DOI:
10.1039/D2VA00275B
(Perspective)
Environ. Sci.: Adv., 2023,
2, 356-367
The emergence of microplastics: charting the path from research to regulations
Received
8th November 2022
, Accepted 31st January 2023
First published on 1st February 2023
Abstract
Despite decades of research detailing widespread contamination and potential risks of microplastics (synthetic particles <5 mm) to humans and ecosystems by the scientific community, government agencies have made little progress to address the emerging contaminant class. Research on microplastics has increased exponentially in recent years, however translation of studies and data into knowledge that's useful for management requires clear communication between the scientific and management communities. Legislative mandates to address microplastics in drinking water and aquatic ecosystems in California prompted rapid development of fundamental tools and methods for identifying and assessing risks, including a legal definition, analytical monitoring methods, and risk assessment frameworks. While this scientific progress provides a baseline foundation for developing regulations for microplastics in California and other jurisdictions, additional research is needed to reduce uncertainties and overcome logistical barriers that are preventing the full emergence of microplastics as a regulated contaminant suite. This paper provides critical insights from both scientific and regulatory perspectives regarding recent advances in the field and recommends a path forward to overcome barriers.
Environmental significance
The emergence of microplastics presents significant challenges to scientists and governments to monitor and manage their risks to humans and ecosystems. Research on microplastics has increased exponentially in recent years, however translation of studies and data into knowledge that's useful for management requires clear communication between the scientific and management communities. This paper summarizes recent groundbreaking research and regulations for microplastics in drinking water and aquatic environments in California and provides critical insights and recommends to overcome additional scientific and logistical barriers.
|
Introduction
Although the ingestion of microplastics (“MPs”; typically defined as synthetic particles <5 mm) by marine organisms has been documented as early as 1972,1 and the ability for small MPs to cross intestinal barriers and distribute throughout the blood stream in mammals was first reported in 1960,2 the contaminant suite remains virtually un-regulated globally,3 and significant scientific data gaps remain. MPs' long ‘failure to launch’ from a novel research topic into a serious regulatory investigation can be attributed in part to its innate ‘wickedness’ (defined as an ill-formulated problem with confusing information, many clients and decision makers with conflicting values, and confusing ramifications on systems);4 in terms of political, societal, and scientific complexities.5,6 MPs' scientific complexities arise from their insolubility, diverse formulations, and transformations in the environment, producing infinite combinations of numerous physical and chemical parameters (e.g., shape, size, polymer, density, charge, sorbed and added chemicals, biofouling, etc.). Such complexity presents challenges for environmental monitoring and characterizations of toxicity, significantly hindering efforts to manage the contaminant class using traditional regulatory approaches in which hazards and exposure are directly compared.7 However, recent developments to address the multidimensionality of this emerging contaminant suite now allow for more reliable assessments of risks than previously possible (albeit still with significant uncertainty).8–10 These advances provide the scientific foundation for governmental bodies to make regulatory decisions regarding MPs – however some may choose to act in a precautious manner regardless.7 While these and other recent scientific advances provide fundamental and long-awaited tools to monitor and assess risks of MPs in the environment, managing MP pollution will likely require far-reaching and disruptive actions across many facets of society.6
To date, few governments have attempted to address MP pollution through regulations, with most efforts focused on restricting intentionally added primary production MPs in consumer products3 or restricting releases of pre-production MP pellets from manufacturing facilities.11 In 2018, the State of California (United States of America) set forth the world's first legal mandates to regulate MPs in aquatic ecosystems and in drinking water with the passage of Senate Bills 1422 and 1263.12,13 These ground-breaking legislation require the state's regulatory agencies to legally define MPs, develop a framework for assessing risks to humans and ecosystems, adopt standardized analytical methods for monitoring in the environment, determine sources and pathways into the environment, and implement intervention strategies to reduce further contamination.12,13 While these laws have already impelled progress towards developing regulations for MPs in California and elsewhere,14 significant scientific, technical, and logistical challenges must be overcome until MPs can fully “emerge” as a contaminant suite. This paper provides an overview of this research from the perspective of both a scientist and a regulator and makes key recommendations for addressing remaining challenges. First, some important considerations of defining MPs (both from a scientific and legal perspective) are discussed followed by significant advances in analytical monitoring methodologies; ecological hazard assessment and risk characterization; human health hazard assessment via drinking water; and finally novel strategies to monitor MPs in a cost-effective and logistically feasible manner leveraging recent research advances.
Defining the problem
The first step in addressing any problem is defining it. Although MPs have long been generally understood to be “synthetic plastic particles smaller than 5 mm”, few explicit definitions exist – and none have been universally agreed upon – despite requests from the scientific community.15,16 Beyond obvious benefits to scientists, clear, and legally defensible definitions are critically important to enable regulations. A principal challenge in drawing boundaries around the contaminant suite has been the lack of a comprehensive understanding of the traits influencing these particles' hazards (e.g., size, shape, polymer type, biodegradability, etc.).16 In an attempt to avoid defining MPs so narrowly that potentially hazardous synthetic particles go unreported,7 the California State Water Resources Control Board adopted a broad regulatory definition that encompasses synthetic polymers of any origin and material (e.g., petroleum-, bio- and inorganic-based), with exceptions only for polymers occurring in nature that have not undergone chemical modification (other than by hydrolysis).17 With respect to size, the California definition harmonizes with historical definitions that include particles smaller than 5 mm – therefore including ‘nanoplastics’.18 While the California definition is largely similar to a draft definition introduced by the European Chemicals Agency designed with the intention to restrict intentionally added MPs in products, the California definition is broader due to its inclusion of biodegradable polymers, water-soluble polymers, and particles as small as 1 nm (the European definition excludes particles <100 nm) – significant deviations that reflects the differences in both the intended uses of these definitions (i.e. environmental monitoring vs. product restrictions) and practical restrictions (e.g., confirming presence of <100 nm particles is technically challenging).19
As regulatory agencies and the scientific community at-large move towards a universally harmonized definition with the goal of reducing confusion and enabling reliable comparisons between studies and data, care must be taken to ensure that definitions are fit-for-purpose for the intended use (e.g., monitoring, restrictions, etc.) and broad/flexible enough to capture new and/or poorly documented synthetic polymeric particles. Finally, tools enabling harmonized reporting of the vast diversity of particle traits (e.g., polymers, colors, shapes, sizes, etc.) should be made widely available, be open-source, and be actively improved upon by the scientific community.
Standardizing monitoring methods
The ability to reliably compare monitoring data through the use of harmonized and/or standardized methods is essential to assessing risks and enacting regulations under most jurisdictions worldwide. Although many analytical methods exist for detecting MPs, spectroscopic-based techniques are the most widely used and well-developed.20 While the reliability of spectroscopic (e.g., Fourier transform infrared spectroscopy [FTIR]; Raman) analytical techniques have been demonstrated for MPs previously, their application in regulatory scenarios requires additional demonstration of the methods' capabilities (e.g., specificity, sensitivity, robustness, and repeatability across laboratories).
In the absence of validated methods, the Southern California Coastal Water Research Project conducted an inter-laboratory validation study to meet California's regulatory needs. The study involved participants from 22 laboratories across diverse sectors (industry, government, non-governmental organizations, and academia) and in six different countries, each of which received known types and quantities of spiked MPs within four size fractions (1–20 μm, 20–212 μm, 212–500 μm, >500 μm), four polymer types (polyethylene, polystyrene, polyvinyl chloride, and polyethylene terephthalate), and six colors (clear, white, green, blue, red, and orange) as well as false positives in a simulated drinking water matrix.21 Each laboratory extracted (via filtering/sieving) and analysed the particles according to specific prescribed methodologies using either optical microscopy alone or in combination with FTIR or Raman spectroscopy.21 Mean recovery of particles across all size ranges among laboratories using stereomicroscopy was 76% ± 10% (standard error), however results differed dramatically between the three largest size fractions (i.e., 20–212 μm, 212–500 μm, >500 μm; 92% ± 12% standard deviation) and the smallest size fraction (i.e., 1–20 μm; 32 ± 16% standard deviation).21 Accurate identification of polymer type by FTIR and Raman spectroscopy across laboratories was 95% and 91%, respectively.21 A summary of each analytical methods' strengths and weaknesses is included in Table 1.
Table 1 Overview of strengths and weaknesses of analytical methods used for monitoring MPs from an inter-laboratory validation study (n = 22 laboratories) reported in ref. 21. Values are color-coded by least-desirable to most-desirable within each column (red = least, yellow = intermediate, green = highest)
In addition to determining analytical method performance (e.g., accuracy, precision, etc.), De Frond et al.21 also quantified resources required for each analytical method (e.g., time, money) (Table 1). The interlaboratory validation study found that the standard operating protocol for microplastics extraction and analysis takes considerably longer time than many traditional chemical analyses (e.g., gas chromatography mass spectrometry typically takes less than one hour), with laboratories completing the extraction step in a mean of 15 hours per sample (±26 hours s. d.), visual microscopy in 26 hours per sample (±54 hours s. d.), and FTIR and Raman spectroscopy in 10 (±9 hours s. d.) and 15 hours per sample (±16 hours s. d.), respectively (Table 1).21 To reduce time needed for analysis, the standardized method based on the interlaboratory study allows subsampling of particles for chemical confirmation using spectroscopy,22,23 which was determined using data from the interlaboratory study and described in ref. 21. While this inter-laboratory validation study provided sufficient justification for California's State Water Resources Control Board to adopt standardized MPs analytical methodologies for regulatory use, the agency acknowledges room for improvement and is promoting refinements to these methods – especially in the accurate quantification of particles smaller than 20 μm and in faster analysis times.24
Assessing hazards of microplastics in aquatic ecosystems
Accurately quantifying exposure to MPs using standardized analytical methods is one of two key elements to assessing risks-the other being quantitative hazard thresholds. For MPs, risk assessments have long been challenged by “non-alignments” (i.e., mismatches) between particle types used in toxicity studies and particles documented in the environment, as differences in size, shape, density, and other factors may significantly influence toxicity and the concentrations at which they occur – often by several orders of magnitude.25 As a result, risk assessments that have failed to account for these critically important non-alignments estimated that risks in aquatic environments are negligible or highly improbable (e.g.,26,27). The recent development of a framework to account for these non-alignments,25 availability of high-resolution monitoring data in multiple matrices,28,29 and a weight-of-evidence for mechanistic impacts in aquatic biota30 for the first time provides the scientific foundation for reliably assessing risks to ecosystems.8 Leveraging these recent scientific advances, the Southern California Coastal Water Research Project facilitated a health effects workshop to critically assess evidence of toxicities of MPs to aquatic organisms and humans (via ingestion route of exposure) to develop quantitative toxicity thresholds for managing risks, provide qualitative health guidance for communicating potential risks to consumers with MPs in drinking water, and make recommendations for additional research to refine future hazard assessments.14
The California MPs health effects workshop produced a number of databases, tools, and frameworks relevant for risk assessment and management based on a literature review of published studies. Over 220 toxicity studies were mined for more than 70 unique variables relating to experimental design, test organisms, biological effects, and particle characteristics of MPs used.31 Each study was then screened by two independent reviewers based on quality criteria developed by de Ruijter et al. (2019) (for non-mammalian studies) and by Gouin et al. (2022) for mammalian studies. All reported exposure metrics were obtained from studies, and when unreported, were estimated using reported characteristics and geometric calculations.31 Exploratory data analysis and modelling using binomial logistic regressions revealed insights into particle traits influential for various pathways of toxicity for MPs – demonstrating that while surface area and volume are significant predictors of toxicity (p values = 0.0012 and 0.038, respectively), studies should report (at minimum) particle count and mass (even though neither exposure metrics were statistically significant predictors of toxicity).32 The toxicity databases, tools to estimate particle characteristics, align toxicity studies, screen studies for quality criteria, and more were made freely available in an open-source and open-data repository utilizing an Rshiny web application called the Toxicity of Microplastics Explorer (“ToMEx”) (https://github.com/SCCWRP/aq_mp_tox_shiny).31 Additionally, ToMEx allows users to upload additional published toxicity data – therefore enabling rapid and real-time meta-analyses and assessments of risk and reducing burdens of future assessments.31
To inform California's management of MPs in aquatic ecosystems, the health effects workshop devised a framework that includes four risk-based threshold tiers each with increasing levels of critical actions recommended to be undertaken by the appropriate regulatory bodies, ranging from low concern – prompting additional investigative monitoring – to the highest level of concern – pollution control measures.10 Using the best available data, risk-based thresholds were derived using species sensitivity distributions10 – a statistical approach which is commonly used by regulatory authorities in many jurisdictions globally to set limits in the environment.33 To account for differences between particles used in and between laboratory toxicity studies (68% of which were ‘monodisperse’ – i.e., a single particle type) and in the environment (32% were ‘polydisperse’ – i.e., many different particle types), toxicity data were aligned to each of two ecologically relevant metrics for demonstrated effect mechanisms of MPs in aquatic organisms – food dilution (volume), and tissue-translocation mediated toxicities (surface area).10,30 Large, high-resolution environmental monitoring datasets across multiple locations were used to align data based on size, shape, and density distributions to enable broadly applicable compartment-specific thresholds, however site-specific monitoring data (if available) can be easily substituted to derive thresholds applicable to a given location using the ToMEx application.10,31 The resulting thresholds derived by Mehinto et al. (2022) range from ∼0.5 to 35 particles per L (1 to 5000 μm MPs) for food dilution, and 60 to 4100 particles per L (1 to 5000 μm MPs) for tissue translocation-mediated toxicities. Health effects workshop participants expressed high confidence in both the management framework and the analytical process used to derive the thresholds but expressed medium confidence in the resulting thresholds for food dilution and low confidence for tissue-translocation mediated effects due to the underlying studies not meeting all desired quality criteria (in particular, dose–response effect concentrations such as EC50's).10 Main areas of uncertainties stemmed from: limited data of sufficient quality; the lack of established adverse outcome pathways to support effect mechanisms and ecologically relevant metrics for which data were aligned; and unclear environmental relevance – as few studies used fibers – which are frequently detected in the environment10,34 and suspected to exert higher toxicity relative to other shapes.35,36
Improving study quality and reporting is paramount to reducing uncertainties in future hazard assessments. To assess whether MPs toxicity studies have increased in reliability and applicability to risk assessment over time, quality scores assigned by Mehinto et al.10 (n = 160) were extracted from the ToMEx database (https://sccwrp.shinyapps.io/aq_mp_tox_shiny/) on May 28, 2022, and linear regressions were performed with quality criteria scores (total accumulated score, risk applicability score, technical quality score) as dependent variables and study publication year as the independent variable (Fig. 1). While the total accumulated score increased significantly over time (R2 = 0.04, p < 0.001), it has progressed slowly – with a modelled increase of ∼0.002 points per year (Fig. 1). Improvements in study quality are only significant for technical quality (p < 0.001) however, and applicability to risk assessments have not changed significantly over time (p = 0.447) (Fig. 1).
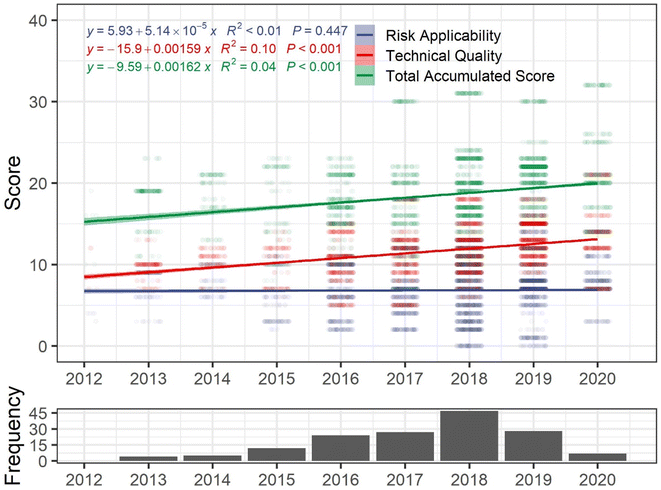 |
| Fig. 1 Quality of microplastics toxicity studies of aquatic organisms compared to year of publication. Top – scatterplot showing quality scores (risk = blue; technical = red) assessed in Thornton Hampton et al.(2022c) for each year with linear regression lines. A significant time trend is only observed for technical quality scores, and not applicability for risk assessment scores. Bottom – relative number of studies published by year is shown as a barplot. Total number of studies = 160. | |
Quality criteria relating to a study's applicability to risk assessment proved to be the limiting factor for inclusion of studies in the health effects workshop assessment,10 which as demonstrated in Fig. 1, has not been trending in a positive trajectory over time. To improve applicability to risk assessments, MPs hazard studies should focus on utilizing at least five exposure concentrations (including a treatment and control), report effect thresholds, use at least one environmentally relevant concentration within the tested range, use environmentally realistic MPs particles (e.g., aged and biofouled), and use a diversity of MPs particles.30,37 While not all hazard studies need to be designed to inform risk assessments, researchers should carefully define the goals of their experiments (e.g., mechanisms of action, qualitative effects screening, quantitative dose–response, etc.) at the outset. Finally, to avoid unnecessary replication and provide the most useful data for risk assessors (and regulators), researchers may consult the ToMEx application and database to identify data gaps.
Characterizing risks to ecosystems
The risk assessment framework developed by California's expert workshop (i.e.,10) was recently applied to monitoring data in San Francisco Bay, California.9 Risks were assessed using the traditional ecotoxicological approach of dividing the predicted environmental concentrations by predicted no-effect concentrations (i.e., risk thresholds derived by10), with a ratio <1 suggesting there is no significant risk associated with the contaminant. As MPs were collected using a manta trawl net, the monitoring data contained systematic biases (i.e., undercounting of particles smaller than the mesh size, and under-counting of fibers) that needed to be accounted for to enable direct comparisons to Mehinto et al.10's thresholds.
MPs from San Francisco Bay were collected in sediment, fish tissue, wastewater discharge, stormwater effluent, and surface water using a variety of sampling techniques and were extracted and counted manually using optical microscopy followed by confirmation of polymer composition via subsampling with FTIR (with attenuated total reflectance) and Raman spectroscopy.38,39 Currently, hazard thresholds for marine ecosystems are only available for surface water, so occurrence data in the other matrices listed were not evaluated for risks.9 Surface water data were assessed for quality according to the criteria defined in Koelmans et al.,40 and while data obtained using manta trawl nets was considered appropriate for risk assessment, data obtained by 1 L grab samples could not be used due to the low sampling volume (at least 500 L required).9 To enable comparisons between the ambient concentrations obtained via manta trawl and the ecological risk thresholds developed by Mehinto et al.,10 three types of corrections were performed:9 monitoring data (>333 μm) was rescaled to the hazard thresholds size distribution (1 to 5000 μm) using probability distributions; systematic exclusion of microfiber particle counts by analysts was accounted for based on a subset of manta trawl samples in which all fibers were counted (i.e.,41); and overcounting of plastic particles due to spectroscopic interference was corrected for using FTIR data reported in Zhu et al.,.38 Uncertainties due to corrections were propagated probabilistically using best available techniques, including derivation of probability distributions and Monte-Carlo modelling.9
The majority of corrected surface water samples from San Francisco Bay (82%) exceeded the most conservative risk threshold (i.e., 0.3 particles per L based on food dilution), indicating that additional investigative monitoring would be warranted according to the risk assessment framework developed by the expert workshop (i.e.,10).9 Far fewer samples exceeded thresholds recommending more costly regulatory actions such as discharge monitoring (27%), management planning such as setting a total maximum daily load or related measures (21%) or controlling MPs emissions at sources (3%).9 In contrast to global risk characterizations that did not account for non-alignments in particle counts resulting from differing sizes measured in the environment versus those causing toxicity in laboratory experiments (e.g.,26,27), risk exceedances documented in Coffin et al.9 are substantial.
Despite relatively high uncertainties, the risk characterization of MPs in San Francisco Bay represents one of the most accurate and reliable comparisons to-date due to the use of alignments, high-quality monitoring data (total assessment score of 13 compared to an average 7.9 in ref. 40), data-driven corrections with errors propagated probabilistically, and medium confidence ecological risk thresholds.9 Corrections for systematic fiber undercounting in manta trawl nets accounted for over 90% of the total uncertainty, while corrections due to spectroscopic subsampling and rescaling for size introduced marginal uncertainties.9 While regulatory agencies have utilized class-based approaches and estimation methods for some toxicants (e.g., toxic equivalency factors for polychlorinated biphenyls), corrections required to assess risks of MPs in this case are significantly more complex – requiring relatively sophisticated modelling tools and large, high-resolution datasets – aspects which will understandably factor into regulatory decisions based on this assessment. Additionally, because the necessary corrections introduced relatively high uncertainties into the risk characterization (e.g., 95% confidence interval includes 3% to 73% of samples exceeding the threshold recommending discharge monitoring), any regulatory decisions based on this risk characterization are unlikely to result in a definitive or severe ruling (e.g., total maximum daily load).
To date, the San Francisco Bay risk characterization (i.e.,9) is among one of two other fully aligned MPs risk assessments in aquatic environments (i.e.,25,42) and represents a rapidly changing paradigm within the field.8 As demonstrated in the first of such MPs risk assessment to account for non-alignments between particle sizes in toxicity studies and the environment,25 risk assessments that do not rescale particle concentrations (e.g.,26,43) dramatically underestimate risks. Owing to the recent rapid progression of the field,44 uncertainties in MPs ecological risk assessments have dropped precipitously in less than two years. In the first such study, Koelmans et al.25 demonstrated the principle of non-alignments by characterizing risks in global freshwater ecosystems using the best available data – which included highly uncertain particle size distributions. Building on Koelmans et al.,25 Coffin et al.9 utilized threshold data from an improved alignment methodology (i.e.10), and rescaled concentrations using high-resolution particle size distribution data from ref. 28. Despite these improvements, the risk assessment in San Francisco Bay suffered from significant uncertainties due to the hazard thresholds being based on studies passing a minimum set of quality standards that excluded other desired criteria such as effect concentrations.10 The most recent aligned MPs risk assessment likely contains the least uncertainty of any such study to date, as Redondo-Hasselerharm42 characterized risks of MPs in freshwater sediments worldwide using strict quality criteria in effect studies (92% of which used environmentally realistic polydisperse MPs), and high-resolution size distribution data. The authors found that for all locations considered, exposure concentrations were either below or in the margin of uncertainty of the hazard concentration affecting 5% of species, therefore concluding that risks to freshwater benthic communities cannot be excluded.42
To enable more reliable ecological risk characterizations of MPs, monitoring studies should collect large volume samples using filtration methods with small mesh sizes (<300 μm) in addition samples obtained using nets – therefore reducing uncertainties introduced by size rescaling and undercounting of fibers while ensuring adequate spatial representation.45 Furthermore, best practices for sample collection, analysis, spectroscopic subsampling, and data reporting should be employed to ensure data are reliable for further assessments.46–49 Finally, the use of high-resolution (and preferably automated) spectroscopic analysis of a small number of samples would enable more accurate size rescaling through the use of site-specific size distribution data, however few laboratories currently have access to such technologies due to their relatively high costs.
Assessing hazards of microplastics to humans through drinking water
In addition to assessing ecological hazards, determining hazards to humans through drinking water was a primary objective of the California health effects workshop. A total of 41 in vitro and 31 in vivo (mouse and rat) studies using mammals were identified from the peer-reviewed literature, however due to the lack of a quantitative method to relative in vitro MPs toxicity data to in vivo settings, only the in vivo studies were further assessed.50 Following an initial screening for quality control, only 12 out of 31 in vivo studies underwent further evaluation by a wider group of experts with specializations in each study's particular field.50 Monodisperse polystyrene spheres were used in 83% of studies passing the first tier of screening, ranging between 0.040 and 20 μm in diameter.50 Four of the studies passing screening criteria reported adverse effects on male reproductive systems, across which demonstrated consistent effects relating to a reduction in the number and proportion of viable sperm, increased sperm deformities, and the apoptosis of sperm cells accompanied by a dose-related expression of cytokines (a biomarker of underlying inflammation).50–54 Due to the lack of a proper analysis of testicular histology in any of these studies, it was not possible to determine whether the observed effects resulted from a direct response to the exposure to the test materials, or a secondary response resulting from inflammation.50 Additional findings of adverse impacts to female reproductive systems in two studies passing the quality screening criteria55,56 increased the expert workshop's confidence of a causal relationship between exposure to polystyrene spheres and the observed impacts.50 The additional studies evaluated by subject-matter experts reported impacts to the heart, liver, thyroid, and gut microbiome, however none contained reliable measurements for apical endpoints and thus were not suitable for assessing hazards.50
Quantitative toxicity thresholds were estimated for each of the reliably apical endpoints using benchmark dose modelling software, resulting in a clustering of point-of-departures around 1 mg kg−1 day−1, and ranging from 0.024 mg kg−1 day−1 for reduced anti-Müllerian hormone concentrations56 to 4.98 mg kg−1 day−1 for reduced liver condition.50,57 Based on the lowest identified threshold (i.e. 0.024 mg kg−1 day−1 for lower anti-Müllerian hormone concentrations from exposure to 0.5 μm polystyrene spheres), a mass-based screening level applicable to 0.50 μm polystyrene spheres in drinking water of 90 μg L−1 was derived using default risk assessment assumptions and practices (20% relative source contribution, composite uncertainty factor of 300, upper 95% percentile 70 years lifetime weighted average drinking water intake rate of 0.053 L kg−1 day−1).50 Extrapolation of this monodisperse screening level to a polydisperse mixture of MPs found in environmental settings comes with extreme uncertainties due to the lack of mammalian toxicity data across multiple polymer types and shapes.50
Significant uncertainties remain in assessing risks to humans through drinking water. The expert workshop deemed that the screening level they derived would be inappropriate for regulatory use due to the lack of established mechanisms of toxicity – in part due to inadequate particle characterization in many of the toxicity studies and uncertainties regarding internal exposure concentrations in test animals.50 Accordingly, in lieu of issuing a regulatory limit for MPs in drinking water, California will require water systems with positive detections to provide qualitative health guidance language to consumers that includes findings from the health effects workshop.24 To reduce uncertainties in future risk assessments, research is needed for quantitative particokinetics in mammals, adverse outcome pathways, in vitro to in vivo quantitative extrapolation, and a reliable toxicologically relevant metric (e.g., surface area) for predicting effects across a diversity of MP particle types.32,50
Charting the path forward to regulatory emergence
While scientific publications on MPs have increased exponentially in recent years58 – even being recognized as the hottest trending contaminant in Environmental Science & Technology in 201939 – the same year that it surpassed per- and polyfluoralkyl substances in total publications,44 the transformation of information and data into knowledge has failed to progress at a proportional rate. Indeed, misconceptions and misunderstandings of what's known and unknown scientifically about MPs continue to pervade both lay-persons and topic-experiences practitioners – particularly regarding risks to biota.5,6 In addition to inherent challenges associated with the complexity and ‘wickedness'of MPs described earlier in this article and elsewhere,5,6 widespread inaccuracies both in the media and even peer-reviewed publications,59 and abysmal sharing of data in published articles,60 two significant barriers to progress are resource constraints and disorganization within the scientific community.
Resource constraints have long restricted progress in MPs research and management. One of the most resource-intensive components is environmental monitoring, as spectroscopic-based techniques require considerably longer analysis times than for most contaminants and are relatively expensive (Table 1). While detailed particle-level information provided by spectroscopic techniques is essential to assessing risks (e.g., size, shape, and polymer distributions),8 it is not necessary to obtain such high-resolution information in all situations. Instead, a diversity of analytical methods will be needed in different locations and purposes (Table 2). For example, manta trawl nets could be used to collect MPs in the marine environment and be analysed with an inexpensive method (e.g., nile red fluorescence;61) to determine spatial and temporal trends in contamination, while a suite of sophisticated forensic monitoring tools could later be used on MPs from a highly polluted area to determine the manufacturer of those plastics and pursue accountability or even litigation (Table 2).
Table 2 General summary of a diversity of sampling and analysis tools for MPs that may be used depending on the intended management and scientific goals within a particular region and jurisdiction
Category |
Driver |
Matrix/location |
Utility |
Technical requirements |
Risk/exposure characterization |
Environmental health |
Oceans, estuaries, etc. |
Compare thresholds to monitoring data |
Low to high |
Human health |
Drinking water, food, etc. |
Characterize exposure |
High |
Environmental & human health |
All matrices |
Meta-analysis |
Very high |
Sources & pathways characterization |
Reduce inputs |
Atmospheric, stormwater, wastewater, etc. |
Estimate flux and removal efficiencies |
Medium to high |
Reduce inputs |
Atmospheric, stormwater, wastewater, etc. |
Pathway attribution |
Medium to high |
Reduce input, polluter accountability |
High risk/exposure areas |
Fingerprinting to determine sources |
Extreme |
Similar to how semi- and non-targeted methods are now being used as initial screening methods for per- and polyfluoroalkyl substances (e.g., total organic fluorine, total oxidizable precursors, etc.),62,63 a tiered monitoring strategy for MPs could be developed and implemented to optimize resource usage by regulatory authorities (Fig. 2). For example, a “tier 1” screening method (e.g., rapid particle counting using nile red dye;61) could be used to estimate potential MPs concentrations and determine if more resource-intensive analytical methods should be applied (Fig. 2). A potential “tier 2” method may involve bulk quantification of MPs by mass (e.g., pyrolysis-gas chromatography/mass-spectrometry),64 which would confirm if suspected particles were indeed MPs (and their mass-based concentrations), but would not capture particle size or shape distributions, and would be limited to polymers for which analytical standards exist (Fig. 2). If mass-based concentrations of MPs are determined to be of regulatory concern, a “tier 3” method could then be used, which would provide necessary information for characterizing risks (Fig. 2).
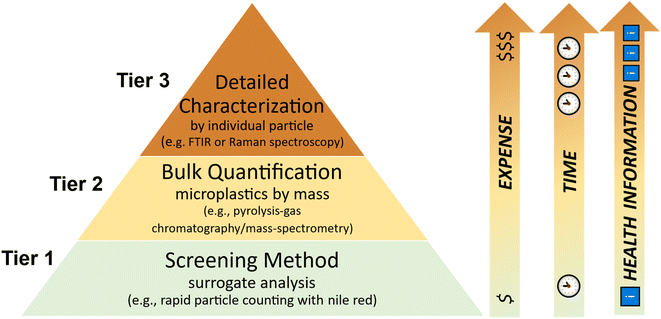 |
| Fig. 2 Proposed tiered monitoring framework for MPs in drinking water or other matrices. Higher tier analytical methods provide additional information regarding health effects but require additional resources (time and money). | |
Availability of a range of tools is essential to reducing resource barriers for MPs research, however such tools must be accessible and affordable to maximize their utility. In the 21st century, an abundance of tools is now available that allow researchers to share and communicate information, data, and evidence rapidly and effectively. Many of these tools have the potential to radically transform the pace at which MPs research evolves-leading to policies and regulations that could significantly reduce the global “plastic toxicity debt” (increasing MPs concentrations caused by unrecoverable degrading environmental macroplastics) that future generations will inevitably inherit.65 For example, many reference databases for spectroscopic analysis are available but are often proprietary, cost-prohibitive, and lack spectra for environmentally-relevant MPs. The recent development of free open databases and software such as Open Specy significantly reduces monitoring costs while increasing transparency, accuracy, and reproducibility of results.66 Other open-source tools have been recently developed to estimate the flow and accumulation of MPs through environments (e.g., “The Full Multi”;67) and humans (e.g., “HEASI”;68) thereby allowing more rapid and transparent understandings of the contaminant suite and prioritization of monitoring efforts. Additional solutions with potentially high impact to the field include harmonization (e.g., language and definitions, analytical and toxicity testing methods, standardized and reference particle mixtures, reporting standards, etc.); effective global and local communication between researchers and decision-makers (e.g., accurate media reporting, peer-to-peer networks, open-access publications); and living evidence synthesis tools (e.g., centralized databases; open-source code repositories; systematic critical reviews).
Significant progress has already been made on the harmonization front, especially with regards to definitions,17 analytical methods,21 reporting,48,49 and hazard study design criteria,30,69 however many additional challenges remain – particularly in developing standardized particle mixtures for hazard testing and monitoring,70 and reporting physical traits of MPs in monitoring studies. The recent development of a living toxicity database for MPs (i.e., ToMEx) is catalysing the formation of higher quality studies designed specifically to fit knowledge gaps and providing a common repository to share published data.31 Further, a recent ambitious effort has been made to centralize all tools, databases, and evidence for MPs (i.e., https://www.plastiverse.org/) according to recommendations by the Global Commission on Evidence to Address Societal Challenges.71 These efforts to widely share evidence could be hindered by current policies employed by traditional funding agencies and grants that often lack requirements for researchers to share science and data openly.60 Finally, such radical sharing efforts may become more sustainable, fair, inter/multi/trans-disciplinary, and well-funded through adoption of novel “decentralized science” methods that leverage cryptoeconomic mechanics (e.g., tokenization, consensus, crowdsourcing, smart contracts, reputation systems) to coordinate scientific collaborations across large scales and complicated networks72 – a method that has recently shown promise in mitigating other global challenges such as climate change.73
Conclusion
As the public becomes increasingly more aware of and concerned about their possible exposure to MPs (recent examples include human blood, breast milk, placenta, and lungs)74–77 and risks to humans and ecosystems,78 governments must respond in a satisfactory and rapid manner to maintain public trust while allocating limited financial and institutional resources wisely to manage other legacy and emerging contaminants effectively. Tiered approaches utilizing a suite of analytical methods in fit-for-purpose applications can maximize data utility while minimizing costs (Fig. 2). As demonstrated in a recent risk probabilistic risk characterization in San Francisco Bay,9 a combination of complimentary sampling techniques and optimized analytical methods are necessary to produce reliable and representative exposure data. However, development of environmental regulations (e.g., total maximum daily loads, maximum contaminant levels) of MPs will require additional advances and harmonization of analytical techniques such that uncertainties of risk characterizations are comparable to other regulated contaminants.
As government agencies tackle MPs, they should leverage and promote recently developed fundamental tools and methods to monitor contamination and assess risks of MPs to humans and environments. Notably, open-source methods to document and model exposure and hazards of MPs as a multidimensional contaminant suite (e.g., Open Specy, The Full Multi, HEASI, ToMEx)31,66–68 enable higher resolution risk characterizations-which are paramount to applying regulatory frameworks to control MPs. Finally, to achieve full emergence of MPs as a regulatory contaminant class while making sensible use of resources, government agencies and scientists alike must be adaptive and innovative in their approaches through the use of open-source technologies, tiered and objective-driven monitoring strategies, and open collaboration and sharing.
Data availability
All data generated and analyzed as part of this study are included in this published article and at https://sccwrp.shinyapps.io/aq_mp_tox_shiny/.
Author contributions
S. C. wrote the main manuscript text, conceptualized, developed and performed the methodology, conducted the formal analysis, curated the data, produced visualizations, and administered the project.
Disclaimer
The views and opinions expressed in this article are those of the author and do not necessarily reflect the official policy or position of any California State agency.
Abbreviations
MPs | Microplastic particles |
FTIR | Fourier transform infrared spectroscopy |
ToMEx | Toxicity of Microplastics Explorer |
Conflicts of interest
The following authors declare that they have no known competing financial interests or personal relationships that could have appeared to influence the work reported in this paper: Scott Coffin.
Acknowledgements
Funding for this work is from the California State Water Resources Control Board. The author expresses gratitude to his innumerable formal and informal collaborators, colleagues, and friends within the plastic pollution researcher community that freely and openly offer resources and help to one another and serve as a constant source of inspiration. The author also would like to thank the California State Water Resources Control Board for providing funding to write this manuscript.
References
- E. J. Carpenter and K. L. Smith, Plastics on the Sargasso Sea Surface, Science, 1972, 175(4027), 1240–1241 CrossRef CAS PubMed.
- E. Sanders and C. T. Ashworth, A study of particulate intestinal absorption and hepatocellular uptake, Exp. Cell Res., 1961, 22, 137–145 CrossRef CAS PubMed.
- D. M. Mitrano and W. Wohlleben, Microplastic regulation should be more precise to incentivize both innovation and environmental safety, Nat. Commun., 2020, 11(1), 5324 CrossRef CAS PubMed.
- C. W. Churchman, Guest editorial: Wicked problems, Manage. Sci., 1967, B141–B142 Search PubMed.
- A. A. Koelmans, E. Besseling, E. Foekema, M. Kooi, S. Mintenig and B. C. Ossendorp,
et al., Risks of Plastic Debris: Unravelling Fact, Opinion, Perception, and Belief, Environ. Sci. Technol., 2017, 51(20), 11513–11519 CrossRef CAS PubMed.
-
M. Wagner, Solutions to Plastic Pollution: A Conceptual Framework to Tackle a Wicked Problem, in Microplastic in the Environment: Pattern and Process, Springer, Cham, 2022, pp. 333–352 Search PubMed.
- S. Coffin, H. Wyer and J. C. Leapman, Addressing the environmental and health impacts of microplastics requires open collaboration between diverse sectors, PLoS Biol., 2021, 19(3), e3000932 CrossRef CAS PubMed.
- A. A. Koelmans, P. E. Redondo-Hasselerharm, N. H. M. Nor, V. N. de Ruijter, S. M. Mintenig and M. Kooi, Risk assessment of microplastic particles, Nat. Rev. Mater., 2022, 138–152 CrossRef , available from https://www.nature.com/articles/s41578-021-00411-y.
- S. Coffin, S. B. Weisberg, C. Rochman, M. Kooi and A. A. Koelmans, Risk characterization of microplastics in San Francisco Bay, California, Microplast. Nanoplast., 2022, 2(1), 19 CrossRef.
- A. C. Mehinto, S. Coffin, A. A. Koelmans, S. M. Brander, M. Wagner and L. M. Thornton Hampton,
et al., Risk-based management framework for microplastics in aquatic ecosystems, Microplast. Nanoplast., 2022, 2(1), 17 CrossRef.
- T. M. Karlsson, L. Arneborg, G. Broström, B. C. Almroth, L. Gipperth and M. Hassellöv, The unaccountability case of plastic pellet pollution, Mar. Pollut. Bull., 2018, 129(1), 52–60 CrossRef CAS PubMed.
-
A. Portantino, SB 1263: Microplastics Materials, 2018, available from https://leginfo.legislature.ca.gov/faces/billTextClient.xhtml?bill_id=201720180SB1263 Search PubMed.
-
A. Portantino, SB-1422 California Safe Drinking Water Act: microplastics, 2018, available from https://leginfo.legislature.ca.gov/faces/billTextClient.xhtml?bill_id=201720180SB1422 Search PubMed.
- S. Coffin and S. B. Weisberg, Understanding health effects pathways and thresholds: filling a critical need to support microplastics management, Microplast. Nanoplast., 2022, 2(1), 11 CrossRef.
-
GESAMP, IMO/FAO/UNESCO-IOC/UNIDO/WMO/IAEA/UN/UNEP/UNDP/ISA Joint Group of Experts on the Scientific Aspects of Marine Environmental Protection, Guidelines or the monitoring and assessment of plastic litter and microplastics in the ocean, ed. P. J. Kershaw, A. Turra and F. Galgani, United Nations Environment Programme (UNEP)http://www.gesamp.org/site/assets/files/2002/rs99e.pdf, 2019, p. 130, Report No.: 99, available from Search PubMed.
- N. B. Hartmann, T. Hüffer, R. C. Thompson, M. Hassellöv, A. Verschoor and A. E. Daugaard,
et al., Are We Speaking the Same Language? Recommendations for a Definition and Categorization Framework for Plastic Debris, Environ. Sci. Technol., 2019, 53(3), 1039–1047 CrossRef CAS PubMed.
-
State Water Resources Control Board, Resolution No. 2020-0021, Adoption of Definition of “Microplastics in Drinking Water”, 2020, available from https://www.waterboards.ca.gov/board_decisions/adopted_orders/resolutions/2020/rs2020_0021.pdf Search PubMed.
-
S. Coffin, Staff Report for the Proposed Definition of Microplastics in Drinking Water, State Water Resources Control Board, Sacramento, CA, 2020, available from https://www.waterboards.ca.gov/drinking_water/certlic/drinkingwater/documents/microplastics/stffrpt_def_mcrplstcs.pdf Search PubMed.
-
European Commission, COMMISSION REGULATION (EU) …/… of XXX amending Annex XVII to Regulation (EC) No 1907/2006 of the European Parliament and of the Council concerning the Registration,
Evaluation, Authorisation and Restriction of Chemicals (REACH) as regards synthetic polymer microparticles, 2022, available from, https://ec.europa.eu/transparency/comitology-register/screen/documents/083921/1/consult?lang=en Search PubMed.
- S. Primpke, S. H. Christiansen, W. Cowger, H. De Frond, A. Deshpande and M. Fischer,
et al., Critical Assessment of Analytical Methods for the Harmonized and Cost Efficient Analysis of Microplastics, Appl. Spectrosc., 2020, 1012–1047 CrossRef CAS PubMed.
- H. De Frond, L. T. Hampton, S. Kotar, K. Gesulga, C. Matuch and W. Lao,
et al., Monitoring microplastics in drinking water: An interlaboratory study to inform effective methods for quantifying and characterizing microplastics, Chemosphere, 2022, 134282 CrossRef CAS PubMed.
-
C. Wong and S. Coffin, SWB-MP2-rev1, Standard Operating Procedures for Extraction and Measurement by Raman Spectroscopy of Microplastic Particles in Drinking Water, 2022, available from https://www.waterboards.ca.gov/drinking_water/certlic/drinkingwater/documents/microplastics/swb-mp2-rev1.pdf Search PubMed.
-
C. Wong and S. Coffin, SWB-MP1-rev1, Standard Operating Procedures for Extraction and Measurement by Infrared Spectroscopy of Microplastic Particles in Drinking Water, 2022, available from https://www.waterboards.ca.gov/drinking_water/certlic/drinkingwater/documents/microplastics/swb-mp1-rev1.pdf Search PubMed.
-
State Water Resources Control Board, Policy Handbook Establishing a Standard Method of Testing and Reporting of Microplastics in Drinking Water, 2022, available from https://www.waterboards.ca.gov/drinking_water/certlic/drinkingwater/docs/2022/mp-hndbk.pdf Search PubMed.
- A. A. Koelmans, P. E. Redondo Hasselerharm, N. H. Mohamed Nor and M. Kooi, Solving the non-alignment of methods and approaches used in microplastic research in order to consistently characterize risk, Environ. Sci. Technol., 2020, 12307–12315 CrossRef CAS PubMed.
- E. E. Burns and A. B. A. Boxall, Microplastics in the aquatic environment: Evidence for or against adverse impacts and major knowledge gaps: Microplastics in the environment, Environ. Toxicol. Chem., 2018, 37(11), 2776–2796 CrossRef CAS PubMed.
- E. Besseling, P. Redondo-Hasselerharm, E. M. Foekema and A. A. Koelmans, Quantifying ecological risks of aquatic micro- and nanoplastic, Crit. Rev. Environ. Sci. Technol., 2019, 49(1), 32–80 CrossRef.
- M. Kooi, S. Primpke, S. M. Mintenig, C. Lorenz and G. Gerdts, Characterizing the multidimensionality of microplastics across environmental compartments, Water Res., 2021, 24 Search PubMed.
- M. Kooi and A. A. Koelmans, Simplifying Microplastic via Continuous Probability Distributions for Size, Shape, and Density, Environ. Sci. Technol. Lett., 2019, 6(9), 551–557 CrossRef CAS.
- V. N. de Ruijter, P. E. Redondo Hasselerharm, T. Gouin and A. A. Koelmans, Quality criteria for microplastic effect studies in the context of risk assessment: A critical review, Environ. Sci. Technol., 2020, 54(09), 11692–11705 CrossRef CAS PubMed.
- L. M. Thornton Hampton, H. Lowman, S. Coffin, E. Darin, H. De Frond and L. Hermabessiere,
et al., A living tool for the continued exploration of microplastic toxicity, Microplast. Nanoplast., 2022, 2(1), 13 CrossRef.
- L. M. Thornton Hampton, S. M. Brander, S. Coffin, M. Cole, L. Hermabessiere and A. A. Koelmans,
et al., Characterizing microplastic hazards: which concentration metrics and particle characteristics are most informative for understanding toxicity in aquatic organisms?, Microplast. Nanoplast., 2022, 2(1), 20 CrossRef.
- S. Belanger, M. Barron, P. Craig, S. Dyer, M. Galay-Burgos and M. Hamer,
et al., Future needs and recommendations in the development of species sensitivity distributions: Estimating toxicity thresholds for aquatic ecological communities and assessing impacts of chemical exposures: Advancing Species Sensitivity Distributions in Ecotoxicology, Integr. Environ. Assess. Manage., 2017, 13(4), 664–674 CrossRef PubMed.
- S. N. Athey and L. M. Erdle, Are We Underestimating Anthropogenic Microfiber Pollution? A Critical Review of Occurrence, Methods, and Reporting, Environ. Toxicol. Chem., 2021, etc.5173 Search PubMed.
- R. Qiao, Y. Deng, S. Zhang, M. B. Wolosker, Q. Zhu and H. Ren,
et al., Accumulation of different shapes of microplastics initiates intestinal injury
and gut microbiota dysbiosis in the gut of zebrafish, Chemosphere, 2019, 236, 124334 CrossRef CAS PubMed.
- C. D. Stienbarger, J. Joseph, S. N. Athey, B. Monteleone, A. L. Andrady and W. O. Watanabe,
et al., Direct ingestion, trophic transfer, and physiological effects of microplastics in the early life stages of Centropristis striata, a commercially and recreationally valuable fishery species, Environ. Pollut., 2021, 285, 117653 CrossRef CAS PubMed.
- L. M. Thornton Hampton, H. Bouwmeester, S. M. Brander, S. Coffin, M. Cole and L. Hermabessiere,
et al., Research recommendations to better understand the potential health impacts of microplastics to humans and aquatic ecosystems, Microplast. Nanoplast., 2022, 2(1), 18 CrossRef.
- X. Zhu, K. Munno, J. Grbic, L. M. Werbowski, J. Bikker and A. Ho,
et al., Holistic Assessment of Microplastics and Other Anthropogenic Microdebris in an Urban Bay Sheds Light on Their Sources and Fate, ACS EST Water, 2021, 1401–1410 CrossRef CAS.
- J. J. Zhu, W. Dressel, K. Pacion and Z. J. Ren,
ES&T in the 21st Century: A Data-Driven Analysis of Research Topics, Interconnections, And Trends in the Past 20 Years, Environ. Sci. Technol., 2021, 55(6), 3453–3464 CrossRef CAS PubMed.
- A. A. Koelmans, N. H. Mohamed Nor, E. Hermsen, M. Kooi, S. M. Mintenig and J. De France, Microplastics in freshwaters and drinking water: Critical review and assessment of data quality, Water Res., 2019, 155, 410–422 CrossRef CAS PubMed.
- C. Hung, N. Klasios, X. Zhu, M. Sedlak, R. Sutton and C. M. Rochman, Methods Matter: Methods for Sampling Microplastic and Other Anthropogenic Particles and Their Implications for Monitoring and Ecological Risk Assessment, Integr. Environ. Assess. Manage., 2021, 17(1), 282–291 CrossRef CAS PubMed.
- P. E. Redondo-Hasselerharm, A. Rico and A. A. Koelmans, Risk assessment of microplastics in freshwater sediments guided by strict quality criteria and data alignment methods, J. Hazard. Mater., 2022, 129814 Search PubMed.
- V. Adam, A. von Wyl and B. Nowack, Probabilistic environmental risk assessment of microplastics in marine habitats, Aquat. Toxicol., 2021, 230, 105689 CrossRef CAS PubMed.
- R. Bakhshoodeh and R. M. Santos, Comparative bibliometric trends of microplastics and perfluoroalkyl and polyfluoroalkyl substances: how these hot environmental remediation research topics developed over time, RSC Adv., 2022, 12(8), 4973–4987 RSC.
- T. M. Karlsson, A. Kärrman, A. Rotander and M. Hassellöv, Comparison between manta trawl and in situ pump filtration methods, and guidance for visual identification of microplastics in surface waters, Environ. Sci. Pollut. Res., 2020, 27(5), 5559–5571 CrossRef PubMed.
- J. Brandt, F. Fischer, E. Kanaki, K. Enders, M. Labrenz and D. Fischer, Assessment of Subsampling Strategies in Microspectroscopy of Environmental Microplastic Samples, Front. Environ. Sci., 2021, 8, 579676 CrossRef.
- E. Miller, M. Sedlak, D. Lin, C. Box, C. Holleman and C. M. Rochman,
et al., Recommended best practices for collecting, analyzing, and reporting microplastics in environmental media: Lessons learned from comprehensive monitoring of San Francisco Bay, J. Hazard. Mater., 2021, 409, 124770 CrossRef CAS PubMed.
- S. M. Brander, V. C. Renick, M. M. Foley, C. Steele, M. Woo and A. Lusher,
et al., Sampling and QA/QC: A guide for scientists investigating the occurrence of microplastics across matrices, Appl. Spectrosc., 2020, 52 Search PubMed.
- W. Cowger, A. Booth, B. Hamilton, S. Primpke, K. Munno and A. Lusher,
et al., EXPRESS: Reporting Guidelines to Increase the Reproducibility and Comparability of Research on Microplastics, Appl. Spectrosc., 2020, 000370282093029 Search PubMed.
- S. Coffin, H. Bouwmeester, S. Brander, P. Damdimopoulou, T. Gouin and L. Hermabessiere,
et al., Development and application of a health-based framework for informing regulatory action in relation to exposure of microplastic particles in California drinking water, Microplast. Nanoplast., 2022, 2(1), 12 CrossRef PubMed.
- B. Hou, F. Wang, T. Liu and Z. Wang, Reproductive toxicity of polystyrene microplastics: In vivo experimental study on testicular toxicity in mice, J. Hazard. Mater., 2021, 405, 124028 CrossRef CAS PubMed.
- X. Xie, T. Deng, J. Duan, J. Xie, J. Yuan and M. Chen, Exposure to polystyrene microplastics causes reproductive toxicity through oxidative stress and activation of the p38 MAPK signaling pathway, Ecotoxicol. Environ. Saf., 2020, 190, 110133 CrossRef CAS PubMed.
- S. Li, Q. Wang, H. Yu, L. Yang, Y. Sun and N. Xu,
et al., Polystyrene microplastics induce blood–testis barrier disruption regulated by the MAPK-Nrf2 signaling pathway in rats, Environ. Sci. Pollut. Res., 2021, 47921–47931 CrossRef CAS PubMed , available from https://link.springer.com/10.1007/s11356-021-13911-9.
- F. Amereh, M. Babaei, A. Eslami, S. Fazelipour and M. Rafiee, The emerging risk of exposure to nano(micro)plastics on endocrine disturbance and reproductive toxicity: From a hypothetical scenario to a global public health challenge, Environ. Pollut., 2020, 261, 114158 CrossRef CAS PubMed.
- R. An, X. Wang, L. Yang, J. Zhang, N. Wang and F. Xu,
et al., Polystyrene microplastics cause granulosa cells apoptosis and fibrosis in ovary through oxidative stress in rats, Toxicology, 2021, 449, 152665 CrossRef CAS PubMed.
- J. Hou, Z. Lei, L. Cui, Y. Hou, L. Yang and R. An,
et al., Polystyrene microplastics lead to pyroptosis and apoptosis of ovarian granulosa cells via NLRP3/Caspase-1 signaling pathway in rats, Ecotoxicol. Environ. Saf., 2021, 212, 112012 CrossRef CAS PubMed.
- Y. Deng, Y. Zhang, B. Lemos and H. Ren, Tissue accumulation of microplastics in mice and biomarker responses suggest widespread health risks of exposure, Sci. Rep., 2017, 7(1), 46687 CrossRef PubMed.
- Y. Zhang, S. Pu, X. Lv, Y. Gao and L. Ge, Global trends and prospects in microplastics research: A bibliometric analysis, J. Hazard. Mater., 2020, 400, 123110 CrossRef CAS PubMed.
- C. Völker, J. Kramm and M. Wagner, On the Creation of Risk: Framing of Microplastics Risks in Science and Media, Global Challenges, 2019, 1900010 Search PubMed.
- T. Jenkins, B. D. Persaud, W. Cowger, K. Szigeti, D. G. Roche and E. Clary,
et al., Current State of Microplastic Pollution Research Data: Trends in Availability and Sources of Open Data, Front. Environ. Sci., 2022, 10, 912107 CrossRef.
- T. Maes, R. Jessop, N. Wellner, K. Haupt and A. G. Mayes, A rapid-screening approach to detect and quantify microplastics based on fluorescent tagging with Nile Red, Sci. Rep., 2017, 7(1), 44501 CrossRef CAS PubMed.
- E. F. Houtz and D. L. Sedlak, Oxidative conversion as a means of detecting precursors to perfluoroalkyl acids in urban runoff, Environ. Sci. Technol., 2012, 46(17), 9342–9349 CrossRef CAS PubMed.
- L. Schultes, G. F. Peaslee, J. D. Brockman, A. Majumdar, S. R. McGuinness and J. T. Wilkinson,
et al., Total fluorine measurements in food packaging: how do current methods perform?, Environ. Sci. Technol. Lett., 2019, 6(2), 73–78 CrossRef CAS.
- E. Fries, J. H. Dekiff, J. Willmeyer, M. T. Nuelle, M. Ebert and D. Remy, Identification of polymer types and additives in marine microplastic particles using pyrolysis-GC/MS and scanning electron microscopy, Environ. Sci.: Processes Impacts, 2013, 15(10), 1949 RSC.
- M. C. Rillig, E. Leifheit and J. Lehmann, Microplastic effects on carbon cycling processes in soils, PLoS Biol., 2021, 19(3), e3001130 CrossRef CAS PubMed.
- W. Cowger, Z. Steinmetz, A. Gray, K. Munno, J. Lynch and H. Hapich,
et al., Microplastic Spectral Classification Needs an Open Source Community: Open Specy to the Rescue!, Anal Chem, 2021, 7543–7548 CrossRef CAS PubMed.
- P. Domercq, A. Praetorius and M. MacLeod, The Full Multi: An open-source framework for modelling the transport and fate of nano- and microplastics in aquatic systems, Environ. Model. Softw., 2022, 148, 105291 CrossRef.
- N. H. Mohamed Nor, M. Kooi, N. J. Diepens and A. A. Koelmans, Lifetime Accumulation of Microplastic in Children and Adults, Environ. Sci., 2021, 55(8), 5084–5096 CrossRef CAS PubMed.
- T. Gouin, R. Ellis-Hutchings, L. M. Thornton Hampton, C. L. Lemieux and S. L. Wright, Screening and prioritization of nano- and microplastic particle toxicity studies for evaluating human health risks – development and application of a toxicity study assessment tool, Microplast. Nanoplast., 2022, 2(1), 2 CrossRef PubMed.
- J. Seghers, E. A. Stefaniak, R. La Spina, C. Cella, D. Mehn and D. Gilliland,
et al., Preparation of a reference material for microplastics in water—evaluation of homogeneity, Anal. Bioanal. Chem., 2021, 385–397 Search PubMed , available from http://link.springer.com/10.1007/s00216-021-03198-7.
-
Global Commission on Evidence to Address Societal Challenges, The Evidence Commission report: A wake-up call and path forward for decisionmakers, evidence intermediaries, and impact-oriented evidence producers, McMaster Health Forum, Hamilton, 2022, available from https://www.mcmasterforum.org/networks/evidence-commission/report/english Search PubMed.
-
J. Ducrée, M. Codyre, R. Walshe and S. Barting, DeSci-Decentralized Science, 2022 Search PubMed.
- A. Sipthorpe, S. Brink, T. Van Leeuwen and I. Staffell, Blockchain solutions for carbon markets are nearing maturity, One Earth, 2022, 5(7), 779–791 CrossRef.
- H. A. Leslie, J. M. van Velzen M, S. H. Brandsma, D. Vethaak, J. J. Garcia-Vallejo and M. H. Lamoree, Discovery and quantification of plastic particle pollution in human blood, Environ. Int., 2022, 107199 CrossRef CAS PubMed.
- L. C. Jenner, J. M. Rotchell, R. T. Bennett, M. Cowen, V. Tentzeris and L. R. Sadofsky, Detection of microplastics in human lung tissue using μFTIR spectroscopy, Sci. Total Environ., 2022, 831, 154907 CrossRef CAS PubMed.
- A. Ragusa, A. Svelato, C. Santacroce, P. Catalano, V. Notarstefano and O. Carnevali,
et al., Plasticenta: First evidence of microplastics in human placenta, Environ. Int., 2021, 146, 106274 CrossRef CAS PubMed.
- A. Ragusa, V. Notarstefano, A. Svelato, A. Belloni, G. Gioacchini and C. Blondeel,
et al., Raman Microspectroscopy Detection and Characterisation of Microplastics in Human Breastmilk, Polymers, 2022, 14(13), 2700 CrossRef CAS PubMed.
- A. I. Catarino, J. Kramm, C. Völker, T. B. Henry and G. Everaert, Risk posed by microplastics: Scientific evidence and public perception, Curr. Opin. Green Sustainable Chem., 2021, 29, 100467 CrossRef CAS.
|
This journal is © The Royal Society of Chemistry 2023 |