DOI:
10.1039/D3MA01046E
(Review Article)
Mater. Adv., 2024,
5, 3587-3601
Hydrogels as carriers deliver stem cells/exosomes for liver injury
Received
23rd November 2023
, Accepted 11th March 2024
First published on 21st March 2024
Abstract
Liver injury, both acute and chronic, poses a significant threat to human health, resulting in high morbidity and mortality rates. The current challenges in the treatment of liver injury are limited regenerative capacity and the few donor organs available. However, advancements in biomaterials and regenerative medicine are gradually mitigating these challenges. Hydrogels, with tissue-like properties and biomimetic characteristics, have become highly investigated biomedical materials, which can provide a microenvironment for stem cell survival and enable the controlled slow release of exosomes. Targeted therapy with hydrogel-encapsulated stem cells/exosomes has been demonstrated to promote tissue repair and regeneration. In this review, we first summarise the characteristics and design of hydrogels, emphasizing the design considerations of hydrogels as carriers for the encapsulation of stem cells/exosomes in the treatment of liver injury. We then provide an overview of the hydrogel preparation and administration strategy. Finally, we conclude that injectable hydrogels loaded with stem cells or exosomes are promising for liver tissue regeneration and repair. However, this field is still in its infancy and further research remains imperative.
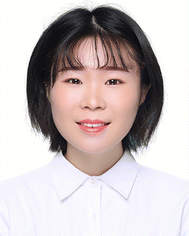
Qiuxia Zheng
| Dr Qiuxia Zheng is a 3rd year Hepatobiliary Surgery PhD at the School of Clinical Medicine, Lanzhou University. Her main focus is on the combination of stem cells and bio-delivery materials for the treatment of liver regeneration. She is interested in the clinical translation of biodegradable biomaterials as delivery systems, which has been the subject of her research from Masters to PhD. |
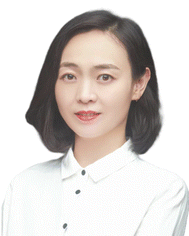
Jia Yao
| Dr Jia Yao is an associate researcher at Gansu's Key Laboratory of Biotherapeutics and Regenerative Medicine. She has extensive experience in peptide drug development, encompassing chemical synthesis, medicinal chemistry, and chemical biology. She combines this background with regenerative medicine to study the application of peptide biomaterials as delivery systems in tissue regeneration. Her current research interest is the use of hydrogels as carriers to encapsulate stem cell-derived exosomes in hair and liver regeneration. |
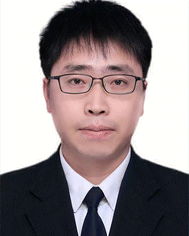
Zongbin Sun
| Dr Zongbin Sun is a second-year PhD candidate in Hepatobiliary Surgery at Lanzhou University School of Medicine. During his master's degree, he focused on the use of biomedical materials in digestive surgery. His current research interests include the use of delivery materials and stem cells to develop new therapeutic approaches for end-stage liver diseases. |
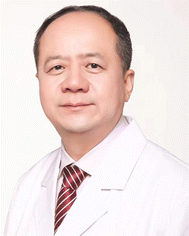
Xun Li
| Dr Xun Li is Professor of Hepatobiliary Surgery department at the First Hospital of Lanzhou University. He is interested in exploring clinical problems and translational research in multiple disciplines. His current main research interests are liver transplantation and liver regeneration, clinical translation of stem cells and exosomes, and bioartificial liver. |
1. Introduction
Liver injury is a common condition with the disappointing conclusion of liver failure and eventually death, resulting in approximately 2 million yearly deaths worldwide.1–3 Remarkably, liver diseases account for 3.5% of all global deaths.4 Hepatic injuries manifest in both acute and chronic forms. Acute liver injury (ALI) is characterized by excessive short-term liver cell death with high morbidity and mortality.5 Various hepatotoxic causes, such as trauma viruses, fat deposits, and medicines, can trigger ALI.4 Trauma is the most prevalent cause of ALI, resulting in rapid bleeding and subsequent liver failure that can be life-threatening.6 On the other hand, autoimmune disorders, alcoholism, and viral infections are common causes of chronic liver damage.7,8 The illness course is protracted, marked by chronic inflammation and liver fibrosis that worsen over time, rendering restoration of normal liver tissue challenging.8 Advanced chronic liver disease, specifically cirrhosis, is characterized by regenerated parenchymal nodules surrounded by fibrous septa, and significant changes in vascular structure, leading to fatal complications such as variceal bleeding, encephalopathy, and hepatopulmonary syndrome.9 In addition, owing to the long-term chronic inflammatory environment, reactive oxygen radicals and peroxidised lipids generate an adverse genotoxic climate that may cause genetic alterations and impairment, eventually promoting tumourigenesis.10 However, regardless of the stage of liver disease, hepatic imbalance impedes normal liver regeneration. Unfortunately, there are currently no effective curative therapies available for chronic liver injury. In the past few decades, cirrhosis and severe liver fibrosis have been difficult to reverse, and effective antifibrotic therapies remain unavailable.11 Therefore, highly effective and specific antifibrotic agents are needed to prevent further damage and enhance the regenerative potential of the liver. Liver transplantation is the optimal treatment option for patients with end-stage liver disease or severe ALI. However, due to the low supply and ever-increasing demand, it is difficult to find suitable liver donors.12 Another major challenge is the toxicity and adverse effects associated with long-term immunosuppression.13 Regenerative medicine is a promising option for repairing impaired tissues to ameliorate the problems of long-term immunosuppression and donor shortage in organ transplantation.
Cell therapy utilizing mesenchymal stem cells (MSCs) or exosomes is an encouraging paradigm for liver diseases that has been gradually translated into clinical practice. MSCs are multipotent stem cells that can reduce hepatocyte apoptosis, foster liver regeneration, reduce inflammation at lesion sites, inhibit hepatic stellate cell activation, and have anti-fibrotic and angiogenic effects in the injured liver.14,15 MSCs also have a preventive and ameliorative effect on the occurrence of acute liver failure after partial hepatectomy.16,17 However, several critical issues warrant attention: (1) stem cells can differentiate abnormally, resulting in tumour formation; (2) host rejection; (3) ectopic tissue formation; and (4) intravenous injection of stem cells retains them in the lung, minimising aggregation at the site of injury.18–20 Interestingly, stem cells can paracrine release some active substances such as exosomes or extracellular vesicles (EVs) that can promote liver repair and ameliorate systemic inflammation, thus providing a cell-free, less immunogenic approach to facilitate liver regeneration and repair.21 Exosomes are typically injected directly into the site of damage or delivered through multiple systemic injections. The disadvantages of intravenous exosome therapy include poor targeting and ease of removal.22 Local delivery, as opposed to systemic intravenous infusion, helps maintain a higher concentration in the targeted tissues. However, both may be susceptible to exosome overdose or quick clearance from the microenvironment, rather than ensuring long-term exosome release.
Hydrogels are biomaterials with a porous structure that enables them to function as slow-release stem cells/exosomes and mimic natural extracellular matrices to match the mechanical properties of target tissues.23–25 Hydrogel-loaded stem cells are an excellent option in the field of regeneration since they can also shield the cells from membrane damage during injection and offer a bionic 3D milieu for cell growth.26 Compared to two-dimensional monolayer cultures, three-dimensional growth conditions are preferable for cell growth and provide a better microenvironment for differentiating stem cells into the liver lineage.27 Thus, hydrogels as carriers can overcome the current limitations of conventional cell or active substance delivery. Moreover, hydrogels can deliver not only living cells but also active substances such as exosomes or EVs,28 proteins,29 drugs,30 peptides,31 and growth factors.32 They avoid rapid removal after infusion into the organism, and when locally injected local injection, which can improve the concentration of exosomes or stem cells in the hepatic injured site to facilitate regeneration and repair of the liver.33 Here are the merits of topical hydrogels: (1) in situ injectable hydrogels exhibit sol-like properties under ambient conditions and are transformed into a gel phase under physiological conditions, which can be directly injected anywhere desired;34 (2) hydrogels are amenable to specific modifications to improve their biological properties such as mechanical properties, making their morphological and functional properties akin to native tissues;35,36 (3) they offer an effective and minimally invasive approach that is easy to administer and provide three-dimensional growth conditions for stem cells to restore tissue function and reconduction.34,37,38 (4) They can slowly release stem cells or exosomes to avoid rapid clearance.
In this review, we focus on developments in the application of injectable hydrogels loaded with stem cells/exosomes in liver injury. Specifically, we discuss some crucial factors in designing these hydrogels as carriers for stem cells or exosomes, as well as the preparation and delivery strategies of hydrogels used in liver injury from a medical practicality perspective. At the end, we also summarise the current limitations and future potential of hydrogel-loaded stem cells/exosomes in treating liver diseases.
2. Characteristics and design of hydrogels
Hydrogels, characterized by their superior physicochemical properties, have emerged as the preferred choice for delivering cell-loaded and bioactive substances. Currently, studies on injectable hydrogels comprising exosomes or MSCs for tissue restoration have been conducted. We provide an overview of several applications (myocardial infarction,39 bone defect,40 spinal cord injury,41 skin wound42) that are now accessible in Fig. 1. Additionally, hydrogels are being used as scaffolds due to the following reasons: (1) the hydrogel's microporous structure and three-dimensional cross-network can promote cellular nutrient exchange and metabolite efflux, as well as promoting targeted drug administration and slowly release of bioactive substances; (2) the elasticity of the biological-like tissues can mimic the extracellular matrix. Therefore, hydrogels as medical materials can be designed to have properties like biocompatibility, tissue-like ECM, and bio-responsiveness to meet the demands of specific biomedical applications. Next, we will discuss the characteristics of hydrogels and the design considerations that should be taken into account when using them as biomaterials.
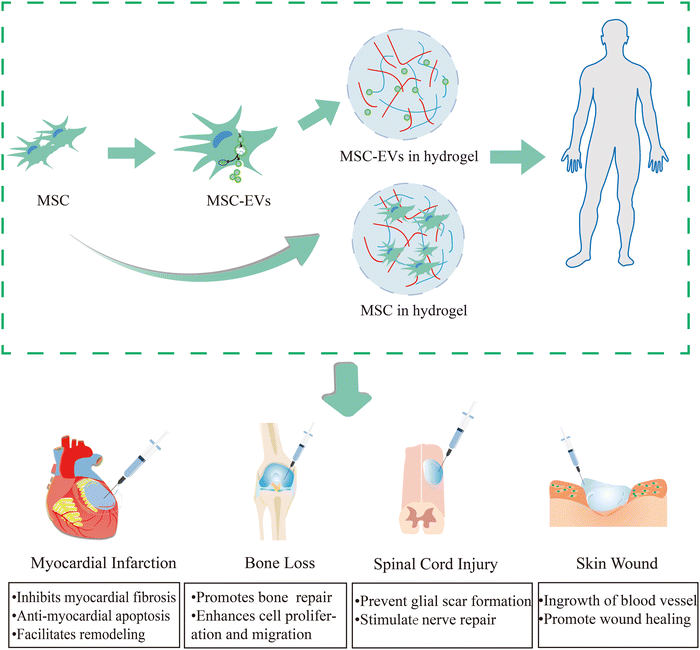 |
| Fig. 1 Injectable hydrogel-loaded stem cells/exosomes in tissue injuries (myocardial infarction,39 bone defect,40 spinal cord injury,41 skin wound42). In the treatment of myocardial infarction, myocardial remodeling will be facilitated by hybrid hydrogels, which will also prevent myocardial fibrosis and apoptosis. As for the therapy of bone loss, it can promote bone repair, and enhance cell proliferation and migration. For spinal cord injury, it can prevent glial scar formation and stimulate nerve repair. In the skin wound it can encourage vascular internalisation and wound healing. | |
2.1 Biocompatibility and safety
Biocompatibility is defined as the ability of a biomaterial to perform its desired function without eliciting any undesirable local or systemic side effects. For example, low immunogenicity, and non-toxicity. A hydrogel with poor biocompatibility can lead to several adverse outcomes within the host tissue, including heightened inflammation, foreign body reaction, and fibrous encapsulation, which can cause the implant to detach from the host tissue and lose its functionality.43 Thus, the highly biomimetic hydrogels must meet some criteria, including no local inflammatory reaction, non-toxicity, non-carcinogenicity, and low immunogenicity.44
2.2 Mechanical properties
Mechanical stability is a prerequisite for biomaterial applications. Elasticity, or stiffness, is important in controlling survival, proliferation, and differentiation.45 Otherwise, the hydrogels are not mechanically stable enough, indicating a high rate of biodegradation, which results in premature disassembly or disintegration.46,47 Notably, the mechanical forces within the surrounding milieu influence the direction of cellular differentiation. Physical cues may modulate and transform biochemical signals, which can be modulated by manipulating the encapsulated hydrogels to control stem cell fate.48,49 Furthermore, poor mechanical properties adversely affect the fate of stem cells or exosome release, thereby limiting the practical application of hydrogels in medicine.47
2.2.1 Effect of stiffness on stem cells.
It is an intricate process through which mechanical signals are detected, transduced, and integrated into a cascade of biochemical signals that ultimately determines the fate of cells.50 First, the mechanical stimulation of stem cells with hydrogels activates cell surface mechanosensory processes, including cellular integrins, focal adhesion kinases (FAKs), and Rho GTPases, to sense the stiffness of the hydrogel.51 Cells respond to the stiffness of the matrix by adjusting the adhesion complexes and cytoskeletal contractile forces according to the requirements of different cell-ECM adhesion strengths.52 Complexes and cytoskeletal contractility activate the downstream effectors of mechanotransduction signaling.53 In general, major mechanotransduction pathways are involved in stiffness-induced signaling, including integrin-dependent FAK signaling,54 Rho/ROCK signaling,55 YAP/TAZ signaling,56 MAPK, and Wnt/β-catenin signaling.57 They are responsible for translating mechanical signals into biochemical signals and determining stem cell fate (Fig. 2).
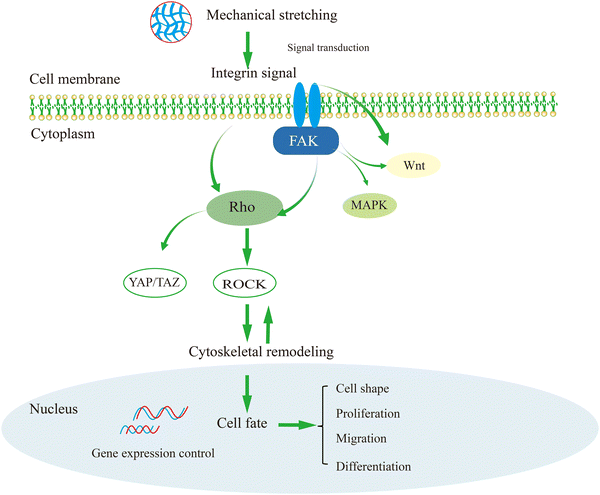 |
| Fig. 2 Effect of hydrogel stiffness on stem cells. Exerting a regulatory effect on stem cell fate by transducing mechanical signals into biochemical ones including FAK signaling,54 Rho/ROCK signaling,55 YAP/TAZ signaling,56 MAPK, and Wnt/β-catenin signaling.57 | |
2.2.2 Effect of stiffness on exosomes.
The regulation of exosomes through the mechanical properties of hydrogels remains unclear. Liu et al.58 reported that soft hydrogels release exosomes more rapidly than stiff hydrogels and promote in vivo repair more efficiently. However, the mechanical properties largely affect the release of exosomes from the hydrogel. Superior mechanical properties reduce the rate of degradation, allowing the slow release of exosomes with the degradation of the hydrogel and improving the long-term retention of exosomes within the hydrogel while improving the encapsulation properties and delivery, resulting in long-lasting therapeutic concentrations and doses for tissue repair.59 However, the long-term retention and stability of exosomes at defective sites are challenged by poor mechanical properties.60
2.3 Swelling
Swelling is influenced by the structural properties of the polymer network, particularly the crosslink density, which in turn influences stem cell differentiation and phenotypic matrix synthesis.61 Thus, the swelling property of hydrogels is an essential factor affecting the differentiation and infiltration of cells.62,63 Uncontrolled swelling even leads to tissue compression in the vicinity, which may be displaced or lost from the implanted sites, and some pores may even collapse, all of which are detrimental to integrity.64,65 For instance, the higher swelling characteristics in hydrogels typically result in volume expansion, which impairs the hydrogel's mechanical properties and squeezes the surrounding tissue in an undesirable way when applied in vivo.66 This can be particularly problematic after hepatectomy, where a highly swollen hydrogel may adversely affect liver repair. In cartilage tissue engineering, hydrogels with high swelling rates (12–35%) have greater expression levels of chondrocyte-specific genes, which are more conducive to the differentiation of mesenchymal stem cells into chondrocyte cell lines. The results may be due to better diffusion of signaling molecules and nutrients in hydrogel samples with high swelling ratios.61 However, an excessive swelling performance means that water molecules have a high diffusion rate under equilibrium conditions, thus inhibiting the attachment and growth of stem cells.67 On the other hand, hydrogels with low swelling are crucial for preserving steady tissue adhesion and reducing tissue inflammation. Nevertheless, currently available strategies for low swelling often result in a reduced tissue adhesion strength of hydrogels.68 Balancing swelling rates is crucial. Lower swelling rates may not provide adequate nutrient and metabolite efflux from the cells, and may also be detrimental to the release of exosomes, leading to less effective treatments. Further research is necessary to determine the acceptable range of swelling rates for different liver disorders, as there is currently none.
2.4 ECM-mimicking microstructures
Biological processes involve intricate interactions among cells, biological ligands, and extracellular matrix (ECM) structures to complete them.69 Tissue development can be more effectively guided by hydrogels that replicate the natural extracellular matrix in terms of its characteristics.44 Furthermore, to facilitate interactions and encourage the healing of damaged tissues, scaffold materials should incorporate ECM-mimicking microstructures.70 Also, the composition of the ECM microstructure will change depending on the tissues and organs to which it is applied to satisfy the demands of different cell development and differentiation. The hydrogel is similar to normal liver ECM (0.3–6 kPa), then the stem cells express higher levels of urea and protein liver marker genes.71 Moreover, applying the hydrogel to tougher tissues like bone defects, encourages stem cells to differentiate into polygonal-shaped bone tissue, which resembles osteoblasts.49,72 In addition, the micropore structure in the hydrogel is an important factor in promoting angiogenesis, controlling cell fate, facilitating successful cell in-growth into host tissues, and transporting nutrients and metabolites. Cell processes such as infiltration, proliferation, migration, and differentiation are related to pores and their interconnection.73,74 Therefore, tissue-matched and ECM-like hydrogels can better influence cellular growth processes and morphology.
2.5 Bio-responsiveness
Bio-responsive hydrogels must be able to undergo structural and chemical characterization in response to shifts in the microenvironment. These shifts play a crucial role in controlling cell development, metabolism, and release, making them essential for biomaterials to meet clinical requirements.75 Bioprinting-based hydrogel bioactive materials can offer a potent toolkit for imitating the spatially specified signals of encouraging regeneration and anti-fibrosis for clinical transformation, allowing researchers to better understand the spatial biological features that lead to fibrosis and wound healing.69 Thermogel-based bioprinting processes to prepare 3D hepatocytes loaded with hydrogel may provide a more accurate depiction of the pathophysiological responses of the liver to external stimuli, thus promoting cell metabolism, making it suitable for hepatotoxicity research.76 In addition, the design of bioactive, biomimetic, and bio-responsive scaffolds relies heavily on the insertion of peptide sequences into hydrogel materials, as peptides can enhance cell adhesion or stimulate signaling pathways.77
2.6 Dose of hydrogel-loaded stem cells/exosomes
The optimal dose of cells or exosomes to be added to the hydrogel is also a critical aspect of the design, yet it remains ambiguous. Generally, the number of stem cells required for treatment is 1 × 106. In partially hepatectomised fibrotic livers, the local injection of 2 × 106 MSCs was more effective in alleviating the effect of collagen deposition, suggesting that stem cell therapy is dose-dependent.78 Similarly, exosome injection is also dose-dependent, with systemic administration of 100 μg mL−1 benefiting the proliferation of hepatocytes compared to 50 μg mL−1.79 In a rat model of CCl4 liver fibrosis, MSC with a cell count of 3 × 106 was comparable to 80 μg mL−1 of stem cell-derived exosomes in terms of therapeutic benefits.80 HucMSC-Exos (administered at 16 mg kg−1) via the tail vein exerted antioxidant and antiapoptotic effects in a carbon tetrachloride (CCl4)-induced liver failure mouse model, resulting in effective treatment of liver failure.81 Exosome therapy has the advantage of allowing more flexibility in controlling the dose, frequency, and duration of administration.82 The advantage of exosome therapy lies in its flexibility to control dosage, frequency, and duration of administration. Thus, determining the optimal therapeutic dose of stem cells or exosomes encapsulated in hydrogels depends on individual factors such as injection modality, cell type, disease severity, and body weight. Based on these results, the optimal dose is considered for some factors for local injection as follows: (1) injectable doses differ between species due to differences in immunity; (2) the optimal dose may be related to disease type due to diverse injury microenvironments for different diseases. (3) There is dose-dependent to some extent, but if MSCs reach the threshold of affecting neighboring cells through paracrine action, there may be a lack of dose–response. In addition, the volume of hydrogel injection is related to the size of the cross-section after hepatectomy; hence, it is determined in different situations.
2.7 Release control
Ensuring stable and controlled release of stem cells or exosomes is crucial for achieving therapeutic effects. Several approaches for drug release from hydrogels exist, but hydrogels control the release of cells or exosomes mainly through degradation or sometimes in combination with mesh size and electrostatic interactions. Hydrogels can be degraded by simple dissolution, hydrolysis, enzymatic degradation, or a combination of these methods.83 For example, the controlled release of hepatocyte growth factor by enzymatic degradation of gelatin hydrogels can facilitate liver regeneration in cirrhotic rats, with sustained release for >3 weeks.84 In the literature, when the size of the extracellular vesicles (EVs) is larger than that of the hydrogel mesh, the release is controlled not only by the degradation rate but also by the mesh size.85 In addition, the positively charged hydrogel attracts negatively charged exosome membranes, allowing the release of exosomes from the hydrogel to be controlled by electrostatic binding, thereby enabling optimal therapeutic release at the desired time.86 Regarding the release time of hydrogels for cells or exosomes, most studies report a sustained release time of several weeks, commonly 4 weeks.
3. Stem cells/exosomes in liver diseases
Stem cells/exosomes have demonstrated a curative effect on liver diseases, which can downregulate infiltrating macrophages and thereby exert immunomodulatory effects, resist the activation of hepatic stellate cells with antifibrotic effects, stimulate hepatocyte proliferation, inhibit apoptosis and inflammatory responses, release several bioactive molecules by paracrine secretion, and promote liver regeneration.15,87,88 Soluble factors secreted by MSCs, including EVs or exosomes, can also ameliorate liver fibrosis and promote liver regeneration by regulating the function of macrophages, neutrophils, and monocytes. The ability of EVs or exosomes to directly infiltrate damaged target organs may be more effective than stem cells.89 Song et al.90 discovered that exosomes derived from human umbilical cord mesenchymal stem cells (hucMSCs) alleviated hepatocyte injury, improved liver function, and promoted liver regeneration after partial hepatectomy in rats, possibly because miR-124 in exosomes negatively regulates Foxg1 expression, thereby promoting hepatocyte proliferation. Xu et al.91 revealed that miR-182-5p in hypoxic bone marrow mesenchymal cell-derived exosomes promotes liver regeneration after partial hepatectomy through FOXO1-mediated macrophage polarisation. In addition, MSC-derived exosomes can reverse liver fibrosis and reduce inflammation by targeting the KLF6/STAT3 pathway in macrophages to deliver miR-148a.92 Thus, exosomes demonstrate functions similar to those of derived stem cells, and studies have shown that stem cells exert their therapeutic effects via paracrine exosomes.
Exosomes are nanosized phospholipid bilayers encapsulated by vesicles released from cells with a diameter of 30–150 nm.93 Typically, the molecular composition of exosomes includes proteins (member and nuclear proteins), metabolites, amino acids, and nucleic acids (mRNA, non-coding RNA species, and DNA).94 The process of exosome formation begins with the invagination of the cell membrane to form intracellular multivesicular bodies (MVBs) containing intraluminal vesicles (ILVs), which eventually fuse with the cell membrane and continuously release exosomes.95 Exosome release is regulated by various proteins, related enzymes, and stimuli. For instance, some proteins are directly or indirectly involved in mapping MVB to the plasma membrane via docking and fusion, followed by exosome release into the extracellular matrix.96,97 Eventually, the released exosomes interact with cell surface receptors in neighbouring or distant cells to deliver information molecules, such as cargo consisting of encapsulated lipids, proteins, and RNA, to recipient cells, thus triggering modifications in the expression and biological functions of the target cells.96,98 Exosomes mainly fuse with target cells as follows:99 (1) mutual recognition of ligands and receptors to activate intracellular signals; (2) fusing of the plasma membrane of target cells with the exosome membrane, and (3) internalisation of exosomes by target cells via endocytosis.
4. Hydrogel encapsulated MSC/exosomes in liver injury
Stem cell therapy attenuates liver inflammation, improves liver function, and promotes liver regeneration.100 Clinically, stem cell transplantation is generally performed through the portal vein, hepatic artery, and systemic intravenous infusion.101 However, with the immunosuppressive properties of stem cells, they are prone to be cleared quickly, while if injected intravenously, more stem cells are retained in the lungs.102 Moreover, several regenerative functions of MSCs are regulated by paracrine functions that are mainly secreted in the form of EV cargo.103 However, variations in the microenvironment in vivo, such as hypoxia and ischaemia, might promptly destroy MSCs, which would then cause the cessation of EV release.104 Injectable hydrogels serve as promising scaffolds to encapsulate stem cells or some active material, thus reducing the chance of clearance by the harsh microenvironment while providing a slow-releasing effect.
4.1 For post-partial hepatectomy
Partial hepatectomy (PH) is the main procedure for treating benign and malignant liver disorders.105 The postoperative liver volume and number of liver cells decrease rapidly, and the limited liver regeneration capacity is a risk factor for postoperative liver failure, which occurs in 1.2–11.0% of cases and is the most serious complication and major cause of mortality.106 Application of hydrogel-loaded stem cells or exosomes to liver resection after PH surgery allows rapid penetration of stem cells or exosomes into target tissues through continuous slow release.39,107 This streamlined delivery mechanism reduces the time required for systemic intravenous injection to reach the liver and minimizes elimination. As a result, these cells or exosomes effectively bolster hepatocyte growth capacity. In addition, inevitable postoperative adhesions and bleeding problems need to be resolved. The currently clinically available materials for haemostasis and adhesion prevention are fibrin glues, which have no promoting effect on tissue regeneration, have a certain immunogenicity, low adhesion strength, easily fall off, and are expensive.108,109 Thus, the application of hydrogel injections during liver resection offers the dual advantage of preserving hemostasis and preventing post-operative adhesions. Meanwhile, some solid sheets and film haemostatic products failed to completely cover the irregularly injured areas. However, injectable hydrogels can be loaded with stem cells or secreted active substances, which promote tissue regeneration and completely cover the injury site, compared with solid sheets and film products applied to irregular incisions to prevent postoperative adhesion and haemostasis.110,111
Thus, research related to the application of hydrogels after hepatectomy mainly focuses on the following three aspects: (1) hydrogels loaded with stem cells and active substances secreted by stem cells to promote liver regeneration; (2) hydrogels as physical barriers to reduce postoperative abdominal adhesions, and (3) hydrogels function as a haemostatic material to stop haemorrhage (Table 1). Li et al.110 introduced a bionic hybrid hydrogel consisting of oxidised hyaluronic acid (OHA), glycolic chitosan, and MenSC-derived conditioned medium (CM) (Fig. 3). By achieving controlled release of cytokines from the CM, this hybrid hydrogel when compared to the control groups accelerated liver function recovery (p < 0.05) and enhanced the expression of proliferation factors (PCNA, cyclin D1) after partial hepatectomy (p < 0.05). In addition, bleeding time (all data were shown as mean ± standard deviation, n = 4) was greater than 200 s in the control group, whereas in the hydrogel group it was less than 100 s. Also, no significant post-operative adhesions were seen in the experimental group compared to the control group. Wu et al.112 developed a composite hydrogel encapsulating MSCs-exos, which in addition to having good biocompatibility and hemostatic properties, encouraged hepatocyte proliferation by releasing exosomes. Thus, injectable hydrogels serve as potential new modalities for liver regeneration after hepatectomy.
Table 1 Studies related to the application of hydrogel after partial hepatectomy
Hydrogel types |
Materials components |
Advantages |
Ref. |
Thermal-responsive hydrogel |
Fe3O4@rGO, the polymer of NDP |
Haemostatic; prevent tumour recurrence; vascular embolization |
Yan et al., 2022113 |
Injectable chitosan hydrogel |
Dodecyl-modified N-carboxyethyl chitosan & oxidised konjac glucomannan |
Prevent adhesions; anti-inflammatory response; haemostatic |
Wei et al., 2022114 |
Self-healing hydrogel |
OHA; glycol chitosan; MenSCs-CM |
Arrest bleeding; anti-adhesion; and promote liver regeneration |
Li et al., 2021110 |
Injectable chitosan hydrogel |
Carboxymethyl chitosan and hyaluronic acid |
Haemostatic; good biodegradability |
Xia et al., 2021115 |
Anti-adhesion double-layered hydrogel |
Alginate–carboxymethyl cellulose; alginate–gelatin |
Anti-adhesion; promote wound healing |
Qi et al., 2019116 |
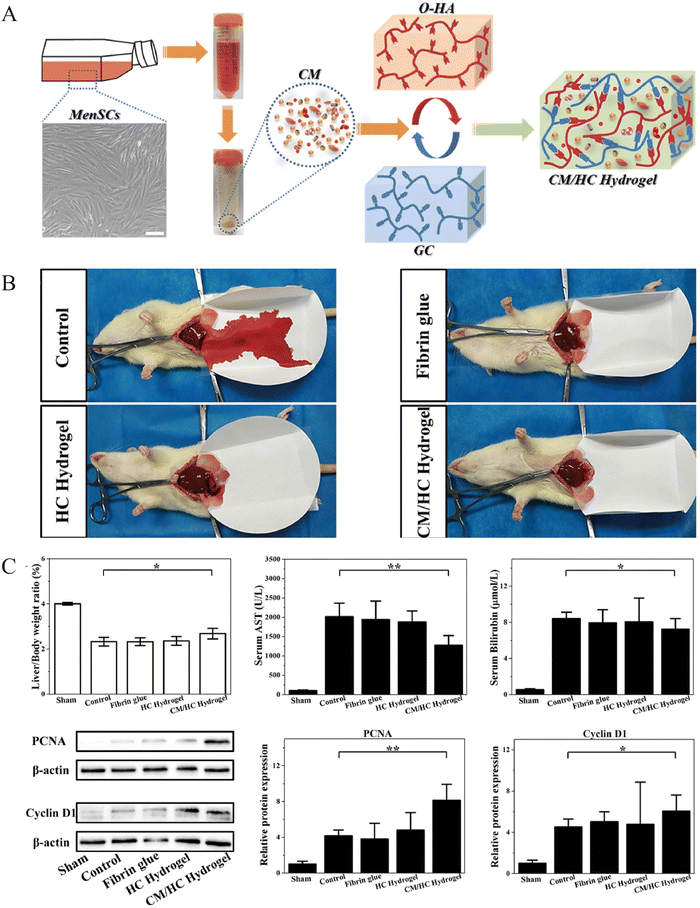 |
| Fig. 3 Preparation of the loaded menstrual blood-derived stem cell (MenSC)-derived conditioned medium (CM) hydrogel and observation of liver regeneration, hemostasis effects after hepatectomy. (A) Preparation of hybrid hydrogel-loaded MenSCs-CM. (B) Bleeding time and blood loss for hydrogel and control treatments. Data represent mean ± SD, n = 4. (C) Assessment of liver regeneration by liver to body weight ratio, blood AST and bilirubin levels, and western blot analysis of PCNA and cyclin D1 for cell proliferation in different therapy groups at 24 hours after partial liver resection. Data represent mean ± SD, n = 6; *p < 0.05, **p < 0.01. Reprinted from Li et al.110 with permission from Elsevier. Copyright (2021). | |
4.2 For chronic liver injury
Chronic liver injury causes inflammation and fibrosis in the liver. Activated myofibroblasts in the liver secrete extracellular matrix proteins through these cells, resulting in fibrous scarring, while the main source of these myofibroblasts is the resident hepatic stellate cells.117 Stem cells or secreted exosomes suppress HSC (hepatic stellate cell), activation of hepatic stellate cells, and reverse liver fibrosis.118 Injecting the stem cell/exosome-loaded hydrogel into the peritoneal cavity causes the exosomes to slowly release. Generally, medications injected intraperitoneally are typically absorbed into the abdominal cavity's tissues directly, or via the omentum or portal vein, into the blood circulation, and ultimately into the tissues.119 Thus, the released exosomes can be absorbed by the liver directly or by circulation. This process promotes liver regeneration by acting as an anti-inflammatory and fibrotic agent. Notably, Mardpour et al.85 reported a PEG-loaded MSC-EV hydrogel injected into the peritoneal cavity, which provided sufficient free space to accommodate the EV-loaded hydrogels and could control the continuous release of EVs with swelling compared to free-EV groups, allowing EVs to enter the liver tissue continuously (Fig. 4). This provided a new approach to improve liver function and reduce apoptosis and fibrosis of hepatocytes. Thus, sustainable systemic delivery of injectable hydrogel-controlled released EVs can enhance the sustained concentration of MSC-EVs in circulation, thereby prolonging bioavailability at targeted sites.
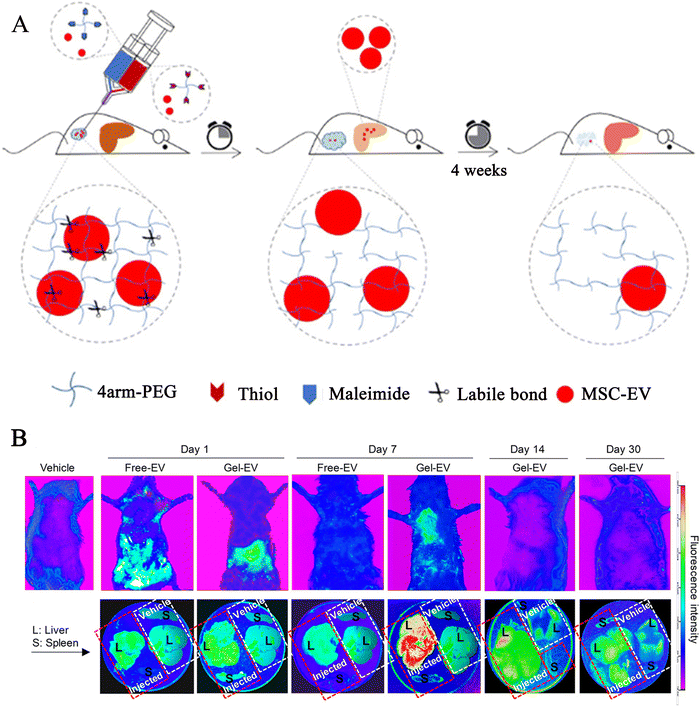 |
| Fig. 4 The preparation of loaded exosomes click hydrogel and explored the PKH-26 labelled exosomes biodistribution in hepatic tissues at different time points. (A) Preparation process of tetra-PEG click hydrogel delivery of MSC-EVs. (B) Biodistribution images (the whole body of rats, liver and spleen, respectively) for PKH-26 labelled MSC-EV loaded hydrogels and free EVs by intraperitoneal injection at 1, 7, 14, and 30 days in a rat model of chronic liver failure. Rescued from Mardpour et al.85 with permission from ASC. Copyright (2019). | |
However, for conventional bulk hydrogels, which are suitable for precise local injection at injured tissue sites, the lesions in chronic liver disease are more diffuse because most chronic liver lesions are associated with liver fibrosis, which increases the risk of bleeding with intrahepatic local injections. However, injectable nano or microscopic hydrogels are more suitable for treating liver disease because of the assurance of cell activity and bioactivity in the microgel and the greater number and flexibility of injection options.
5. Administration strategy in treating liver injury
Research has indicated that hydrogel biomaterials possess the capability to serve as bioactive materials with therapeutic significance, particularly for applications of drug loading, exosomes, and cell delivery. Injectable hydrogels combined with cells/exosomes offer a more promising delivery technique for the treatment of liver damage. These hydrogels can be designed to be bioactive, bioresponsive, and ECM-like liver biomaterials. Currently, hydrogels with varying therapeutic substance contents are mostly delivered in the following ways: (1) direct injection into the liver resection site during hepatectomy can provide compression to reduce bleeding and prevent adhesions. Additionally, hydrogels can also slowly and consistently release active substances that promote liver regeneration.110 (2) In the treatment of chronic liver injury, hydrogels containing active compounds are injected into the peritoneal cavity to exert antifibrotic, anti-inflammatory, anti-apoptotic, and regenerative effects.120,121 (3) Improving cirrhosis with the intrahepatic multisite injection of cell-loaded hydrogel, which reduced collagen matrix deposition and improved liver structure and function.122 In the next sections, we will discuss how to prepare and design hydrogels that can be better applied to liver injury diseases.
6. Preparation of hydrogel in liver injury
Currently, there are various types of injectable hydrogel preparations for loaded exosomes/stem cells such as covalent bonding, 3D printing, and photo-crosslinked hydrogels. These hydrogels have several advantages, such as easy incorporation and maintenance of cell or exosome viability, local injection, easy manipulation, and moderate gelation time.
6.1 Covalent bonding hydrogel
Several covalent processes can be employed to produce injectable hydrogels. These include Michael addition reactions, Schiff base reactions, and click chemical reactions.123 Intraperitoneally, clickable PEG derivatives and MSC-EVs were administered to produce EV-laden networks that could regulate the release of EVs by gradually swelling to home in on the chronic liver injuries and provide antifibrotic effects. Importantly, it is an extremely effective, “green” response and without by-production during applications.85,124 Additionally, this kind of hydrogel is appropriate for delivering cells, as post-injection encapsulated MSCs retain over 90% cell vitality.125
6.2 3D printing hydrogel
Hydrogels possess the remarkable flexibility and versatility of technology to build human tissue through three-dimensional (3D) printing.126 Recent advancements in 3D bioprinting technology have demonstrated the feasibility of creating functional liver tissue, opening up exciting possibilities for liver regeneration and transplantation.127,128 Goulart et al.129 discovered that the 3D printing of human liver iPS-derived parenchymal cells in the form of spherical aggregates could significantly enhance liver function and prolong the long-term survival time of tissues in vitro through the alginate/pluronic hydrogel mixture. 3D printing enables the creation of hepatic lobular structures that promote cell–cell and cell–matrix interactions, allowing for the simulation of the microenvironment of liver fibrosis.130,131 In the future, it may serve as a substitute for animal testing in drug development, thereby reducing associated time and costs.
6.3 Photo-crosslinked hydrogel
Photo-crosslinked hydrogels are synthetic biomaterials that are generated through cross-linking reactions triggered by light, either ultraviolet (UV) or visible light. They are biologically active and frequently utilised to make in situ hydrogels. Gelatin methacrylate (GelMA), which is frequently included as a photo-initiator, covalently cross-links under UV light to permanently fix the shape of hydrogels.132 Furthermore, GelMA is an extracellular matrix (ECM)-like hydrogel material due to the incorporation of the arginine–glycine–aspartate (RGD) sequence and matrix metalloproteinase (MMP) motifs, which stimulates cell adhesion and proliferation.133 Xia et al.112 designed a photo-crosslinked hydrogel loaded with exosomes composed of GelMA, dopamine-modified alginate (Alg-DA), and exos (GelMA/Alg-DA/Exo). The hydrogel was applied after hepatectomy and demonstrated good adhesion and biocompatibility, and effective haemostasis while also promoting hepatocyte regeneration.
6.4 Thermosensitive hydrogel
A promising alternative for injectable hydrogels is thermosensitive hydrogels, which can undergo a sol–gel transition in response to temperature changes. Biomedical thermosensitive hydrogels are typically designed to gel in situ at a physiological temperature (37 °C), enabling direct gelation at the desired site without the need for additional chemical reactions.134 The majority of the substances used are naturally occurring polymers like gelatin or chitosan. These materials have good biocompatibility and could promote nutrient exchange and metabolic substance discharge for stem cells, which is conducive to cell viability.135,136 Meanwhile, the suitable pore sizes may display a sustained-release profile. Chiang et al.33 developed a temperature-sensitive hydrogel containing hepatocyte-like cells (iPSC-Heps), carboxymethyl–hexanoyl chitosan, and hepatocyte growth factor (HGF-CHC). These hydrogels were administered intrahepatically in acute liver failure and were found to reduce hepatic necrosis and improve liver function.
6.5 Natural hydrogel
Natural hydrogels can provide a microenvironment similar to the extracellular matrix (ECM). These materials exhibit a low immune response, promote angiogenesis, improve cell adhesion, and haemostasis.137 The liver extracellular matrix (ECM) is a natural hydrogel scaffold frequently employed in liver regeneration research, which preserves the natural components of the liver, promotes easy gelation, induces efficient hepatocyte differentiation from stem cells and can be used as an injectable medium for hepatocytes.138
6.6 Microspheres
In regenerative medicine, hydrogel microspheres containing medicines, bioactive materials, and cells are frequently utilised. Their design facilitates the growth of cells within their cavities, resulting in the formation of cell aggregates.139 Additionally, they can shield the cells from antibodies and immune cells while permitting the diffusion of nutrients, proteins, and medications into and out of the polymer matrix.140 Hydrogel microspheres loaded with cells, bioactive substances, and drugs are widely used in regenerative medicine. Hydrogel microspheres allow cells to proliferate in the cavities of the microspheres, forming cell aggregates, and the microspheres protect the cells from antibodies and immune cells while allowing nutrients, proteins, and drugs to diffuse in and out of the polymer matrix.
7. Limitations and perspectives
Substantial progress has been made in the use of injectable hydrogels. However, some challenges still need to be addressed when designing hydrogels. Hydrogels are crosslinked polymer networks that typically contain meshes that are orders of magnitude smaller than a cell.141 Therefore, dense polymer networks limit cell proliferation, differentiation, mass transport, tissue ingrowth, and vascular invasion.142 These shortcomings may result in low cell viability after transplantation into the host tissue, which has previously been reported as only 1–20%.107,143 Second, natural polymer materials are low-cost, effective, biodegradable, and biocompatible and are often used to fabricate injectable hydrogel materials that encapsulate stem cells.26,144 However, most natural injectable hydrogels have poor mechanical strength and stability, resulting in insufficient mechanical support for cells or exosomes;145 poor mechanical performance also leads to stem cell or exosome release, thereby quickly losing their effect. In addition, good biocompatibility, such as appropriate pH and temperature, is required to provide a suitable microenvironment for stem cell survival and exosome viability.
The liver is a vital intra-abdominal organ, so injectable hydrogel-loaded stem cells or exosomes can have sufficient space and opportunity to play a therapeutic role in liver surgery. However, hydrogel applications for liver diseases are currently considered restricted. Exploring microgels or nanohydrogels that encapsulate stem cell-secreted exosomes via intravenous injection holds promise as a potential solution. Recent reports on inhibiting collagen synthesis and deposition in fibrotic mice by cationic nanohydrogels loaded with siRNA showed effective antifibrotic effects by targeting collagen cells after systemic intravenous administration.146 Therefore, nanohydrogels loaded with miRNAs or proteins in exosomes, which exert components that promote liver regeneration or reduce fibrosis, potentially provide a new research direction for treating liver diseases.
Future applications of hydrogels in the treatment of liver disease will become more widespread and efficient as material science advances. This is because, by injecting directly into the liver or adjacent to the target location (such as the peritoneal cavity), hydrogels can ensure a sufficiently concentrated therapeutic dose at the site of liver injury and avoid the premature removal of loaded cells or exosomes.
8. Conclusions
Injectable hydrogels loaded with stem cells or exosomes are feasible for treating liver diseases, especially for regeneration and repair after partial hepatectomy. Still, research on using hydrogels to treat liver injury requires continuous improvement. On the one hand, there is a demand for developing new nano hydrogel materials with targeting properties that will allow effective therapeutic effects of hydrogels, even with systemic administration. On the other hand, to enable better clinical applications, more research on optimal hydrogel design factors in liver disease is necessary. Nonetheless, the clinical application of hydrogels in the treatment of liver disorders offers novel therapeutic alternatives and warrants further research.
Abbreviations
ALI | Acute liver injury |
MSCs | Mesenchymal stem cells |
EVs | Extracellular vesicle |
huc-MSCs | Human umbilical cord mesenchymal stem cells |
ECM | Extracellular matrix |
MVBs | Intracellular multivesicular bodies |
ILVs | Intraluminal vesicles |
PH | Partial hepatectomy |
OHA | Oxidised hyaluronic acid |
CM | Conditioned medium |
HSC | Hepatic stellate cell |
FAKs | Focal adhesion kinases |
Author contributions
Qiuxia Zheng: writing – original draft, visualization, conceptualization. Jia Yao: project administration, writing – review & editing, funding acquisition. Zongbin Sun: data curation, investigation. Yongcui Mao: visualization, data curation. Jiayun Wei: validation. Ye Xie: writing – review & editing. Xuekai Hu: data curation. Xun Li: conceptualization, supervision, funding acquisition.
Conflicts of interest
The authors affirm that they have no known financial or interpersonal issues that would have appeared to have an impact on the research described in this publication.
Acknowledgements
The National Natural Science Foundation of China (82060119) and Gansu Provincial Clinical Medical Research Center for General Surgery (20JR10FA661) contributed to this research.
References
- A. Garrido and N. Djouder, Trends Cancer, 2021, 7, 29 CrossRef CAS PubMed.
- Z. Zhang and F.-S. Wang, J. Hepatol., 2013, 59, 183 CrossRef PubMed.
- S. K. Asrani, H. Devarbhavi, J. Eaton and P. S. Kamath, J. Hepatol., 2019, 70, 151 CrossRef PubMed.
- F. Lin, W. Chen, J. Zhou, J. Zhu, Q. Yao, B. Feng, X. Feng, X. Shi, Q. Pan, J. Yu, L. Li and H. Cao, Cell Death Dis., 2022, 13, 271 CrossRef CAS PubMed.
- Y. A. Samra, M. F. Hamed and A. R. El-Sheakh, J. Biochem. Mol. Toxicol., 2020, 34, e22470 CrossRef CAS PubMed.
- A. Hetherington, F. S. Cardoso, E. L. W. Lester and C. J. Karvellas, Curr. Opin. Crit. Care, 2022, 28, 184 CrossRef PubMed.
- A. Caligiuri, A. Gentilini, M. Pastore, S. Gitto and F. Marra, Cells, 2021, 10, 2759 CrossRef CAS PubMed.
- L. Yao, X. Hu, K. Dai, M. Yuan, P. Liu, Q. Zhang and Y. Jiang, Stem Cell Res. Ther., 2022, 13, 308 CrossRef CAS PubMed.
- C. Lejealle, V. Paradis, O. Bruno, E. de Raucourt, C. Francoz, O. Soubrane, D. Lebrec, P. Bedossa, D. Valla, H. Mal, V. Vilgrain, F. Durand and P.-E. Rautou, Chest, 2019, 155, 123 CrossRef PubMed.
- G. K. Michalopoulos and B. Bhushan, Nat. Rev. Gastroenterol. Hepatol., 2021, 18, 40 CrossRef PubMed.
- D. Schuppan, M. Ashfaq-Khan, A. T. Yang and Y. O. Kim, Matrix Biol., 2018, 68–69, 435 CrossRef CAS PubMed.
- K.-C. Huang, M.-H. Chuang, Z.-S. Lin, Y.-C. Lin, C.-H. Chen, C.-L. Chang, P.-C. Huang, W.-S. Syu, T.-W. Chiou, Z.-H. Hong, Y.-C. Tsai, H.-J. Harn, P.-C. Lin and S.-Z. Lin, Cell Transplant., 2019, 28, 100S Search PubMed.
- A. Zarrinpar and R. W. Busuttil, Nat. Rev. Gastroenterol. Hepatol., 2013, 10, 434 CrossRef CAS PubMed.
- D. van Poll, B. Parekkadan, C. H. Cho, F. Berthiaume, Y. Nahmias, A. W. Tilles and M. L. Yarmush, Hepatology, 2008, 47, 1634 CrossRef CAS PubMed.
- M. Alfaifi, Y. W. Eom, P. N. Newsome and S. K. Baik, J. Hepatol., 2018, 68, 1272 CrossRef CAS PubMed.
- H.-R. Ding, J.-L. Wang, Z.-T. Tang, Y. Wang, G. Zhou, Y. Liu, H.-Z. Ren and X.-L. Shi, Front. Physiol., 2019, 10, 412 CrossRef PubMed.
- H.-M. Tautenhahn, S. Brückner, S. Baumann, S. Winkler, W. Otto, M. von Bergen, M. Bartels and B. Christ, Ann. Surg., 2016, 263, 546 CrossRef PubMed.
- S. Wang, L. Guo, J. Ge, L. Yu, T. Cai, R. Tian, Y. Jiang, R. C. Zhao and Y. Wu, Stem Cells, 2015, 33, 3315 CrossRef CAS PubMed.
- Y. Zhang, Y. Li, L. Zhang, J. Li and C. Zhu, Stem Cell Res. Ther., 2018, 9, 59 CrossRef CAS PubMed.
- M. Mendt, K. Rezvani and E. Shpall, Bone Marrow Transplant., 2019, 54, 789 CrossRef PubMed.
- A. Psaraki, L. Ntari, C. Karakostas, D. Korrou-Karava and M. G. Roubelakis, Hepatology, 2022, 75, 1590 CrossRef CAS PubMed.
- N. Zhang, Y. Song, Z. Huang, J. Chen, H. Tan, H. Yang, M. Fan, Q. Li, Q. Wang, J. Gao, Z. Pang, J. Qian and J. Ge, Biomaterials, 2020, 255, 120168 CrossRef CAS PubMed.
- R. Dimatteo, N. J. Darling and T. Segura, Adv. Drug Delivery Rev., 2018, 127, 167 CrossRef CAS PubMed.
- B. J. Klotz, D. Gawlitta, A. J. W. P. Rosenberg, J. Malda and F. P. W. Melchels, Trends Biotechnol., 2016, 34, 394 CrossRef CAS PubMed.
- M. Guan, C. Liu, Q. Zheng, G. Chu, H. Wang, J. Jin, H. Wu, J. Chen, Q. Huang, Z. Deng and Y. Wang, Int. J. Biol. Macromol., 2023, 232, 123479 CrossRef CAS PubMed.
- X. Yang, H. Yang, X. Jiang, B. Yang, K. Zhu, N. C.-H. Lai, C. Huang, C. Chang, L. Bian and L. Zhang, Carbohydr. Polym., 2021, 256, 117574 CrossRef CAS PubMed.
- T. T. Lau, L. W. Ho and D.-A. Wang, Biomaterials, 2013, 34, 6659 CrossRef CAS PubMed.
- K. Zhang, X. Zhao, X. Chen, Y. Wei, W. Du, Y. Wang, L. Liu, W. Zhao, Z. Han, D. Kong, Q. Zhao, Z. Guo, Z. Han, N. Liu, F. Ma and Z. Li, ACS Appl. Mater. Interfaces, 2018, 10, 30081 CrossRef CAS PubMed.
- F. Lee, J. E. Chung and M. Kurisawa, J. Controlled Release, 2009, 134, 186 CrossRef CAS PubMed.
- E. Mei, C. Chen, C. Li, X. Ding, J. Chen, Q. Xi, S. Zhou, J. Liu and Z. Li, Biomacromolecules, 2021, 22, 5339 CrossRef CAS PubMed.
- K. Kulkarni, R. L. Minehan, T. Gamot, H. A. Coleman, S. Bowles, Q. Lin, D. Hopper, S. E. Northfield, R. A. Hughes, R. E. Widdop, M.-I. Aguilar, H. C. Parkington and M. P. Del Borgo, ACS Appl. Mater. Interfaces, 2021, 13, 58279 CrossRef CAS PubMed.
- N. Zhao, A. Suzuki, X. Zhang, P. Shi, L. Abune, J. Coyne, H. Jia, N. Xiong, G. Zhang and Y. Wang, ACS Appl. Mater. Interfaces, 2019, 11, 18123 CrossRef CAS PubMed.
- C.-H. Chiang, W.-W. Wu, H.-Y. Li, Y. Chien, C.-C. Sun, C.-H. Peng, A. T.-L. Lin, C.-S. Huang, Y.-H. Lai, S.-H. Chiou, S.-I. Hung, Y.-L. Chang, Y.-T. Lan, D.-M. Liu, C.-S. Chien, T.-I. Huo, S.-D. Lee and C.-Y. Wang, Cell Transplant., 2015, 24, 541 Search PubMed.
- J. Radhakrishnan, U. M. Krishnan and S. Sethuraman, Biotechnol. Adv., 2014, 32, 449 CrossRef CAS PubMed.
- Z. Zheng, Y. Tan, Y. Li, Y. Liu, G. Yi, C.-Y. Yu and H. Wei, J. Controlled Release, 2021, 335, 216 CrossRef CAS PubMed.
- K. Flégeau, R. Pace, H. Gautier, G. Rethore, J. Guicheux, C. Le Visage and P. Weiss, Adv. Colloid Interface Sci., 2017, 247, 589 CrossRef PubMed.
- M. Sepantafar, R. Maheronnaghsh, H. Mohammadi, S. Rajabi-Zeleti, N. Annabi, N. Aghdami and H. Baharvand, Biotechnol. Adv., 2016, 34, 362 CrossRef CAS PubMed.
- J. Xu, Q. Feng, S. Lin, W. Yuan, R. Li, J. Li, K. Wei, X. Chen, K. Zhang, Y. Yang, T. Wu, B. Wang, M. Zhu, R. Guo, G. Li and L. Bian, Biomaterials, 2019, 210, 51 CrossRef CAS PubMed.
- J. Tang, X. Cui, Z. Zhang, Y. Xu, J. Guo, B. G. Soliman, Y. Lu, Z. Qin, Q. Wang, H. Zhang, K. S. Lim, T. B. F. Woodfield and J. Zhang, Adv. Healthcare Mater., 2022, 11, e2100312 CrossRef PubMed.
- Q. Feng, J. Xu, K. Zhang, H. Yao, N. Zheng, L. Zheng, J. Wang, K. Wei, X. Xiao, L. Qin and L. Bian, ACS Cent. Sci., 2019, 5, 440 CrossRef CAS PubMed.
- Y. Luo, L. Fan, C. Liu, H. Wen, S. Wang, P. Guan, D. Chen, C. Ning, L. Zhou and G. Tan, Bioact. Mater., 2022, 7, 98 CAS.
- E. J. Kim, J. S. Choi, J. S. Kim, Y. C. Choi and Y. W. Cho, Biomacromolecules, 2016, 17, 4 CrossRef CAS PubMed.
- R. Rothe, Y. Xu, A. K. Thomas, S. Meister, Y. Zhang, J. Pietzsch and S. Hauser, Biomaterials, 2021, 269, 120637 CrossRef CAS PubMed.
- M. Shagidulin, N. Onishchenko, V. Sevastianov, M. Krasheninnikov, A. Lyundup, A. Nikolskaya, A. Kryzhanovskaya, S. Voznesenskaia, M. Gorelova, N. Perova, I. Kozlov, A. Venediktov, G. Piavchenko and S. Gautier, Gels, 2023, 9, 456 CrossRef CAS PubMed.
- H. Aurich, M. Sgodda, P. Kaltwasser, M. Vetter, A. Weise, T. Liehr, M. Brulport, J. G. Hengstler, M. M. Dollinger, W. E. Fleig and B. Christ, Gut, 2009, 58, 570 CrossRef CAS PubMed.
- F. Mirahmadi, M. Tafazzoli-Shadpour, M. A. Shokrgozar and S. Bonakdar, Mater. Sci. Eng., C, 2013, 33, 4786 CrossRef CAS PubMed.
- E. J. Lee, E. Kang, S.-W. Kang and K. M. Huh, Carbohydr. Polym., 2020, 244, 116432 CrossRef CAS PubMed.
- J. J.-M. Su, C.-H. Lin, H. Chen, S.-Y. Lee and Y.-M. Lin, Polymers, 2021, 13, 2354 CrossRef CAS PubMed.
- A. J. Engler, S. Sen, H. L. Sweeney and D. E. Discher, Cell, 2006, 126, 677 CrossRef CAS PubMed.
- J. Zhang, H. Yang, B. E. Abali, M. Li, Y. Xia and R. Haag, Small, 2019, 15, e1901920 CrossRef PubMed.
- M. Smyth, C. Fournier, C. Driemeier, C. Picart, E. J. Foster and J. Bras, Biomacromolecules, 2017, 18, 2034 CrossRef CAS PubMed.
- H. Cao, L. Duan, Y. Zhang, J. Cao and K. Zhang, Signal Transduction Targeted Ther., 2021, 6, 426 CrossRef CAS PubMed.
- K. H. Vining and D. J. Mooney, Nat. Rev. Mol. Cell Biol., 2017, 18, 728 CrossRef CAS PubMed.
- B. Xu, G. Song, Y. Ju, X. Li, Y. Song and S. Watanabe, J. Cell. Physiol., 2012, 227, 2722 CrossRef CAS PubMed.
- T. Teramura, T. Takehara, Y. Onodera, K. Nakagawa, C. Hamanishi and K. Fukuda, Biochem. Biophys. Res. Commun., 2012, 417, 836 CrossRef CAS PubMed.
- S. Dupont, Exp. Cell Res., 2016, 343, 42 CrossRef CAS PubMed.
- J. Du, Y. Zu, J. Li, S. Du, Y. Xu, L. Zhang, L. Jiang, Z. Wang, S. Chien and C. Yang, Sci. Rep., 2016, 6, 20395 CrossRef CAS PubMed.
- Z. Liu, H. Tong, J. Li, L. Wang, X. Fan, H. Song, M. Yang, H. Wang, X. Jiang, X. Zhou, H. Yuan and Y. Wang, Front. Bioeng. Biotechnol., 2022, 10, 922570 CrossRef PubMed.
- K. Holkar, A. Vaidya, P. Pethe, V. Kale and G. Ingavle, Materialia, 2020, 12, 100736 CrossRef CAS.
- S. Ma, J. Wu, H. Hu, Y. Mu, L. Zhang, Y. Zhao, X. Bian, W. Jing, P. Wei, B. Zhao, J. Deng and Z. Liu, Mater. Today Bio, 2022, 13, 100195 CrossRef CAS PubMed.
- H. A. Lin, M. S. Gupta, D. M. Varma, M. L. Gilchrist and S. B. Nicoll, J. Biomed. Mater. Res., Part A, 2016, 104, 165 CrossRef PubMed.
- S. Dhivya, S. Saravanan, T. P. Sastry and N. Selvamurugan, J. Nanobiotechnol., 2015, 13, 40 CrossRef CAS PubMed.
- H. Park, X. Guo, J. S. Temenoff, Y. Tabata, A. I. Caplan, F. K. Kasper and A. G. Mikos, Biomacromolecules, 2009, 10, 541 CrossRef CAS PubMed.
- H. Kamata, K. Kushiro, M. Takai, U.-I. Chung and T. Sakai, Angew. Chem., Int. Ed., 2016, 55, 9282 CrossRef CAS PubMed.
- S. Saravanan, S. Vimalraj and D. Anuradha, Biomed. Pharmacother., 2018, 107, 908 CrossRef CAS PubMed.
- Y. Zhan, W. Fu, Y. Xing, X. Ma and C. Chen, Mater. Sci. Eng., C, 2021, 127, 112208 CrossRef CAS PubMed.
- J. G. Martins, S. E. A. Camargo, T. T. Bishop, K. C. Popat, M. J. Kipper and A. F. Martins, Carbohydr. Polym., 2018, 197, 47 CrossRef CAS PubMed.
- G. Tian, D. Yang, C. Liang, Y. Liu, J. Chen, Q. Zhao, S. Tang, J. Huang, P. Xu, Z. Liu and D. Qi, Adv. Mater., 2023, 35, 2212302 CrossRef CAS PubMed.
- J. L. Guo and M. T. Longaker, Gels, 2022, 9, 19 CrossRef PubMed.
- S. Liu, A. C. Moore, A. B. Zerdoum, H. Zhang, S. L. Scinto, H. Zhang, L. Gong, D. L. Burris, A. K. Rajasekaran, J. M. Fox and X. Jia, Biomaterials, 2018, 180, 24 CrossRef CAS PubMed.
- Y. Hwang, M. Goh, M. Kim and G. Tae, Biomaterials, 2018, 165, 94 CrossRef CAS PubMed.
- N. Huebsch, E. Lippens, K. Lee, M. Mehta, S. T. Koshy, M. C. Darnell, R. M. Desai, C. M. Madl, M. Xu, X. Zhao, O. Chaudhuri, C. Verbeke, W. S. Kim, K. Alim, A. Mammoto, D. E. Ingber, G. N. Duda and D. J. Mooney, Nat. Mater., 2015, 14, 1269 CrossRef CAS PubMed.
- X. Pei, L. Ma, B. Zhang, J. Sun, Y. Sun, Y. Fan, Z. Gou, C. Zhou and X. Zhang, Biofabrication, 2017, 9, 045008 CrossRef PubMed.
- L. Griveau, M. Lafont, H. le Goff, C. Drouglazet, B. Robbiani, A. Berthier, D. Sigaudo-Roussel, N. Latif, C. L. Visage, V. Gache, R. Debret, P. Weiss and J. Sohier, Acta Biomater., 2022, 140, 324 CrossRef CAS PubMed.
- G. Narendran, A. Walunj, A. M. Kumar, P. Jeyachandran, N. S. Awwad, H. A. Ibrahium, M. R. Gorji and D. A. Perumal, Bioengineering, 2023, 10, 416 CrossRef CAS PubMed.
- M. Gori, S. M. Giannitelli, M. Torre, P. Mozetic, F. Abbruzzese, M. Trombetta, E. Traversa, L. Moroni and A. Rainer, Adv. Healthcare Mater., 2020, 9, e2001163 CrossRef PubMed.
- C. S. Bahney, T. J. Lujan, C. W. Hsu, M. Bottlang, J. L. West and B. Johnstone, Eur. Cells Mater., 2011, 22, 43 CrossRef CAS PubMed.
- D. van der Helm, M. C. Barnhoorn, E. S. M. de Jonge-Muller, I. Molendijk, L. J. A. C. Hawinkels, M. J. Coenraad, B. van Hoek and H. W. Verspaget, J. Cell. Mol. Med., 2019, 23, 6238 CrossRef CAS PubMed.
- J.-Y. Wu, A.-L. Ji, Z.-X. Wang, G.-H. Qiang, Z. Qu, J.-H. Wu and C.-P. Jiang, Sci. Rep., 2018, 8, 2471 CrossRef PubMed.
- D. M. Rostom, N. Attia, H. M. Khalifa, M. W. Abou Nazel and E. A. El Sabaawy, Tissue Eng. Regener. Med., 2020, 17, 537 CrossRef CAS PubMed.
- Y. Yan, W. Jiang, Y. Tan, S. Zou, H. Zhang, F. Mao, A. Gong, H. Qian and W. Xu, Mol. Ther., 2017, 25, 465 CrossRef CAS PubMed.
- R. Tamura, S. Uemoto and Y. Tabata, Inflammation Regener., 2016, 36, 26 CrossRef PubMed.
- M. M. S. Abbass, A. A. El-Rashidy, K. M. Sadek, S. E. Moshy, I. A. Radwan, D. Rady, C. E. Dörfer and K. M. Fawzy El-Sayed, Polymers, 2020, 12, 2935 CrossRef CAS PubMed.
- S. Oe, Y. Fukunaka, T. Hirose, Y. Yamaoka and Y. Tabata, J. Controlled Release, 2003, 88, 193 CrossRef CAS PubMed.
- S. Mardpour, M. H. Ghanian, H. Sadeghi-Abandansari, S. Mardpour, A. Nazari, F. Shekari and H. Baharvand, ACS Appl. Mater. Interfaces, 2019, 11, 37421 CrossRef CAS PubMed.
- K. Wang, R. N. Dong, J. Z. Tang, H. C. Li, J. L. Dang, Z. X. Zhang, Z. Yu, B. L. Guo and C. G. Yi, Chem. Eng. J., 2022, 430, 132664 CrossRef CAS.
- B. Huang, X. Cheng, H. Wang, W. Huang, Z. la Ga Hu, D. Wang, K. Zhang, H. Zhang, Z. Xue, Y. Da, N. Zhang, Y. Hu, Z. Yao, L. Qiao, F. Gao and R. Zhang, J. Transl. Med., 2016, 14, 45 CrossRef PubMed.
- Y. Lin, M. Yan, Z. Bai, Y. Xie, L. Ren, J. Wei, D. Zhu, H. Wang, Y. Liu, J. Luo and X. Li, J. Nanobiotechnol., 2022, 20, 432 CrossRef CAS PubMed.
- Y. Watanabe, A. Tsuchiya and S. Terai, Clin. Mol. Hepatol., 2021, 27, 70 CrossRef PubMed.
- X.-J. Song, L. Zhang, Q. Li, Y. Li, F.-H. Ding and X. Li, Life Sci., 2021, 265, 118821 CrossRef CAS PubMed.
- J. Xu, P. Chen, C. Yu, Q. Shi, S. Wei, Y. Li, H. Qi, Q. Cao, C. Guo, X. Wu and G. Di, FASEB J., 2022, 36, e22553 CAS.
- S. Tian, X. Zhou, M. Zhang, L. Cui, B. Li, Y. Liu, R. Su, K. Sun, Y. Hu, F. Yang, G. Xuan, S. Ma, X. Zheng, X. Zhou, C. Guo, Y. Shang, J. Wang and Y. Han, Stem Cell Res. Ther., 2022, 13, 330 CrossRef CAS PubMed.
- C. Zhu, L. Li, Z. Wang, M. Irfan and F. Qu, Biosens. Bioelectron., 2020, 160, 112213 CrossRef CAS PubMed.
- D. K. Jeppesen, A. M. Fenix, J. L. Franklin, J. N. Higginbotham, Q. Zhang, L. J. Zimmerman, D. C. Liebler, J. Ping, Q. Liu, R. Evans, W. H. Fissell, J. G. Patton, L. H. Rome, D. T. Burnette and R. J. Coffey, Cell, 2019, 177, 428 CrossRef CAS PubMed.
- R. Kalluri and V. S. LeBleu, Science, 2020, 367, 23 CrossRef PubMed.
- C. Emanueli, A. I. U. Shearn, G. D. Angelini and S. Sahoo, Vasc. Pharmacol., 2015, 71, 24 CrossRef CAS PubMed.
- C. M. Fader, D. G. Sánchez, M. B. Mestre and M. I. Colombo, Biochim. Biophys. Acta, 2009, 1793, 1901 CrossRef CAS PubMed.
- F. Qadir, M. A. Aziz, C. P. Sari, H. Ma, H. Dai, X. Wang, D. Raithatha, L. G. L. Da Silva, M. Hussain, S. P. Poorkasreiy, I. L. Hutchison, A. Waseem and M.-T. Teh, Mol. Cancer, 2018, 17, 97 CrossRef PubMed.
- L. Urbanelli, A. Magini, S. Buratta, A. Brozzi, K. Sagini, A. Polchi, B. Tancini and C. Emiliani, Genes, 2013, 4, 152 CrossRef PubMed.
- S. J. Forbes and P. N. Newsome, J. Hepatol., 2012, 56, 496 CrossRef PubMed.
- D. K. Gupta, S. Sharma, P. Venugopal, L. Kumar, S. Mohanty and S. Dattagupta, Transplant. Proc., 2007, 39, 700 CrossRef CAS PubMed.
- A. Trounson and C. McDonald, Cell Stem Cell, 2015, 17, 11 CrossRef CAS PubMed.
- Y. Jin, J. Wang, H. Li, S. Gao, R. Shi, D. Yang, X. Wang, X. Wang, L. Zhu, X. Wang, C. Chen, K. Ning, Z. Gao, J. Xu and Q. Fu, EBioMedicine, 2018, 34, 231 CrossRef PubMed.
- D. A. Borrelli, K. Yankson, N. Shukla, G. Vilanilam, T. Ticer and J. Wolfram, J. Controlled Release, 2018, 273, 86 CrossRef CAS PubMed.
- D. Erdogan, O. R. C. Busch, D. J. Gouma and T. M. van Gulik, Liver Int., 2009, 29, 175 CrossRef PubMed.
- M. Huang, J. Jiao, H. Cai, Y. Zhang, Y. Xia, J. Lin, Z. Shang, Y. Qian, F. Wang, H. Wu, X. Kong and J. Gu, Hepatology, 2022, 76, 1706 CrossRef CAS PubMed.
- Y. Tang, S. Lin, S. Yin, F. Jiang, M. Zhou, G. Yang, N. Sun, W. Zhang and X. Jiang, Biomaterials, 2020, 232, 119727 CrossRef CAS PubMed.
- K. Fukushima, H. Tanaka, P. Kadaba Srinivasan, K. Pawlowsky, B. Kögel, S. Uemoto, Y. Ku and R. H. Tolba, Eur. Surg. Res., 2018, 59, 48 CrossRef CAS PubMed.
- C. Fan, J. Fu, W. Zhu and D.-A. Wang, Acta Biomater., 2016, 33, 51 CrossRef CAS PubMed.
- Z. Li, Y. Zhao, X. Ouyang, Y. Yang, Y. Chen, Q. Luo, Y. Zhang, D. Zhu, X. Yu and L. Li, Bioact. Mater., 2022, 11, 41 CAS.
- E. Zhang, B. Song, Y. Shi, H. Zhu, X. Han, H. Du, C. Yang and Z. Cao, Proc. Natl. Acad. Sci. U. S. A., 2020, 117, 32046 CrossRef CAS PubMed.
- W. Xia, G. Lai, Y. Li, C. Zeng, C. Sun, P. Zhang, G. Zhu, L. Li and L. Wu, Front. Bioeng. Biotechnol., 2023, 11, 1170212 CrossRef PubMed.
- X. Yan, T. Sun, Y. Song, W. Peng, Y. Xu, G. Luo, M. Li, S. Chen, W.-W. Fang, L. Dong, S. Xuan, T. He, B. Cao and Y. Lu, Nano Lett., 2022, 22, 2251 CrossRef CAS PubMed.
- X. Wei, C. Cui, C. Fan, T. Wu, Y. Li, X. Zhang, K. Wang, Y. Pang, P. Yao and J. Yang, Int. J. Biol. Macromol., 2022, 199, 401 CrossRef CAS PubMed.
- L. Xia, S. Wang, Z. Jiang, J. Chi, S. Yu, H. Li, Y. Zhang, L. Li, C. Zhou, W. Liu and B. Han, Carbohydr. Polym., 2021, 264, 117965 CrossRef CAS PubMed.
- P. Qi, Y. G. Zheng, S. Ohta, N. Kokudo, K. Hasegawa and T. Ito, ACS Biomater. Sci. Eng., 2019, 5, 4790 CrossRef CAS PubMed.
- T. Kisseleva and D. Brenner, Nat. Rev. Gastroenterol. Hepatol., 2021, 18, 151 CrossRef PubMed.
- D. G. You, B. H. Oh, V. Q. Nguyen, G. T. Lim, W. Um, J. M. Jung, J. Jeon, J. S. Choi, Y. C. Choi, Y. J. Jung, J. Lee, D.-G. Jo, Y. W. Cho and J. H. Park, J. Controlled Release, 2021, 336, 285 CrossRef CAS PubMed.
- Y. Li, B.-w Liu, W.-l Du, Z.-c Song, Q. Zhao, L.-q Fan, J.-q Yang, Q.-j Li, M.-x Wang, Z.-k Jiao and Z.-d Zhang, Zhonghua Zhongliu Zazhi, 2004, 26, 638 Search PubMed.
- S. Mardpour, M. H. Ghanian, H. Sadeghi-Abandansari, S. Mardpour, A. Nazari, F. Shekari and H. Baharvand, ACS Appl. Mater. Interfaces, 2019, 11, 37421 CrossRef CAS PubMed.
- K. H. Hussein, K.-M. Park, L. Yu, H.-H. Kwak and H.-M. Woo, Mater. Sci. Eng., C, 2020, 116, 111160 CrossRef CAS PubMed.
- Y. Zhao, B. Xu, W. Liang, Y. Ding, J. Li, Y. Zhang, F. Xu, H. Zhou and Y. Xu, Tissue Eng., Part A, 2019, 25, 1167 CrossRef CAS PubMed.
- E. Pishavar, H. Luo, M. Naserifar, M. Hashemi, S. Toosi, A. Atala, S. Ramakrishna and J. Behravan, Int. J. Mol. Sci., 2021, 22, 6203 CrossRef CAS PubMed.
- D. P. Nair, M. Podgorski, S. Chatani, T. Gong, W. Xi, C. R. Fenoli and C. N. Bowman, Chem. Mater., 2014, 26, 724 CrossRef CAS.
- J. Pupkaite, J. Rosenquist, J. Hilborn and A. Samanta, Biomacromolecules, 2019, 20, 3475 CrossRef CAS PubMed.
- J. Jang, J. Y. Park, G. Gao and D.-W. Cho, Biomaterials, 2018, 156, 88 CrossRef CAS PubMed.
- E. Goulart, Methods Mol. Biol., 2023, 2575, 269 CrossRef PubMed.
- A. Faulkner-Jones, C. Fyfe, D.-J. Cornelissen, J. Gardner, J. King, A. Courtney and W. Shu, Biofabrication, 2015, 7, 044102 CrossRef PubMed.
- E. Goulart, L. C. de Caires, K. A. Telles-Silva, B. H. S. Araujo, S. A. Rocco, M. Sforca, I. L. de Sousa, G. S. Kobayashi, C. M. Musso, A. F. Assoni, D. Oliveira, E. Caldini, S. Raia, P. I. Lelkes and M. Zatz, Biofabrication, 2020, 12, 015010 CrossRef CAS PubMed.
- D. Huang, S. B. Gibeley, C. Xu, Y. Xiao, O. Celik, H. N. Ginsberg and K. W. Leong, Adv. Funct. Mater., 2020, 30, 1909553 CrossRef CAS PubMed.
- A. M. Carvalho, R. Bansal, C. C. Barrias and B. Sarmento, Adv. Mater., 2024, 36, 2307673 CrossRef CAS PubMed.
- Y. Ai, F. Dai, W. Li, F. Xu, H. Yang, J. Wu, K. Yang, L. Li, F. Ai and L. Song, Mater. Today Bio, 2023, 23, 100882 CrossRef CAS PubMed.
- K. Yue, G. Trujillo-de Santiago, M. M. Alvarez, A. Tamayol, N. Annabi and A. Khademhosseini, Biomaterials, 2015, 73, 254 CrossRef CAS PubMed.
- L. Xu, J. Mu, Z. Ma, P. Lin, F. Xia, X. Hu, J. Wu, J. Cao, S. Liu, T. Huang, D. Ling, J. Gao and F. Li, ACS Appl. Mater. Interfaces, 2023, 15, 37193 CrossRef CAS PubMed.
- X. Ke, M. Li, X. Wang, J. Liang, X. Wang, S. Wu, M. Long and C. Hu, Carbohydr. Polym., 2020, 229, 115516 CrossRef CAS PubMed.
- C. Hua, J. Liu, X. Hua and X. Wang, Dose-Response, 2020, 18, 1559325820941323 CrossRef CAS PubMed.
- P. Maghsoudlou, F. Georgiades, H. Smith, A. Milan, P. Shangaris, L. Urbani, S. P. Loukogeorgakis, B. Lombardi, G. Mazza, C. Hagen, N. J. Sebire, M. Turmaine, S. Eaton, A. Olivo, J. Godovac-Zimmermann, M. Pinzani, P. Gissen and P. De Coppi, PLoS One, 2016, 11, e0155324 CrossRef PubMed.
- B. Wang, W. Li, D. Dean, M. K. Mishra and K. S. Wekesa, J. Biomed. Mater. Res., Part A, 2018, 106, 829 CrossRef CAS PubMed.
- T. T. Lau, L. Q. P. Lee, W. Leong and D.-A. Wang, Biomed. Mater., 2012, 7, 065003 CrossRef CAS PubMed.
- R. P. H. Meier, E. Montanari, P. Morel, J. Pimenta, H.-J. Schuurman, C. Wandrey, S. Gerber-Lemaire, R. Mahou and L. H. Bühler, Methods Mol. Biol., 2017, 1506, 259 CrossRef CAS PubMed.
- J. Li and D. J. Mooney, Nat. Rev. Mater., 2016, 1, 16071 CrossRef CAS PubMed.
- Z.-K. Cui, S. Kim, J. J. Baljon, B. M. Wu, T. Aghaloo and M. Lee, Nat. Commun., 2019, 10, 3523 CrossRef PubMed.
- A. Mellati, E. Hasanzadeh, M. Gholipourmalekabadi and S. E. Enderami, Mater. Sci. Eng., C, 2021, 131, 112489 CrossRef CAS PubMed.
- X. Lin, C. T. Tsao, M. Kyomoto and M. Zhang, Adv. Healthcare Mater., 2021, e2101479, DOI:10.1002/adhm.202101479.
- R. Yang, J. Huang, W. Zhang, W. Xue, Y. Jiang, S. Li, X. Wu, H. Xu, J. Ren and B. Chi, Carbohydr. Polym., 2021, 273, 118607 CrossRef CAS PubMed.
- L. Kaps, L. Nuhn, M. Aslam, A. Brose, F. Foerster, S. Rosigkeit, P. Renz, R. Heck, Y. O. Kim, I. Lieberwirth, D. Schuppan and R. Zentel, Adv. Healthcare Mater., 2015, 4, 2809 CrossRef CAS PubMed.
|
This journal is © The Royal Society of Chemistry 2024 |