18448–18458 CrossRef CAS PubMed.
- K. L. D. M. Weerawardene and C. M. Aikens, Theoretical Insights into the Origin of Photoluminescence of Au25(SR)18− Nanoparticles, J. Am. Chem. Soc., 2016, 138, 11202–11210 CrossRef CAS PubMed.
- S. Antonello, T. Dainese, M. De Nardi, L. Perotti and F. Maran, Insights into the Interface Between the Electrolytic Solution and The Gold Core in Molecular Au25 Clusters, ChemElectroChem, 2016, 3, 1237–1244 CrossRef CAS.
- Y. Negishi, T. Iwai and M. Ide, Continuous Modulation of Electronic Structure of Stable Thiolate-Protected Au25 Cluster by Ag doping, Chem. Commun., 2010, 46, 4713–4715 RSC.
- Y. Song, J. Zhong, S. Yang, S. Wang, T. Cao, J. Zhang, P. Li, D. Hu, Y. Pei and M. Zhu, Crystal Structure of Au25(SePh)18 Nanoclusters and Insights into their Electronic, Optical and Catalytic Properties, Nanoscale, 2014, 6, 13977–13985 RSC.
- C. E. Briant, B. R. C. Theobald, J. W. White, L. K. Bell, D. M. P. Mingos and A. J. Welch, Synthesis and X-ray Structural Characterization of the Centred Icosahedral Gold Cluster Compound [Au13(PMe2Ph)10Cl2](PF6)3; the Realization of a Theoretical Prediction, J. Chem. Soc., Chem. Commun., 1981, 201–202 RSC.
- R. C. B. Copley and D. M. P. Mingos, Synthesis and Characterization of the Centred Icosahedral Cluster Series [Au9MIB4Cl4(PMePh2)8][C2B9H12], where MIB = Au, Ag or Cu, J. Chem. Soc., Dalton Trans., 1996, 491–500 RSC.
- Y. Shichibu, K. Suzuki and K. Konishi, Facile Synthesis and Optical Properties of Magic-Number Au13 Clusters, Nanoscale, 2012, 4, 4125–4129 RSC.
- M. Sugiuchi, Y. Shichibu, T. Nakanishi, Y. Hasegawa and K. Konishi, Cluster–π Electronic Interaction in a Superatomic Au13 Cluster Bearing σ-bonded Acetylide Ligands, Chem. Commun., 2015, 51, 13519–13522 RSC.
- Z.-H. Gao, J. Dong, Q.-F. Zhang and L.-S. Wang, Halogen Effects on the Electronic and Optical Properties of Au13 Nanoclusters, Nanoscale Adv., 2020, 2, 4902–4907 RSC.
- N. Kito, S. Takano, S. Masuda, K. Harano and T. Tsukuda, Au13 Superatom Bearing Two Terpyridines at Coaxial Positions: Photoluminescence Quenching via Complexation with 3d Metal Ions, Bull. Chem. Soc. Jpn., 2023, 96, 1045–1051 CrossRef CAS.
- Y. Yang, Q. Zhang, Z.-J. Guan, Z.-A. Nan, J.-Q. Wang, T. Jia and W.-W. Zhan, Enantioselective Synthesis of Homochiral Au13 Nanoclusters and Their Chiroptical Activities, Inorg. Chem., 2019, 58, 3670–3675 CrossRef CAS PubMed.
- Y. Shichibu, Y. Ogawa, M. Sugiuchi and K. Konishi, Chiroptical Activity of Au13 Clusters: Experimental and Theoretical Understanding of the Origin of Helical Charge Movements, Nanoscale Adv., 2021, 3, 1005–1011 RSC.
- J.-H. Yu, Z.-R. Yuan, J. Xu, J.-G. Wang, M. Azam, T.-D. Li, Y.-Z. Li and D. Sun, Monoarsine-protected Icosahedral Cluster [Au13(AsPh3)8Cl4]+: Comparative Studies on Ligand Effect and Surface Reactivity with its Stibine Analogue, Chem. Sci., 2023, 14, 6564–6571 RSC.
- Y. Shichibu, F. Zhang, Y. Chen, M. Konishi, S. Tanaka, H. Imoto, K. Naka and K. Konishi, Diarsine- vs Diphosphine-protected Au13 clusters: Effect of Subtle Geometric Differences on Optical Property and Electronic Structure, J. Chem. Phys., 2021, 155, 054301 CrossRef CAS PubMed.
- Y.-Z. Li, R. Ganguly, K. Y. Hong, Y. Li, M. E. Tessensohn, R. Webster and W. K. Leong, Stibine-Protected Au13 Nanoclusters: Syntheses, Properties and Facile Conversion to GSH-protected Au25 Nanocluster, Chem. Sci., 2018, 9, 8723–8730 RSC.
- M. R. Narouz, K. M. Osten, P.
J. Unsworth, R. W. Y. Man, K. Salorinne, S. Takano, R. Tomihara, S. Kaappa, S. Malola, C.-T. Dinh, J. D. Padmos, K. Ayoo, P. J. Garrett, M. Nambo, J. H. Horton, E. H. Sargent, H. Häkkinen, T. Tsukuda and C. M. Crudden,
N-Heterocyclic, Carbene-Functionalized Magic Number Gold Nanoclusters, Nat. Chem., 2019, 11, 419–425 CrossRef CAS PubMed.
- P. Luo, X.-J. Zhai, S. Bai, Y.-B. Si, X.-Y. Dong, Y.-F. Han and S.-Q. Zang, Highly Efficient Circularly Polarized Luminescence from Chiral Au13 Clusters Stabilized by Enantiopure Monodentate NHC Ligands, Angew. Chem., Int. Ed., 2023, 62, e202219017 CrossRef CAS PubMed.
- X. Wang, R. Liu, L. Tian, J. Bao, C. Zhao, F. Niu, D. Cheng, Z. Lu and K. Hu, Highly Luminescent NHC-Stabilized Au13 Clusters as Efficient Excited-State Electron Donors, J. Phys. Chem. C, 2022, 126, 18374–18382 CrossRef CAS.
- M. Bevilacqua, M. Roverso, S. Bogialli, C. Graiff and A. Biffis, From Au11 to Au13: Tailored Synthesis of Superatomic Di-NHC/PPh3-Stabilized Molecular Gold Nanoclusters, Inorg. Chem., 2023, 62, 1383–1393 CrossRef CAS PubMed.
- P. Luo, S. Bai, X. Wang, J. Zhao, Z. N. Yan, Y. F. Han, S. Q. Zang and T. C. Mak, Tuning the Magic Sizes and Optical Properties of Atomically Precise Bidentate N–Heterocyclic Carbene–Protected Gold Nanoclusters via Subtle Change of N–Substituents, Adv. Opt. Mater., 2021, 9, 2001936 CrossRef CAS.
- E. L. Albright, S. Malola, S. I. Jacob, H. Yi, S. Takano, K. Mimura, T. Tsukuda, H. Häkkinen, M. Nambo and C. M. Crudden, Enantiopure Chiral Au13 Nanoclusters Stabilized by Ditopic N-Heterocyclic Carbenes: Synthesis, Characterization, and Electrocatalytic Reduction of CO2, Chem. Mater., 2024, 36, 1279–1289 CrossRef CAS.
- Y. Fukumoto, T. Omoda, H. Hirai, S. Takano, K. Harano and T. Tsukuda, Diphosphine-Protected IrAu12 Superatom with Open Site(s): Synthesis and Programmed Stepwise Assembly, Angew. Chem., Int. Ed., 2024, 63, e202402025 CrossRef PubMed.
- M. Suyama, S. Takano, T. Nakamura and T. Tsukuda, Stoichiometric Formation of Open-Shell [PtAu24(SC2H4Ph)18]− via Spontaneous Electron Proportionation between [PtAu24(SC2H4Ph)18]2− and [PtAu24(SC2H4Ph)18]0, J. Am. Chem. Soc., 2019, 141, 14048–14051 CrossRef CAS PubMed.
- D. R. Kauffman, D. Alfonso, C. Matranga, H. Qian and R. Jin, A Quantum Alloy: The Ligand-Protected Au25−xAgx(SR)18 Cluster, J. Phys. Chem. C, 2013, 117, 7914–7923 CrossRef CAS.
- H. Hirai, T. Nakashima, S. Takano, Y. Shichibu, K. Konishi, T. Kawai and T. Tsukuda, IrAu12 Superatom Modified by Chiral Diphosphines: Doping-induced Enhancement of Chiroptical Activity, J. Mater. Chem. C, 2023, 11, 3095–3100 RSC.
- S.-S. Zhang, L. Feng, R. D. Senanayake, C. M. Aikens, X.-P. Wang, Q.-Q. Zhao, C.-H. Tung and D. Sun, Diphosphine-protected Ultrasmall Gold Nanoclusters: Opened Icosahedral Au13 and Heart-shaped Au8 clusters, Chem. Sci., 2018, 9, 1251–1258 RSC.
- J. T. A. Gilmour and N. Gaston, 5-Fold Symmetry in Superatomic Scandium Clusters: Exploiting Favourable Orbital Overlap to Sequester Spin, Phys. Chem. Chem. Phys., 2020, 22, 4051–4058 RSC.
- Y. Shichibu, Y. Negishi, T. Watanabe, N. K. Chaki, H. Kawaguchi and T. Tsukuda, Biicosahedral Gold Clusters [Au25(PPh3)10(SCnH2n+1)5Cl2]2+ (n = 2–18): A Stepping Stone to Cluster-Assembled Materials, J. Phys. Chem. C, 2007, 111, 7845–7847 CrossRef CAS.
- S. Park and D. Lee, Synthesis and Electrochemical and Spectroscopic Characterization of Biicosahedral Au25 Clusters, Langmuir, 2012, 28, 7049–7054 CrossRef CAS PubMed.
- M. Mitsui, Y. Wada, R. Kishii, D. Arima and Y. Niihori, Evidence for Triplet-State-Dominated Luminescence in Biicosahedral Superatomic Molecular Au25 Clusters, Nanoscale, 2022, 14, 7974–7979 RSC.
- Q. Li, C. J. Zeman IV, Z. Ma, G. C. Schatz and X. W. Gu, Bright NIR-II Photoluminescence in Rod-Shaped Icosahedral Gold Nanoclusters, Small, 2021, 17, 2007992 CrossRef CAS PubMed.
- R. Jin, C. Liu, S. Zhao, A. Das, H. Xing, C. Gayathri, Y. Xing, N. L. Rosi, R. R. Gil and R. Jin, Tri-icosahedral Gold Nanocluster [Au37(PPh3)10(SC2H4Ph)10X2]+: Linear Assembly of Icosahedral Building Blocks, ACS Nano, 2015, 9, 8530–8536 CrossRef CAS PubMed.
- L. Luo, Z. Liu, X. Du and R. Jin, Near-Infrared Dual Emission from the Au42(SR)32 Nanocluster and Tailoring of Intersystem Crossing, J. Am. Chem. Soc., 2022, 144, 19243–19247 CrossRef CAS PubMed.
- Y. Wang, Z. Liu, A. Mazumder, C. G. Gianopoulos, K. Kirschbaum, L. A. Peteanu and R. Jin, Tailoring Carbon Tails of Ligands on Au52(SR)32 Nanoclusters Enhances the Near-Infrared Photoluminescence Quantum Yield from 3.8 to 18.3%, J. Am. Chem. Soc., 2023, 145, 26328–26338 CrossRef CAS PubMed.
- W.-Q. Shi, L. Zeng, R.-L. He, X.-S. Han, Z.-J. Guan, M. Zhou and Q.-M. Wang, Near-unity NIR Phosphorescent Quantum Yield from a Room-Temperature Solvated Metal Nanocluster, Science, 2024, 383, 326–330 CrossRef CAS PubMed.
- W. Ishiii, Y. Okayasu, Y. Kobayashi, R. Tanaka, S. Kato, Y. Nishikawa, T. Kawai and T. Nakashima, Excited State Engineering in Ag29 Nanocluster through Peripheral Modification with Silver(I) Complexes for Bright Near-Infrared Photoluminescence, J. Am. Chem. Soc., 2023, 145, 11236–11244 CrossRef PubMed.
|
This journal is © the Partner Organisations 2024 |
Click here to see how this site uses Cookies. View our privacy policy here.