DOI:
10.1039/D3MA00706E
(Review Article)
Mater. Adv., 2024,
5, 68-82
Carbohydrate polymer-supported metal and metal oxide nanoparticles for constructing electrochemical sensors
Received
14th September 2023
, Accepted 29th November 2023
First published on 30th November 2023
Abstract
Carbohydrate polymers have attracted significant attention due to their favorable characteristics, such as availability, non-toxicity, biocompatibility, and biodegradability, making them promising materials for many medical and industrial applications. Additionally, they can be reinforced with metal and metal oxide nanoparticles (NPs), resulting in the formation of nanocomposites (NCs), which can be incorporated in electrochemical sensors for enhancing their conductivity and catalytic activity. These NPs increase the catalytic activity of electrochemical sensors via redox cycling, while carbohydrate polymers are used to stabilize NPs against self-aggregation and act as matrix for them. The redox cycling process, which depends on NPs as redox mediators, facilitates the oxidation or reduction of the target analytes, leading to an optimized current signal. Carbohydrate polymers are more favorable than synthetic polymers given that they are readily available, cheap, and non-toxic materials. To date, various carbohydrate polymers, such as alginate, cellulose, chitosan, dextran, gums, locust bean gum, latex, mucilage, pectin, and starch, have been used for the preparation of electroactive metal/metal oxide–carbohydrate polymer NCs. Subsequently, these NCs have been employed for the detection of biological analytes, such as drugs, enzymes, hormones, aflatoxins, biomarkers, carbohydrates, and natural oxidants, and water pollutants, such as pesticides, dyes, metal ions, and phenolics. However, there are fewer studies regarding the use of carbohydrate polymers in electrochemical sensors compared with other applications. Overall, metal/metal oxide–carbohydrate polymers are considered promising materials for the detection of many target analytes with enhanced sensitivity, low detection limits, and optimized selectivity.
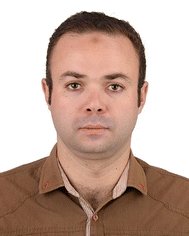
Moustafa Zahran
| Moustafa Zahran earned his Bachelor's Degree in Chemistry (2012), his Master's Degree in Organic Chemistry (2016), and PhD in Organic Electrochemistry (2022) from El-Menoufia University, Shebin El-Kom, Egypt. He is the Manager of Water Safety Management, Lab Sector, Menoufia Company for Water and Wastewater (MCWW), Holding Company for Water and Wastewater (HCWW), Egypt. His research is focused on the construction of electrochemical sensors based on metallic nanoparticles to detect organic pollutants in surface water. Currently, he is working on the development of water and wastewater treatment technologies. |
Introduction
Nanomaterials are widely used in biomedical, environmental, and industrial applications.1–3 Nowadays, a great attention has been focused on nanomaterials, especially with a size in the range of 1–100 nm, as electrode modifiers due to their high surface energy, specific surface area, reactivity, and superb catalytic activity compared to their bulk counterparts.4 Additionally, they show superior chemical, physical, mechanical, and electronic characteristics.5,6 Consequently, nanomaterials play a crucial role in the construction of high-performance electrochemical sensors.
Nanomaterial-based electrochemical sensors are robust analytical tools that show high sensitivity, selectivity, ease of miniaturization, and rapid response compared to conventional electrochemical sensors.7 Thus, they have been extensively used for the detection of biological molecules, such as drugs, enzymes, biomarkers, hormones, and toxins.8 Additionally, they have been used for the detection of water pollutants, such as organic pollutants9–11 and heavy metals.12
A variety of nanomaterials, including zero-dimensional, one-dimensional, two-dimensional, and hybrid nanomaterials, has been used in chemical- and bio-sensing.13 Zero-dimensional nanomaterials, including metal and metal oxide nanoparticles (NPs), are considered brilliant electrode modifiers due to their high surface area and catalytic activity.
Metal NPs and their oxides are broadly used in various applications, such as electronics, optics, information technologies, and batteries, because of their large specific surface area, adsorption activity, and high catalytic activity.14 Additionally, they are extensively used in biomedicine and environmental applications due to their favorable optical, chemical, mechanical, electrical, and magnetic characteristics.15–18 These NPs can be produced using a variety of physical, chemical, and biological methods.19 Biological methods are regarded as a green route for the synthesis of metal and metal oxide NPs without requiring harmful chemicals and reagents.20,21 They depend on the use of biological resources such as plants,22 animals,23 algae,24 and fungi.25 Plants represent a valuable source of carbohydrate polymers, which act as powerful reducing and capping agents for the synthesis of metal– and metal oxide–carbohydrate polymer nanocomposites (NCs). Alternatively, the as-synthesized NPs can be dispersed in carbohydrate polymers to form the NCs.
Carbohydrate polymers have attracted significant attention considering that they are bio-renewable and greener materials with diverse physical and chemical characteristics, making them good candidates for many medical and industrial applications.26–30 The incorporation of metal NPs as nanofillers in carbohydrate polymers, such as chitin, chitosan, alginate, dextran, and gums, has significantly improved the features of carbohydrate polymers.31 Consequently, metal NP–carbohydrate polymer composites have been used in many industrial and biological applications.32 For example, the removal of metal ions and dyes from water.33 Moreover, conducting polymers such as carbohydrate polymers reinforced with metal-based NPs have been used in electrochemical sensors.34
Recently, a review article was published on metal/metal oxide NPs-carbohydrate polymers as promising materials in industrial and biological applications.32 Alternatively, the present review is focused on the use of reactive metal and metal oxide NPs, which are easily oxidized and reduced on the electrode surface, capped by carbohydrate polymers as an efficient strategy for the electrochemical detection of different analytes especially biological molecules and water pollutants for the first time.
Electrochemical sensors
Electrochemistry is a branch of science related to the oxidation and reduction of substances on the surface of electrodes. Generally, electrochemical approaches can be divided into potentiometric and potentiostatic methods. The potentiostatic method involves the use of a potentiostat, which is an instrument that forces a change in voltage away from the open-circuit potential to initiate redox reactions in an electrolytic cell, and is considered more favorable for chemical analysis.35 The potentiostat is connected to an electrochemical cell, which contains three electrodes, i.e., reference, auxiliary, and working electrodes. The electrochemical cell is filled with electrolyte, which is responsible for the ion transport between the electrodes. Different concentrations of the target analyte are dissolved in the electrolyte, which is subsequently oxidized or reduced on the electrode surface. The potential at which the oxidation or reduction peak appears depends on the chemistry of the analyte, types of electrodes, and electrolytic conditions such as the type of electrolyte and its pH. The produced current depends on the concentration of the target analyte in the electrolyte solution.36
Electrochemical sensors have been demonstrated to have greater applicability compared with other detection methods, such as spectrophotometry, gas chromatography, and high-performance liquid chromatography, in different fields ranging from healthcare to the environment due to their favorable features such as portability, cost-effectiveness, rapid analysis, and lower solvent consumption.4,37,38 They have been extensively used for detecting different types of substances such as drugs, sweat ions, bioactive molecules, pesticides, dyes, mycotoxins, and heavy metals.8,39–43 Basically, electrochemical sensors convert chemical reactions of the targeted substances into electrical impulses with variations in potential, current, impedance, and conductivity.44 These variations are used as electrochemical signals to investigate the composition and/or concentration of the targeted substances.45 Different electrochemical methods such as potentiometric, amperometric, chronoamperometric, voltammetric, and impedimetric or electrochemical impedance spectroscopy (EIS) can be used for monitoring the electrochemical signal (s) of the substance of interest.
Potentiometric sensors are based on the measurement of the variation in potential at different concentrations of the target analyte under the conditions of no current flow, while amperometric and chronoamperometric sensors are based on the measurement of the current produced from the oxidation or reduction of the target analyte.46,47
Voltammetric methods are extensively used for detecting target analytes, which include different techniques such as cyclic voltammetry (CV), differential pulse voltammetry (DPV), square wave voltammetry (SWV), differential pulse stripping voltammetry (DPSV), linear sweep voltammetry (LSV), and square wave anodic stripping voltammetry (SWASV).8
Impedimetric methods rely on receptors that result in an increase or decrease in resistance in the presence of the target analyte, while amperometric methods are based on the determination of the concentration of the target analyte at a constant potential.8Fig. 1 shows the electrochemical signal of the voltametric, EIS, amperometry, and chronoamperometry methods, which are mainly used in electroanalytical applications. Among them, voltametric sensors are frequently used in the electrochemical detection of analytes given that they are simple, sensitive, and selective and possess desirable features such as fast response and lower detection limits.48
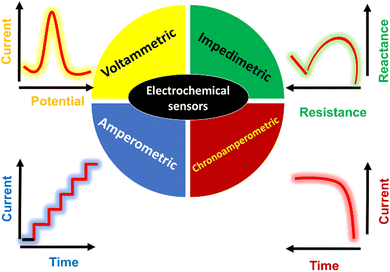 |
| Fig. 1 Schematic representation of the electrochemical methods mainly used in sensing applications. | |
Electrochemical sensors have been developed to enhance their sensitivity, reproducibility, selectivity, affordability, and stability. This development can be achieved by modifying the electrode surface with recognition and redox probes such as biofilms, enzymes, metal–organic frameworks, and nanomaterials.49–52 Electrode modification with nanomaterials has gained significant interest, given that it results in an enhancement in electrocatalytic activity, reduction in the peak-to-peak separation, improvement in the current response, and increase in the electrode surface area.38 The modification of electrodes with nanomaterials can be achieved via dipping or sticking the electrode in a nanosuspension, electrodeposition, coating, spray coating, drop casting, spin coating, or carbon pasting.8,53 Nanomaterials such as metallic nanoparticles (NPs) act as redox mediators, enabling the sensitive detection of the target analytes. For example, AgNPs were studied as redox mediators for enhancing the detection of bromocresol green dye. The SWV method was used for achieving redox mediation or redox cycling, where the oxidation of bromocresol green (forward pulse) was followed by its reduction (backward pulse). The backward current was enhanced in presence of AgNPs, leading to an increase in the net peak current, which is a combination of the forward and backward current.54Fig. 2 shows how the presence of AgNPs as a redox mediator enhances the oxidation of bromocresol green dye.
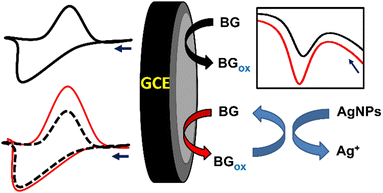 |
| Fig. 2 Schematic representation of the detection of bromocresol green dye using AgNPs as a redox mediator.54 | |
Polymer-based nanocomposites
A polymer is a macromolecule composed of repeating structural units connected by covalent chemical bonds. Polymers have become very popular due to their attractive features such as lightweight, tunable strength and elasticity, easy shaping and coloring, tunable transparency, and thermal and electric insulation.55 Therefore, they have been extensively used in many applications such as photonics, optoelectronics, energy storage devices, and medical monitoring sensors.56–59 Alternatively, they can be combined with other nanomaterials to form polymer NCs. Polymer NCs are composed of organic or inorganic nanomaterials (nanofillers) dispersed in a polymer matrix. These NCs combine the characteristics of the nanofillers and polymers, resulting in macroscopic features (strength, hardness, and toughness) in the NCs.60 Accordingly, they have been utilized in various applications such as adhesives, anticorrosive coatings, drilling fluids, electronic devices, and electric circuits.32,61–63
Metal-synthetic polymer NCs as electrode modifiers for sensing applications
The modification of the surface of electrodes with polymers results in the creation of functional materials, which can be used in sensing applications. The modification can be achieved via different approaches such as electro-grafting, self-assembly, and electrodeposition.64 Additionally, the polymer can be processed using the molecular imprinting technique before its adsorption on the electrode surface. This technique creates molecular cavities in the polymeric matrix, which are produced in the presence of template molecules, forming artificial binding sites, which leads to an enhancement in the selectivity of the sensor.65,66 Alternatively, the surface of electrodes can be modified using metal–polymer NCs via different pathways such as polymerization with subsequent metal ion reduction, metal ion reduction with subsequent polymerization, and direct adsorption (Fig. 3). Previously, metal NPs were incorporated in synthetic polymers to modify the surface of electrodes for the construction of electrochemical sensors.67,68 Herein, the focus is on carbohydrate polymers mixed with metal and metal oxide NPs as efficient alternatives to synthetic polymer-based NCs.
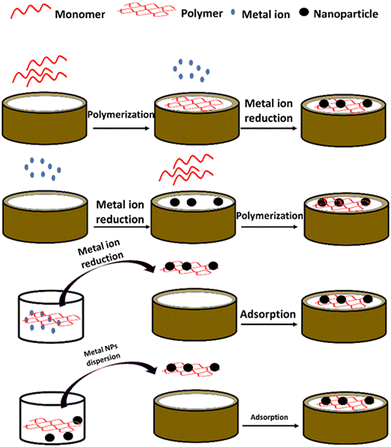 |
| Fig. 3 Schematic representation of the different pathways used for electrode modification with metal–polymer NCs. | |
Metal/metal oxide–carbohydrate polymer NCs for sensing applications
Carbohydrate polymers are biodegradable materials that can be broken down into smaller compounds by biological processes and organisms such as bacteria and fungi.69 They can be derived from various resources such as plants, seafood, and algae (Fig. 4). They are broadly utilized in many medical applications such as bone tissue engineering, tissue regeneration, drug delivery systems, and imaging.70 Carbohydrate polymers as natural polymers are more favorable than synthetic polymers given that they are economical, readily available, non-toxic, biodegradable, biocompatible, and cost-effective materials. Consequently, they have been used in industrial applications such as binders, drug release modifiers, film coatings, viscosity enhancers, stabilizers, disintegrants, emulsifiers, gelling agents, bioadhesives, sensors, and water treatment.71–74 Additionally, carbohydrate polymers and their NCs can be used as corrosion inhibitors75 and adsorbents for the removal of heavy metals76 and organic pollutants.77 Recently, they have been receiving increasing attention as electrode modifiers for the detection of analytes.
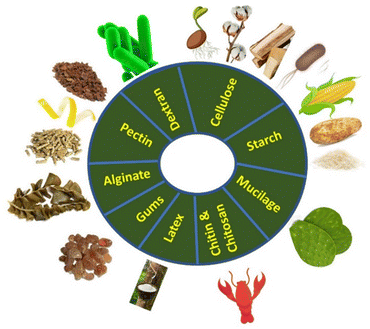 |
| Fig. 4 Schematic representation of the different pathways used for the modification of electrodes with metal–polymer NCs. | |
Fig. 5 shows the key trend of “carbohydrate polymers” and “metal NPs” in the field of “electrochemical sensors” in the period of 2010–2020. Obviously, there was an exponential increase in publications annually.
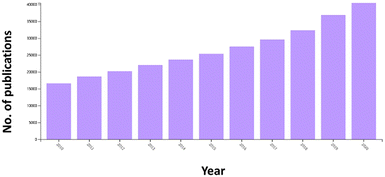 |
| Fig. 5 Visualization of research impact of “carbohydrate polymers” and “metal and metal oxide NPs” in the field of “electrochemical sensors” in the period 2000 to 2022 according to the results of Web of Science Core Collection. | |
Previously, carbohydrate polymers such as cellulose, chitin, chitosan, and pectin have been reported as electrode modifiers for the electrochemical detection of drugs and environmental pollutants.78–81 Also, to realize superior catalytic activity, high sensitivity, and low detection limits, carbohydrate polymers have been incorporated with other natural polymers,82 synthetic polymers,83,84 carbon nanotubes,81,83,85 graphene oxide,86 organoclay,87 and metal/metal oxide NPs for the fabrication of electrochemical sensors (Fig. 6).
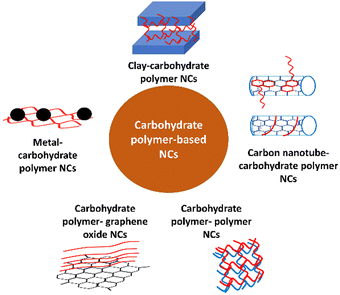 |
| Fig. 6 Carbohydrate polymer-based NCs used in electroanalytical applications. | |
For example, cellulose polymer was mixed with carbon nanotubes and copper ions for the selective detection of tryptophan enantiomers.81 The sensor exhibited stronger complexation ability for L-Trp than D-Trp, leading to a difference in the oxidation peaks between the two enantiomers (Fig. 7). In this review, the focus is on NCs formed from carbohydrate polymers, such as alginate, cellulose, chitin, chitosan, dextran, pectin, and gums and metal/metal oxide NPs.
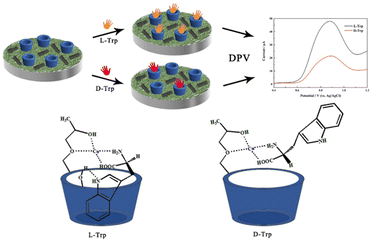 |
| Fig. 7 Schematic representation the of electrochemical chiral recognition of tryptophan isomers (reprinted from ref. 81 with permission from Elsevier). | |
Fig. 4 shows the carbohydrate polymers and their sources that have been used for the preparation of metal/metal oxide–carbohydrate polymer NCs for subsequent immobilization on the surface of electrodes for electroanalytical applications. Additionally, the advantages and sensing properties of carbohydrate polymers are explained in Table 1.
Table 1 Advantages and sensing properties of carbohydrate polymers
Carbohydrate polymer |
Properties/advantages |
Sensing properties |
Ref. |
Alginate |
It a high number of hydroxyl groups required for NP synthesis. |
It is easily electropolymerized at the electrode surfaces providing functional groups for interaction with analytes. |
87 and 88
|
Cellulose |
It has increased surface area and superior dispersion of NPs. |
It enhances the electrocatalysis. |
89
|
Chitosan |
It has good membrane-forming ability, high permeability, good adhesion, and high mechanical strength. |
Its reactive amino and hydroxyl functional groups interact with the recognition elements. |
90
|
Dextran |
It stabilizes NPs against aggregation. |
It provides steric hindrance, preventing the unspecific adsorption of interferences. |
91
|
Gelatin |
It is a good dispersing agent and excellent immobilization matrix. |
It is used in biosensors due to the presence of amino acids. |
92
|
Gum |
It has high strength and good viscosity. |
It forms highly sensitive NCs. |
93
|
Pectin |
It has hydroxyl and carboxyl groups required for the synthesis of NPs. |
It presents good electrocatalytic capacity. |
94
|
Starch |
It is a good reducing agent required for the synthesis of NPs. |
It provides more active sites to carry analyte and for oxidation/reduction. |
95
|
These nanocomposites can be prepared using various techniques such as sonochemical, microwave, mechanochemical, evaporation, and electrochemical methods.32 They combine the biodegradability, compatibility, and stability of carbohydrate polymers and conductivity of metal/metal oxide NPs, making them excellent candidates for electroanalytical applications. Next, these NCs are adsorbed on the electrode surface through coating, drop casting, dipping, electrodeposition, and carbon paste (Table 2).
Table 2 Metal and metal oxide-carbohydrate polymer NCs as electrode modifiers for the construction of electrochemical sensors
Carbohydrate Polymer |
NP/NC |
Electrode material |
Modification method |
Analyte |
Method |
Mechanism |
LOD (nM) |
Ref. |
A: amperometry, Abs: antibodies, CA: chronoamperometry, Con A: Concanavalin A, CEA: carcinoembryonic antigen, CPE: carbon paste electrode, CV: cyclic voltammetry, DPSV: differential pulse stripping voltammetry, GCE: glassy carbon electrode, GO: graphene oxide, LSV: linear sweep voltammetry, LOD: limit of detection, MWCNT: multi-walled carbon nanotube, NC: nanocomposite, NP: nanoparticle, NS: nanosheet, PANI: polyaniline, PPY: polypyrrole, rGO: reduced graphene oxide, SPCE: screen-printed carbon electrode, SWASV: square wave anodic stripping voltammetry, SWCNT: single-walled carbon nanotube, SWV: square wave voltammetry, SWASV: square wave anodic stripping voltammetry, and TEMPO: 2,2,6,6 tetramethylpiperidine-1-oxyl. |
Alginate |
CuO NP |
Aodium alginate-g-polyallylamine/rGO/CuO GCE |
Drop casting |
Cd2+ |
CV & CA |
|
1.27 |
105
|
Cellulose |
CuO NSs |
CuO NSs/cellulose acetate butyrate/GCE |
Coating |
Hydrazine |
LSV |
Analyte oxidation |
150 |
117
|
|
Fe3O4 NPs |
Cellulose nanofiber/Fe3O4 NC/GCE |
Coating |
Hydrazine |
DPV |
Analyte oxidation |
0.5 |
89
|
|
|
Cellulose nanofiber/Fe3O4 NC/CPE |
Carbon paste |
4-Chlorophenol |
DPV |
Analyte oxidation |
0.5 |
118
|
|
|
Methyl cellulose/graphene oxide/Fe3O4 NC/GCE |
Drop casting |
Uric acid |
DPV |
Analyte oxidation |
170 |
119
|
|
Au NPs |
Au NPs/cellulose nanocrystals/SPCE |
Drop casting |
4,4′-Methylene diphenyl diamine |
DPV |
Analyte oxidation |
57 |
114
|
|
Ag NPs |
AgNPs/cellulose nanofibers/graphite electrode |
Drop casting |
Ascorbic acid |
DPV |
Analyte reduction |
29 |
115
|
|
|
|
|
Dopamine |
|
|
34 |
|
|
|
|
|
Paracetamol |
|
|
19 |
|
|
|
Graphite/Ag NPs/TEMPO-oxidized cellulose nanofibers/GCE |
Coating |
Dopamine |
A |
Analyte oxidation |
0. 5 |
113
|
|
|
Ag/cellulose/GCE |
Drop casting |
DNA |
DPSV |
Modifier signal inhibition |
0.023 |
116
|
|
|
Cellulose–Ag NPs/CPE |
Carbon paste |
Ornidazole |
SWV |
Analyte reduction |
758 |
112
|
|
ZnO NPs |
PANI–carboxymethyl cellulose/ZnO NC/GCE |
|
Hydrogen peroxide |
DPV |
Analyte reduction |
450 |
120
|
Chitosan |
Au NPs |
Au NPs/carbon nano-onions/SWCNT/chitosan/GCE |
|
CEA |
SWV |
Modifier signal inhibition |
100 fg mL−1 |
90
|
|
Nano-porous Au film electrode |
Polypyrrole/chitosan/nano-porous Au film electrode |
|
Metronidazole |
DPV |
Analyte oxidation |
0.9 |
84
|
Dextran |
Au NPs |
Con A/Au NPs/phenoxy dextran/rGO/SPCE |
|
Glucose |
DPSV |
Modifier signal inhibition |
20 000 |
136
|
|
|
Dextran–Au NPs/SPCE |
Electro-deposition |
Cr(VI) & Cr(III) |
LSV |
Analyte reduction |
10 |
137
|
|
Fe3O4 NPs |
Abs–polyaldehyde–dextran–Fe3O4 NPs/SPCE |
|
Peroxidase |
A |
|
0.44 ng mL−1 |
91
|
Gums (almond gum) |
Fe2O3 NPs |
Almond gum/Mg–Fe2O3 NPs/graphite electrode |
|
Paracetamol |
CV |
|
|
142
|
|
Bi2O3/MgO/Fe2O3 NC |
Almond gum/Bi2O3/MgO/Fe2O3 NC/graphite electrode |
|
Paracetamol |
CV |
|
|
143
|
Gums (arabic gum) |
Ag NPs |
Arabic gum/Ag NPs/CPE |
Carbon paste |
Morin |
|
Analyte oxidation |
0.216 |
150
|
|
|
Arabic gum/Ag NPs/GCE |
Dipping |
Methylene blue |
SWASV |
Analyte oxidation |
64, 440 μg L−1 |
151
|
|
|
|
|
|
|
Modifier signal amplification |
0.96 μg L−1 |
|
Gums (guar gum) |
Ag–TiO2 NPs |
Guar gumAg–TiO2 NPs/GCE |
Drop casting |
Hydroquinone |
SWV |
Analyte oxidation |
195.2 |
156
|
|
|
|
|
Catechol |
|
|
161 |
|
|
|
|
|
Resorcinol |
|
|
77.58 |
|
|
|
|
|
Nitrite |
|
|
101 |
|
Gums (locust bean gum) |
Ag NPs |
Ag NPs/copper-micro mesh grid electrode |
Drop casting |
Glucose |
DPV |
Analyte oxidation |
1 |
93
|
Latex |
Ag NPs |
AgNPs–latex/GCE |
Dipping |
Eriochrome black T |
SWV |
Analyte oxidation |
2.92 μg L−1 |
166
|
|
|
|
|
|
|
Modifier signal inhibition |
7.01 μg L−1 |
|
Mucilage |
Ag NPs |
AgNPs–mucilage/GCE |
Dipping |
Chlorpyrifos |
SWV |
Modifier signal inhibition |
17.2 ng L−1 |
173
|
|
Ag NPs &Au NPs |
AgNPs–AuNPs–mucilage/GCE |
Coating |
Prostatic specific antigen |
EIS |
Modifier signal amplification |
0.087 pg mL−1 |
172
|
Pectin |
CuO NPs |
Graphene/pectin/CuO NPs/GCE |
Drop casting |
Glucose |
A |
Analyte oxidation |
210 |
180
|
|
|
|
|
Hydrogen peroxide |
A |
Analyte reduction |
350 |
|
|
CuO NPs |
CuO NPs/MWCNT/pectin/GCE |
Electro-deposition |
Glycerol |
A |
Analyte oxidation |
5.8 × 10−6 g dm−3 |
178
|
|
Au NPs |
Aptamer/pectin–Au NPs/GO/GCE |
Electro-deposition |
Aflatoxin M1 |
DPV |
Inhibition of modifier signal |
0.2 ng L−1 |
94
|
Pectin–chitosan |
CuO NPs |
Lipase/magnetic NPs–chitosan/CuO NP/MWCNT/pectin/GCE |
Drop casting |
Glycerol |
A |
Analyte oxidation |
|
179
|
Starch |
Cu2O |
Cu2O–starch/CPE |
Carbon paste |
Glucose |
CV |
Analyte oxidation |
70 000 |
95
|
Co |
CoNPs/starch/CPE |
Carbon paste |
Paracetamol |
DPV |
Analyte oxidation |
0.99 |
184
|
The previously studied analytes including biological molecules and water pollutants are shown in Fig. 8. The biological analytes include drugs, biomarkers, carbohydrates, enzymes, hormones, aflatoxins, and natural antioxidants, while the water pollutants include inorganic compounds, metal ions, pesticides, dyes, and phenolics. The composition of metal/metal oxide carbohydrate polymer NC-based modified electrodes, electrode modification methods, electrochemical techniques, sensing mechanisms, and their detection limits are presented in Table 2. The sensing mechanisms include direct oxidation/reduction of the analyte or inhibition/amplification of the electrochemical response of the modifier.
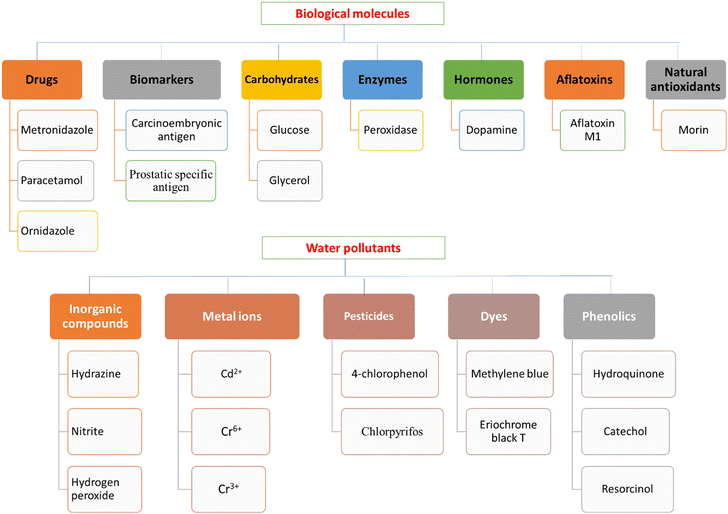 |
| Fig. 8 Classification of the biological molecules and water pollutants electrochemically detected using metal–carbohydrate polymer NCs. | |
Alginate
Sodium alginate, a water-soluble anionic polyelectrolyte, is formed from two glycan monomers, i.e., α-L-guluronic acid and β-D-mannuronic acid, linked in the (1 → 4) positions of the linear block copolymer structure.96 Alginate-based polymers have attractive features such as biodegradability, high mechanical strength, and high adsorption capacity, making them desirable materials in many biomedical applications such as drug delivery, diagnosis, tissue engineering, microencapsulation, and wound healing.97,98 Additionally, they have been used in industrial applications such as concrete.99 Polyelectrolytes including alginate polymer have attracted significant attention due to their applications in various electrochemical devices such as batteries, supercapacitors, solar cells, and sensors.100–104 Recently, a NC formed from sodium alginate polymer, CuO NPs, polyallylamine, and reduced graphene oxide was immobilized on a glassy carbon electrode for the electrochemical assessment of Cd2+ in river water using CV and chronoamperometry.105
Cellulose
Cellulose, a polymer obtained from animals and plants, is regarded as the most abundant material in nature.106,107 It has been used for the preparation of materials possessing antimicrobial properties,108 extracting chlorobenzenes from water,109 producing components used in the automotive, packaging, and building materials sectors,110 and preparing materials for lithium-ion batteries.111 Recently, it has been extensively utilized for the preparation of metal– and metal oxide–cellulose NCs as electrode modifiers for electroanalytical applications. Fig. 9 exhibits the synthesis of an Ag–cellulose NC and its subsequent adsorption on the electrode surface.112 Metal–cellulose NCs such as Au– and Ag–cellulose NCs have been used for detecting organic molecules. An Au–cellulose NC/screen-printed electrode was studied as an electrochemical sensor for assessing 4,4′-methylene diphenyl diamine based on its oxidation using DPV via two-electron transfer, while an Ag–cellulose/carbon paste electrode and Ag–cellulose/glassy carbon electrode were utilized for the detection of ornidazole and dopamine via two-electron transfer, respectively.112–114
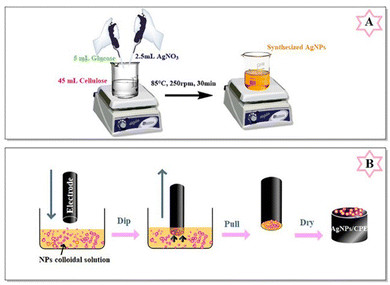 |
| Fig. 9 Schematic representation of Ag–cellulose NC synthesis and subsequent adsorption on the electrode surface (reprinted from ref. 112 with permission from Elsevier). | |
Additionally, an AgNP/cellulose nanofiber/graphite electrode was used for the sensitive detection of ascorbic acid, dopamine, and paracetamol based on their reduction.115
Ag–cellulose NCs were also used for the detection of DNA hybridization based on the inhibition of the AgNP signal.116 Alternatively, metal oxide–cellulose NCs such as CuO–, Fe3O4–, and ZnO–cellulose NCs were used for detecting organic and inorganic analytes. For example, hydrazine was electrochemically detected via its LSV and DPV oxidation on CuO nanosheet–cellulose acetate butyrate- and Fe3O4–cellulose NC-modified glassy carbon electrodes, respectively.89,117 Notably, the Fe3O4–cellulose NCs showed a lower detection limit than CuO nanosheet–cellulose acetate butyrate. The oxidation mechanism of hydrazine using CuO NSs/cellulose acetate butyrate was based on the transfer of four electrons, while the Fe3O4–cellulose NCs showed an electron transfer coefficient of 0.44 using the Tafel plot slope, confirming the irreversible oxidation of hydrazine. Also, an Fe3O4–cellulose NC was used as a carbon paste electrode modifier for the detection of 4-chlorophenol via its oxidation using DPV.118 The value of the electron transfer coefficient, which was calculated using the Tafel plot slope, was 0.22, confirming the irreversible oxidation of 4-chlorophenol on the surface of the Fe3O4–cellulose NC-modified electrode. An Fe3O4–cellulose NC was also used for the detection of uric acid in urine.119 Additionally, a ZnO–carboxymethyl cellulose NC was applied for the reduction of hydrogen peroxide for its detection via the DPV technique.120
Chitin and chitosan
Chitin, a linear homopolymer of N-acetyl-D-glucosamine, is the second most abundant polymeric polysaccharide after cellulose.121 It is a white, hard, and non-elastic material, which can be obtained from shrimp and crab shells, while chitosan, a copolymer of α-(1 → 4) glucosamine, is derived from chitin by deacetylation.122,123 The biocompatibility, biodegradability, and non-toxicity of chitin and chitosan polymers make them efficient for application in drug delivery.124 Recently, water-soluble based chitosan composites have been used in sensing and biomedical engineering.125 Additionally, chitosan polymers have been used for the preparation of hydrogels for application in tissue engineering and drug delivery.126 Moreover, a chitin nanofiber hydrogel was studied as a conductive material for the construction of a wearable sensor.127
Alternatively, chitin and chitosan have been extensively used in the construction of electrochemical sensors.128 For example, potentiometric sensors based on a chitin-grafted polyaniline electrode and chitosan nanocomposite were used for the electrochemical sensing of copper ions129 and monohydrogen phosphate ions,130 respectively. Metal–chitosan NCs were prepared for used in the construction of electrochemical sensors. For example, Au NPs, carbon nano-onions, and single-walled carbon nanotubes were incorporated in chitosan to form an electroactive NC for the determination of a carcinoembryonic antigen.90 The detection strategy was based on the inhibition of the modifier signal in presence of the carcinoembryonic antigen. Moreover, a chitosan/polypyrrole-modified nano-porous film Au electrode was applied for the assessment of metronidazole drug due to its high electrical conductivity and selectivity. The assessment of the drug was achieved using DPV through its oxidation.84
Dextran
Dextran, a neutral hydrophilic polymer produced from sucrose by Leuconostoc mesenteroides, has attracted significant attention in medical applications because of its nontoxicity, biodegradability, and biocompatibility.131 Furthermore, is highly efficient for the preparation of drug delivery systems132,133 and energy storage devices.134,135 Its metal and metal oxide NCs can be used as efficient electrode modifiers for targeting analytes. For example, Au–dextran NC as a screen-printed carbon electrode modifier was used for the biological detection of glucose using DPSV. The mechanism of detection was based on the inhibition of the modifier signal in the presence of glucose.136 Also, it was used as a non-enzymatic sensor for the detection of Cr(VI) and Cr(III) using LSV based on their electrochemical reduction. Furthermore, dextran was used as a sorbent for the extraction, separation, and the analysis of chromium ions.137
Recently, a dextran–Fe3O4 NC immobilized on a screen-printed electrode was used as an immunosensor for the assessment of peroxidase using chronoamperometry, where hydroquinone was used as the redox mediator.91 The mechanism for the chronoamperometric determination of peroxidase is shown in Fig. 10.
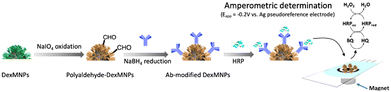 |
| Fig. 10 Schematic representation of the amperometric determination of peroxidase using polyaldehyde–dextran metal NPs (reprinted from ref. 91 with permission from Elsevier). | |
Gums
Gums are polysaccharides that contain sugar units such as glucose, galactose, arabinose, xylose, and mannose.66 They include almond, Arabic, guar, and locust bean gums. Gums can form three-dimensional interconnected molecular networks called gels. The gel strength relies on the structure and concentration of the gum, as well as parameters such as ionic strength, pH, and temperature. These gums have been used for the preparation of NCs to be used in the construction of electrochemical sensors.
Almond gum
Almond gum, an exudate produced by the trunk, branches, and fruits of the Prunus amygdalus tree, is composed of polysaccharides such as arabinose, galactose, uronic acid, and protein.138 It can be used as preservative, emulsifier, stabilizer, and food strengthener in the food industry.139,140 Additionally, it has been applied in the pharmaceutical industry for improving the binding and emulsification features of drug formulations.141 Recently, an almond gum/Mg–Fe2O3 NP/graphite electrode and almond gum/Bi2O3/MgO/Fe2O3 NC/graphite electrode were used for detecting paracetamol using the CV technique.142,143
Arabic gum
Arabic gum, a water-soluble polymer originating from the Acacia Senegal tree, is broadly used for food and drug production. It is composed of highly branched polysaccharides containing charged groups such as amine and carboxyl, which make it an efficient stabilizing agent for NPs.144 Also, has been is applied in the food industry,145 oil industry,146 membrane technology,147 and corrosion inhibition.148 Additionally, it has been used for the preparation of metal–Arabic gum NCs for pharmaceutical and medical applications.149 Recently, Ag–Arabic gum NCs have been used as electrode modifiers for the electrochemical detection of analytes. For example, Ag–Arabic gum NC incorporated in a modified carbon paste electrode was utilized for detecting morin based on its irreversible oxidation after its hydrogen bonding interaction with the modified electrode (Fig. 11).150 Additionally, an Ag–Arabic gum NC/glassy carbon electrode was used for detecting methylene blue dye based on its oxidation and modifier signal amplification. The oxidation was achieved via two-electron and one-proton transfer (Fig. 12), while the signal amplification mechanism depends on the role of methylene blue analyte as the redox mediator, which catalyzes the oxidation of AgNPs.151
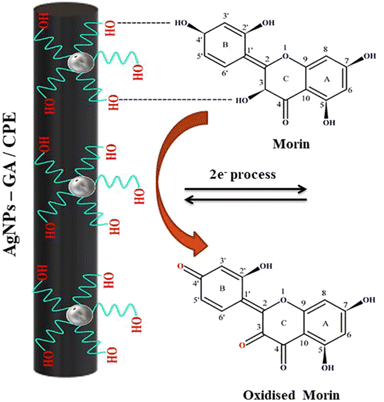 |
| Fig. 11 Schematic representation of the mechanism of the electrochemical oxidation of morin at AgNP–gum Arabic (GA)/CPE (reprinted from ref. 150 with permission from Elsevier). | |
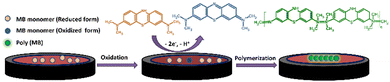 |
| Fig. 12 Schematic representation of the oxidation and subsequent polymerization of methylene blue at gum Arabic-AgNPs/GCE (reprinted from ref. 151 with permission from Elsevier). | |
Guar gum
Guar gum, a water-soluble polysaccharide, is obtained from the seeds of Cyamopsis tetragonolobus.152 It has been applied in drug delivery153 and solar cell154 systems. Additionally, it was mixed with Au nanoclusters for the preparation of a fluorescence sensor to screen anti-diabetic agents in plant extracts.155
Additionally, it has been used for the preparation of electroactive NCs. Recently, Ag–TiO2 NPs mixed with guar gum were used as a glassy carbon electrode modifier for the electrochemical detection of hydroquinone, catechol, resorcinol, and nitrite using SWV based on their oxidation via one-electron transfer (Fig. 13).156 Compared with the ZnO NP–carboxymethyl cellulose composite, the Ag–TiO2 NP–guar gum composite can be used for the detection of hydroquinone with a lower detection limit (Table 2).
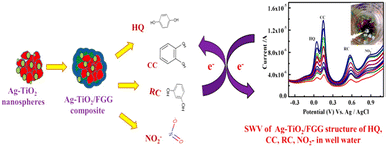 |
| Fig. 13 Schematic representation of Ag–TiO2/functionalized guar gum NC for the detection of hydroquinone, catechol, resorcinol, and nitrite (reprinted from ref. 156 with permission from Elsevier). | |
Locust bean gum
Locust bean gum, which can be extracted from the carob fruit, is composed of a mixture of components such as polysaccharides, protein, fat, and fibers.157 It was used in the formation of a network hydrogel for the preparation of 3D printing products.158 Additionally, it has been used for drug delivery159 and in the food industry.160 Alternatively, it was mixed with Ag NPs for the preparation of an effective electrode modifier for sensing glucose, allowing the maximum accommodation of glucose enzyme before the sensing process.93 The Ag NP–locust bean gum composite showed the highest sensitivity and lowest detection limit towards glucose compared to other carbohydrate polymer-based nanocomposites such as Au NP–dextran136 and Cu2O NP–starch NCs.95
Latex (natural rubber)
Natural latex is composed of micelles of rubber, lipids, amino acids, nucleic acids, and proteins.161 It has been mixed with inorganic NPs for the construction of NCs, which can be used in applications such as catalysis, chromatography, ceramics, electronics, biotechnology, medicine, and drug delivery.162–165 Additionally, latex has been incorporated with Ag NPs to form wound dressings.161 Alternatively, an Ag–latex NC was studied as an electrochemical probe for detecting Eriochrome black T using the SWV technique. The sensing process was based on the oxidation of Eriochrome black T and the inhibition of the oxidation peak current of Ag NPs. The oxidation of Eriochrome black T was achieved through one-electron transfer (Fig. 14), while the inhibition of the AgNP signal was attributed to the displacement of the AgNPs by Eriochrome black T at the electrode surface (Fig. 15).166
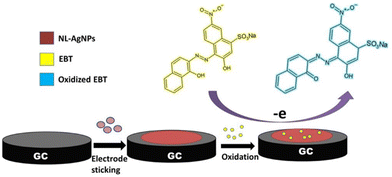 |
| Fig. 14 Schematic representation of Eriochrome black T (EBT) oxidation at the natural latex–AgNPs/GCE (reprinted from ref. 166 with permission from Elsevier). | |
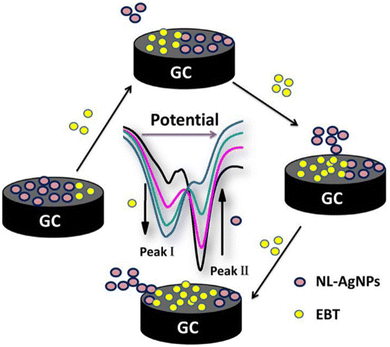 |
| Fig. 15 Schematic representation of the electrochemical displacement of AgNPs by Eriochrome black T (EBT) (reprinted from ref. 166 with permission from Elsevier). | |
Mucilage
Mucilage, a complex water-soluble polysaccharides, is composed of monosaccharides and uronic acids.167 It has been utilized in numerous applications such as the food industry,167 delivery of bioactive compounds,168 emulsification,169 catalysis,170 and water treatment.171 Recently, it has been used for the preparation of metal-mucilage NCs as electroactive materials. For example, an Ag/Au-mucilage NC was used as an immunosensor for the detection of the prostatic specific antigen using EIS. The presence of Au NPs and Ag NPs enhanced the conductivity of the mucilage, while the presence of the analyte resulted in a decrease in conductivity and an increase in impedance.172 Additionally, an Ag-mucilage NC was used as a glassy carbon electrode modifier for the detection of chlorpyrifos based on the inhibition of the peak current of AgNP oxidation.173
Pectin
Pectin, a heteropolysaccharide, is composed of galacturonic acid, rhamnose, arabinose, and xylose.174 It has been used in many applications such as, drug delivery,175 tissue engineering,176 and the food industry.177 It has also been used for the preparation of metal–pectin NCs for electroanalytical applications. For example, CuO–pectin NC was incorporated with multiwalled carbon nanotubes on the surface of a glassy carbon electrode for the detection of glycerol based on its oxidation using amperometry.178 Similarly, CuO–pectin NC was immobilized with chitosan, lipase, multiwalled carbon nanotubes, and magnetic NPs on the surface of a glassy carbon electrode for sensing glycerol based on its oxidation using amperometry (Fig. 16).179 Additionally, a graphene/pectin/CuO NP/GCE was used for the detection of glucose and hydrogen peroxide in human serum based on their amperometric oxidation and reduction respectively.180 This sensor exhibited many advantages such as simplicity and high sensitivity, stability and reproducibility. Recently, an Au–pectin NC was studied as an aptameric sensor for the detection of aflatoxin M1 using DPV based on the inhibition of the modifier signal.94
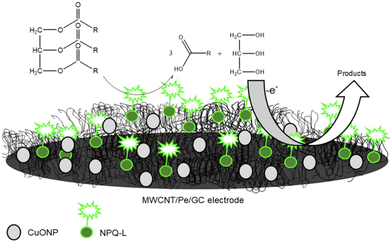 |
| Fig. 16 Schematic representation of glycerol determination using copper oxide/multiwalled carbon nanotube/pectin/GCE (reprinted from ref. 179 with permission from Elsevier). | |
Starch
Starch is a high-molecular-weight carbohydrate that is produced by polymerizing glucose molecules. It has been utilized for developing conducting biofilms, fuel-cell coatings, supercapacitor electrodes, solar cells, and colorimetric and electrochemical sensors.181–183 Cu2O–starch NC as a modified carbon paste electrode has been used as an electrochemical sensor for the assessment of glucose using the CV technique based on its oxidation.95 Additionally, a CoNP/starch/CPE was used for the detection of paracetamol based on its oxidation via two-electron and two-proton transfer.184
Conclusion
In summary, electrochemical sensors are considered promising tools for the detection of various analytes. Additionally, the efficiency of electrochemical sensors can be improved by using metal– and metal oxide–carbohydrate polymer NCs as electrode modifiers. Carbohydrate polymers act as efficient reducing and stabilizing agents for the synthesis of metal and metal oxide NPs. Additionally, they can be used for the homogenous distribution of NPs. Herein, metal– and metal oxide–carbohydrate polymer NCs represent a valuable tool for the electrochemical detection of biological molecules and water pollutants. Initially, these NCs can be incorporated in electrochemical sensors via polymerization with subsequent metal ion reduction, metal ion reduction with subsequent polymerization, or through direct adsorption. Next, the electrodes modified with metal– or metal oxide–carbohydrate polymer NCs can be used as electrochemical sensors for the detection of analytes using different electrochemical methods such as voltammetry, EIS, amperometry, and chronoamperometry. The sensing of the analytes depends on their oxidation/reduction on the surface of the modified electrodes or the amplification/inhibition of the electrochemical response of the modifier in the presence of the analyte of interest. The role of carbohydrate polymers in these electrochemical sensors is crucial given that they are responsible for the synthesis, stabilization, and distribution of NPs as well as for their interaction with the target analytes, facilitating the detection process. Overall, metal/metal oxide NP–carbohydrate polymer nanocomposites represent a promising tool for the electrochemical detection of different analytes. They may be useful for detecting numerous biological and environmental molecules. However, the selectivity of carbohydrate polymers is still poor compared to molecularly imprinted polymers, enzymes, and aptamers. Thus, the selectivity of carbohydrate polymers-based electrochemical sensors needs to be enhanced. In this case, it is recommended to enhance the selectivity of these electrochemical systems as the primary step in their commercialization.
Conflicts of interest
There are no conflicts to declare.
References
- M. A. Ahmad, M. Adeel, N. Shakoor, R. Javed, M. Ishfaq, Y. Peng, M. Zain, I. Azeem, I. Ali and M. Usman, Sci. Total Environ., 2023, 164861 CrossRef CAS PubMed.
- P. Sowmiya, T. S. Dhas, D. Inbakandan, N. Anandakumar, S. Nalini, K. U. Suganya, R. Remya, V. Karthick and C. V. Kumar, Micron, 2023, 103486 CrossRef CAS PubMed.
- X. Teng, Q. Ling, T. Liu, L. Li and C. Lu, TrAC, Trends Anal. Chem., 2023, 117020 CrossRef CAS.
- H. Du, Y. Xie and J. Wang, TrAC, Trends Anal. Chem., 2021, 135, 116178 CrossRef CAS.
- J. Gañán, G. Martínez-García, S. Morante-Zarcero, D. Pérez-Quintanilla and I. Sierra, Microchem. J., 2022, 108136 Search PubMed.
- M. Zahran and A. H. Marei, Int. J. Biol. Macromol., 2019, 136, 586–596 CrossRef CAS PubMed.
- P. Kalambate, P. Thirabowonkitphithan, P. Kaewarsa, K. Permpoka, A. Radwan, R. Shakoor, R. Kalambate, H. Khosropour, Y. Huang and W. Laiwattanapaisal, Mater. Today Chem., 2022, 26, 101235 CrossRef CAS.
- M. M. Zahran, Z. Khalifa, M. Zahran and M. A. Azzem, Mater. Adv., 2021, 2, 7350–7365 RSC.
- F. S. Belaïdi, L. Farouil, L. Salvagnac, P. Temple-Boyer, I. Séguy, J.-L. Heully, F. Alary, E. Bedel-Pereira and J. Launay, Biosens. Bioelectron., 2019, 132, 90–96 CrossRef PubMed.
- M. Díaz-González, M. Gutiérrez-Capitán, P. Niu, A. Baldi, C. Jiménez-Jorquera and C. Fernández-Sánchez, TrAC, Trends Anal. Chem., 2016, 77, 186–202 CrossRef.
- S. S. R. Shanlee, R. Sundaresan, S.-M. Chen, R. Balaji, T. Jeyapragasam, J.-Y. Peng and A. I. Jothi, Surf. Interfaces, 2023, 103020 CrossRef.
- S. Y. Ejeta and T. Imae, Anal. Chim. Acta, 2021, 1152, 338272 CrossRef CAS PubMed.
- F. Yuan, Y. Xia, Q. Lu, Q. Xu, Y. Shu and X. Hu, Talanta, 2022, 244, 123419 CrossRef CAS PubMed.
- M. S. Kuyukina, M. V. Makarova, O. N. Pistsova, G. G. Glebov, M. A. Osipenko and I. B. Ivshina, Heliyon, 2022, 8, e11632 CrossRef CAS PubMed.
- D. Ayodhya, Results Chem., 2023, 100874 CrossRef CAS.
- M. Jouyandeh, S. M. Sajadi, F. Seidi, S. Habibzadeh, M. T. Munir, O. Abida, S. Ahmadi, D. Kowalkowska-Zedler, N. Rabiee and M. Rabiee, OpenNano, 2022, 100104 CrossRef.
- S. K. R. Namasivayam, S. Kumar, K. Samrat and R. A. Bharani, Environ. Res., 2023, 231, 116150 CrossRef PubMed.
- N. Sa, P. Tejaswani, S. P. Pradhan, K. A. Alkhayer, A. Behera and P. K. Sahu, J. Drug Delivery Sci. Technol., 2023, 84, 104521 CrossRef CAS.
- A. R. Bagheri, N. Aramesh, M. S. Hasnain, A. K. Nayak and R. S. Varma, Chem. Phys. Impact, 2023, 100255 CrossRef.
- R. V. Bordiwala, Results Chem., 2023, 100832 CrossRef CAS.
- P. Papolu and A. Bhogi, Mater. Today: Proc., 2023, 92, 924–927 CAS.
- R. H. Waghchaure and V. A. Adole, J. Indian Chem. Soc., 2023, 100987 CrossRef CAS.
- M. Vijayakumar, K. Priya, S. Ilavenil, B. Janani, V. Vedarethinam, T. Ramesh, M. V. Arasu, N. A. Al-Dhabi, Y.-O. Kim and H.-J. Kim, Int. J. Biol. Macromol., 2020, 165, 1402–1409 CrossRef CAS PubMed.
- P. Barciela, M. Carpena, N.-Y. Li, C. Liu, S. Jafari, J. Simal-Gandara and M. Prieto, Adv. Colloid Interface Sci., 2022, 102829 Search PubMed.
- V. Pareek, A. Bhargava and J. Panwar, Mater. Sci. Eng., B, 2020, 262, 114771 CrossRef CAS.
- V. V. Padil, J. Y. Cheong, K. AkshayKumar, P. Makvandi, E. N. Zare, R. Torres-Mendieta, S. Wacławek, M. Černík, I.-D. Kim and R. S. Varma, Carbohydr. Polym., 2020, 247, 116705 CrossRef CAS PubMed.
- C. Verma and M. Quraishi, Chem. Eng. J. Adv., 2022, 100428 Search PubMed.
- F. D. Moghaddam, G. Heidari, E. N. Zare, E. Djatoubai, A. C. Paiva-Santos, F. R. Bertani and A. Wu, Carbohydr. Polym., 2022, 120510 Search PubMed.
- R. Ansari, S. M. Sadati, N. Mozafari, H. Ashrafi and A. Azadi, Eur. Polym. J., 2020, 128, 109607 CrossRef CAS.
- X. Tang, J. Liu, R. Yan and Q. Peng, Int. J. Biol. Macromol., 2023, 124902 CrossRef CAS PubMed.
- Y. Zena, S. Periyasamy, M. Tesfaye, Z. Tumsa, M. Jayakumar, B. A. Mohamed, P. Asaithambi and T. M. Aminabhavi, Int. J. Biol. Macromol., 2023, 124803 CrossRef CAS PubMed.
- J. Aslam, S. Zehra, M. Mobin, M. Quraishi, C. Verma and R. Aslam, Carbohydr. Polym., 2023, 120936 CrossRef CAS PubMed.
- D.-N. Phan, M. Q. Khan, N.-T. Nguyen, T.-T. Phan, A. Ullah, M. Khatri, N. N. Kien and I.-S. Kim, Carbohydr. Polym., 2021, 252, 117175 CrossRef CAS PubMed.
- B. Dakshayini, K. R. Reddy, A. Mishra, N. P. Shetti, S. J. Malode, S. Basu, S. Naveen and A. V. Raghu, Microchem. J., 2019, 147, 7–24 CrossRef CAS.
- S. Ren, J. Zeng, Z. Zheng and H. Shi, Sens. Actuators, A, 2021, 329, 112821 CrossRef CAS.
- M. Zahran, Heliyon, 2023, 9, e19943 CrossRef CAS PubMed.
- S. Fu, Y. Zhu, Y. Zhang, M. Zhang, Y. Zhang, L. Qiao, N. Yin, K. Song, M. Liu and D. Wang, Microchem. J., 2021, 171, 106776 CrossRef CAS.
- I. Suresh, S. Selvaraj, N. Nesakumar, J. B. B. Rayappan and A. J. Kulandaiswamy, Trends, Environ. Anal. Chem., 2021, 31, e00137 CrossRef CAS.
- P. Joshi, P. Riley, K. Y. Goud, R. K. Mishra and R. Narayan, Curr. Opin. Electrochem., 2022, 32, 100920 CrossRef CAS.
- L. Mo, X. Ma, L. Fan, J. H. Xin and H. Yu, Chem. Eng. J., 2023, 454, 140473 CrossRef CAS.
- Q. Tian, S. Chen, J. Yu, M. Zhang, N. Gao, X. Yang, C. Wang, X. Duan and L. Zang, J. Mater. Chem. C, 2022, 10, 10171–10195 RSC.
- Z. Song, Y. Zhou, X. Han, J. Qin and X. Tang, J. Mater. Chem. B, 2021, 9, 1175–1188 RSC.
- M. Zahran, R. M. El-Shabasy, A. Elrashedy, W. Mousa, M. Nayel, A. Salama, A. Zaghawa and A. Elsify, RSC Adv., 2023, 13, 31795–31810 RSC.
- S. R. Benjamin and E. J. M. R. Junior, Curr. Opin. Environ. Sci. Health, 2022, 100381 CrossRef.
- L. Xing, W. Zhang, L. Fu, J. M. Lorenzo and Y. Hao, Food Chem., 2022, 385, 132555 CrossRef CAS PubMed.
- E. Zdrachek and E. Bakker, Anal. Chem., 2018, 91, 2–26 CrossRef PubMed.
- K. Ghoshal, Adv. Sens. Technol., 2023, 261–295 Search PubMed.
- R. Porada, K. Jedlińska, J. Lipińska and B. Baś, J. Electrochem. Soc., 2020, 167, 037536 CrossRef CAS.
- M. Çorman, G. Ozcelikay, A. Cetinkaya, S. Kaya, C. Armutcu, E. Özgür, L. Uzun and S. Ozkan, TrAC, Trends Anal. Chem., 2022, 150, 116573 CrossRef.
- L. Zhang, M. Yin, X. Wei, J. Sun and D. Xu, Anal. Biochem., 2022, 656, 114882 CrossRef CAS PubMed.
- S. K. Krishnan, E. Singh, P. Singh, M. Meyyappan and H. S. Nalwa, RSC Adv., 2019, 9, 8778–8881 RSC.
- J. M. Gonçalves, P. R. Martins, D. P. Rocha, T. A. Matias, M. S. Juliao, R. A. Munoz and L. Angnes, J. Mater. Chem. C, 2021, 9, 8718–8745 RSC.
- A. Abbas and H. M. Amin, Microchem. J., 2022, 175, 107166 CrossRef CAS.
- M. Zahran, A. M. Beltagi, M. Rabie, R. Maher, A. A. Hathoot and M. A. Azzem, R. Soc. Open Science, 2023, 10, 221621 CrossRef CAS PubMed.
- C. Mukherjee, D. Varghese, J. Krishna, T. Boominathan, R. Rakeshkumar, S. Dineshkumar, C. B. Rao and A. Sivaramakrishna, Eur. Polym. J., 2023, 112068 CrossRef CAS.
- I. Ayesta, M. Azkune, M. A. Illarramendi, E. Arrospide, J. Zubia and G. Durana, Optical Fiber Technology, 2023, 75, 103209 CrossRef CAS.
- S. Chen, Y. Chen, X. Mu, P. Wang, L. Miao, S. Tanemura and H. Cai, Sustainable Mater. Technol., 2023, e00635 CrossRef CAS.
- H. Li, Y. Wang, T. Gao, L. Cao, Y. Liu, Z. An, Z. Lin, S. Zhang, Z. Zhang and C. Zhang, Opt. Commun., 2023, 539, 129510 CrossRef CAS.
- S. Yang, S. Zhang, F. Hu, J. Han and F. Li, Coord. Chem. Rev., 2023, 485, 215116 CrossRef CAS.
- O. S. Obisesan, T. O. Ajiboye, S. D. Mhlanga and H. T. Mufhandu, Colloids Surf., B, 2023, 227, 113342 CrossRef CAS PubMed.
- O. K. Abubakre, R. O. Medupin, I. B. Akintunde, O. T. Jimoh, A. S. Abdulkareem, R. A. Muriana, J. A. James, K. O. Ukoba, T.-C. Jen and K. O. Yoro, J. Sci.: Adv. Mater. Devices, 2023, 100557 CAS.
- J. O. Oseh, N. M. Mohd, A. O. Gbadamosi, A. Agi, S. O. Blkoor, I. Ismail, K. C. Igwilo and A. I. Igbafe, Geoenergy Sci. Eng., 2023, 211416 CrossRef CAS.
- S. Peng, X. Du, Z. Liang, M. Ma, Y. Guo and L. Xiong, J. Energy Storage, 2023, 60, 106636 CrossRef.
- M. Fantin and A. A. Isse, Curr. Opin. Electrochem., 2023, 101313 CrossRef CAS.
- S. Ranjbari, A. Mohammadinejad, T. P. Johnston, P. Kesharwani, R. K. Oskuee, M. Rezayi and A. Sahebkar, Eur. Polym. J., 2023, 111916 CrossRef CAS.
- A. George, P. A. Shah and P. S. Shrivastav, Eur. Polym. J., 2019, 112, 722–735 CrossRef CAS.
- K. Okamoto, H. Kawakami, Y.-A. Chien, T. Kurioka, W.-T. Chiu, P. Chakraborty, T. Nakamoto, Y.-J. Hsu, M. Sone and T.-F. M. Chang, Micro Nano Eng., 2023, 18, 100175 CrossRef CAS.
- Y. Shen and L. Zheng, Int. J. Electrochem. Sci., 2023, 18, 6–12 CrossRef.
- N. K. Gurajala, L. Sanjeev, B. Teja, T. S. Kumar and J. E. Manikanta, Mater. Today: Proc., 2023 DOI:10.1016/j.matpr.2023.05.161.
- R. K. Gupta, P. Guha and P. P. Srivastav, Food Chem. Adv., 2022, 1, 100135 CrossRef.
- S. Davidović, V. Lazić, I. Vukoje, J. Papan, S. P. Anhrenkiel, S. Dimitrijević and J. M. Nedeljković, Colloids Surf., B, 2017, 160, 184–191 CrossRef PubMed.
- T. J. Jayeoye, U. Sirimahachai, P. Wattanasin and T. Rujiralai, Microchem. J., 2022, 182, 107949 CrossRef CAS.
- T. S. Ngo, C. T. Tracey, A. G. Navrotskaya, A. V. Bukhtiyarov, P. V. Krivoshapkin and E. F. Krivoshapkina, Carbohydr. Polym., 2023, 304, 120471 CrossRef CAS PubMed.
- R. S. Riseh, M. G. Vazvani, M. Hassanisaadi, V. K. Thakur and J. F. Kennedy, Int. J. Biol. Macromol., 2023, 123708 CrossRef PubMed.
- M. Shahini, B. Ramezanzadeh and H. E. Mohammadloo, J. Mol. Liq., 2021, 325, 115110 CrossRef CAS.
- B. Fouda-Mbanga, E. Prabakaran and K. Pillay, Biotechnol. Rep., 2021, 30, e00609 CrossRef CAS PubMed.
- M. R. Abukhadra, A. M. El-Sherbeeny, M. A. El-Meligy and M. Luqman, Int. J. Biol. Macromol., 2021, 167, 335–344 CrossRef CAS PubMed.
- G. M. Alves, J. L. da Silva and N. R. Stradiotto, Measurement, 2021, 169, 108356 CrossRef.
- P. Ranganathan, B. Mutharani, S.-M. Chen and P. Sireesha, Carbohydr. Polym., 2019, 214, 317–327 CrossRef CAS PubMed.
- R. Sakthivel, S. Palanisamy, S.-M. Chen, S. Ramaraj, V. Velusamy, P. Yi-Fan, J. M. Hall and S. K. Ramaraj, J. Taiwan Inst. Chem. Eng., 2017, 80, 663–668 CrossRef CAS.
- J. Ji, L. Qu, Z. Wang, G. Li, W. Feng and G. Yang, Microchem. J., 2022, 175, 107133 CrossRef CAS.
- H. Pei, F. Chen, X. Niu, Q. Jia, R. Guo, N. Liu and Z. Mo, J. Electroanal. Chem., 2021, 895, 115525 CrossRef CAS.
- V. Gautam, K. P. Singh and V. L. Yadav, Int. J. Biol. Macromol., 2018, 111, 1124–1132 CrossRef CAS PubMed.
- M. Sadeghi and M. Shabani-Nooshabadi, Chemosphere, 2022, 307, 135722 CrossRef CAS PubMed.
- C. M. Primo, E. Buffon and N. R. Stradiotto, Fuel, 2021, 302, 121180 CrossRef CAS.
- X. Wang, C. Karaman, Y. Zhang and C. Xia, Chemosphere, 2023, 331, 138813 CrossRef CAS PubMed.
- M. Elfiky, R. Kumar and A. Beltagi, J. Electroanal. Chem., 2022, 926, 116926 CrossRef CAS.
- E. Lulek, J. Soleymani, M. Molaparast and Y. N. Ertas, Talanta, 2023, 124846 CrossRef CAS PubMed.
- Z. Deng, Z. Wu, H. Rokni, Z. Yin, J. Lin, H. Zhang and S. Cheraghi, Chemosphere, 2022, 309, 136568 CrossRef CAS PubMed.
- M. Rizwan, S. Elma, S. A. Lim and M. U. Ahmed, Biosens. Bioelectron., 2018, 107, 211–217 CrossRef CAS PubMed.
- S. Gao, R. M. Torrente-Rodríguez, M. Pedrero, J. M. Pingarrón, S. Campuzano, J. Rocha-Martin and J. M. Guisán, Talanta, 2022, 247, 123549 CrossRef CAS PubMed.
- B. Thirumalraj, R. Sakthivel, S.-M. Chen, C. Rajkumar, L.-K. Yu and S. Kubendhiran, Microchemical J., 2019, 146, 673–678 CrossRef CAS.
- D. R. Bagal-Kestwal and B.-H. Chiang, Int. J. Biol. Macromol., 2021, 189, 993–1007 CrossRef CAS PubMed.
- A. Chrouda, D. Ayed, K. Zinoubi, H. Majdoub and N. Jaffrezic-Renault, Food Chem. Adv., 2022, 1, 100068 CrossRef.
- A. Jiménez-Rodríguez, E. Sotelo, L. Martinez, Y. Huttel, M. U. González, A. Mayoral, J. M. García-Martín, M. Videa and J. L. Cholula-Díaz, RSC Adv., 2021, 11, 13711–13721 RSC.
- R. M. Hassan, Int. J. Biol. Macromol., 2021, 184, 926–935 CrossRef CAS PubMed.
- V. Hegde, U. Uthappa, T. Altalhi, H.-Y. Jung, S. S. Han and M. D. Kurkuri, Mater. Today Commun., 2022, 104813 CrossRef CAS.
- J. Yang, J. Yao and S. Guan, Sens. Actuators, B, 2020, 322, 128526 CrossRef CAS.
- V. Murugappan and A. Muthadhi, Mater. Today: Proc., 2022, 65, 839–845 CAS.
- N. S. El-Sayed, S. A. Al Kiey, A. Darwish, G. Turky and S. Kamel, Int. J. Biol. Macromol., 2022, 218, 420–430 CrossRef CAS PubMed.
- A. Fuzlin, N. Mazuki, N. Khan, M. Saadiah, M. M. Hasan, Y. Nagao and A. Samsudin, Mater. Chem. Phys., 2022, 287, 126207 CrossRef CAS.
- R. J. Silva, V. Klobukoski, J. I. de Paula, I. C. Riegel-Vidotti and M. Vidotti, Electrochim. Acta, 2022, 429, 140914 CrossRef CAS.
- L. Tang, P. Wu, H. Zhuang, Z. Qin, P. Yu, K. Fu, P. Qiu, Y. Liu and Y. Zhou, Int. J. Biol. Macromol., 2023, 241, 124564 CrossRef CAS PubMed.
- X. Wu, J. Li, Y. Zhu, W. Cai, J. Xu, Y. Qin and Y. Kong, J. Energy Storage, 2023, 66, 107483 CrossRef.
- T. Tripathy, R. K. Saren, S. Banerjee and S. Senapati, Mater. Chem. Phys., 2023, 305, 127995 CrossRef CAS.
- X. Bao, F. Dong, Y. Yu, Q. Wang, P. Wang, X. Fan and J. Yuan, Int. J. Biol. Macromol., 2020, 164, 1237–1245 CrossRef CAS PubMed.
- S. Venkatarajan and A. Athijayamani, Mater. Today: Proc., 2021, 37, 3620–3624 CAS.
- D. Demircan and B. Zhang, Carbohydr. Polym., 2017, 157, 1913–1921 CrossRef CAS PubMed.
- H. Bagheri, T. G. Aqda and M. E. Najafabadi, Microchem. J., 2018, 142, 265–272 CrossRef CAS.
- P. Narayanasamy, P. Balasundar, S. Senthil, M. R. Sanjay, S. Siengchin, A. Khan and A. M. Asiri, Int. J. Biol. Macromol., 2020, 150, 793–801 CrossRef CAS PubMed.
- Y. Zhu, S. Xiao, M. Li, Z. Chang, F. Wang, J. Gao and Y. Wu, J. Power Sources, 2015, 288, 368–375 CrossRef CAS.
- F. Ettadili, S. Aghris, F. Laghrib, A. Farahi, M. Bakasse, S. Lahrich and M. El Mhammedi, Int. J. Biol. Macromol., 2023, 242, 124995 CrossRef CAS PubMed.
- S. A. Al Kiey, A. M. Khalil and S. Kamel, Int. J. Biol. Macromol., 2023, 239, 124302 CrossRef CAS PubMed.
- D. Büyüktaş, M. Ghaani, C. Rovera, D. Carullo, R. T. Olsson, F. Korel and S. Farris, Heliyon, 2023, 9, e15327 CrossRef PubMed.
- A. Santhosh, S. Sandeep, D. J. Bound, S. Nandini, S. Nalini, G. Suresh, N. K. Swamy, J. R. Rajabathar and A. Selvaraj, Surf. Interfaces, 2021, 26, 101377 CrossRef CAS.
- H. Liu, D. Wang, Z. Song and S. Shang, Cellulose, 2011, 18, 67–74 CrossRef CAS.
- N. Alahmadi, H. S. Alhasan, H. Gomaa, A. A. Abdelwahab and M. Y. Emran, Microchem. J., 2022, 183, 107909 CrossRef CAS.
- Z. Deng, Z. Wu, M. Alizadeh, H. Zhang, Y. Chen and C. Karaman, Environ. Res., 2023, 219, 114995 CrossRef CAS PubMed.
- E. Sohouli, E. M. Khosrowshahi, P. Radi, E. Naghian, M. Rahimi-Nasrabadi and F. Ahmadi, J. Electroanal. Chem., 2020, 877, 114503 CrossRef CAS.
- R. H. Althomali, K. A. Alamry, M. A. Hussein and R. Guedes, Diamond Relat. Mater., 2022, 122, 108803 CrossRef CAS.
- J. Lv, X. Lv, M. Ma, D.-H. Oh, Z. Jiang and X. Fu, Carbohydr. Polym., 2022, 120142 Search PubMed.
- M. M. Al-Rooqi, M. M. Hassan, Z. Moussa, R. J. Obaid, N. H. Suman, M. H. Wagner, S. S. Natto and S. A. Ahmed, J. Saudi Chem. Soc., 2022, 101561 CrossRef CAS.
- K. Mohan, A. R. Ganesan, P. Ezhilarasi, K. K. Kondamareddy, D. K. Rajan, P. Sathishkumar, J. Rajarajeswaran and L. Conterno, Carbohydr. Polym., 2022, 287, 119349 CrossRef CAS PubMed.
- M. Yahyaei, F. Mehrnejad, H. Naderi-Manesh and A. H. Rezayan, Carbohydr. Polym., 2018, 191, 191–197 CrossRef CAS PubMed.
- J. Chalitangkoon, A. Ronte and P. Monvisade, J. Taiwan Inst. Chem. Eng., 2023, 149, 105001 CrossRef CAS.
- P. Sánchez-Cid, A. Romero, M. Díaz, M. de-Paz and V. Perez-Puyana, J. Mol. Liq., 2023, 379, 121735 CrossRef.
- X. Li, L. Jiang, M. Yan, H. Bi and Q. Wang, Int. J. Biol. Macromol., 2023, 242, 124780 CrossRef CAS PubMed.
- A. N. Raja, Int. J. Biol. Macromol., 2020, 164, 4231–4244 CrossRef PubMed.
- V. K. Singh, C. S. Kushwaha and S. Shukla, Int. J. Biol. Macromol., 2020, 147, 250–257 CrossRef CAS PubMed.
- C. Topcu, B. Caglar, A. Onder, F. Coldur, S. Caglar, E. K. Guner, O. Cubuk and A. Tabak, Mater. Res. Bull., 2018, 98, 288–299 CrossRef CAS.
- D. Ikkene, A. Arteni, H. Song, H. Laroui, J. Six and K. Ferji, Carbohydr. Polym., 2020, 234, 115943 CrossRef CAS PubMed.
- P. D. Karmakar and S. Pal, Int. J. Biol. Macromol., 2021, 183, 718–726 CrossRef CAS PubMed.
- J. Yue, L. He, Y. Tang, L. Yang, B. Wu and J. Ni, J. Photochem. Photobiol., B, 2019, 197, 111530 CrossRef CAS PubMed.
- S. B. Aziz, M. Brza, K. Mishra, M. Hamsan, W. O. Karim, R. M. Abdullah, M. Kadir and R. T. Abdulwahid, J. Mater. Res. Technol., 2020, 9, 1137–1150 CrossRef CAS.
- M. Hamsan, S. B. Aziz, M. Azha, A. Azli, M. Shukur, Y. M. Yusof, S. K. Muzakir, N. S. Manan and M. F. Z. Kadir, Mater. Chem. Phys., 2020, 241, 122290 CrossRef CAS.
- B. Li, A. Yu and G. Lai, Carbon, 2018, 127, 202–208 CrossRef CAS.
- H. Filik and A. A. Avan, Food Chem., 2019, 292, 151–159 CrossRef CAS PubMed.
- M. Ahmadian, H. Derakhshankhah and M. Jaymand, J. Ind. Eng. Chem., 2023, 124, 102–131 CrossRef CAS.
- F. Rasool, I. Zahoor, W. S. Ayoub, T. A. Ganaie, A. H. Dar, S. Farooq and T. A. Mir, Food Chem. Adv., 2023, 100366 CrossRef.
- S. N. Suresh, C. Puspharaj and R. Subramani, Food Hydrocolloids Health, 2022, 2, 100087 CrossRef.
- V. Sharma, B. Singh and P. Sharma, J. Drug Delivery Sci. Technol., 2023, 84, 104470 CrossRef CAS.
- M. Hanumanthappa, J. H. Siddalingappa, Y. Channabasaveshwara, S. Sundararajan, M. Vishwas, S. K. Srinivasaiah, S. B. Siddegowda and B. Nandeesh, Chem. Phys. Mater., 2022, 1, 330–337 Search PubMed.
- H. Madanakumara, H. Jayanna, C. Yelamaggad, S. Soundeswaran, M. Vishwas, K. Shamala, B. Surendra and N. Basavaraju, Sens. Int., 2022, 3, 100193 CrossRef.
- K. Le Van Vu, N. T. T. Tran, D. N. Nguyen, L. T. T. Nguyen and T. D. Phan, Radiat. Phys. Chem., 2023, 211, 111061 CrossRef.
- J. Ma, T. Li, Q. Wang, C. Xu, W. Yu, H. Yu, W. Wang, Z. Feng, L. Chen and J. Hou, Food Chem., 2023, 413, 135680 CrossRef CAS PubMed.
- A. M. Hassan, M. Ayoub, M. Eissa, T. Musa, H. Bruining and R. Farajzadeh, Energy, 2019, 181, 162–172 CrossRef CAS.
- P. Chai, P. Choy, W. Teoh, E. Mahmoudi and W. Ang, J. Environ. Chem. Eng., 2021, 9, 105638 CrossRef CAS.
- C. Verma and M. Quraishi, Int. J. Biol. Macromol., 2021, 184, 118–134 CrossRef CAS PubMed.
- A. I. El-Batal, M. Abd Elkodous, G. S. El-Sayyad, N. E. Al-Hazmi, M. Gobara and A. Baraka, Int. J. Biol. Macromol., 2020, 165, 169–186 CrossRef CAS PubMed.
- A. S. Agnihotri, M. Nidhin, S. Rison, K. Akshaya and A. Varghese, Appl. Surf. Sci. Adv., 2021, 6, 100181 CrossRef.
- M. Zahran, Z. Khalifa, M. A. Zahran and M. A. Azzem, Electrochim. Acta, 2021, 394, 139152 CrossRef CAS.
- T.-A. Le and T.-P. Huynh, Eur. Polym. J., 2023, 111852 CrossRef CAS.
- S. S. Garg and J. Gupta, Int. J. Biol. Macromol., 2023, 229, 476–485 CrossRef CAS PubMed.
- A. Gunasekaran, A. Sorrentino, A. M. Asiri and S. Anandan, Sol. Energy, 2020, 208, 160–165 CrossRef CAS.
- X. Geng, R. Xue, S. Teng, W. Fan, G. Wang, J. Li, Y. Liu, Z. Huang and W. Yang, Anal. Chim. Acta, 2023, 1267, 341393 CrossRef CAS PubMed.
- R. Ramya, D. Nathiya, P. Thivya and J. Wilson, Microchem. J., 2021, 170, 106734 CrossRef CAS.
- M. Petitjean and J. R. Isasi, Int. J. Biol. Macromol., 2021, 180, 570–577 CrossRef CAS PubMed.
- O. E. Adedeji, J.-Y. Choi, G. E. Park, H. J. Kang, M. O. Aminu, J. H. Min, C. E. Chinma, K.-D. Moon and Y. H. Jung, Innovative Food Sci. Emerging Technol., 2022, 80, 103086 CrossRef CAS.
- L. M. Giuliani, N. S. Pegoraro, C. Camponogara, B. F. Osmari, T. de Bastos Brum, J. B. Reolon, G. C. Rechia, S. M. Oliveira and L. Cruz, J. Drug Delivery Sci. Technol., 2022, 78, 103960 CrossRef CAS.
- T. Li, W. Chi, Y. Ning, S. Xu and L. Wang, Int. J. Biol. Macromol., 2023, 226, 267–278 CrossRef CAS PubMed.
- A. E. D. Maddalozzo, R. Frassini, C. P. Fontoura, M. M. Rodrigues, C. O. da Silva Frozza, C. A. Figueroa, M. Giovanela, C. Aguzzoli, M. Roesch-Ely and J. da Silva Crespo, Reactive Funct. Polym., 2022, 177, 105316 CrossRef CAS.
- N. B. Guerra, G. S. A. Pegorin, M. H. Boratto, N. R. de Barros, C. F. de Oliveira Graeff and R. D. Herculano, Mater. Sci. Eng., C, 2021, 126, 112126 CrossRef CAS PubMed.
- J. Jayadevan, M. Manathanath, M. M. Mathew and U. G. Panicker, Int. J. Biol. Macromol., 2023, 231, 123286 CrossRef CAS PubMed.
- P. P. R. Krishnan, P. A. Kumar and K. Prabhakaran, Ceram. Int., 2022, 48, 14839–14848 CrossRef.
- W. Wichaita, D. Promlok, N. Sudjaipraparat, S. Sripraphot, T. Suteewong and P. Tangboriboonrat, Eur. Polym. J., 2021, 159, 110740 CrossRef CAS.
- M. Zahran, Z. Khalifa, M. A.-H. Zahran and M. A. Azzem, Electrochim. Acta, 2020, 356, 136825 CrossRef CAS.
- H. Cakmak, H. Ilyasoglu-Buyukkestelli, E. Sogut, V. H. Ozyurt, C. E. Gumus-Bonacina and S. Simsek, Food Hydrocolloids Health, 2023, 100131 CrossRef CAS.
- S. S. Fernandes, P. da Silva Cardoso, M. B. Egea, J. P. Q. Martínez, M. R. S. Campos and D. M. Otero, Food Res. Int., 2023, 113125 CrossRef CAS PubMed.
- Y. Yang, V. K. Gupta, Y. Du, M. Aghbashlo, P. L. Show, J. Pan, M. Tabatabaei and A. Rajaei, Int. J. Biol. Macromol., 2023, 124800 CrossRef CAS PubMed.
- G. Hosseinzadeh, S. Zinatloo-Ajabshir and A. Yousefi, Ceram. Int., 2022, 48, 6078–6086 CrossRef CAS.
- N. Adjeroud, S. Elabbas, B. Merzouk, Y. Hammoui, L. Felkai-Haddache, H. Remini, J.-P. Leclerc and K. Madani, J. Electroanal. Chem., 2018, 811, 26–36 CrossRef CAS.
- E. Darvishi, H. Ehzari, M. Shahlaei, L. Behbood and E. Arkan, Bioelectrochemistry, 2021, 139, 107744 CrossRef CAS PubMed.
- M. Zahran, Z. Khalifa, M. A. Zahran and M. A. Azzem, Microchem. J., 2021, 106173 CrossRef CAS.
- I. S. V. da Silva, R. M. F. de Sousa, A. de Oliveira, W. J. de Oliveira, L. A. C. Motta, D. Pasquini and H. Otaguro, Carbohydr. Polym., 2018, 202, 203–210 CrossRef PubMed.
- A. Rehman, T. Ahmad, R. M. Aadil, M. J. Spotti, A. M. Bakry, I. M. Khan, L. Zhao, T. Riaz and Q. Tong, Trends Food Sci. Technol., 2019, 90, 35–46 CrossRef CAS.
- M. Shabeena, S. Kouser, A. Prabhu, G. Nagaraja, D. Warale and D. Manasa, J. Drug Delivery Sci. Technol., 2023, 82, 104320 CrossRef CAS.
- M. Suhasini, K. Rajeshwari, S. Bindya, A. Hemavathi, P. M. Vishwanath, A. Syed, R. Eswaramoorthy, R. G. Amachawadi, C. Shivamallu, V. K. Chattu, S. S. Majani and S. P. Kollur, Heliyon, 2023, 9, e15792 CrossRef PubMed.
- F. J. Arévalo, Y. Osuna-Sánchez, J. Sandoval-Cortés, A. Di Tocco, A. M. Granero, S. N. Robledo, M. A. Zon, N. R. Vettorazzi, J. L. Martínez and E. P. Segura, Sens. Actuators, B, 2017, 244, 949–957 CrossRef.
- A. Di Tocco, S. N. Robledo, Y. Osuna, J. Sandoval-Cortez, A. M. Granero, N. R. Vettorazzi, J. Martínez, E. Segura, A. Iliná and M. A. Zon, Talanta, 2018, 190, 30–37 CrossRef CAS PubMed.
- V. Mani, R. Devasenathipathy, S.-M. Chen, S.-F. Wang, P. Devi and Y. Tai, Electrochim. Acta, 2015, 176, 804–810 CrossRef CAS.
- L. He, C. Lin, Y. Zhao, W. Gao, H. Zhang, B. Lin and D. Sun, Ceram. Int., 2022, 48, 8104–8111 CrossRef CAS.
- P. Rai, S. Mehrotra, K. Gautam, A. K. Kar, A. Saxena, S. Patnaik, S. Anbumani, A. Pandey, S. Priya and S. K. Sharma, Carbohydr. Polym., 2023, 302, 120368 CrossRef CAS PubMed.
- K. Wu, D. Yang, J. Guo, P. Fu, R. Wu, Y. Zheng and M. Wu, Sol. Energy, 2021, 213, 126–135 CrossRef CAS.
- S. M. Azab, J. Electroanal. Chem., 2019, 840, 319–327 CrossRef CAS.
|
This journal is © The Royal Society of Chemistry 2024 |