DOI:
10.1039/D3MA00733B
(Review Article)
Mater. Adv., 2024,
5, 3113-3134
Harnessing osteoimmunity to treat peri-implant inflammatory osteolysis†
Received
19th September 2023
, Accepted 26th February 2024
First published on 13th March 2024
Abstract
Osteoimmunity is an interactive schema by which organisms maintain bone homeostasis. It permeates bone remodeling in healthy or diseased states, such as osseointegration of an oral implant material–alveolar bone socket or inflammatory osteolysis at the plaque-oral tissue–implant material interface. Once the self-mobilized osteoimmune system is insufficient to resist the spread of infection or inflammation caused by progressive periodontitis, smoking, or diabetes, peri-implant bone homeostasis will be tilted toward inflammatory osteolysis, as evidenced by an inflammatory response, osteoblast suppression, and osteoclast hyperactivity. Therefore, in addition to etiological treatment, harnessing osteoimmunity to enhance bone homeostasis stability and tissue anti-inflammatory capacity has also become a therapeutic approach for peri-implantitis. Here, we extract therapeutic targets in terms of cellular, protein, epigenetic modification, ribonucleic acid (RNA), and podosome-related osteoimmune mechanisms in inflammatory osteolysis, summarize the existing bioactive materials, implant surface modification, extracellular vesicles and other bioengineering techniques using osteoimmunity in the treatment of inflammatory bone loss, and look at potential targets for harnessing osteoimmunotherapy in peri-implantitis.
1. Introduction
Osteoimmunity involves interactions between the skeletal and immune systems to regulate the bone microenvironment. The balance between the skeletal and immune systems is disturbed in the face of sudden implant placement in a traumatic manner, which inevitably imposes an osteoimmune burden. Under normal circumstances, osteoimmunity can tolerate and develop a bone shield-off reaction in the form of interface bone.1 Once the stimulus exceeds the osteoimmunity tolerable range, rejection reactions such as marginal bone loss will progress until the osteoimmunity burden subsides.2 Therefore, it has been argued that implants that remain stable in the alveolar bone which is a lifelong remodeling part of the skeletal system and consistently suffering occlusal stress should not be considered inert biomaterials. Instead, they should be classified as osteoimmunomodulatory and co-participate in the process of alveolar bone remodeling.3 If bacterial plaque microorganisms cause hyperimmune rejection, the implant will transition from being osteoimmunomodulatory to being targeted by the immune system. This may lead to inflammation and osteolysis around the implant. Unlike inflammatory bone metabolic diseases caused by autoimmune disorders, periodontitis or peri-implantitis begins with polymicrobial synergy and dysbiosis.4 Additionally, distinct from periodontitis, osteoclast differentiation-related pathways are relatively more active in peri-implant inflammation, with a higher ratio of a receptor activator of a nuclear factor kappa-B (NF-κB) ligand (RANKL)/osteoprotegerin (OPG).5 Classical osteolysis begins with the induction of mononuclear macrophages by RANKL and a macrophage colony-stimulating factor (M-CSF), followed by a gradual process of cell fusion and cytoskeletal reorganization to achieve osteoclast maturation and subsequent acquisition of bone resorption capacity.6 During inflammation, tumor necrosis factor (TNF) and interleukin-6 (IL-6) can contribute to osteoclast differentiation even without RANKL. This mechanism appears to be specific to inflammatory osteolysis.7 In addition to the direct role of the RANKL-RANK-OPG axis, bone homeostasis is also indirectly and synergistically regulated by immune cells, such as macrophages, dendritic cells, and neutrophils.8,9 In conclusion, inflammatory factors secreted by immune cells aggravate the progression of infectious peri-implantitis. The process continues until inflammation subsides and bone homeostasis is restored. After this, osteoimmunity continues to drive bone repair and re-establish bone homeostasis.
To prevent peri-implant inflammatory osteolysis, intact and healthy soft-tissue integration is the first barrier.10 Signals from an impaired soft-tissue barrier can elicit an osteoimmune response. The implant itself may also induce an autoimmune response. Implant-derived titanium ions may enhance the Toll-like receptor 4 (TLR4)-mediated inflammatory response and increase the RANKL-OPG ratio, leading to inflammatory osteolysis.11 Unlike reactive oxygen species (ROS)-induced cellular inflammation, titanium dioxide particle-induced inflammation12,13 does not depend on reactive oxygen species but on the acetylation of histone H3 lysine 56 (H3K56), causing DNA damage (early DNA damage marker – elevated γH2AX).14 Any biomaterial that wishes to act on the host to cure or ameliorate a disease faces the threat of autoimmune rejection. It is essential to modulate the host's immune microenvironment in response to the biomaterial and harness it toward an ideal, favorable, and stable bone microenvironment, which is also called “osteoimmunomodulation” of bone regeneration.15 Bioactive materials such as polymers, ceramics, metals, monolithic architected composites, microporous nanoparticles, fibrous hydrogel networks, and 3D printed scaffolds directly recruit endogenous immune cells and stem cells for tissue healing and regeneration.16 In addition, bioactive materials that mediate bioelectricity also have a modulating effect on osteoimmunity due to the active bioelectrical properties of the bone tissue, such as the piezoelectric, pyroelectric, and ferroelectric charges mediated by collagen fibrils as well as the electro-osmosis and streaming potential present in bone tubules and laminae. The separation of hydrogen bonds between hydroxyapatite (HA) and collagen also plays a role.17 Similarly, the use of implant surface modification and extracellular vesicles can also help in the treatment of osteoimmune-mediated osseointegration to inflammatory immune-mediated osteolysis.
Understanding the causes of inflammatory osteolysis and the unfavorable bone microenvironment in which it occurs is a central aspect of understanding potential targets for treating osseointegration failure. This review provides an overview of advancements made in treating inflammatory osteolysis. It describes the viable approaches and prospects of osteoimmunomodulation in treating peri-implant inflammatory osteolysis regarding implant surface modification, bioactive materials, and extracellular vesicles.
2. Cascade of osteolysis reactions
2.1. The cytological process of osteolysis
There is always a dynamic combination of bone loss and bone regeneration in the bone microenvironment in terms of catabolism and anabolism. The skeletal microenvironment is regulated by the involvement of the skeletal and immune systems through the interaction of bone marrow-derived mesenchymal stem cell-derived osteoblasts and bone marrow-derived hematopoietic stem cell-derived immune cells, which regulate osteogenesis and osteolysis (Fig. 1).
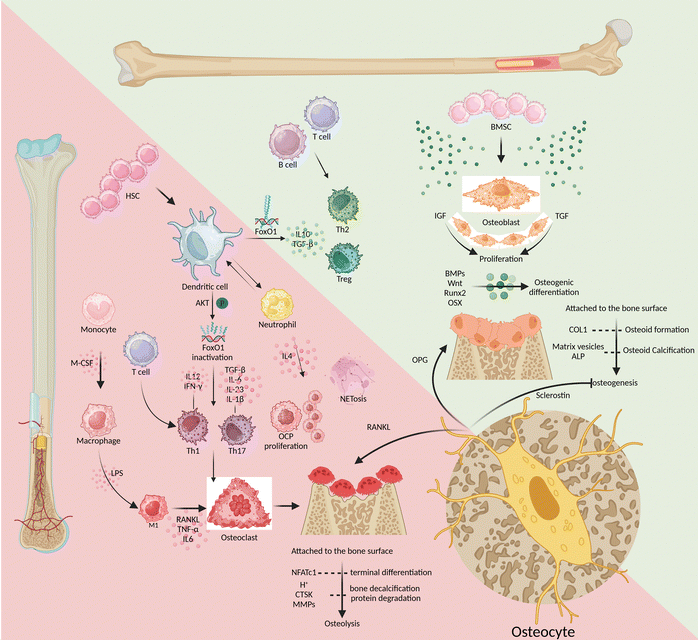 |
| Fig. 1 The triad of bone marrow mesenchymal stem cells, hematopoietic stem cells, and osteocytes regulates the processes of osteolysis and osteogenesis. (Note: the green upper triangular section represents osteogenesis, while the lower red triangular area represents osteolysis.) | |
Bone marrow-derived mesenchymal stem cells
Growth factors such as insulin-like growth factor-I (IGF-I) and transforming growth factor (TGF) promote osteoblast proliferation. Meanwhile, the bone formation protein (BMP) and Wnt signaling-mediated transcription factors, like Runt-related transcription factor 2 (Runx2) and osterix (OSX), promote osteogenic differentiation.18 As bone formation progresses, mature osteoblasts adhere to the surface of the bone matrix and produce protein-like type I collagen (COL1). This contributes to osteoid formation, which is then calcified through the secretion of matrix vesicles and alkaline phosphatase (ALP).18 The integrity of the mature bone structure is maintained through the process of osteoclastic resorption and osteoblastic reconstruction, which involves the continuous development of both osteoblasts and osteoclasts. M-CSF and RANKL turn on the differentiation of monocyte-macrophages toward osteoclast differentiation, while the nuclear factor of activated T cells 1 (NFATc1) controls the terminal differentiation of osteoclasts.19 As they mature, osteoclasts release both protons (H+) and enzymes that break down the bone matrix, including cathepsin K (CTSK) and matrix metalloproteinases (MMPs). These processes lead to the decalcification of bones and the degradation of proteins. Osteogenic inhibition and osteoclast induction are mainly triggered by two substances secreted by osteocytes: sclerostin and RANKL, respectively.20 After being cocultured with osteocytes, osteoclast precursor cells displayed more powerful ability to induce osteoclasts. Specifically, their ability to express RANKL was much higher than that of osteoclasts cocultured with osteoblasts, up to tens of times higher.21 In the maturation phase of bone growth, osteocytes have a greater advantage in OPG expression than osteoblasts.22 This makes osteocytes incredibly important in the bone microenvironment as they regulate the balance between osteogenesis and osteolysis. There is a lack of studies that target osteocytes to induce osteogenesis during the early stages of osseointegration and osteoclastogenesis during bone remodelling. Such temporal and sequential targeting of osteocytes for the regulation of bone homeostasis would be a major challenge.
Bone marrow-derived hematopoietic stem cells
Among immune cells, T lymphocytes express minimal levels of RANKL and have little involvement in osteolysis, similar to B lymphocytes.23–25 However, the absence of T/B lymphocytes can negatively impact bone health. This is because B lymphocytes produce less IL10, which reduces the bone microenvironment's ability to repair inflammation effectively.26 On the other hand, patients with periodontitis had a significantly higher percentage of CD4+ T helper (Th) cells, such as Th1 and Th17 cells, accompanied by a high expression of inflammatory infiltrative factors and an increase in the number of osteoclasts.27,28 Similarly, research has found that IL-6 can significantly promote the differentiation and maturation of dendritic cells (DCs), which can ultimately contribute to the progression of periodontitis.29 Mature DCs bidirectionally regulate bone homeostasis, eliciting osteoprotective and osteodestructive responses by activating and inactivating FoxO1, respectively.8 DCs help in activating Th1 cells by releasing IL-12 and interferon-γ (IFN-γ), and they promote the differentiation of Th17 cells by releasing TGF-β, IL-6, IL-23, and IL-1β. These cascades of amplified signals continue to sustain the inflammatory response and osteoclastic induction in response to RANKL activation.30–32 Alternatively, DCs can induce regulatory T-cell (Treg) responses by secreting IL-10 and TGF-β to resist the further development of inflammatory osteolysis.33 Triggered by Porphyromonas gingivalis (Pg) derived LPS, neutrophils overexpress IL-4, with consequent initiation of IL4/IL4R/ERK signaling in CD115(+) osteoclast precursors (OCPs) to promote their proliferation and subsequent osteolysis.34,35 When phagocytes remove exogenous inflammatory factors, the produced extracellular traps (NETs) are susceptible to mineralization of dental calculus, further stimulating inflammation and osteolysis. NETs secreted by neutrophils mediate TLR4 signaling and NET-associated proteins, including histones and neutrophil elastase, to induce rapid osteoclastogenesis, especially when NETs are carbamylated.9
In conclusion, in response to stimulation by inflammatory factors such as the LPS, antigen-presenting cells like DCs activate the proliferation and differentiation of Th1 and Th17 cells. This is followed by clearance of inflammatory stimuli by trending neutrophils and macrophages but also inevitable by tissue destruction due to high expression of RANKL and MMPs. At the same time as hyperinflammation, Th2 cells and Tregs also increase in number, differentiate, and release anti-inflammatory factors like IL-10. This helps to mitigate the harm caused by Th1 and Th17 cells and promotes the healing of damaged tissues. Targeted modulation of immune cells is not a substitute for etiological treatment that removes exogenous antigens, but rather a way to assist in the amplification of intrinsic autoimmunity. Enhancing the efficiency of antigen presentation and antigen clearance, while directing the body towards tissue repair as soon as possible, will be the desired efficacy of immunomodulation in peri-implantitis.
2.2. Protein regulation of osteolysis
RANKL-OPG competition is the most direct and critical aspect of regulating osteogenesis and osteolysis. The development of osteoclasts and subsequent bone resorption are hindered by RANKL inhibitors.36 The lack of OPG (an inhibitor of bone resorption that antagonizes RANKL-rank binding) will bring about bone loss so severe that it becomes one of the criteria for modeling spontaneous alveolar bone loss.37 Based on this theory, denosumab is a competitive antagonist of RANKL that can effectively inhibit osteoporosis or tumor bone metastasis. However, in some patients, it may significantly increase the risk of osteonecrosis of the jaw after tooth extraction.38–40 This may be related to the inhibition of microvascular and immune mobilization by bisphosphonate. After partial elimination of inflammatory factors (inhibition of TNF-α, IL-1α/β, or IL-6), however, the progression of osteonecrosis can be significantly inhibited.41 Thus, the role of osteoimmunity in bone protection is not only to maintain the osteoblast–osteoclast balance but also to activate osteoclasts to remove necrotic areas while suppressing inflammation to promote bone tissue repair during inflammatory infections (Fig. 2).
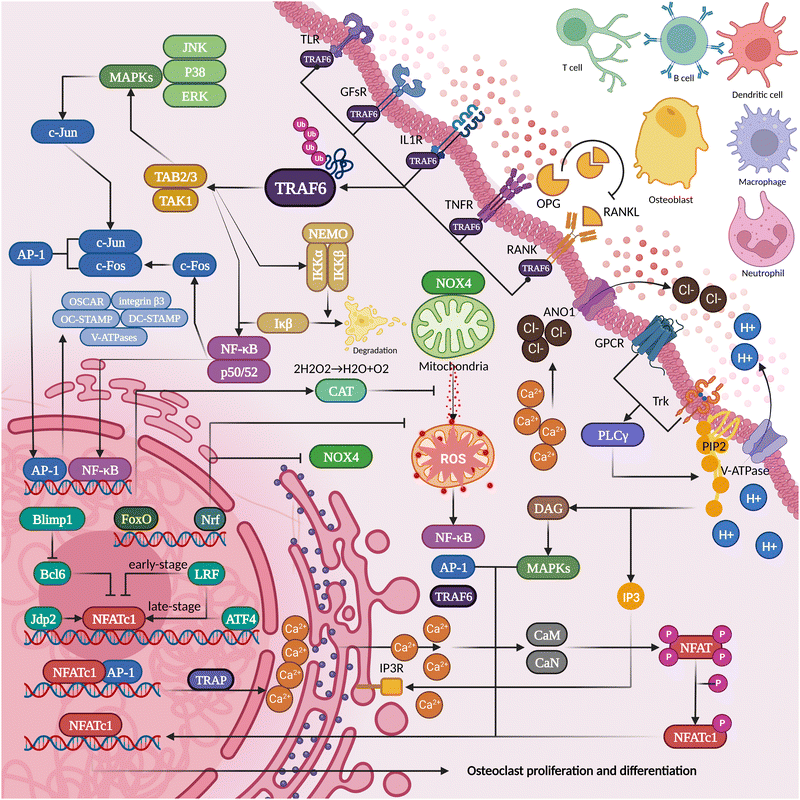 |
| Fig. 2 The proteins secreted and pericytes present in the inflammatory osteolysis microenvironment can potentially influence the differentiation of osteoclasts. | |
Role of MAPK/NF-κB/c-Jun downstream of TRAF6 in the cytoplasm.
In addition to RANK receptors, activation of Toll-like receptors (TLRs), IL1R, TNFR, and growth factor receptors (GFsRs) also synergizes with the induction of inflammatory osteolysis by RANKL.42 Upon activation of RANK signaling, TNF receptor-associated factor 6 (TRAF6) autoubiquitinate to form nondegradative K63-linked ubiquitin chains, which recruit TGF-activated kinase 1 (TAK1)–TAK1-binding protein 1/2 (TAB1/2) complex formation.43 RANKL-induced osteoclast differentiation is dependent on the activation of TRAF6 and TAK1, the deletion of which results in impaired activation of mitogen-activated protein kinases (MAPKs) and NF-κB.44,45 In the MAPK signaling pathway, the c-Jun N-terminal kinase (JNK) protects osteoclast precursor cells from RANKL-induced apoptosis during differentiation and facilitates the formation and differentiation of RANKL-activated osteoclasts.46 The inhibition of p38 interfered with osteoclast formation but had a little effect on the bone resorption effect of osteoclasts.47 The osteoclast-inducing effect of MAPK extracellular signaling-regulated kinase (ERK) (MEK) remains controversial.48,49 Furthermore, when IKKγ is lacking, the transcription factor NF-κB is unable to enter the nucleus, hindering the expression of genes related to osteoclast formation.50 In the absence of JunB, there is a decrease in the number of osteoclasts, and this reduction is even more pronounced when c-Fos is also lacking.51,52 Reductions in both NF-κB and activator protein 1 (AP-1) (composed of c-Fos and Jun proteins) in osteoclasts lead to osteosclerosis. Inhibition of cytoplasmic proteins downstream of TRAF6 may cause alterations in many cellular functions. Direct inhibition of TRAF6 or cell membrane proteins may have more immediate efficacy, but the end points of the inhibitory effect need to be clarified to avoid toxic side effects of treatment.
Regulation of proteins in the nucleus.
Osteoclast formation and survival are inextricably linked to the osteoclast-associated receptor (OSCAR), which is dependent on the transcriptional induction of the AP-1 complex.53 Additionally, the AP-1 complex promotes the formation of bone resorption structures (actin rings and ruffled membranes) through the activation of integrin β3.54 Furthermore, the AP-1 complex contributes to the fusion of osteoclast precursor cells through the activation of the dendritic cell-specific transmembrane protein (DC-STAMP), osteoclast stimulatory transmembrane protein (OC-STAMP), and vacuolar-ATPase subunit d2 (V-ATPase).55–57 Osteoclastic differentiation requires NFATc1 as an essential component. Its activation relies on TRAF6, c-Fos, and p50/p52 NF-κB.58 Additionally, NFATc1 is responsible for mediating activating transcription factor 4 (ATF4), B lymphocyte-induced maturation protein 1 (Blimp1), leukemia/lymphoma-related factor (LRF), or Jun dimerization protein (Jdp2) to function.59–63 Moreover, the binding of NFATc1 to the AP-1 complex is also involved in TRAP transcription.64 Secretory TRAP will act as a bone matrix resorber in the acidic environment between osteoclasts and the bone surface. However, immunotherapy against transcription factors also faces toxic side effects caused by a wide range of downstream regulatory sites. Addressing this issue is a major challenge.
Mitochondrial oxidative stress.
Induced by inflammatory and osteolytic stimuli, nicotinamide adenine dinucleotide phosphate (NADPH) oxidase 4 (NOX4) in the mitochondrial electron transport chain generates large amounts of ROS and exacerbates the induction of inflammation and osteolytic differentiation by the transcription factors AP-1 (c-Jun/c-Fos), NF-κB and NFATc1.65–67 The transcription factor nuclear factor-erythroid 2-related factor 2 (Nrf2) is a negative regulator of NOX4, preventing further development of oxidative stress and osteoclasts.68 Meanwhile, the transcription factor FoxO induces catalase (CAT). This helps in regulating the excessive production of ROS and plays a negative regulatory role in hyperactive osteoclast differentiation.69 Therefore, the development of mitochondrial-targeted drugs for Nrf2 and FoxO facilitates the stabilisation of the mitochondrial respiratory transport electron chain and the control of ROS overexpression, which is beneficial for the treatment of osteoblastic hyperactivity.
Calcium ion effect.
In addition, RANKL-induced osteolysis is inseparable from Ca2+ migration. The G protein-coupled receptor (GPCR) and tyrosine kinase receptor (Trk) catalyze the hydrolysis of membrane phospholipid phosphatidylinositol4,5 bisphosphate (PIP2) upon inflammatory stimulation to produce diacylglycerol (DAG) and inositol triphosphate (IP3). DAG induces MAPK signaling to enhance c-Fos and NF-κB expression. IP3 induces Ca2+ release from the endoplasmic reticulum, which subsequently activates the dephosphorylation of NFAT by calcineurin (CaN). As a result, NFAT can move into the nucleus and regulate genes on its own or in conjunction with transcription factors like AP-1.70–72 Additionally, in response to RANKL–RANK signaling, the calcium-activated chloride channel anoctamin 1 (Ano1) upregulates NFATc1 expression in response to intracellular Ca2+ activation and releases chloride ions that act in concert with the proton pump (H+) for bone resorption.73 The development of drugs targeting inhibition of the GPCR and Trk or downstream IP3 might be one of the feasible ways to inactivate intracellular calcium signalling and thus inhibit bone resorption.
2.3. Molecular modification of osteolysis
Upstream of the protein regulation of bone homeostasis, the surfaces of DNA, RNA, and histones play a role in signal transduction through modifications such as acetylation and methylation. Differences in the regulation of epigenetic modifications can be identified through comparisons between healthy and diseased tissues. Histone deacetylases (HDACs) 1, 5, 8, and 9 and histone demethylase lysine demethylase 4B (KDM4B) were highly expressed in the gingival tissue of patients with periodontitis.74 Meanwhile, HDAC inhibitors (HDACi) positively affect bone regeneration in periodontal tissues. Inflammatory osteolysis induced by Pg bacteria achieved bone regeneration with HDACi treatment, especially inhibitors of class I and class II HDACs.75 In addition, the histone methyltransferase enhancer of zeste homolog 2 (EZH2) was able to upregulate the expression of VCAM-1, which leads to the inhibition of miR-101 expression and subsequently suppresses periodontal inflammatory bone resorption.76 Drugs targeting molecular modifications may hold promise for assisting bone immunomodulation.
Osteogenesis.
Epigenetic modifications can tilt bone homeostasis toward osteogenesis. HDAC1 and HDACi2 are important regulators of osteogenic differentiation, with upregulation of osteogenic markers other than OCN.77 Additionally, HDAC1 targets the promoters of genes responsible for the osteoclast function (e.g., NFATc1 and OSCAR) to impede their expression and suppress osteoclast differentiation.78 Reducing the expression of HDACs 4, 5, 9, 10, and 11 leads to an increase in the size and demineralization activity of multinucleated giant cells. However, it has little impact on the total number of multinucleated giant cells formed.79 HDAC6 is crucial in destabilizing the osteoclast skeleton, hindering osteoclast migration, and preventing the formation of podosomes.80 HDAC7 inhibited the transcription of NFATc1, CTSK, and DC-STAMP to prevent osteoclast differentiation, but it also inhibited the maturation of osteoblasts.81 RANKL helps in keeping FoxO in an inactive state through pathways like phosphorylation. Meanwhile, sirtuin 1 (SIRT1) activates FoxO by deacetylating it and also reduces the levels of H2O2. This inhibits the increase and differentiation of osteoclasts.82 It was found that SIRT3 plays a role in maintaining mitochondrial homeostasis by deacetylating cytochrome c oxidase subunit 4 isoform 2 (COX4I2).83 Additionally, SIRT6 was found to deacetylate STAT5, which inactivates IL-15/JAK3/STAT5.84 These actions serve as negative regulators of the inflammatory response and RANKL-induced osteolysis. In osteoblasts, lysine-specific demethylase (LSD1) inhibits PBX1 translation to promote osteoblast differentiation. It positively regulates miR-6881-3p, which targets the inhibition of pre-B-cell leukemia homeobox 1 (PBX1) to promote osteogenic differentiation and the inhibition of additional sex combs like-2 (ASXL2) to inhibit osteoclastic differentiation.85 Inhibition of the KDM4B expression causes compensatory activation of KDM1A, reducing the production of proinflammatory factors and osteoclasts.74 Methyltransferase-like 3 (METTL3), the core methyltransferase that installs an N6-methyladenosine (m6A) modification on RNA, stabilizes the mitochondrial function by reducing the iNOS/NO expression, thereby inhibiting osteolysis under inflammatory conditions.86 The above studies suggest that epigenetic modifications are feasible for the treatment of inflammatory osteolysis.
Osteolysis.
Similarly, epigenetic modifications can tilt bone homeostasis toward osteolysis. HDAC3 promotes osteoclast differentiation, and its reduced expression can increase the induction of osteogenic differentiation.87,88 The p300/CREB-binding protein-associated factor (PCAF) induces osteolysis by acetylating H3K27 in the C–X–C motif chemokine ligand 12 (CXCL12) promoter region in osteoblasts.89 It also acetylates NFATc1 in osteoclasts and runt-related transcription factor 2 (Runx2) in osteoblasts to simultaneously induce osteoclast differentiation and inhibit osteogenic differentiation.90 The PCAF also activates Wnt5a to promote osteolysis.91 Differential recruitment of the histone H3K4 methyltransferase SET domain containing 1A (SETD1A) leads to differential polarization of macrophages and osteoclasts.92 Enhancement of DNA methyltransferase will silence osterix (Osx) and prevent osteogenic differentiation.93 The euchromatic histone lysine methyltransferase 2 (EHMT2) monomethylates H3K27 to H3K27me1, which recruits MMP9 for proteolysis of the histone H3N-terminal tail (H3NT), thereby triggering osteoclast-specific gene expression.94 EZH2 catalyzes the trimethylation of H3K27, which targets the Ndrg2 promoter to inhibit osteoblast differentiation. It also promotes osteoclast differentiation, further exacerbating bone destruction.95 Disruptor of the telomeric silencing 1-like protein (DOT1L), which catalyzes the trimethylation of H3K79, increases the level of ROS in osteoclasts and the ability of osteoclasts to fuse and osteolysis.96 RANKL-induced osteoclast differentiation activates protein arginine methyltransferase (PRMT1/5) expression in a JNK-dependent manner, which methylates NF-κB and induces osteoclastic formation and bone resorption.97,98 The H3K27 demethylase Jumonji domain-containing 3 (Jmjd3), activated at the transcriptional start site of NFATc1, responds to RANKL-stimulated osteoclastic induction by demethylating H3K27me3.99 In osteoclasts, LSD1 demethylates H3K4 and H3K9 to stabilize HIF-α, promoting osteolysis during RANKL-mediated osteoclast differentiation. However, LSD1 inhibitors do not affect methylation levels of H3K4 and H3K9 during the inhibition of osteolysis.100,101 Upon stimulation of the receptor activator of the NF-κB ligand, the KDM4B-CCAR1-MED1 complex is localized to the promoters of multiple genes related to osteoclasts. This complex induces the formation of osteoclasts through the process of H3K9 demethylation. It leads to NF-κB p65 recruitment via a direct interaction between KDM4B and p65.102 N6-methyladenosine (m6A) demethylase fat mass and obesity-associated protein (FTO) overexpression contribute to the transcription factor NF-κB. This leads to an increase in the expression of osteoclast genes like NFATc1 when stimulated by RANKL.103 The RNA methyltransferases METTL16 and FTO are osteodestructive factors, while YTHDF2, a recognition protein of m6A, is an osteoprotective factor.104 But the lack of specificity for the gene site is the fatal weakness of epigenetic drugs.
2.4. RNA mechanism of osteolysis
Differences in RNA expression were found in oral fluid biomarkers of periodontitis and peri-implantitis. This is of potential value for diagnosing and treating inflammatory osteolysis around teeth or implants. For example, the upregulation of miRNA-142-3p and miRNA-146a is representative in diagnosing periodontitis.105 MicroRNAs, including miR-let-7g and miR-27a, show a significant increase in peri-implantitis cases, contrasting with the dramatic decrease observed in miR-145.106 These findings suggest that these microRNAs could potentially aid in the development of effective treatments for inflammatory osteolysis in the future.
MicroRNAs.
MicroRNAs are involved in osteoclast differentiation in inflammatory osteolysis. The oral inflammatory environment will lead to high expression of hsa-miR-374b-5p. This can then lead to the inhibition of U2AF homology motif kinase 1 (UHMK1), ultimately increasing osteoclastic differentiation.107 The negative regulation of RUNX1 by miR-28 favors osteoclast differentiation, while its deletion causes a decrease in the number of osteoclasts.108 MiR-21 inhibits programmed cell death 4 (Pdcd4) expression, which in turn rescues the inhibition of c-Fos by Pdcd4 and thus promotes inflammatory osteolysis.109 In addition, miR-21 is also involved in the activation of T cells and stimulates their secretion of RANKL.110 MiR-182 targets FoxO3 and mastermind-like transcriptional coactivator 1 (Maml1) to promote TNF-α-induced inflammatory bone resorption but is inhibited by the recombination signal binding protein for the immunoglobulin kappa J region (RBP-J).111
MicroRNAs are also involved in autoimmune repair of inflammatory osteolysis. MiR-29a reduces the secretion of RANKL and CXCL12 by osteoblasts and plays an osteoprotective role.89 MiR-142-3p has a significant inhibitory role in the differentiation of monocytes/macrophages/dendritic cells into osteoclasts. Depending on RANKL activation, miR-142-3p targets and promotes the high expression of protein kinase C-α (PKC-α), which in turn induces the apoptosis of osteoclasts and facilitates the arrest of osteolysis progression.112 Similarly, negative regulation of C–X–C motif chemokine receptor 2 (CXCR2) by miR-155-5p hinders osteoclast differentiation.113 Overexpression of miR-335-5p and miR-218 inhibited inflammatory osteolysis in the alveolar bone.114,115 MiR-1260b inhibits Wnt5a and ATF6β expression, impairing RANKL pathway-mediated osteoclastic activity. Human gingiva-derived MSC (GMSC) derived exosomes containing miR-1260b are beneficial in the treatment of bone loss in periodontitis.116,117 Unlike siRNA and protein targeted drugs, miRNA's mimetics and inhibitors have poor specificity, which also leads to limited clinical applications.
Long noncoding RNAs (lncRNAs).
LncRNA acts as an antagonist of microRNA, inhibiting the binding of microRNA to messenger RNA (mRNA) and exerting immunomodulatory effects. The LncRNA differentiation antagonizing nonprotein coding RNA (DANCR) positively regulates the expression of Jagged1 and promotes osteoclast differentiation.118 The LncRNA noncoding repressor of NFAT (NRON) effectively promotes the nuclear translocation of the NF-κB repressing factor (NKRF), preventing NF-κB from activating the transcription of NFATc1. This can ultimately lead to a reduction in bone resorption for patients with periodontitis.119 It was found that miR-221-5p prevented the activation of NF-κB and the resulting osteoclastic differentiation by CXCR3-CXCL10. However, the osteoclast-specific lncRNA (OC-lncRNA) countered miR-221-5p by sponging it, which led to osteolysis.120 LncRNA metastasis-associated lung adenocarcinoma transcript 1 (MALAT1) competitively binds miR-124 to inactivate the latter's inhibitory effect on NFATc1 expression, thus promoting the development of bone loss in periodontitis.121 Endogenous antagonists of mRNA or miRNA in the development of oral inflammatory bone diseases may be the key to future inflammatory regulation and osteolysis treatment in the oral environment.
2.5. Progression of podosomes to osteolysis
Formation of podosomes.
Podosomes, which function to degrade the extracellular matrix (ECM), are found in cells such as monocytes, macrophages, osteoclasts, and migratory dendritic cells.122–125 It is involved in physiological processes, including cell migration, bone remodeling, and angiogenesis.126–128 Unlike podosomes in macrophages or dendritic cells, which are dispersed on the ventral adhesion side or at the tip of the migration direction, podosomes gradually evolve from clusters and rings to belts (on glass) or sealing zones (on bone) during osteoclast development.129 The β1 and β3 integrins that provide the ECM attachment,130 RhoA-inactivating proteins like p190RHOGAP,131 and reduced local contractility132 constitute the three elements of podosome formation. RhoA knockout mice and corresponding RhoA-deficient primary macrophages exhibit impaired bone resorption and osteoclast induction. On the other hand, high levels of RhoA can activate Akt-mTOR-NFATc1 signaling, leading to the promotion of osteoclast development.133 Phosphatidylinositol 3,4-bisphosphate (PtdIns (3, 4) P2) and PI3K-induced PtdIns (3, 4, 5) P3 accumulate at the podosome formation site and recruit WASP and TKS5 to participate in the formation of the core and cap of the podosome, respectively.134 Myosin IIA, together with regulators such as LSP1, induces the generation of oscillatory protrusions that release hydrolases such as MT1-MMP.135 The podosome has ARP2/3 complex-mediated branched F-actin as its core with a cap-like structure consisting of cross-linked F-actin and bundled F-actin at the head and surrounded by a ring of adhesion proteins attached to the ECM via integrins at the tail. The middle-exposed region is present with the transmembrane protein membrane-type metalloproteinase-1 (MT1-MMP) and cell surface adhesion receptor CD44, which are directly involved in the regulation of ECM degradation by hydrolases.129 With the help of calpain-induced WASP cleavage, the supervillain further completes the degradation of the podosome.136 A disintegrin and metalloproteinase (ADAM) 17 mediates the shedding of membrane-bound cytokines and corresponding membrane proteins. In vivo and in vitro experiments enhancing ADAM 17 activity significantly increased LPS-induced shedding of TNF-α and TNFRII in macrophages while activating TLR-mediated podosome disassembly, synergistically disrupting cell migration and invasion capacity137 (Fig. 3). The treatment method of intervening in podosome formation can inhibit the osteoclast function, but further clinical trials are needed to test its safety.
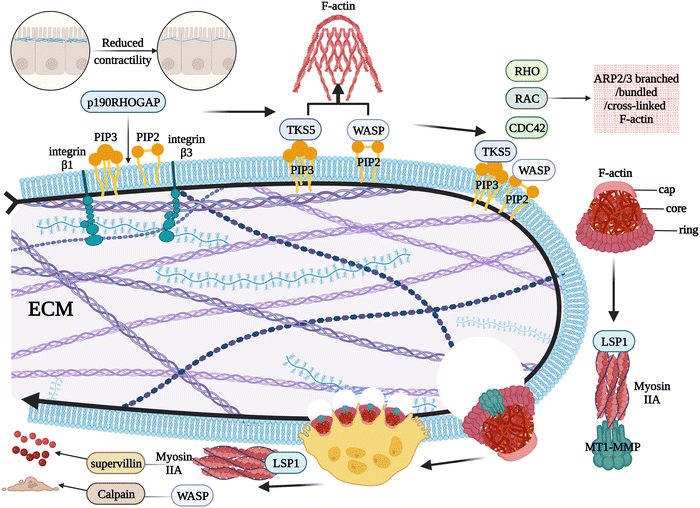 |
| Fig. 3 The process of how podosomes interact with the extracellular matrix on the cell membrane surface. | |
Functional proteins of podosomes.
Podosomes' formation, maturation, and transformation are inseparable from CDC42, RAC, and Ras homolog (RHO) in RHO GTPases, respectively. In vitro and in vivo experiments with knockdown or inhibition of RAC1 and RAC2 have demonstrated the indispensable importance of RAC1/2 in the formation of the sealing zone of osteoclasts.138,139 RHO interferes with the fusion of the podosome ring to the belt at an early stage of osteoclast differentiation.140,141 Rho or its activating effector mDia2 reduces the level of microtubule acetylation in the podosome structure by activating HDAC6, bringing about the structural and functional defects in the podosome and impairment of osteoclast differentiation.140 Targeted protein therapy to prevent podosome maturation and accelerate podosome decomposition is theoretically feasible, but further animal and clinical trials are still needed to validate it.
Podosomes during bone resorption.
RANKL triggers macrophage podosomes to migrate inward, extending actin-rich protrusions that facilitate fusion with neighboring cells. The fused macrophages are filled with actin-rich zipper-like structures to complete cell–cell junctions.142 Mature osteoclasts adhere firmly to the ECM through the adhesion rings of the podosome and CD44 below the nucleus, with dense podosomes arranged closely inside the giant osteoclast membrane to form a closed zone for bone resorption.143 Osteoclasts on the bone surface produce a more expansive sealing zone and a higher density of podosomes than in flat, smooth glass.144 The ruffled border of osteoclasts on the bone has a denser actin fiber network than that in calcite.144 In the collagen-rich environment, type I collagen fibers will activate a linear podosome that is anchored to the ECM in a structure containing not integrins but discoidin domain receptor 1 (DDR1) with lRhoGTPase-Cdc42 and its specific guanine nucleotide-exchange factor (GEF)-tuba structure.145 In addition to regulating the degradation of the ECM by hydrolases, the podosome in macrophages also has a role in recruiting deoxyribonuclease (DNase) to degrade extracellular or bacterial DNA.146 The podosome within macrophages releases MMP2 and MMP9.147 However, relying on hydrolytic enzymes and lacking an acidic environment, or having difficulty breaking the disulfide bonds between fibers, makes it challenging to degrade the ECM completely. We have not yet explored the role of the Na+–H+ exchanger NHE1, which mediates the acidic environment, and oxidoreductase, which reduces disulfide bonds, in the degradation of the ECM by podosomes.
3. Bioengineered treatment of osteolysis
3.1. Electrobiomaterials
Electrobiomaterials favor bone remodeling.
Appropriate mechanical stress and exogenous electrical stimulation can enhance bone remodeling and osseointegration of bone grafts with autogenous bones.148 Piezoelectricity in collagen fibers, fluid-generated streaming potentials in bone microtubules and the flexoelectric effect in cortical bones are important electro-mechanical foundations for harnessing bone remodeling.149,150 Hydroxyapatite significantly induced osteogenic differentiation after electropolarization treatment, while electropolarized synthetic carbonate-substituted apatite scaffolds also improved osteoclastic resorption through increased interfacial wettability.151 Combining the tricalcium phosphate gelatin scaffold with electroacupuncture stimulation (ES) induced a pH change in the culture medium. This resulted in the activation of osteoclasts and osteoblasts in coculture systems, leading to bone formation in rats with bone defects.152 The simultaneous activation of osteoclastic and osteoblastic activities by electrical stimulation will facilitate the in situ remodeling and fusion of bone graft materials with autogenous bones to accelerate the regenerative repair of bone defects.
Electrobiomaterials favor restoration of the bone electrophysiological microenvironment.
Physiological movements such as standing and walking will generate a piezoelectric negative potential of about 300 μV, which is considered to be an electrophysiological environment favourable for osteocyte activity and maintenance of bone homeostasis. Higher electronegativity and currents of 1–10 μA cm−2 occur at fracture and wound healing sites.153 The electrophysiological microenvironment required for bone transformation under disease conditions will be significantly altered. The electrobioactive material aims to mimic the natural electrophysiological microenvironment of healthy bone tissue. This is achieved through various exogenous stimuli such as dielectric, piezoelectric, and pyroelectric properties. The material also generates spontaneous ferroelectric, electroosmotic, and flow potentials to promote osteogenic and angiogenic effects while also having anti-inflammatory, anti-osteolytic, and antibacterial properties for therapeutic purposes. Immunomodulation of biomaterials in inflammatory osteolysis lays the foundation for osteogenic induction. Immunomodulation in electroactive scaffolds is manifested in the chemotaxis of immune cells by electrical stimulation to reduce inflammation and improve a bone microenvironment conducive to tissue regeneration. Monocytes and macrophages tend to avoid electrical stimulation, thus reducing the levels of inflammatory cells and corresponding proinflammatory cytokines in the electrical environment.154 In addition, there is an electric field-dependent regulation of phagocytosis by macrophages. With the aggregation of phagocytic receptors, activation of phosphoinositide 3-kinase (PI3K) and extracellular regulated protein kinases (ERK), mobilization of intracellular calcium, and polarization of actin, macrophages induce the enhanced phagocytosis of microorganisms and necrotic cells, which is crucial for tissue debridement, as well as subsequent osteogenic induction and osteoclastic remodeling.155 Self-promoting electroactive mineralised scaffolds induce osteogenesis by activating voltage-gated channels and relying on the electroactive surface of the material to inhibit bacterial adhesion.133 Time-sequential modulation of electrical stimulation using a fully biodegradable piez–triboelectric hybrid nanogenerator lays the foundation for future treatment of abnormal electrophysiological environments in disease settings.156 In addition to conductive biomaterials (carbon-based nanomaterials, metallic conductive materials, and conducting polymers) that can be stimulated by exogenous power sources, nanogenerators, such as piezoelectric materials, can also rely on mechanical force conduction. Similarly, materials with superior optoelectronic properties like Mxenes can be stimulated by light of different wavelengths and penetration depths,153 which are expected to produce time-sequential modulations that can intervene in the functioning of tissues and cells.
Bioelectric synergies favor inflammatory osteolysis treatment.
Bioelectrical activation can be used alone or in synergistic pairings to achieve microenvironmental modulation. A vaccine technology that uses electrical stimulation to program autologous dendritic cells may be able to enhance the dendritic cell expression of FoxO1, inducing its anti-inflammatory regulatory role in the context of osteoimmune.157 Electrical and chemical excitation of membrane-covered porous titanium scaffolds have anti-inflammatory and osteogenic differentiation-inducing efficacy.158 Photosensitive graphene materials can promote osteogenic differentiation by altering the surface potential distribution and activating voltage-gated calcium channels through visible light stimulation.159 The above materials combine chemical effects or visible light to synergistically stimulate osteoimmune homeostasis in inflammatory environments. Researchers could continue to fill in the gaps between the electrical stimulation and the modulation of tissues and cellular features by various physical properties, such as magnetism, temperature, and force. In addition, future developments should also focus on the time-sequential treatment of inflammatory osteolysis, relying on time-sequential changes in the magnitude of pulsed electrical energy to modulate the immune/osteogenic process accordingly.
Diabetes, with its high glucose and oxidative stress hyperactivity, contributes to the severity and persistence of microbial attack and corresponding tissue damage. The use of chitosan or nanoparticles to create a biomimetic electro-microenvironment has been found to induce anti-inflammatory changes in M1 macrophages under high glucose conditions. This is achieved through the inhibition of protein kinase B (AKT2) and interferon regulatory factor (IRF5) expression in the PI3K-AKT signaling pathway. Additionally, these bioelectric synergies enhance the osteogenic differentiation of human bone marrow mesenchymal stem cells (BMSCs) in a coculture environment, leading to a beneficial osteoimmune microenvironment.160,161 Positive charge treatment of the drug delivery system achieved anti-inflammatory transformation and effectively promoted wound healing in diabetic mice.162 By combining the electrical conductivity of graphene oxide, the osteoconductivity of polarized hydroxyapatite, and the anti-inflammatory properties of polydopamine, researchers have created active scaffolds that can significantly enhance bioelectrical signals. These scaffolds have shown the potential to promote bone regeneration in periodontal bone defects in diabetic rats.163 However, there is still a lack of clinical evidence to support the use of electrobiomaterials to modulate the bone microenvironment in diseases. The reasons for limiting clinical studies of electro-biomaterials may lie in the stability and safety of the electrical output of these electro-active materials, the long-term biosafety and the metabolic toxicity of degradable conductive polymers.
3.2. Implant surface modification
From osteogenesis to antibacterial properties.
The surface of titanium can vary in several ways, including roughness, microscopic discontinuities, hydrophilicity/hydrophobicity, chemistry, and electrical charges. Such differences can significantly impact proteins, cells, and bacterial adhesion, which in turn affects the success of osseointegration and continuous bone remodeling. The ideal implant surface should have adhesive properties that are antimicrobial but favorable for cell adhesion, thus giving rise to the development of various antimicrobial coatings.
Osteogenesis.
Enhancing the osseointegration of titanium implants initially focused on the physical or chemical modification of the surface morphology. Physical surface protrusions are known to play an important role in the survival of bacteria and cells and in their functional differentiation. Nanopillar arrays of 200 nm were able to induce osteogenic differentiation in human adipose-derived stem cells (hADSCs) and induce ectopic osteogenesis in vivo.164 Drawing on the structural properties of nanopillars, hollow titanium oxide TiO2 nanotubes synergised with releasable co-doped calcium or/and strontium can significantly enhance osteogenic properties.165 The prominence of the nano/micron structure to modulate bioactivity actually stems from the high degree of biomimicry of the material to the natural extracellular matrix of the host bone and bone healing hematoma. These bionic micro- and nanostructures on the implant surface will result in increased osteogenic and angiogenic activity of BMSCs and vascular endothelial cells and generate an anti-inflammatory predominantly osteoimmune microenvironment.166 To further improve biocompatibility, a team prepared a hybrid coating of titanium phosphate and TiO2, where protein adhesion and the activity and osteogenic function of BMSCs were significantly enhanced and followed by stronger bone-implant contact bond strength.167 When TiO2 is doped with weaker Zn–O bonds, the superiority of osseointegration and the ability of Zn2+ release with subsequent ROS for bacterial killing will be balanced well, promoting the osseointegration of tibiae of rats infected with Staphylococcus aureus.168 Combining physical protrusion structures that facilitate osteogenesis with surface coatings that enhance biocompatibility and/or improve antimicrobial properties is one of the future directions for solving the difficulties of osseointegration of implants in a bacterial environment.
Antibacterial.
In the field of biomimetic physical antimicrobials, physical nanoprotrusions on insect wings are mimicked to be able to penetrate or stretch bacterial cell walls to provide contact-killing antimicrobial effects. Rough structures of 10–100 μm formed by sandblasting or 1–3 μm formed by acid etching do not have the antimicrobial properties exhibited by nanostructures of 100–250 nm, as evidenced by bacterial penetration and envelope deformation ratios.169,170 And different levels of roughness have corresponding differences in the cell viability and function. Similar to the micron-sized rough surface obtained by sandblasting and acid etching, the nanomorphology after alkali-hydrothermal treatment was superior to the smooth titanium surface in promoting osteogenic activity. Moreover, the attachment of nanomorphology to the gingival epithelium was superior to that of smooth titanium surfaces.171 This suggests that hard and soft tissue closure may not require the clinically typical segmented implant with a smooth neck and rough body. One-piece nanoimplants are theoretically sufficient for a satisfactory hard and soft tissue closure. Moreover, nanotubes with an inner diameter of less than 60 nm are able to aggregate fibronectin and albumin, which play an important role in cell adhesion.172 Therefore, the physical protrusion of the nanoscale structure facilitates antimicrobial resistance and the improvement of all cellular functions. It is the basis for solving the difficulties of implant osseointegration in a bacterial environment.
Osteogenesis and antibacterial.
In a further development of the balance between antimicrobial/antifouling and promotion of cell adhesion, implant surfaces began to be screened for protein adhesion and cell membrane phospholipid affinity. Amphipathic ions were able to improve antifouling properties by reducing the adsorption of bovine serum albumin and fibrinogen. At the same time, it increases the affinity of cell membrane phosphorylcholine to promote the adhesion and proliferation of osteoblasts. It even promotes osteogenic differentiation because the phosphate group stimulates osteogenic signalling.173 Amphoteric polymer coatings carrying positive and negative charges have better antimicrobial effects and osseointegration due to a stronger hydration layer and electrolyte affinity.174,175 Chitosan is rapidly developing in antimicrobial coatings due to its favourable affinity for amphoteric ions. Combining the advantages of nanostructures on 3D-printed titanium surfaces, the antimicrobial coating of quaternized chitosan significantly inhibits infection and promotes wound healing.176 Functionalized chitosan resists fungal adhesion and bacterial adhesion by increasing positive charges, inhibiting non-nutritive fatty acid growth, transforming into natural antibiotics, and incorporating silver nanoparticles.176–179 However, due to the rapid absorption and metabolism of chitosan in the organism, the timeliness of its biological effects will also need to be addressed. The functionalisation of chitosan will become more and more sophisticated in the future, but the complexity and catabolism of coupling materials such as metals, ions, nucleic acids or peptides will also be an issue. How to simplify the complexity will become a major challenge.
From antibacterial to anti-inflammatory.
Advanced mechanical modifications and coating technologies on implant surfaces have contributed significantly to the promotion of osseointegration and antimicrobial activity, especially with hydroxyapatite, calcium, silicon, and strontium coatings for osseointegration and silver, zinc, and copper for the maintenance of antimicrobial activity.180–183 Hydroxyapatite coatings are even more systematically involved in bone immunomodulation, promoting bone formation and angiogenesis as well as the ability to modulate macrophage immune responses.184 However, the above modifications, such as mechanical, physical or chemical, are not efficient compared to the biomodification by direct loading of proteins, although biomodification also faces problems such as coating peeling, high cost of coating preservation, and limited clinical use.
Antibacterial.
Tissue delivery of growth factors reprograms local tissue cells and improves the bone microenvironment, which is not conducive to bone regeneration. Sustained-release antibiotic delivery systems, antimicrobial enzymes or chitosan polymer coatings further enhance the biocompatibility of the material while maintaining antimicrobial efficiency.185–187 The tetra-armed polyethene glycol hydrogel significantly prevents biofilm formation by non-specific adsorption of proteins, bacteria and platelets. With the co-deposition of plant polyphenols and/or other components, it can also significantly improve antimicrobial properties.188 Ti–Ag–Cu and Mg–Ag–Cu nanoparticle coatings significantly reduced the adhesion of Staphylococcus aureus, Staphylococcus epidermidis, and Escherichia coli with little effect on cell viability.189 Slow-release basic fibroblast growth factor (bFGF) and bone morphogenetic protein-2 (BMP-2) core/shell fibrous scaffolds induced M2 transformation of macrophages, which effectively promoted angiogenesis and osteogenesis of periodontal membrane stem cells (PDLSCs).190 However, there is still a gap between the desired results under laboratory conditions and the clinical situation. The stability and reliability of this highly bioselective for adhesion and manipulation of osteoimmune deserves to be validated in further clinical trials.
Anti-inflammatory.
In addition to the management of pathogenic bacteria, however, the regulation of inflammation requires direct intervention on macrophages, which play a decisive role in disease progression. Therefore, researchers designed a macrophage temporally sequential responsive coating. A photosensitive coating that responds to yellow light to produce antimicrobial properties and near-infrared light to produce a photothermal effect to promote osteogenic differentiation holds great promise for the treatment of peri-implantitis.191 The coating promoted macrophage secretion of factors contributing to osteogenic differentiation when the implant was initially placed, and promoted inflammatory macrophage apoptosis after bone maturation, significantly enhancing osseointegration.192 This gives hope for treatment in the clinic to improve the bone microenvironment through autoimmune modulation.
Osteoimmunity.
Various coating technologies are also being explored for the possibility of modulating osteoimmunity. Hydrophilic nanostructured titanium coatings with surface sandblasting and acid etching (SLA) can remodel macrophage homeostasis to reduce the inflammatory response in type 2 diabetes and promote the M2 macrophage function to enhance bone healing.193 Electrostatically spun nanofiber coatings of poly(lactic-co-glycolic acid) (PLGA)-loaded aspirin (ASA) promoted cellular osteogenic differentiation and inhibited M1 polarization as well as RANKL-induced osteoclast differentiation. In vitro experiments have even demonstrated a sustained release effect for up to 60 days and the inhibition of titanium particle-induced peri-implant osteolysis.194 A strategy for functionalizing titanium surfaces has been developed, which involves the integration of mesoporous polydopamine (PDA) nanoparticles, a donor of nitric oxide (NO) called sodium nitroprusside (SNP), and an osteogenic growth peptide (OGP). This approach combines resistance to drug-resistant bacteria with the modulation of the bone immune microenvironment to promote osteogenesis in MSCs and anti-inflammatory effects in RAW264.7 cells through multiple paracrine signaling pathways.195 The above studies have demonstrated a variety of ways to modulate osteoimmune, with few comparisons of the differences in efficacy between them. More research studies are needed to model the process of innate osteoimmune, compare the various approaches and explore the most beneficial regimen for osseointegration in the clinic.
From anti-inflammatory to bone remodeling.
The above study achieves the dual role of promoting osteogenesis and modulating inflammation, which facilitates the osseointegration of implants in inflammatory and other disorders. In the early stage of osseointegration, it is not only necessary to ensure the appropriate functioning of osteogenic differentiation, but also to inhibit osteoclastic activity. Silicon (Si) substitutes not only promote osteogenic induction of BMSCs but also reduce osteoclast production. The preparation of Si-substituted nanostructured hydroxyapatite-coated titanium implants was able to effectively inhibit inflammation-induced osteoclastic hyperostosis under diabetes, exhibiting optimal osseointegration efficiency.196 The preparation of nanostructured akermanite-coated titanium implants can effectively inhibit osteoclastic hyperostosis manifested by the imbalance of bone homeostasis in osteoporosis and achieve bone regenerative healing in osteoporosis.197 The metal–polyphenol coating containing magnesium ions demonstrated a synergistic effect of osteoinductive metal ions (Mg2+) and polyphenols (EGCG) that significantly inhibited osteoclast maturation and enhanced osteoblast differentiation, favoring osseointegration of titanium implants on rabbits.198 After being stimulated by near-infrared light, the surface of black TiO2 nanotubes immobilized with IL-4/polydopamine released IL-4, which helped in reducing inflammation, inhibiting osteoclast differentiation and function, and enabling optimal osseointegration over a period of four weeks.199 These methods ensure initial osseointegration of the implant, but lack the distant promotion of dynamic reconstruction of the peri-implant bone tissue.
As the alveolar bone undergoes remodelling, the restructuring of the peri-implant osseointegration continues in concert with osteogenesis and osteoclastogenesis. Active coatings are available that focus on inducing both osteoblasts and osteoclasts simultaneously. This helps to obtain the role of osteoclast metabolic factors in re-inducing osteogenesis and osteolysis. This, in turn, maintains and improves the structural strength of bone tissues.200 Chlorine-substituted hydroxyapatite/polydopamine composite coatings promote osteoclast and osteoblast induction through the eosinophilic nature of the former and the adhesive nature of the latter, respectively.201 However, no animal studies have been performed to demonstrate this. Coatings of miR-21 nanocapsules on titanium can promote the development of both osteoblasts and osteoclasts, leading to faster bone remodeling and maturation. This process significantly increases the strength of osseointegration for implants.202 However, there is a lack of sufficient basic and animal studies on temporal sequential modulation of osteogenic and osteoclastic activities. Future surface modifications that can be applied clinically may need to direct osteogenic hyperactivity during the early stages of osseointegration, osteoclastic enhancement during remodelling, and bone homeostatic equilibrium during stabilization.
3.3. Extracellular vesicles
Due to safety concerns, stem cell therapy with immunomodulation and multidirectional functional differentiation is difficult to standardize for clinical applications. Extracellular vehicles (EVs) are critical paracrine components of stem cells that regulate the extracellular microenvironment.
EVs of MSCs originating from the endoderm.
ADSC therapy facilitated inflammatory clearance and tissue regeneration after mechanical debridement of periodontitis but was less effective than ADSC-derived EVs.203 BMSC-derived EVs were found to promote human periodontal cell migration, proliferation, and osteogenic differentiation (hPDLCs). Additionally, these EVs were observed to significantly inhibit the osteoclastic differentiation of inflammatory infiltrates in areas of periodontitis bone defects under nanocomposite hydrogel loading, thus effectively slowing down the advancement of periodontal inflammation and immune damage.204 The use of dental pulp stem cell (DPSC)-derived EVs combined with the chitosan hydrogel helped in improving periodontal inflammatory osteolysis. This treatment worked by inhibiting proinflammatory development and promoting osteogenesis, which was linked to the presence of miR-1246.205 In addition to the mobilization of anti-inflammatory polarization of mononuclear macrophages, the modulation of the immune microenvironment also involves the suppression of T cells through gingival mesenchymal stem cell (GMSC)-derived EVs and the induction of Treg generation. This indirectly reverses the immune inflammatory environment and greatly enhances the regeneration of damaged periodontal tissues.206 Inflammation-stimulated stem cells seem to lean toward anti-inflammatory modulation. For example, under inflammatory conditions, dental follicle stem cell (DFSC)-derived EVs function as ROS-eliminating antioxidants, decreasing the RANKL/OPG ratio of PDLSCs and increasing the M2 phenotype polarization of macrophages via inhibition of JNK signaling and activation of ERK signaling, respectively.207 Injectable delivery platforms of GMSC-EVs or DFSC-EVs demonstrated healing effects on inflammatory periodontal osteolysis in animals due to the sustained release of EVs.206,207 As can be seen, EVs of MSCs are powerful enough to use osteoimmune to regulate the balance between osteogenesis and osteolysis. Even after inflammatory stimulation, these EVs demonstrated osteointegration-promoting effects. Further clinical studies are still needed in the future to verify the significant promotion of osteointegration by EVs of MSCs.
EVs of immune cells originating from the yolk sac.
Yolk sac-derived immune cell EVs modulate osteoimmune interactions, balancing inflammation and maintaining bone homeostasis. IL10, enriched in M2-EVs, is the messenger of osteogenesis promotion and osteoclastic inhibition, mediating the dual treatment of inflammation and osteolysis in periodontitis mice.208 Interestingly, inflammation-stimulated osteoclast-derived exosomes (iOC-Exos) have a negative feedback role in promoting osteogenic activity. The iOC-Exos targeted ephrinA2/EphA2 in the osteoblast membrane and then delivered the lncRNA LIOCE to stabilize the osteogenic transcription factor osterix to promote bone formation.209 In addition to stronger immunomodulatory effects of EVs of immune cells, they are also very effective in promoting osteogenic differentiation.
EVs of neuronal cells originating from the ectoderm.
Neuralization has the potential to balance multisystem homeostasis in the regulation of the bone immune microenvironment, as hydrogel-encapsulated Schwann cell-derived exosomes (SC-Exos) promoted anti-inflammation, angiogenesis, and osteogenesis in vitro.210 More future studies on EVs of neuronal cells in bone homeostasis regulation are needed to explore their commonalities and specificities.
EVs originating from bacteria and plants.
Bacterial-derived EVs also hold great promise in the immunomodulatory treatment of inflammatory osteolysis. A vaccine composed of Pg-derived EVs (Pg-EVs) combined with a TLR agonist produced Pg-specific antibodies in mice, which safely yet stably maintained the suppressive viability of Pg in mice.211 Bacterial-derived EVs can also act as antibiotic carriers to kill pathogenic bacteria and eliminate the infection.212 Plant-derived exosomes, such as ginger exosome-like nanoparticles (GELNs), can target Pg for killing and have potential therapeutic promise against periodontal or peri-implant inflammatory osteolysis.213 These EVs have some antimicrobial effects, but there is still a lack of exploration of their biomodulatory effects in animals and humans.
4. Discussion
This review provides a comprehensive overview of the intricate interplay between cells, proteins, RNA, epigenetic modifications, and podosomes in regulating the osteoimmune microenvironment during inflammatory osteolysis. This review summarizes recent advancements in promoting the osteogenic differentiation of osteogenic progenitor cells and preventing inflammatory polarization and osteolytic differentiation of immune cells to enhance peri-implant osseointegration as well as prevent inflammatory bone loss. While identifying key therapeutic targets is challenging owing to the complex and ever-changing nature of the microenvironment in inflammatory osteolysis, recent research has yielded promising results. For example, Withaferin-A has been found to induce M1 macrophages to release proinflammatory cytokines and oxidative stress markers, reverse the M1/M2 cell ratio, and encourage the differentiation of M1 macrophages into M2 macrophages.214 Moreover, M2 exosomes derived from M2-type macrophages, as well as IL10 and betamethasone sodium phosphate, have been shown to promote the repolarization of M1 to M2 macrophages and significantly reduce inflammatory expression in mice with rheumatoid arthritis.215 Future studies could further simplify the process of bone immunomodulation and more efficiently reverse poor osteogenesis in inflammatory environments.
To promote pro-inflammation in localized regions during bone remodeling and anti-inflammation in localized regions during bone neogenesis, future treatments must account for the spatiotemporal-dependent modulation of inflammatory properties. Moreover, the spatial distribution of cells is crucial in directing cellular behavior and maintaining tissue homeostasis, which likewise facilitates the regulation of the bone immune microenvironment. Recent research has found that Tai Chi-shaped multicellular patterns of MSCs and macrophages in a 2
:
1 numerical ratio play a more significant role in mediating paracrine inhibition of inflammation and regulating osteogenic differentiation than star- and interlacing-shaped multicellular patterns.216 This finding presents a new approach to implant surface modification.
In addition to direct osteoimmune modulation, researchers have explored immunomodulation-related bone microbiology, such as probiotics, which have been found to have anti-bone loss effects by increasing the Treg-Th17 cell ratio and modulating inflammation-related factors.217 This technique may prove to be a powerful tool for regulating bone microenvironment homeostasis in the future.
Current research has yet to uncover the specific bone immune mechanisms underlying inflammatory osteolysis around implants. The academic community has not reached a consensus on the long-term stable immune inflammatory response between implants and surrounding soft and hard tissues. Similarly, apart from bacterial factors, there is also no consensus on the pathogenesis of periprosthetic inflammation, adding uncertainty to the treatment of inflammatory osteolysis around implants. This review outlines the targets for bone immune mechanisms, including cells, proteins, epigenetic modifications, RNA, and podosomes, and introduces the development prospects of electrobioactive materials, implant surface modification, and extracellular vesicles in the treatment of periprosthetic inflammation. However, there are still many biomimetic methods for regulating bone immunity that warrant further research. Similarly, the output voltage of electrobioactive materials, the long-term stability and safety of materials, the specificity of targeted drugs, and issues such as implant surface delamination and cost are also worthy of further investigation.
Overall, recent advances in understanding the complex mechanisms underlying the osteoimmune microenvironment offer exciting opportunities for developing new therapeutic approaches to prevent and treat inflammatory bone loss. The development of the three osteoimmunotherapeutic approaches to peri-implant inflammatory osteolysis described above is not independent and should perhaps cross over into each other in future clinical use. In the early intervention of osteoimmunity at the time of implant placement, bionic treatment of the implant surface can be combined with electrobiomaterials in order to achieve temporal and spatial specificity of osteoimmunomodulation in the microenvironment of inflammatory osteolysis. At different stages of disease progression, treatment of EVs around diseased tissues with inflammatory osteolysis may enable in vitro modulation of osteoimmunotherapy for peri-implantitis. Future clinical use that combines the advantages of the above methods will certainly be rewarding in the treatment of inflammatory osteolysis around implants.
5. Conclusions
There are many potential therapeutic targets underlying cells, proteins, epigenetic modifications, RNA, and podosomes in the osteoimmune mechanisms of peri-implant inflammatory osteolysis. However, bioengineering treatments such as electrobiomaterials, implant surface modifications, and EVs have not fully developed specific targets for harnessing osteoimmune to treat peri-implant inflammatory osteolysis. Therefore, the integration of bioengineering treatment approaches and immune targets is the direction for future research.
Author contributions
Zijun Chen: conceptualization, writing – original draft, and visualization. Yuxi Wang: conceptualization, visualization, and writing – review and editing. Rui Yang: supervision and writing – review and editing. Xiangdong Liu: visualization and writing – review and editing. Guanhua Zhang: visualization and writing – review and editing. Qun Lu: writing – review and editing. Wei Ma: writing – review and editing. Yingliang Song: conceptualization, project administration, and funding acquisition.
Conflicts of interest
The authors declare that the research was conducted in the absence of any commercial or financial relationships that could be construed as a potential conflict of interest.
Acknowledgements
The authors thank Prof. Yingliang Song, Prof. Wei Ma, and Prof. Qun Lu for their invaluable suggestions on improving the manuscript. All figures were created with BioRender.com. This study was financially supported by the National Natural Science Foundation of China (82170991).
References
- R. Trindade, T. Albrektsson, S. Galli, Z. Prgomet, P. Tengvall and A. Wennerberg, Osseointegration and foreign body reaction: Titanium implants activate the immune system and suppress bone resorption during the first 4 weeks after implantation, Clin. Implant Dent. Relat. Res., 2018, 20(1), 82–91 CrossRef.
- T. Albrektsson, T. Jemt, J. Mölne, P. Tengvall and A. Wennerberg, On inflammation-immunological balance theory-A critical apprehension of disease concepts around implants: Mucositis and marginal bone loss may represent normal conditions and not necessarily a state of disease, Clin. Implant Dent. Relat. Res., 2019, 21(1), 183–189 CrossRef.
- T. Albrektsson, P. Tengvall, L. Amengual, P. Coli, G. A. Kotsakis and D. Cochran, Osteoimmune regulation underlies oral implant osseointegration and its perturbation, Front. Immunol., 2022, 13, 1056914 CrossRef CAS.
- M. Kuboniwa, J. R. Houser, E. L. Hendrickson, Q. Wang, S. A. Alghamdi and A. Sakanaka,
et al., Metabolic crosstalk regulates Porphyromonas gingivalis colonization and virulence during oral polymicrobial infection, Nat. Microbiol., 2017, 2(11), 1493–1499 CrossRef CAS PubMed.
- Y. Liu, Q. Liu, Z. Li, A. Acharya, D. Chen and Z. Chen,
et al., Long non-coding RNA and mRNA expression profiles in peri-implantitis vs periodontitis, J. Periodontal Res., 2020, 55(3), 342–353 CrossRef CAS PubMed.
- R. Faccio, D. V. Novack, A. Zallone, F. P. Ross and S. L. Teitelbaum, Dynamic changes in the osteoclast cytoskeleton in response to growth factors and cell attachment are controlled by beta3 integrin, J. Cell Biol., 2003, 162(3), 499–509 CrossRef CAS PubMed.
- K. Yokota, K. Sato, T. Miyazaki, Y. Aizaki, S. Tanaka and M. Sekikawa,
et al., Characterization and Function of Tumor Necrosis Factor and Interleukin-6-Induced Osteoclasts in Rheumatoid Arthritis, Arthritis Rheumatol., 2021, 73(7), 1145–1154 CrossRef CAS PubMed.
- L. Song, G. Dong, L. Guo and D. T. Graves, The function of dendritic cells in modulating the host response, Mol. Oral Microbiol., 2018, 33(1), 13–21 CrossRef CAS PubMed.
- L. J. O'Neil, C. B. Oliveira, X. Wang, M. Navarrete, A. Barrera-Vargas and J. Merayo-Chalico,
et al., Neutrophil extracellular trap-associated carbamylation and histones trigger osteoclast formation in rheumatoid arthritis, Ann. Rheum. Dis., 2023, 82(5), 630–638 CrossRef PubMed.
- M. Zhou, J. Wang, J. Wang, J. Yu, S. Huang and T. Wang,
et al., Construction of a Localized and Long-Acting CCN2 Delivery System on Percutaneous Ti Implant Surfaces for Enhanced Soft-Tissue Integration, ACS Appl. Mater. Interfaces, 2023, 15(19), 22864–22875 CrossRef CAS PubMed.
- T. Wachi, T. Shuto, Y. Shinohara, Y. Matono and S. Makihira, Release of titanium ions from an implant surface and their effect on cytokine production related to alveolar bone resorption, Toxicology, 2015, 327, 1–9 CrossRef CAS PubMed.
- M. Irshad, N. Scheres, W. Crielaard, B. G. Loos, D. Wismeijer and M. L. Laine, Influence of titanium on in vitro fibroblast-Porphyromonas gingivalis interaction in peri-implantitis, J. Clin. Periodontol., 2013, 40(9), 841–849 CrossRef CAS PubMed.
- M. I. Setyawati, P. K. Khoo, B. H. Eng, S. Xiong, X. Zhao and G. K. Das,
et al., Cytotoxic and genotoxic characterization of titanium dioxide, gadolinium oxide, and poly(lactic-co-glycolic acid) nanoparticles in human fibroblasts, J. Biomed. Mater. Res., Part A, 2013, 101(3), 633–640 CrossRef.
- T. Toyooka, T. Amano and Y. Ibuki, Titanium dioxide particles phosphorylate histone H2AX independent of ROS production, Mutat. Res., 2012, 742(1–2), 84–91 CAS.
- J. Wen, D. Cai, W. Gao, R. He, Y. Li and Y. Zhou,
et al., Osteoimmunomodulatory Nanoparticles for Bone Regeneration, Nanomaterials, 2023, 13(4), 692 CrossRef CAS.
- A. K. Gaharwar, I. Singh and A. Khademhosseini, Engineered biomaterials for in situ tissue regeneration, Nat. Revi. Mater., 2020, 5(9), 686–705 CrossRef CAS.
- B. C. Heng, Y. Bai, X. Li, L. W. Lim, W. Li and Z. Ge,
et al., Electroactive Biomaterials for Facilitating Bone Defect Repair under Pathological Conditions, Adv. Sci., 2023, 10(2), e2204502 CrossRef.
- K. Okamoto, T. Nakashima, M. Shinohara, T. Negishi-Koga, N. Komatsu and A. Terashima,
et al., Osteoimmunology: The Conceptual Framework Unifying the Immune and Skeletal Systems, Physiol. Rev., 2017, 97(4), 1295–1349 CrossRef CAS PubMed.
- H. Takayanagi, S. Kim, T. Koga, H. Nishina, M. Isshiki and H. Yoshida,
et al., Induction and activation of the transcription factor NFATc1 (NFAT2) integrate RANKL signaling in terminal differentiation of osteoclasts, Dev. Cell, 2002, 3(6), 889–901 CrossRef CAS PubMed.
- B. Soós, Á. Szentpétery, H. G. Raterman, W. F. Lems, H. P. Bhattoa and Z. Szekanecz, Effects of targeted therapies on bone in rheumatic and musculoskeletal diseases, Nat. Rev. Rheumatol., 2022, 18(5), 249–257 CrossRef.
- T. Nakashima, M. Hayashi, T. Fukunaga, K. Kurata, M. Oh-Hora and J. Q. Feng,
et al., Evidence for osteocyte regulation of bone homeostasis through RANKL expression, Nat. Med., 2011, 17(10), 1231–1234 CrossRef CAS PubMed.
- I. Kramer, C. Halleux, H. Keller, M. Pegurri, J. H. Gooi and P. B. Weber,
et al., Osteocyte Wnt/beta-catenin signaling is required for normal bone homeostasis, Mol. Cell. Biol., 2010, 30(12), 3071–3085 CrossRef CAS.
- C. Sobacchi, A. Frattini, M. M. Guerrini, M. Abinun, A. Pangrazio and L. Susani,
et al., Osteoclast-poor human osteopetrosis due to mutations in the gene encoding RANKL, Nat. Genet., 2007, 39(8), 960–962 CrossRef CAS.
- H. Takayanagi, Osteoimmunology: shared mechanisms and crosstalk between the immune and bone systems, Nat. Rev. Immunol., 2007, 7(4), 292–304 CrossRef CAS.
- M. Tsukasaki, N. Komatsu, K. Nagashima, T. Nitta, W. Pluemsakunthai and C. Shukunami,
et al., Host defense against oral microbiota by bone-damaging T cells, Nat. Commun., 2018, 9(1), 701 CrossRef PubMed.
- I. Könnecke, A. Serra, T. El Khassawna, C. Schlundt, H. Schell and A. Hauser,
et al., T and B cells participate in bone repair by infiltrating the fracture callus in a two-wave fashion, Bone, 2014, 64, 155–165 CrossRef PubMed.
- Y. Yuan, H. Zhang, Q. Gu, X. Xu, R. Yu and H. Huang, Analysis of Th-cell subsets in local and systemic environments from experimental periodontitis rats, Mol. Oral Microbiol., 2023, 38(2), 83–92 CrossRef CAS PubMed.
- G. Monasterio, B. Fernández, F. Castillo, C. Rojas, E. A. Cafferata and L. Rojas,
et al., Capsular-defective Porphyromonas gingivalis mutant strains induce less alveolar bone resorption than W50 wild-type strain due to a decreased Th1/Th17 immune response and less osteoclast activity, J. Periodontol., 2019, 90(5), 522–534 CrossRef CAS PubMed.
- G. R. Souto, C. M. Queiroz-Junior, M. H. de Abreu, F. O. Costa and R. A. Mesquita, Pro-inflammatory, Th1, Th2, Th17 cytokines and dendritic cells: a cross-sectional study in chronic periodontitis, PLoS One, 2014, 9(3), e91636 CrossRef PubMed.
- P. R. Cury, J. P. Carmo, V. V. Horewicz, J. N. Santos and J. A. Barbuto, Altered phenotype and function of dendritic cells in individuals with chronic periodontitis, Arch. Oral Biol., 2013, 58(9), 1208–1216 CrossRef CAS PubMed.
- Y. T. Teng, D. Mahamed and B. Singh, Gamma interferon positively modulates Actinobacillus actinomycetemcomitans-specific RANKL+ CD4+ Th-cell-mediated alveolar bone destruction in vivo, Infect. Immun., 2005, 73(6), 3453–3461 CrossRef CAS PubMed.
- X. Yin, S. Chen and S. C. Eisenbarth, Dendritic Cell Regulation of T Helper Cells, Annu. Rev. Immunol., 2021, 39(1), 759–790 CrossRef CAS PubMed.
- G. P. Garlet, C. R. Cardoso, F. S. Mariano, M. Claudino, G. F. de Assis and A. P. Campanelli,
et al., Regulatory T cells attenuate experimental periodontitis progression in mice, J. Clin. Periodontol., 2010, 37(7), 591–600 CrossRef CAS.
- Q. Jin, H. Yang, Z. Jing, W. Hong-Hua, S. Ben-Jing and W. Li-Ting,
et al., IL4/IL4R signaling promotes the osteolysis in metastatic bone of CRC through regulating the proliferation of osteoclast precursors, Mol. Med., 2021, 27(1), 152 CAS.
- B. J. Conti, K. B. Santiago, E. O. Cardoso, F. L. Conte, M. A. Golim and M. T. Cruz,
et al., Effect of propolis on Th2 and Th17 cells: interplay with EtxB- and LPS-treated dendritic cells, Brazilian J. Med. Biol. Res., 2023, 56, e12659 CrossRef CAS PubMed.
- H. Qiu, C. Hosking, E. Rothzerg, A. Samantha, K. Chen and V. Kuek,
et al., ADR3, a next generation i-body to human RANKL, inhibits osteoclast formation and bone resorption, J. Biol. Chem., 2023, 299(2), 102889 CrossRef CAS PubMed.
- W. S. Simonet, D. L. Lacey, C. R. Dunstan, M. Kelley, M. S. Chang and R. Lüthy,
et al., Osteoprotegerin: a novel secreted protein involved in the regulation of bone density, Cell, 1997, 89(2), 309–319 CrossRef CAS PubMed.
- S. R. Cummings, J. San Martin, M. R. McClung, E. S. Siris, R. Eastell and I. R. Reid,
et al., Denosumab for prevention of fractures in postmenopausal women with osteoporosis, New Engl. J. Med., 2009, 361(8), 756–765 CrossRef CAS PubMed.
- J. E. Brown and R. E. Coleman, Denosumab in patients with cancer-a surgical strike against the osteoclast, Nat. Rev. Clin. Oncol., 2012, 9(2), 110–118 CrossRef CAS PubMed.
- A. A. Khan, A. Morrison, D. A. Hanley, D. Felsenberg, L. K. McCauley and F. O'Ryan,
et al., Diagnosis and management of osteonecrosis of the jaw: a systematic review and international consensus, J. Bone Min. Res., 2015, 30(1), 3–23 CrossRef PubMed.
- M. Morita, R. Iwasaki, Y. Sato, T. Kobayashi, R. Watanabe and T. Oike,
et al., Elevation of pro-inflammatory cytokine levels following anti-resorptive drug treatment is required for osteonecrosis development in infectious osteomyelitis, Sci. Rep., 2017, 7, 46322 CrossRef CAS PubMed.
- S. Mitchell, J. Vargas and A. Hoffmann, Signaling via the NFκB system, Wiley Interdiscip. Rev.: Syst. Biol. Med., 2016, 8(3), 227–241 CAS.
- M. C. Walsh, J. Lee and Y. Choi, Tumor necrosis factor receptor- associated factor 6 (TRAF6) regulation of development, function, and homeostasis of the immune system, Immunol. Rev., 2015, 266(1), 72–92 CrossRef CAS.
- E. Sumiya, T. Negishi-Koga, Y. Nagai, A. Suematsu, T. Suda and M. Shinohara,
et al., Phosphoproteomic analysis of kinase-deficient mice reveals multiple TAK1 targets in osteoclast differentiation, Biochem. Biophys. Res. Commun., 2015, 463(4), 1284–1290 CrossRef CAS PubMed.
- M. C. Walsh, G. K. Kim, P. L. Maurizio, E. E. Molnar and Y. Choi, TRAF6 autoubiquitination-independent activation of the NFkappaB and MAPK pathways in response to IL-1 and RANKL, PLoS One, 2008, 3(12), e4064 CrossRef PubMed.
- J. P. David, K. Sabapathy, O. Hoffmann, M. H. Idarraga and E. F. Wagner, JNK1 modulates osteoclastogenesis through both c-Jun phosphorylation-dependent and -independent mechanisms, J. Cell Sci., 2002, 115(Pt 22), 4317–4325 CrossRef CAS.
- X. Li, N. Udagawa, K. Itoh, K. Suda, Y. Murase and T. Nishihara,
et al., p38 MAPK-mediated signals are required for inducing osteoclast differentiation but not for osteoclast function, Endocrinology, 2002, 143(8), 3105–3113 CrossRef CAS PubMed.
- H. Hotokezaka, E. Sakai, K. Kanaoka, K. Saito, K. Matsuo and H. Kitaura,
et al., U0126 and PD98059, specific inhibitors of MEK, accelerate differentiation of RAW264.7 cells into osteoclast-like cells. The, J. Biol. Chem., 2002, 277(49), 47366–47372 CrossRef CAS PubMed.
- I. Breitkreutz, M. S. Raab, S. Vallet, T. Hideshima, N. Raje and D. Chauhan,
et al., Targeting MEK1/2 blocks osteoclast differentiation, function and cytokine secretion in multiple myeloma, Br. J. Haematol., 2007, 139(1), 55–63 CrossRef CAS PubMed.
- G. Swarnkar, K. Shim, A. M. Nasir, K. Seehra, H. P. Chen and G. Mbalaviele,
et al., Myeloid Deletion of Nemo Causes Osteopetrosis in Mice Owing to Upregulation of Transcriptional Repressors, Sci. Rep., 2016, 6, 29896 CrossRef CAS PubMed.
- O. Jacenko, c-fos and bone loss: a proto-oncogene regulates osteoclast lineage determination, BioEssays, 1995, 17(4), 277–281 CrossRef CAS PubMed.
- L. Kenner, A. Hoebertz, F. T. Beil, N. Keon, F. Karreth and R. Eferl,
et al., Mice lacking JunB are osteopenic due to cell-autonomous osteoblast and osteoclast defects, J. Cell Biol., 2004, 164(4), 613–623 CrossRef CAS PubMed.
- K. Kim, J. H. Kim, J. Lee, H. M. Jin, S. H. Lee and D. E. Fisher,
et al., Nuclear factor of activated T cells c1 induces osteoclast-associated receptor gene expression during tumor necrosis factor-related activation-induced cytokine-mediated osteoclastogenesis, J. Biol. Chem., 2005, 280(42), 35209–35216 CrossRef CAS PubMed.
- K. P. McHugh, K. Hodivala-Dilke, M. H. Zheng, N. Namba, J. Lam and D. Novack,
et al., Mice lacking beta3 integrins are osteosclerotic because of dysfunctional osteoclasts, J. Clin. Investig., 2000, 105(4), 433–440 CrossRef CAS PubMed.
- M. Yagi, T. Miyamoto, Y. Sawatani, K. Iwamoto, N. Hosogane and N. Fujita,
et al., DC-STAMP is essential for cell-cell fusion in osteoclasts and foreign body giant cells, J. Exp. Med., 2005, 202(3), 345–351 CrossRef CAS PubMed.
- H. Miyamoto, T. Suzuki, Y. Miyauchi, R. Iwasaki, T. Kobayashi and Y. Sato,
et al., Osteoclast stimulatory transmembrane protein and dendritic cell–specific transmembrane protein cooperatively modulate cell–cell fusion to form osteoclasts and foreign body giant cells, J. Bone Min. Res., 2012, 27(6), 1289–1297 CrossRef CAS PubMed.
- S. H. Lee, J. Rho, D. Jeong, J. Y. Sul, T. Kim and N. Kim,
et al., v-ATPase V0 subunit d2-deficient mice exhibit impaired osteoclast fusion and increased bone formation, Nat. Med., 2006, 12(12), 1403–1409 CrossRef CAS PubMed.
- T. Yamashita, Z. Yao, F. Li, Q. Zhang, I. R. Badell and E. M. Schwarz,
et al., NF-kappaB p50 and p52 regulate receptor activator of NF-kappaB ligand (RANKL) and tumor necrosis factor-induced osteoclast precursor differentiation by activating c-Fos and NFATc1, J. Biol. Chem., 2007, 282(25), 18245–18253 CrossRef CAS PubMed.
- H. Cao, S. Yu, Z. Yao, D. L. Galson, Y. Jiang and X. Zhang,
et al., Activating transcription factor 4 regulates osteoclast differentiation in mice, J. Clin. Investig., 2010, 120(8), 2755–2766 CrossRef CAS PubMed.
- Y. Miyauchi, K. Ninomiya, H. Miyamoto, A. Sakamoto, R. Iwasaki and H. Hoshi,
et al., The Blimp1-Bcl6 axis is critical to regulate osteoclast differentiation and bone homeostasis, J. Exp. Med., 2010, 207(4), 751–762 CrossRef CAS PubMed.
- K. Nishikawa, T. Nakashima, M. Hayashi, T. Fukunaga, S. Kato and T. Kodama,
et al., Blimp1-mediated repression of negative regulators is required for osteoclast differentiation, Proc. Natl. Acad. Sci. U. S. A., 2010, 107(7), 3117–3122 CrossRef CAS PubMed.
- K. Tsuji-Takechi, T. Negishi-Koga, E. Sumiya, A. Kukita, S. Kato and T. Maeda,
et al., Stage-specific functions of leukemia/lymphoma-related factor (LRF) in the transcriptional control of osteoclast development, Proc. Natl. Acad. Sci. U. S. A., 2012, 109(7), 2561–2566 CrossRef CAS PubMed.
- K. Maruyama, M. Fukasaka, A. Vandenbon, T. Saitoh, T. Kawasaki and T. Kondo,
et al., The transcription factor Jdp2 controls bone homeostasis and antibacterial immunity by regulating osteoclast and neutrophil differentiation, Immunity, 2012, 37(6), 1024–1036 CrossRef CAS PubMed.
- A. R. Hayman, S. J. Jones, A. Boyde, D. Foster, W. H. Colledge and M. B. Carlton,
et al., Mice lacking tartrate-resistant acid phosphatase (Acp 5) have disrupted endochondral ossification and mild osteopetrosis, Development, 1996, 122(10), 3151–3162 CrossRef CAS PubMed.
- W. Wang, X. Liang, X. Liu, J. Bai, W. Zhang and W. Li,
et al., NOX4 blockade suppresses titanium nanoparticle-induced bone destruction via activation of the Nrf2 signaling pathway, J. Nanobiotechnol., 2022, 20(1), 241 CrossRef CAS PubMed.
- J. Li, L. Wang, B. Wang, Z. Zhang, L. Jiang and Z. Qin,
et al., NOX4 is a potential therapeutic target in septic acute kidney injury by inhibiting mitochondrial dysfunction and inflammation, Theranostics, 2023, 13(9), 2863–2878 CrossRef CAS PubMed.
- S. Fan, H. Pan, J. Huang, Z. Lei and J. Liu, Hyperoside exerts osteoprotective effect on dexamethasone-induced osteoblasts by targeting NADPH Oxidase 4 (NOX4) to inhibit the reactive oxygen species (ROS) accumulation and activate c-Jun N-terminal kinase (JNK) pathway, Bioengineered, 2022, 13(4), 8657–8666 CrossRef CAS PubMed.
- M. Liu, S. Liu, Q. Zhang, Y. Fang, Y. Yu and L. Zhu,
et al., Curculigoside attenuates oxidative stress and osteoclastogenesis via modulating Nrf2/NF-κB signaling pathway in RAW264.7 cells, J. Ethnopharmacol., 2021, 275, 114129 CrossRef CAS PubMed.
- S. M. Bartell, H. N. Kim, E. Ambrogini, L. Han, S. Iyer and S. Serra Ucer,
et al., FoxO proteins restrain osteoclastogenesis and bone resorption by attenuating H2O2 accumulation, Nat. Commun., 2014, 5, 3773 CrossRef PubMed.
- G. Mognol, F. Carneiro, B. Robbs, D. Faget and J. Viola, Cell cycle and apoptosis regulation by NFAT transcription factors: new roles for an old player, Cell Death Dis., 2016, 7, e2199 CrossRef CAS PubMed.
- M. Shalev and A. Elson, The roles of protein tyrosine phosphatases in bone-resorbing osteoclasts, Biochim. Biophys. Acta, Mol. Cell Res., 2019, 1866(1), 114–123 CrossRef CAS PubMed.
- G. Yuan and S. Yang, Effect of Regulator of G Protein Signaling Proteins on Bone, Front. Endocrinol., 2022, 13, 842421 CrossRef PubMed.
- W. Sun, S. Guo, Y. Li, J. Li, C. Liu and Y. Chen,
et al., Anoctamin 1 controls bone resorption by coupling Cl− channel activation with RANKL-RANK signaling transduction, Nat. Commun., 2022, 13(1), 2899 CrossRef CAS PubMed.
- J. E. Kirkpatrick, K. L. Kirkwood and P. M. Woster, Inhibition of the histone demethylase KDM4B leads to activation of KDM1A, attenuates bacterial-induced pro-inflammatory cytokine release, and reduces osteoclastogenesis, Epigenetics, 2018, 13(5), 557–572 CrossRef PubMed.
- M. D. Cantley, P. M. Bartold, V. Marino, D. P. Fairlie, G. T. Le and A. J. Lucke,
et al., Histone deacetylase inhibitors and periodontal bone loss, J. Periodontal Res., 2011, 46(6), 697–703 CrossRef CAS PubMed.
- L. Wang, Y. He and W. Ning, Role of enhancer of zeste homolog 2 in osteoclast formation and periodontitis development by downregulating microRNA-101-regulated VCAM-1, J. Tissue Eng. Regener. Med., 2021, 15(6), 534–545 CrossRef CAS PubMed.
- F. Paino, M. La Noce, V. Tirino, P. Naddeo, V. Desiderio and G. Pirozzi,
et al., Histone deacetylase inhibition with valproic acid downregulates osteocalcin gene expression in human dental pulp stem cells and osteoblasts: evidence for HDAC2 involvement, Stem Cells, 2014, 32(1), 279–289 CrossRef CAS PubMed.
- K. Kim, J. Lee, J. H. Kim, H. M. Jin, B. Zhou and S. Y. Lee,
et al., Protein inhibitor of activated STAT 3 modulates osteoclastogenesis by down-regulation of NFATc1 and osteoclast-associated receptor, J. Immunol., 2007, 178(9), 5588–5594 CrossRef CAS PubMed.
- N. C. Blixt, B. K. Faulkner, K. Astleford, R. Lelich, J. Schering and E. Spencer,
et al., Class II and IV HDACs function as inhibitors of osteoclast differentiation, PLoS One, 2017, 12(9), e0185441 CrossRef PubMed.
- Y. Zilberman, C. Ballestrem, L. Carramusa, R. Mazitschek, S. Khochbin and A. Bershadsky, Regulation of microtubule dynamics by inhibition of the tubulin deacetylase HDAC6, J. Cell Sci., 2009, 122(Pt 19), 3531–3541 CrossRef CAS PubMed.
- Y. Wang, R. Abrol, J. Y. W. Mak, K. Das Gupta, D. Ramnath and D. Karunakaran,
et al., Histone deacetylase 7: a signalling hub controlling development, inflammation, metabolism and disease, FEBS J., 2023, 290(11), 2805–2832 CrossRef CAS PubMed.
- H. N. Kim, L. Han, S. Iyer, R. de Cabo, H. Zhao and C. A. O'Brien,
et al., Sirtuin1 Suppresses Osteoclastogenesis by Deacetylating FoxOs, Mol. Endocrinol., 2015, 29(10), 1498–1509 CrossRef CAS PubMed.
- Y. Zhang, Y. Liu, M. Hou, X. Xia, J. Liu and Y. Xu,
et al., Reprogramming of Mitochondrial Respiratory Chain Complex by Targeting SIRT3-COX4I2 Axis Attenuates Osteoarthritis Progression, Adv. Sci., 2023, 10(10), e2206144 CrossRef PubMed.
- M. L. Ji, H. Jiang, Z. Li, R. Geng, J. Z. Hu and Y. C. Lin,
et al., Sirt6 attenuates chondrocyte senescence and osteoarthritis progression, Nat. Commun., 2022, 13(1), 7658 CrossRef CAS PubMed.
- Z. Liu, N. Zhang, B. Xin, Y. Shi, Z. Liang and Y. Wan,
et al., Exosomes from LSD1 knockdown breast cancer cells activate osteoclastogenesis and inhibit osteoblastogenesis, Int. J. Biol. Macromol., 2023, 235, 123792 CrossRef CAS PubMed.
- D. Li, J. He, C. Fang, Y. Zhang, M. He and Z. Zhang,
et al., METTL3 Regulates Osteoclast Biological Behaviors via iNOS/NO-Mediated Mitochondrial Dysfunction in Inflammatory Conditions, Int. J. Mol. Sci., 2023, 24(2), 1403 CrossRef CAS PubMed.
- N. C. Huynh, V. Everts, P. Pavasant and R. S. Ampornaramveth, Inhibition of Histone Deacetylases Enhances the Osteogenic Differentiation of Human Periodontal Ligament Cells, J. Cell. Biochem., 2016, 117(6), 1384–1395 CrossRef CAS PubMed.
- L. Pham, B. Kaiser, A. Romsa, T. Schwarz, R. Gopalakrishnan and E. D. Jensen,
et al., HDAC3 and HDAC7 have opposite effects on osteoclast differentiation, J. Biol. Chem., 2011, 286(14), 12056–12065 CrossRef CAS PubMed.
- W. S. Lian, J. Y. Ko, Y. S. Chen, H. J. Ke, C. K. Hsieh and C. W. Kuo,
et al., MicroRNA-29a represses osteoclast formation and protects against osteoporosis by regulating PCAF-mediated RANKL and CXCL12, Cell Death Dis., 2019, 10(10), 705 CrossRef PubMed.
- J. H. Kim, K. Kim, I. Kim, S. Seong, J. T. Koh and N. Kim, Overexpression of Neurogenin 1 Negatively Regulates Osteoclast and Osteoblast Differentiation, Int. J. Mol. Sci., 2022, 23(12), 6708 CrossRef CAS PubMed.
- M. Deb, D. Laha, J. Maity and H. Das, SETD2-mediated epigenetic regulation of
noncanonical Wnt5A during osteoclastogenesis, Clin. Epigenet., 2021, 13(1), 192 CrossRef CAS PubMed.
- A. Garcia-Gomez, T. Li, M. Kerick, F. Català-Moll, N. R. Comet and J. Rodríguez-Ubreva,
et al., TET2- and TDG-mediated changes are required for the acquisition of distinct histone modifications in divergent terminal differentiation of myeloid cells, Nucleic Acids Res., 2017, 45(17), 10002–10017 CrossRef CAS PubMed.
- H. Sepulveda, A. Villagra and M. Montecino, Tet-Mediated DNA Demethylation Is Required for SWI/SNF-Dependent Chromatin Remodeling and Histone-Modifying Activities That Trigger Expression of the Sp7 Osteoblast Master Gene during Mesenchymal Lineage Commitment, Mol. Cell. Biol., 2017, 37(20), e00177-17 CrossRef PubMed.
- K. Kim, Y. Shin, J. Kim, T. S. Ulmer and W. An, H3K27me1 is essential for MMP-9-dependent H3N-terminal tail proteolysis during osteoclastogenesis, Epigenet. Chromatin, 2018, 11(1), 23 CrossRef PubMed.
- F. Cheng, H. Li, J. Liu, F. Yan, Y. Chen and H. Hu, EZH2 regulates the balance between osteoclast and osteoblast differentiation to inhibit arthritis-induced bone destruction, Genes Immunity, 2022, 23(3–4), 141–148 CrossRef CAS PubMed.
- Y. Gao and W. Ge, The histone methyltransferase DOT1L inhibits osteoclastogenesis and protects against osteoporosis, Cell Death Dis., 2018, 9(2), 33 CrossRef PubMed.
- J. H. Choi, A. R. Jang, M. J. Park, D. I. Kim and J. H. Park, Melatonin Inhibits Osteoclastogenesis and Bone Loss in Ovariectomized Mice by Regulating PRMT1-Mediated Signaling, Endocrinology, 2021, 162(6), bqab057 CrossRef PubMed.
- M. Ding, E. Cho, Z. Chen, S. W. Park and T. H. Lee, (S)-2-(Cyclobutylamino)-N-(3-(3,4-dihydroisoquinolin-2(1H)-yl)-2-hydroxypropyl)isonicotinamide Attenuates RANKL-Induced Osteoclast Differentiation by Inhibiting NF-κB Nuclear Translocation, Int. J. Mol. Sci., 2023, 24(5), 4327 CrossRef CAS PubMed.
- T. Yasui, J. Hirose, S. Tsutsumi, K. Nakamura, H. Aburatani and S. Tanaka, Epigenetic regulation of osteoclast differentiation: possible involvement of Jmjd3 in the histone demethylation of Nfatc1, J. Bone Min. Res., 2011, 26(11), 2665–2671 CrossRef CAS PubMed.
- M. Ding, Z. Chen, E. Cho, S. W. Park and T. H. Lee, Crucial Role of Lysine-Specific Histone Demethylase 1 in RANKL-Mediated Osteoclast Differentiation, Int. J. Mol. Sci., 2023, 24(4), 3605 CrossRef CAS PubMed.
- K. Doi, K. Murata, S. Ito, A. Suzuki, C. Terao and S. Ishie,
et al., Role of Lysine-Specific Demethylase 1 in Metabolically Integrating Osteoclast Differentiation and Inflammatory Bone Resorption Through Hypoxia-Inducible Factor 1α and E2F1, Arthritis Rheumatol., 2022, 74(6), 948–960 CrossRef CAS PubMed.
- S. J. Yi, Y. J. Jang, H. J. Kim, K. Lee, H. Lee and Y. Kim,
et al., The KDM4B-CCAR1-MED1 axis is a critical regulator of osteoclast differentiation and bone homeostasis, Bone Res., 2021, 9(1), 27 CrossRef CAS PubMed.
- J. Zhuang, H. Ning, M. Wang, W. Zhao, Y. Jing and X. Liu,
et al., Downregulated fat mass and obesity-associated protein inhibits bone resorption and osteoclastogenesis by nuclear factor-kappa B inactivation, Cell. Signalling, 2021, 87, 110137 CrossRef CAS PubMed.
- Y. Qiao, J. Li, D. Liu, C. Zhang, Y. Liu and S. Zheng, Identification and experimental validation of key m6A modification regulators as potential biomarkers of osteoporosis, Front. Genet., 2022, 13, 1072948 CrossRef CAS PubMed.
- F. Asa’ad, C. Garaicoa-Pazmiño, C. Dahlin and L. Larsson, Expression of MicroRNAs in Periodontal and Peri-Implant Diseases: A Systematic Review and Meta-Analysis, Int. J. Mol. Sci., 2020, 21(11), 4147 CrossRef PubMed.
- X. Wu, X. Chen, W. Mi, T. Wu, Q. Gu and H. Huang, MicroRNA sequence analysis identifies microRNAs associated with peri-implantitis in dogs, Biosci. Rep., 2017, 37(5), BSR20170768, DOI:10.1042/BSR20170768.
- R. Mueller, D. Bajric, H. G. Keceli, A. Keller, H. Dommisch and A. Elsharawy,
et al., hsa-miR-374b-5p regulates expression of the gene U2AF homology motif (UHM) kinase 1, J. Periodontal Res., 2021, 56(6), 1028–1036 CrossRef CAS PubMed.
- X. Zhang, Z. Zhao, Y. Chen, X. Han and Y. Jie, Extracellular vesicles secreted by human periodontal ligament induced osteoclast differentiation by transporting miR-28 to osteoclast precursor cells and further promoted orthodontic tooth movement, Int. Immunopharmacol., 2022, 113(Pt B), 109388 CrossRef CAS PubMed.
- N. Chen, B. D. Sui, C. H. Hu, J. Cao, C. X. Zheng and R. Hou,
et al., microRNA-21 Contributes to Orthodontic Tooth Movement, J. Dent. Res., 2016, 95(12), 1425–1433 CrossRef CAS PubMed.
- L. Wu, Y. Su, F. Lin, S. Zhu, J. Wang and Y. Hou,
et al., MicroRNA-21 promotes orthodontic tooth movement by modulating the RANKL/OPG balance in T cells, Oral Dis., 2020, 26(2), 370–380 CrossRef PubMed.
- C. H. Miller, S. M. Smith, M. Elguindy, T. Zhang, J. Z. Xiang and X. Hu,
et al., RBP-J-Regulated miR-182 Promotes TNF-α-Induced Osteoclastogenesis, J. Immunol., 2016, 196(12), 4977–4986 CrossRef CAS PubMed.
- J. B. Fordham, K. Guilfoyle, A. R. Naqvi and S. Nares, MiR-142-3p is a RANKL-dependent inducer of cell death in osteoclasts, Sci. Rep., 2016, 6, 24980 CrossRef CAS PubMed.
- H. Jiang, H. Kitaura, L. Liu, I. Mizoguchi and S. Liu, The miR-155-5p inhibits osteoclast differentiation through targeting CXCR2 in orthodontic root resorption, J. Periodontal Res., 2021, 56(4), 761–773 CrossRef CAS PubMed.
- J. Lian, X. Wu, Y. Liu, W. Qiu, X. Zhu and X. Wang,
et al., Potential roles of miR-335-5p on pathogenesis of experimental periodontitis, J. Periodontal Res., 2020, 55(2), 191–198 CrossRef CAS PubMed.
- J. Guo, X. Zeng, J. Miao, C. Liu, F. Wei and D. Liu,
et al., MiRNA-218 regulates osteoclast differentiation and inflammation response in periodontitis rats through Mmp9, Cell. Microbiol., 2019, 21(4), e12979 CrossRef PubMed.
- Y. Nakao, T. Fukuda, Q. Zhang, T. Sanui, T. Shinjo and X. Kou,
et al., Exosomes from TNF-α-treated human gingiva-derived MSCs enhance M2 macrophage polarization and inhibit periodontal bone loss, Acta Biomater., 2021, 122, 306–324 CrossRef CAS PubMed.
- C. Hayashi, T. Fukuda, K. Kawakami, M. Toyoda, Y. Nakao and Y. Watanabe,
et al., miR-1260b inhibits periodontal bone loss by targeting ATF6β mediated regulation of ER stress, Front. Cell Dev. Biol., 2022, 10, 1061216 CrossRef PubMed.
- X. Zhang, Y. Zhao, Z. Zhao, X. Han and Y. Chen, Knockdown of DANCR reduces osteoclastogenesis and root resorption induced by compression force via Jagged1, Cell Cycle, 2019, 18(15), 1759–1769 CrossRef CAS PubMed.
- J. Li, F. Jin, M. Cai, T. Lin, X. Wang and Y. Sun, LncRNA Nron Inhibits Bone Resorption in Periodontitis, J. Dent. Res., 2022, 101(2), 187–195 CrossRef CAS PubMed.
- Y. Xin, Y. Liu, J. Li, D. Liu, C. Zhang and Y. Wang,
et al., A Novel lncRNA Mediates the Delayed Tooth Eruption of Cleidocranial Dysplasia, Cells, 2022, 11(17), 2729 CrossRef CAS PubMed.
- C. Zhang, L. Pan, H. Zhang, T. Ke, Y. Yang and L. Zhang,
et al., Osteoblasts-Derived Exosomal lncRNA-MALAT1 Promotes Osteoclastogenesis by Targeting the miR-124/NFATc1 Signaling Axis in Bone Marrow-Derived Macrophages, Int. J. Nanomed., 2023, 18, 781–795 CrossRef CAS PubMed.
- S. Campillo, L. Bohorquez, E. Gutiérrez-Calabrés, D. García-Ayuso, V. Miguel and M. Griera,
et al., Indoxyl sulfate- and P-cresol-induced monocyte adhesion and migration is mediated by integrin-linked kinase-dependent podosome formation, Exp. Mol. Med., 2022, 54(3), 226–238 CrossRef CAS PubMed.
- F. Hu, D. Zhu, H. Dong, P. Zhang, F. Xing and W. Li,
et al., Super-resolution microscopy reveals nanoscale architecture and regulation of podosome clusters in primary macrophages, iScience, 2022, 25(12), 105514 CrossRef PubMed.
- M. Portes, T. Mangeat, N. Escallier, O. Dufrancais, B. Raynaud-Messina and C. Thibault,
et al., Nanoscale architecture and coordination of actin cores within the sealing zone of human osteoclasts, eLife, 2022, 11, e75610 CrossRef CAS PubMed.
- A. E. I. Hamouda, C. Schalla, A. Sechi, M. Zenke, R. K. Schneider and T. Hieronymus, Met-Signaling Controls Dendritic Cell Migration in Skin by Regulating Podosome Formation and Function, J. Invest. Dermatol., 2023, 143(8), 1548–1558 CrossRef CAS PubMed.
- C. Cougoule, C. Lastrucci, R. Guiet, R. Mascarau, E. Meunier and G. Lugo-Villarino,
et al., Podosomes, But Not the Maturation Status, Determine the Protease-Dependent 3D Migration in Human Dendritic Cells, Front. Immunol., 2018, 9, 846 CrossRef PubMed.
- Y. L. Wu, C. H. Zhang, Y. Teng, Y. Pan, N. C. Liu and P. X. Liu,
et al., Propionate and butyrate attenuate macrophage pyroptosis and osteoclastogenesis induced by CoCrMo alloy particles, Mil. Med. Res., 2022, 9(1), 46 CAS.
- P. Swiatlowska, B. Sit, Z. Feng, E. Marhuenda, I. Xanthis and S. Zingaro,
et al., Pressure and stiffness sensing together regulate vascular smooth muscle cell phenotype switching, Sci. Adv., 2022, 8(15), eabm3471 CrossRef CAS PubMed.
- S. Linder, P. Cervero, R. Eddy and J. Condeelis, Mechanisms and roles of podosomes and invadopodia, Nat. Rev. Mol. Cell Biol., 2023, 24(2), 86–106 CrossRef CAS PubMed.
- S. Huveneers, S. Arslan, B. van de Water, A. Sonnenberg and E. H. Danen, Integrins uncouple Src-induced morphological and oncogenic transformation, J. Biol. Chem., 2008, 283(19), 13243–13251 CrossRef CAS PubMed.
- G. Burgstaller and M. Gimona, Actin cytoskeleton remodelling via local inhibition of contractility at discrete microdomains, J. Cell Sci., 2004, 117(Pt 2), 223–231 CrossRef CAS PubMed.
- I. Kaverina, T. E. Stradal and M. Gimona, Podosome formation in cultured A7r5 vascular smooth muscle cells requires Arp2/3-dependent de-novo actin polymerization at discrete microdomains, J. Cell Sci., 2003, 116(Pt 24), 4915–4924 CrossRef CAS PubMed.
- Z. Li, D. He, B. Guo, Z. Wang, H. Yu and Y. Wang,
et al., Self-promoted electroactive biomimetic mineralized scaffolds for bacteria-infected bone regeneration, Nat. Commun., 2023, 14(1), 6963 CrossRef CAS PubMed.
- Y. Zhou, Z. Feng, F. Cao, X. Liu, X. Xia and C. H. Yu, Abl-mediated PI3K activation regulates macrophage podosome formation, J. Cell Sci., 2020, 133(11), jcs234385 CrossRef CAS PubMed.
- G. Leung, Y. Zhou, P. Ostrowski, S. Mylvaganam, P. Boroumand and D. J. Mulder,
et al., ARPC1B binds WASP to control actin polymerization and curtail tonic signaling in B cells, JCI Insight, 2021, 6(23), e149376 CrossRef PubMed.
- R. Bhuwania, S. Cornfine, Z. Fang, M. Krüger, E. J. Luna and S. Linder, Supervillin couples myosin-dependent contractility to podosomes and enables their turnover, J. Cell Sci., 2012, 125(Pt 9), 2300–2314 CAS.
- A. S. Cabron, K. El Azzouzi, M. Boss, P. Arnold, J. Schwarz and M. Rosas,
et al., Structural and Functional Analyses of the Shedding Protease ADAM17 in HoxB8-Immortalized Macrophages and Dendritic-like Cells, J. Immunol., 2018, 201(10), 3106–3118 CrossRef CAS PubMed.
- S. Razzouk, M. Lieberherr and G. Cournot, Rac-GTPase, osteoclast cytoskeleton and bone resorption, Eur. J. Cell Biol., 1999, 78(4), 249–255 CrossRef CAS PubMed.
- M. Croke, F. P. Ross, M. Korhonen, D. A. Williams, W. Zou and S. L. Teitelbaum, Rac deletion in osteoclasts causes severe osteopetrosis, J. Cell Sci., 2011, 124(Pt 22), 3811–3821 CrossRef CAS PubMed.
- O. Destaing, F. Saltel, B. Gilquin, A. Chabadel, S. Khochbin and S. Ory,
et al., A novel Rho-mDia2-HDAC6 pathway controls podosome patterning through microtubule acetylation in osteoclasts, J. Cell Sci., 2005, 118(Pt 13), 2901–2911 CrossRef CAS PubMed.
- H. Liao, Q. Tu, Y. Kang, G. Mao, Z. Li and S. Hu,
et al., CircNFIX regulates chondrogenesis and cartilage homeostasis by targeting the miR758-3p/KDM6A axis, Cell Proliferation, 2022, 55(11), e13302 CrossRef CAS PubMed.
- A. Balabiyev, N. P. Podolnikova, A. Mursalimov, D. Lowry, J. M. Newbern and R. W. Roberson,
et al., Transition of podosomes into zipper-like structures in macrophage-derived multinucleated giant cells, Mol. Biol. Cell, 2020, 31(18), 2002–2020 CrossRef CAS PubMed.
- A. Chabadel, I. Bañon-Rodríguez, D. Cluet, B. B. Rudkin, B. Wehrle-Haller and E. Genot,
et al., CD44 and beta3 integrin organize two functionally distinct actin-based domains in osteoclasts, Mol. Biol. Cell, 2007, 18(12), 4899–4910 CrossRef CAS PubMed.
- D. Geblinger, B. Geiger and L. Addadi, Surface-induced regulation of podosome organization and dynamics in cultured osteoclasts, ChemBioChem, 2009, 10(1), 158–165 CrossRef CAS PubMed.
- A. Juin, J. Di Martino, B. Leitinger, E. Henriet, A. S. Gary and L. Paysan,
et al., Discoidin domain receptor 1 controls linear invadosome formation via a Cdc42-Tuba pathway, J. Cell Biol., 2014, 207(4), 517–533 CrossRef CAS PubMed.
- K. Pal, Y. Zhao, Y. Wang and X. Wang, Ubiquitous membrane-bound DNase activity in podosomes and invadopodia, J. Cell Biol., 2021, 220(7), e202008079 CrossRef CAS PubMed.
- K. El Azzouzi, C. Wiesner and S. Linder, Metalloproteinase MT1-MMP islets act as memory devices for podosome reemergence. The, J. Cell Biol., 2016, 213(1), 109–125 CrossRef CAS PubMed.
- Y. D. Bansod, M. Kebbach, D. Kluess, R. Bader and U. van Rienen, Computational Analysis of Bone Remodeling in the Proximal Tibia Under Electrical Stimulation Considering the Piezoelectric Properties, Front. Bioeng. Biotechnol., 2021, 9, 705199 CrossRef PubMed.
- S. Ramtani, Electro-mechanics of bone remodelling, Int. J. Eng. Sci., 2008, 46(11), 1173–1182 CrossRef.
- C. Witt, T. Kaiser and A. Menzel, Modelling and numerical simulation of remodelling processes in cortical bone: An IGA approach to flexoelectricity-induced osteocyte apoptosis and subsequent bone cell diffusion, J. Mech. Phys. Solids, 2023, 173, 105194 CrossRef CAS.
- L. Bergara-Muguruza, K. Mäkelä, T. Yrjälä, J. Salonen, K. Yamashita and M. Nakamura, Surface Electric Fields Increase Human Osteoclast Resorption through Improved Wettability on Carbonate-Incorporated Apatite, ACS Appl. Mater. Interfaces, 2021, 13(49), 58270–58278 CrossRef CAS PubMed.
- C. H. Yao, B. Y. Yang and Y. E. Li, Remodeling Effects of the Combination of GGT Scaffolds, Percutaneous Electrical Stimulation, and Acupuncture on Large Bone Defects in Rats, Front. Bioeng. Biotechnol., 2022, 10, 832808 CrossRef PubMed.
- X. Zhang, T. Wang, Z. Zhang, H. Liu, L. Li and A. Wang,
et al., Electrical stimulation system based on electroactive biomaterials for bone tissue engineering, Mater. Today, 2023, 68, 177–203 CrossRef CAS.
- L. Leppik, K. M. C. Oliveira, M. B. Bhavsar and J. H. Barker, Electrical stimulation in bone tissue engineering treatments, Eur. J. Trauma Emerg. Surg., 2020, 46(2), 231–244 CrossRef PubMed.
- J. I. Hoare, A. M. Rajnicek, C. D. McCaig, R. N. Barker and H. M. Wilson, Electric fields are novel determinants of human macrophage functions, J. Leukocyte Biol., 2016, 99(6), 1141–1151 CrossRef CAS PubMed.
- J. Liu, S. Li, S. Zhou, Z. Chen, J. Xu and N. Cui,
et al., A high-performance, biocompatible, and fully biodegradable piezo-triboelectric hybrid nanogenerator based on PVA/Glycine/PVA heterostructured piezoelectric film, Nano Energy, 2024, 122, 109310 CrossRef CAS.
- J. Liu, J. Jiang, C. G. X. Deng, X. S. Huang, S. Huang and Z. J. Liu,
et al., Nanochannel Electro-Injection as a Versatile Platform for Efficient RNA/DNA Programming on Dendritic Cells, Small, 2023, 19(43), e2303088 CrossRef PubMed.
- T. Zhou, L. Yan, C. Xie, P. Li, L. Jiang and J. Fang,
et al., A Mussel-Inspired Persistent ROS-Scavenging, Electroactive, and Osteoinductive Scaffold Based on Electrochemical-Driven In Situ Nanoassembly, Small, 2019, 15(25), e1805440 CrossRef PubMed.
- X. Long, X. Wang, L. Yao, S. Lin, J. Zhang and W. Weng,
et al., Graphene/Si-Promoted Osteogenic Differentiation of BMSCs through Light Illumination, ACS Appl. Mater. Interfaces, 2019, 11(47), 43857–43864 CrossRef CAS PubMed.
- X. Dai, B. C. Heng, Y. Bai, F. You, X. Sun and Y. Li,
et al., Restoration of electrical microenvironment enhances bone regeneration under diabetic conditions by modulating macrophage polarization, Bioact. Mater., 2021, 6(7), 2029–2038 CAS.
- P. Kazimierczak, M. Koziol and A. Przekora, The Chitosan/Agarose/NanoHA Bone Scaffold-Induced M2 Macrophage Polarization and Its Effect on Osteogenic Differentiation In Vitro, Int. J. Mol. Sci., 2021, 22(3), 1109 CrossRef CAS PubMed.
- Y. Jiang, W. Zhao, S. Xu, J. Wei, F. L. Lasaosa and Y. He,
et al., Bioinspired design of mannose-decorated globular lysine dendrimers promotes diabetic wound healing by orchestrating appropriate macrophage polarization, Biomaterials, 2022, 280, 121323 CrossRef CAS PubMed.
- Y. Li, L. Yang, Y. Hou, Z. Zhang, M. Chen and M. Wang,
et al., Polydopamine-mediated graphene oxide and nanohydroxyapatite-incorporated conductive scaffold with an immunomodulatory ability accelerates periodontal bone regeneration in diabetes, Bioact. Mater., 2022, 18, 213–227 CAS.
- S. Zhang, B. Ma, F. Liu, J. Duan, S. Wang and J. Qiu,
et al., Polylactic Acid Nanopillar Array-Driven Osteogenic Differentiation of Human Adipose-Derived Stem Cells Determined by Pillar Diameter, Nano Lett., 2018, 18(4), 2243–2253 CrossRef CAS PubMed.
- Y. Zhang, K. Wang, Y. Song, E. Feng, K. Dong and Y. Han,
et al., Ca substitution of Sr in Sr-doped TiO2 nanotube film on Ti surface for enhanced osteogenic activity, Appl. Surf. Sci., 2020, 528, 147055 CrossRef CAS.
- L. Bai, P. Chen, Y. Zhao, R. Hang, X. Yao and B. Tang,
et al., A micro/nano-biomimetic coating on titanium orchestrates osteo/angio-genesis and osteoimmunomodulation for advanced osseointegration, Biomaterials, 2021, 278, 121162 CrossRef CAS PubMed.
- N. Jiang, Z. Guo, D. Sun, Y. Li, Y. Yang and C. Chen,
et al., Promoting Osseointegration of Ti Implants through Micro/Nanoscaled Hierarchical Ti Phosphate/Ti Oxide Hybrid Coating, ACS Nano, 2018, 12(8), 7883–7891 CrossRef CAS PubMed.
- J. Ye, B. Li, M. Li, Y. Zheng, S. Wu and Y. Han, ROS induced bactericidal activity of amorphous Zn-doped titanium oxide coatings and enhanced osseointegration in bacteria-infected rat tibias, Acta Biomater., 2020, 107, 313–324 CrossRef CAS PubMed.
- J. Jenkins, J. Mantell, C. Neal, A. Gholinia, P. Verkade and A. H. Nobbs,
et al., Antibacterial effects of nanopillar surfaces are mediated by cell impedance, penetration and induction of oxidative stress, Nat. Commun., 2020, 11(1), 1626 CrossRef CAS PubMed.
- G. Zhu, G. Wang and J. J. Li, Advances in implant surface modifications to improve osseointegration, Mater. Adv., 2021, 2(21), 6901–6927 RSC.
- X. Miao, D. Wang, L. Xu, J. Wang, D. Zeng and S. Lin,
et al., The response of human osteoblasts, epithelial cells, fibroblasts, macrophages and oral bacteria to nanostructured titanium surfaces: a systematic study, Int. J. Nanomed., 2017, 12, 1415–1430 CrossRef CAS PubMed.
- I. P. Torres-Avila, I. I. Padilla-Martínez, N. Pérez-Hernández, A. E. Bañuelos-Hernández, J. C. Velázquez and J. L. Castrejón-Flores,
et al., Surface Modification of the Ti-6Al-4V Alloy by Anodic Oxidation and Its Effect on Osteoarticular Cell Proliferation, Coatings, 2020, 10(5), 491 CrossRef CAS.
- R. Xu, X. Cui, Q. Xin, M. Lu, Z. Li and J. Li,
et al., Zwitterionic PMCP-functionalized titanium surface resists protein adsorption, promotes cell adhesion, and enhances osteogenic activity, Colloids Surf., B, 2021, 206, 111928 CrossRef CAS PubMed.
- J. Yang, H. Chen, S. Xiao, M. Shen, F. Chen and P. Fan,
et al., Salt-Responsive Zwitterionic Polymer Brushes with Tunable Friction and Antifouling Properties, Langmuir, 2015, 31(33), 9125–9133 CrossRef CAS PubMed.
- Y. He, J. Hower, S. Chen, M. T. Bernards, Y. Chang and S. Jiang, Molecular Simulation Studies of Protein Interactions with Zwitterionic Phosphorylcholine Self-Assembled Monolayers in the Presence of Water, Langmuir, 2008, 24(18), 10358–10364 CrossRef CAS PubMed.
- J. Kodama, H. Chen, T. Zhou, J. Kushioka, R. Okada and H. Tsukazaki,
et al., Antibacterial efficacy of quaternized chitosan coating on 3D printed titanium cage in rat intervertebral disc space, Spine J., 2021, 21(7), 1217–1228 CrossRef PubMed.
- A. Niemczyk, A. Goszczyńska, M. Gołda-Cępa, A. Kotarba, P. Sobolewski and M. El Fray, Biofunctional catheter coatings based on chitosan-fatty acids derivatives, Carbohydr. Polym., 2019, 225, 115263 CrossRef CAS PubMed.
- Y. Tan, M. Leonhard, S. Ma, D. Moser and B. Schneider-Stickler, Efficacy of carboxymethyl chitosan against Candida tropicalis and Staphylococcus epidermidis monomicrobial and polymicrobial biofilms, Int. J. Biol. Macromol., 2018, 110, 150–156 CrossRef CAS PubMed.
- K. Yang, K. Kim, E. Lee, S. Liu, S. Kabli and S. Alsudir,
et al., Robust Low Friction Antibiotic Coating of Urethral Catheters Using a Catechol-Functionalized Polymeric Hydrogel Film, Front. Mater., 2019, 6, 274 CrossRef.
- H. C. Li, D. G. Wang, C. Hu, J. H. Dou, H. J. Yu and C. Z. Chen, Effect of Na(2)O and ZnO on the microstructure and properties of laser cladding derived CaO-SiO(2) ceramic coatings on titanium alloys, J. Colloid Interface Sci., 2021, 592, 498–508 CrossRef CAS PubMed.
- I. Ullah, M. A. Siddiqui, H. Liu, S. K. Kolawole, J. Zhang and S. Zhang,
et al., Mechanical, Biological, and Antibacterial Characteristics of Plasma-Sprayed (Sr,Zn) Substituted Hydroxyapatite Coating, ACS Biomater. Sci. Eng., 2020, 6(3), 1355–1366 CrossRef CAS PubMed.
- D. Ke, A. A. Vu, A. Bandyopadhyay and S. Bose, Compositionally graded doped hydroxyapatite coating on titanium using laser and plasma spray deposition for bone implants, Acta Biomater., 2019, 84, 414–423 CrossRef CAS PubMed.
- H. Liu, R. Liu, I. Ullah, S. Zhang, Z. Sun and L. Ren,
et al., Rough surface of copper-bearing titanium alloy with multifunctions
of osteogenic ability and antibacterial activity, J. Mater. Sci. Technol., 2020, 48, 130–139 CrossRef CAS.
- L. Bai, Z. Du, J. Du, W. Yao, J. Zhang and Z. Weng,
et al., A multifaceted coating on titanium dictates osteoimmunomodulation and osteo/angio-genesis towards ameliorative osseointegration, Biomaterials, 2018, 162, 154–169 CrossRef CAS PubMed.
- W. Zhou, Z. Jia, P. Xiong, J. Yan, M. Li and Y. Cheng,
et al., Novel pH-responsive tobramycin-embedded micelles in nanostructured multilayer-coatings of chitosan/heparin with efficient and sustained antibacterial properties, Mater. Sci. Eng., C, 2018, 90, 693–705 CrossRef CAS PubMed.
- Y. Tan, S. Ma, M. Leonhard, D. Moser, R. Ludwig and B. Schneider-Stickler, Co-immobilization of cellobiose dehydrogenase and deoxyribonuclease I on chitosan nanoparticles against fungal/bacterial polymicrobial biofilms targeting both biofilm matrix and microorganisms, Mater. Sci. Eng., C, 2020, 108, 110499 CrossRef CAS PubMed.
- A. Valverde, L. Pérez-Álvarez, L. Ruiz-Rubio, M. A. Pacha Olivenza, M. B. García Blanco and M. Díaz-Fuentes,
et al., Antibacterial hyaluronic acid/chitosan multilayers onto smooth and micropatterned titanium surfaces, Carbohydr. Polym., 2019, 207, 824–833 CrossRef CAS PubMed.
- L. L. Guo, Y. F. Cheng, X. Ren, K. Gopinath, Z. S. Lu and C. M. Li,
et al., Simultaneous deposition of tannic acid and poly(ethylene glycol) to construct the antifouling polymeric coating on Titanium surface, Colloids Surf., B, 2021, 200, 111592 CrossRef CAS PubMed.
- M. Bottagisio, V. Balzano, L. Ciambriello, L. Rosa, G. Talò and A. B. Lovati,
et al., Exploring multielement nanogranular coatings to forestall implant-related infections, Front. Cell. Infect. Microbiol., 2023, 13, 1128822 CrossRef CAS PubMed.
- T. Ding, W. Kang, J. Li, L. Yu and S. Ge, An in situ tissue engineering scaffold with growth factors combining angiogenesis and osteoimmunomodulatory functions for advanced periodontal bone regeneration, J. Nanobiotechnol., 2021, 19(1), 247 CrossRef CAS PubMed.
- S. Zhao, Y. Xu, W. Xu, Z. Weng, F. Cao and X. Wan,
et al., Tremella-Like ZnO@Col-I-Decorated Titanium Surfaces with Dual-Light-Defined Broad-Spectrum Antibacterial and Triple Osteogenic Properties, ACS Appl. Mater. Interfaces, 2020, 12(27), 30044–30051 CrossRef CAS PubMed.
- Z. Wang, Y. Niu, X. Tian, N. Yu, X. Yin and Z. Xing,
et al., Switching On and Off Macrophages by a “Bridge-Burning” Coating Improves Bone-Implant Integration under Osteoporosis, Adv. Funct. Mater., 2021, 31(7), 2007408 CrossRef CAS.
- R. S. B. Lee, S. M. Hamlet, H. J. Moon and S. Ivanovski, Re-establishment of macrophage homeostasis by titanium surface modification in type II diabetes promotes osseous healing, Biomaterials, 2021, 267, 120464 CrossRef CAS PubMed.
- Y. Wei, Z. Liu, X. Zhu, L. Jiang, W. Shi and Y. Wang,
et al., Dual directions to address the problem of aseptic loosening via electrospun PLGA @ aspirin nanofiber coatings on titanium, Biomaterials, 2020, 257, 120237 CrossRef CAS PubMed.
- Y. L. Yu, J. J. Wu, C. C. Lin, X. Qin, F. R. Tay and L. Miao,
et al., Elimination of methicillin-resistant Staphylococcus aureus biofilms on titanium implants via photothermally-triggered nitric oxide and immunotherapy for enhanced osseointegration, Mil. Med. Res., 2023, 10(1), 21 CAS.
- L. Liu, X. Wang, Y. Zhou, M. Cai, K. Lin and B. Fang,
et al., The synergistic promotion of osseointegration by nanostructure design and silicon substitution of hydroxyapatite coatings in a diabetic model, J. Mater. Chem. B, 2020, 8(14), 2754–2767 RSC.
- J. Cui, L. Xia, K. Lin and X. Wang, In situ construction of a nano-structured akermanite coating for promoting bone formation and osseointegration of Ti-6Al-4V implants in a rabbit osteoporosis model, J. Mater. Chem. B, 2021, 9(46), 9505–9513 RSC.
- S. Lee, Y. Y. Chang, J. Lee, S. K. Madhurakkat Perikamana, E. M. Kim and Y. H. Jung,
et al., Surface engineering of titanium alloy using metal-polyphenol network coating with magnesium ions for improved osseointegration, Biomater. Sci., 2020, 8(12), 3404–3417 RSC.
- B. Chen, Y. Liang, Y. Song, Y. Liang, J. Jiao and H. Bai,
et al., Photothermal-Controlled Release of IL-4 in IL-4/PDA-Immobilized Black Titanium Dioxide (TiO(2)) Nanotubes Surface to Enhance Osseointegration: An In Vivo Study, Materials, 2022, 15(17), 5962 CrossRef CAS PubMed.
- H. Schell, J. Lienau, D. R. Epari, P. Seebeck, C. Exner and S. Muchow,
et al., Osteoclastic activity begins early and increases over the course of bone healing, Bone, 2006, 38(4), 547–554 CrossRef PubMed.
- C. S. Hsu, S. L. Haag, M. T. Bernards and Q. Li, Evaluation of chlorine substituted hydroxyapatite (ClHAP)/polydopamine composite coatings on Ti64, Colloids Surf., B, 2020, 189, 110799 CrossRef CAS PubMed.
- Z. Geng, Y. Yu, Z. Li, L. Ma, S. Zhu and Y. Liang,
et al., miR-21 promotes osseointegration and mineralization through enhancing both osteogenic and osteoclastic expression, Mater. Sci. Eng., C, 2020, 111, 110785 CrossRef CAS PubMed.
- E. Mohammed, E. Khalil and D. Sabry, Effect of Adipose-Derived Stem Cells and Their Exo as Adjunctive Therapy to Nonsurgical Periodontal Treatment: A Histologic and Histomorphometric Study in Rats, Biomolecules, 2018, 8(4), 167 CrossRef PubMed.
- L. Liu, S. Guo, W. Shi, Q. Liu, F. Huo and Y. Wu,
et al., Bone Marrow Mesenchymal Stem Cell-Derived Small Extracellular Vesicles Promote Periodontal Regeneration, Tissue Eng., Part A, 2021, 27(13–14), 962–976 CrossRef CAS PubMed.
- Z. Shen, S. Kuang, Y. Zhang, M. Yang, W. Qin and X. Shi,
et al., Chitosan hydrogel incorporated with dental pulp stem cell-derived exosomes alleviates periodontitis in mice via a macrophage-dependent mechanism, Bioact. Mater., 2020, 5(4), 1113–1126 Search PubMed.
- J. Zarubova, M. M. Hasani-Sadrabadi, E. Dashtimoghadam, X. Zhang, S. Ansari and S. Li,
et al., Engineered Delivery of Dental Stem-Cell-Derived Extracellular Vesicles for Periodontal Tissue Regeneration, Adv. Healthcare Mater., 2022, 11(12), e2102593 CrossRef PubMed.
- Y. Huang, Q. Liu, L. Liu, F. Huo, S. Guo and W. Tian, Lipopolysaccharide-Preconditioned Dental Follicle Stem Cells Derived Small Extracellular Vesicles Treating Periodontitis via Reactive Oxygen Species/Mitogen-Activated Protein Kinase Signaling-Mediated Antioxidant Effect, Int. J. Nanomed., 2022, 17, 799–819 CrossRef CAS PubMed.
- X. Chen, Z. Wan, L. Yang, S. Song, Z. Fu and K. Tang,
et al., Exosomes derived from reparative M2-like macrophages prevent bone loss in murine periodontitis models via IL-10 mRNA, J. Nanobiotechnol., 2022, 20(1), 110 CrossRef CAS PubMed.
- L. Ren, F. Zeng, J. Deng, Y. Bai, K. Chen and L. Chen,
et al., Inflammatory osteoclasts-derived exosomes promote bone formation by selectively transferring lncRNA LIOCE into osteoblasts to interact with and stabilize Osterix, FASEB J., 2022, 36(2), e22115 CrossRef CAS PubMed.
- Z. Hao, L. Ren, Z. Zhang, Z. Yang, S. Wu and G. Liu,
et al., A multifunctional neuromodulation platform utilizing Schwann cell-derived exosomes orchestrates bone microenvironment via immunomodulation, angiogenesis and osteogenesis, Bioact. Mater., 2023, 23, 206–222 CAS.
- R. Nakao, H. Hasegawa, B. Dongying, M. Ohnishi and H. Senpuku, Assessment of outer membrane vesicles of periodontopathic bacterium Porphyromonas gingivalis as possible mucosal immunogen, Vaccine, 2016, 34(38), 4626–4634 CrossRef CAS PubMed.
- W. Huang, Q. Zhang, W. Li, M. Yuan, J. Zhou and L. Hua,
et al., Development
of novel nanoantibiotics using an outer membrane vesicle-based drug efflux mechanism, J. Controlled Release, 2020, 317, 1–22 CrossRef CAS PubMed.
- K. Sundaram, D. P. Miller, A. Kumar, Y. Teng, M. Sayed and J. Mu,
et al., Plant-Derived Exosomal Nanoparticles Inhibit Pathogenicity of Porphyromonas gingivalis, iScience, 2019, 21, 308–327 CrossRef CAS PubMed.
- M. K. Neog, F. Sultana and M. Rasool, Targeting RAW 264.7 macrophages (M1 type) with Withaferin-A decorated mannosylated liposomes induces repolarization via downregulation of NF-κB and controlled elevation of STAT-3, Int. Immunopharmacol., 2018, 61, 64–73 CrossRef CAS PubMed.
- H. Li, Y. Feng, X. Zheng, M. Jia, Z. Mei and Y. Wang,
et al., M2-type exosomes nanoparticles for rheumatoid arthritis therapy via macrophage re-polarization, J. Controlled Release, 2022, 341, 16–30 CrossRef CAS PubMed.
- B. Zhang, F. Han, Y. Wang, Y. Sun, M. Zhang and X. Yu,
et al., Cells-Micropatterning Biomaterials for Immune Activation and Bone Regeneration, Adv. Sci., 2022, 9(18), e2200670 CrossRef PubMed.
- H. Y. Dar, S. Pal, P. Shukla, P. K. Mishra, G. B. Tomar and N. Chattopadhyay,
et al., Bacillus clausii inhibits bone loss by skewing Treg-Th17 cell equilibrium in postmenopausal osteoporotic mice model, Nutrition, 2018, 54, 118–128 CrossRef CAS PubMed.
Footnotes |
† All claims expressed in this review are solely those of the authors and do not necessarily represent those of their affiliated organizations, or those of the Royal Society of Chemistry, the editors and the reviewers. Any product that may be evaluated in this review, or claim that may be made by its manufacturer, is not guaranteed or endorsed by the Royal Society of Chemistry. |
‡ Co-first authors: Zijun Chen and Yuxi Wang. |
|
This journal is © The Royal Society of Chemistry 2024 |