DOI:
10.1039/D3SC01857A
(Perspective)
Chem. Sci., 2023,
14, 7611-7619
Nanoelectrochemistry in electrochemical phase transition reactions
Received
9th April 2023
, Accepted 21st June 2023
First published on 22nd June 2023
Abstract
Electrochemical phase transition is important in a range of processes, including gas generation in fuel cells and electrolyzers, as well as in electrodeposition in battery and metal production. Nucleation is the first step in these phase transition reactions. A deep understanding of the kinetics, and mechanism of the nucleation and the structure of the nuclei and nucleation sites is fundamentally important. In this perspective, theories and methods for studying electrochemical nucleation are briefly reviewed, with an emphasis on nanoelectrochemistry and single-entity electrochemistry approaches. Perspectives on open questions and potential future approaches are also discussed.
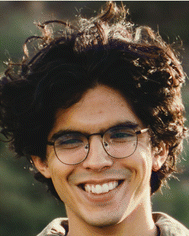 Elías Mondaca-Medina | Elias Mondaca received his B.A. in Chemistry from Colorado College in 2021, where he worked on developing electrochemical sensors for water quality diagnostics. He is now pursuing his PhD in Analytical Chemistry from the University of Texas at Austin, currently investigating electrochemical phase transitions on carbon surfaces. |
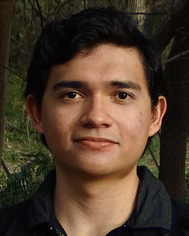 Roberto García Carrillo | Roberto received his B.S. in Chemistry and Nanotechnology from Tecnológico de Monterrey, Mexico, in 2021, working on simulations of galvanostatic deposition of metallic nanoparticles. He then completed his M.S. in Nanotechnology in the same institution working in electrochemical single-entity characterization of bimetallic nanoparticles in 2023. He is currently a first-year Chemistry PhD student at the University of Texas at Austin. |
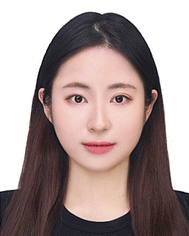 Hyein Lee | Hyein Lee is a PhD student in chemistry at the University of Texas at Austin. She received her B.S/M.S. in Chemistry from Kyung Hee University, South Korea in 2017/2022. She is currently investigating dynamic electrocatalysis to control the selectivity and the activity of energy conversion reactions. |
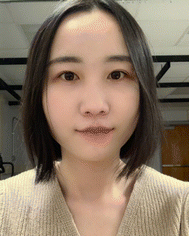 Yufei Wang | Yufei received her B.S. from Hebei University, where she explored chiral separation in Pharmacy. She is pursuing her doctoral degree at The University of Texas at Austin, working on studying the electrochemical activity of metal dissolution at the nanoscale. |
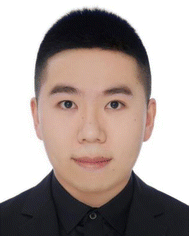 He Zhang | He Zhang received his PhD from Changchun Institute of Applied Chemistry, Chinese Academy of Sciences & University of Science and Technology of China (2020). He then took on a research assistantship at the same institute, working on bioelectrocatalysis and bio-photoelectrocatalysis. In 2023, he joined the Ren group where he focuses on the research of single-entity electrochemistry. |
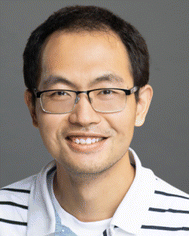 Hang Ren | Hang Ren is currently an assistant professor in the Department of Chemistry at the University of Texas at Austin. He received B.S. in Chemistry from Sun Yat-Sen University and PhD in Analytical Chemistry under Mark E. Meyerhoff from the University of Michigan. He was a postdoc associate at the University of Utah with Henry S. White. His current research interests include revealing the heterogeneity and dynamics of complex electrochemical interfaces via nanoelectrochemistry and scanning electrochemical probe microscopy, with applications in corrosion, energy storage, and conversion. He has received several awards, including ACS PRF New Doctoral Award, DARPA Young Investigator Award, NIH Maximizing Investigators' Research Award, DARPA Director's Award, NSF CAREER Award, Scialog Fellow, and Sloan Research Fellowship. |
1. Introduction
Phase transition occurs in various electrochemical reactions that are technologically and industrially important. For example, gas bubbles can form at the electrode surface during gas evolution reactions when the gas species are locally supersaturated near the electrode. Some of the important reactions include the chlorine evolution reaction (CER) in the chloralkaline process, as well as the hydrogen evolution reaction (HER) and oxygen evolution reaction (OER) in water electrolysis. The bubbles can block active sites on the electrode, creating additional overpotential and energy loss in the electrolysis. On the other hand, the detachment and motion of bubbles can create convection and enhance mass transfer in electrocatalysis. The evolution of bubbles in electrochemical reactions has been reviewed recently.1,2 Electrochemical formation of a new solid phase, often known as electro-crystallization when the solid is crystalline, is also fundamentally important in aluminum production, electroplating, as well as in batteries based on metal anodes.3–6
Electrochemical phase transition often needs nucleation to be the first step. Nucleation describes the clustering of ions, atoms, or molecules via fluctuation to form an assembly of a critical size before the further growth of the new phase is energetically favorable. Therefore, the kinetics and mechanisms of nucleation at electrochemical interfaces can greatly impact many electrochemical processes involving phase transition. For example, designing an electrochemical interface that induces smooth nucleation and growth is key to developing safer batteries.7,8 Tuning the nucleation behavior to better manage bubbles in electrocatalytic gas evolution reactions can increase the efficiency of electrolyzers.9
In this perspective, we focus on the nucleation process in the electrochemical phase transition. The readers are referred to other relevant papers for other related processes, e.g., growth, aggregation, and Ostwald ripening.10–15 We will first introduce the theories and models in electrochemical nucleation. Some experimental methods to study the nucleation of solids and gas bubbles at electrochemical interfaces will then be highlighted, with an emphasis on nanoscale electrochemical methods. Finally, open questions and perspectives on future directions in studying electrochemical nucleation will be provided.
2. Nucleation theories in the electrochemical phase transition
Several general nucleation theories have been developed, which can be used to understand and analyze the experimental results in electrochemical nucleation to reveal mechanistic insight. Here, we will primarily focus on classical nucleation theory, which forms the basis for many other theories. The atomistic nucleation model and non-classical nucleation model will also be discussed.
2.1 Classical nucleation theory (CNT)
First developed by Volmer, Weber,16 and Zeldovich,17 CNT is a continuum theory describing the energetics and kinetics of nucleation, which has been successfully applied in electrochemical phase transition processes. According to CNT, forming a new phase requires overcoming an activation barrier determined by the cluster of a critical size called the critical nucleus. This critical nucleus results from the competition between the volume energy for forming a new phase and the interfacial energy for forming a new surface (Fig. 1a). CNT predicts the rate of nucleation as: | 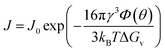 | (1) |
In eqn (1), J is the nucleation rate, J0 is the pre-exponential factor, γ is the interfacial energy of the interface between the electrolyte and the new phase, Φ(θ) is a geometric factor related to the shape of the nucleus, kB is Boltzmann's constant, T is the temperature, and ΔGv is the volume energy for forming the new phase. In electrochemical nucleation, the driving force is controlled electrochemically. For example, in the electrochemically induced bubble nucleation, the supersaturation ratio of gas at the electrode can be controlled by the current of the gas evolution reaction. In electrodeposition, supersaturation is directly controlled by the overpotential. If a 3-dimensional nucleus is assumed in electrodeposition, the nucleation rate can be expressed as | 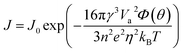 | (2) |
In eqn (2), Va is the atomic volume of the electrodeposited atom, η is the overpotential, n is the number of electrons of the reaction, and e is the elementary charge.12 If 2-dimensional nucleation is operating, the η−2 term in the exponent is replaced with η−1. CNT has been widely used in electrochemical nucleation (vide infra), which successfully explains the kinetics of nucleation as a function of supersaturation ratio, as shown in Ag nucleation and bubble nucleation.18–22 However, because the critical nuclei often contain only a small number of atoms,23,24 whether macroscopic interfacial energy still holds is debatable.
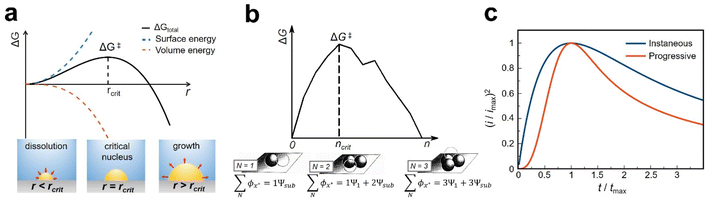 |
| Fig. 1 (a) Free energy in forming a new phase vs. size of the new phase showing a barrier at a critical size (rcrit) predicted by classical nucleation theory (CNT). Adapted with permission from ref. 35. Copyright 2019 American Chemical Society. (b) Free energy in forming clusters of discrete numbers of atoms in the atomistic nucleation model. Adapted with permission from ref. 25 & 26. (c) Current–time traces predicted by the Scharifker–Hills model showing progressive vs. instantaneous nucleation. | |
2.2 Atomistic nucleation model
The atomistic nucleation model, developed by Milchev,25 describes the formation of nuclei as the addition and removal of individual atoms to clusters (Fig. 1b). This model considers the interactions between individual atoms (binding energy), and individual atoms and the substrate without invoking the macroscopic interfacial energy, leading to a discrete character for the critical nucleus size as opposed to classical nucleation theory (CNT).26
2.3 Non-classical nucleation theory
Non-classical nucleation theories have also been proposed to explain some of the observations in phase transitions. Specifically, a two-step nucleation process has been observed in the crystallization of ionic solids: a metastable intermediate phase is first formed, followed by reorganization into an ordered structure.27,28 These observations are inconsistent with CNT, which predicts a single nucleation step without a metastable intermediate. On the other hand, it has been suggested that even when nucleation involves a multi-step mechanism, the fundamental concepts in CNT still hold.29
3. Methods to study electrochemical nucleation
Nucleation at the electrochemical interface can be controlled and measured electrochemically. In electrodeposition, the location of nucleation and the average nucleation rate can be measured by counting the density of the particles formed on the electrode surface after the deposition using SEM or AFM. For example, the nucleation rate during Ag and Pt deposition as a function of potential and their preferential sites on HOPG has been identified using this method.30,31In situ methods for studying nucleation at the electrochemical interfaces, especially liquid-cell TEM, have also provided structural and morphological information during the formation of a new phase in electrodeposition.32–34
3.1 Conventional electrochemical methods
Electrochemical nucleation can perturb the current or potential on the electrode surface, which can be used to probe nucleation. For example, the Scharifker–Hills model allows the measurement of the density of the nucleation site and nucleation rate by analyzing current–time traces at constant potential (Fig. 1c).35 One prediction is the existence of two extreme cases in nucleation: progressive and instantaneous nucleation, which corresponds to slow nucleation and fast nucleation, respectively, and result in different current–time traces, as shown in Fig. 1c. Other variations, including the inclusion of the non-steady state electrochemical processes and dispersion of nucleation rate, were developed by Fleischmann, Fletcher, and others.36–38 While the models can be applied to bulk amperometry experiments, the measured nucleation rate is an average over all the activated nucleation sites because most bulk electrodes will contain a distribution of different nucleation sites. Information about the heterogeneity of the nucleation sites and their effect on the nucleation rate is lost in averaging.
3.2 Measuring one nucleation event at a time
Budevski and coworkers have pioneered the study of single nucleation events in preparing atomically flat Ag surfaces,39 which is now classified as single-entity electrochemistry.40 The complication from multiple competing nucleation events can be mitigated by studying one nucleation event at a time. On these “defect-free” surfaces, nucleation is required before the growth of a new layer is favorable, resulting in the layer-by-layer growth of Ag (Fig. 2a). The induction time for nucleation is used to measure the kinetics of nucleation, which verifies the applicability of CNT in electrodeposition.20 While this pioneering work is elucidating, the method requires the preparation of an atomically flat, “defect-free” electrode surface over hundreds of microns to millimeters, which has been demonstrated successfully on Ag.
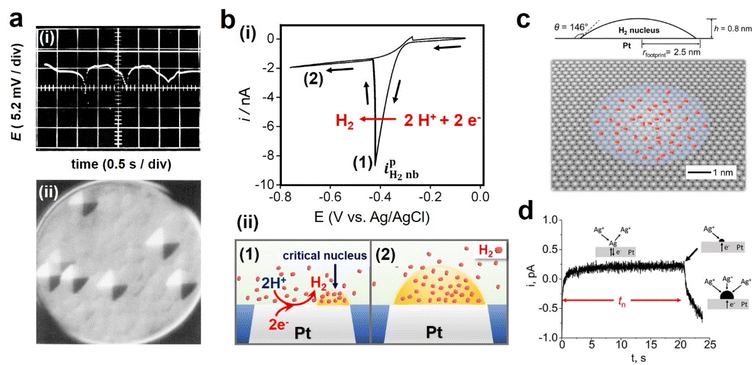 |
| Fig. 2 (a) (i) E–t trace indicating overpotential fluctuation during two-dimensional nucleation of Ag at −65 μA cm−2 on a “defect-free” Ag(100) surface and (ii) growth pyramids on the flat Ag(100) plane. Adapted with permission from ref. 19; (b) the nucleation of H2 nanobubble on a Pt electrode. (i) Cyclic voltammogram, and (ii) schematic illustration of bubble evolution showing (1) critical nucleus and (2) a steady-state H2 bubble formation at a Pt nanoelectrode. Adapted with permission from ref. 35. Copyright 2019 American Chemical Society. (c) Schematic of the cross-section and 3D view of the critical H2 nucleus. Adapted with permission from ref. 35. Copyright 2019 American Chemical Society. (d) i–t curve exhibiting the nucleation and the growth of a single Ag nanoparticle on a Pt nanoelectrode. Reprinted with permission from ref. 46. Copyright 2023 American Chemical Society. | |
3.3 Nanoelectrochemistry and single-entity electrochemistry
Nanoelectrochemistry has greatly expanded the scope of single nucleation measurement. Instead of preparing a defect-free surface, nanoelectrochemistry approaches involve shrinking the electrode to the nanoscale, reducing the number of active nucleation sites to unity. White and coworkers have demonstrated this principle in the nucleation of single nanobubbles, including H2, N2, O2, and CO2.41–44 A typical voltammogram involving the nucleation of a single nanobubble on a nanoelectrode is shown in Fig. 2b: a characteristic peak is observed when a single bubble nucleates and grows at the electrode to block the current. The surface concentration of the dissolved gas molecules and supersaturation ratio can be obtained from this peak current. The supersaturation ratio for bubble nucleation was shown to range from 300 for H2 to 18 in CO2, depending on the gas type, while the number of molecules in the critical nucleus is almost unchanged (50–90) regardless of gas type (Fig. 2c).22,35,44 The same methods can also be applied to study the nucleation of metals, e.g., Hg and Ag, as demonstrated by Mirkin and White, respectively.45,46 Depending on the theoretical framework, the critical nucleus of Ag on carbon was found to be 1 to 9 atoms for CNT and 1 to 5 atoms using the atomistic model in the overpotential range of −130 to −70 mV.46 The nucleation of a single ionic solid particle, like CaCO3, has also been studied on a nanoelectrode.47 Here, supersaturation in terms of the equilibrium of solubility of CaCO3 was induced by electrochemically changing the pH near the electrode. Future work can test the viability of different nucleation theories by evaluating the nucleation rate vs. supersaturation and comparing the results with theoretical predictions.47 Overall, the nanoelectrode method greatly simplifies the interpretation of the nucleation measurements, which provides rich information about nucleation. However, the structure of the nucleation sites on different electrodes is generally difficult to control and characterize. In addition, the electrode materials for making nanodisk electrodes are limited to a few conductors, mainly Pt and Au.
An alternative to the nanoelectrode approach is to use scanning electrochemical probe microscopy methods, especially scanning electrochemical cell microscopy (SECCM). SECCM uses an electrolyte-filled glass capillary with a < 1 μm diameter opening, often called nanopipette, to measure local electrochemistry.48 A combined reference/counter electrode is inserted in the bulk solution side of the nanopipette. The contact between the nanoscopic droplet at the end of the nanopipette and the substrate defines a nanoelectrode as the working electrode, allowing local nanoelectrochemical measurement at different electrode materials. In addition, scanning the nanopipette probe allows electrochemical mapping, and the lateral resolution ranging from a few microns to 30 nm has been reported,49 depending on the size of the nanopipette, the wettability of the substrate, and the mode of scanning (e.g., lateral hopping distance in the hopping scan). Sub-10 nm diameter nanopipettes have been reported, which promises further improvement in the resolution of SECCM.50 We have demonstrated mapping the nucleation of H2 bubbles on polycrystalline Pt using SECCM (Fig. 3a).51 In this study, we found a heterogeneous distribution of energetics of nucleation for H2 bubbles which was not correlated with the crystal facets. The fast gas exchange at the droplet–air interface acts as a sink to remove H2 generated at the electrode surface. As a result, higher currents and higher proton concentrations are needed to induce nucleation compared with those in nanoelectrode experiments. This approach has been applied to study gas bubble nucleation on other substrates and single nanoparticles.52–54 The results also suggest that when inferring the activity of gas evolution reactions from the current, the possibility of bubble formation that lowers the current should be considered.
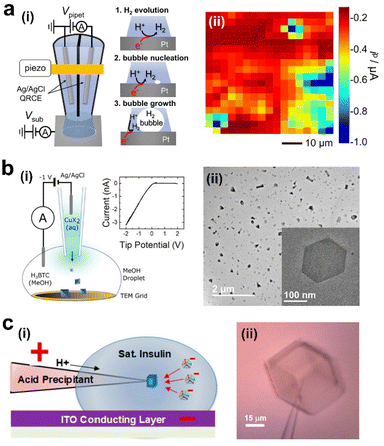 |
| Fig. 3 Nanopipette-based study of nucleation. (a) Left: schematic of mapping the distribution of bubble nucleation on an electrode surface using SECCM. Right: map of nucleation peak current (ip). Adapted with permission from ref. 49. Copyright 2019 American Chemical Society. (b) Controlled synthesis of metal–organic framework crystals using a nanopipette. (i) Schematic and i–V curve. (ii) TEM image. Reprinted with permission from ref. 54. (c) Controlled nucleation and growth of a single insulin crystal at the tip of a nanopipette. Left: schematic. Right: an optical image. Reprinted with permission from ref. 56. Copyright 2019 American Chemical Society. | |
Unwin and coworkers have also used SECCM to show that Ag particle nucleation on basal terraces of HOPG involves nucleation, aggregative growth, and detachment mechanism.55 By comparing macroscale and nanoscale measurements, it was shown that the nucleation site density, calculated using the Scharifker–Hills model, does not agree with the number of particles observed with scanning electron microscopy (SEM) and atomic force microscopy (AFM). By SECCM, however, they demonstrated that the disparity in the number of particles was related to a detachment mechanism. This specific mechanism could only be assessed by analyzing nucleation and growth at the nanoscale.
A related nanopipette technique is to induce and study nucleation by controlling the ionic flux in the nanopipette. Siwy and coworkers have demonstrated the formation of ionic precipitates by biasing a potential across nanopores in dilute ionic solutions.56–59 The precipitates are very unstable and undergo nucleation and re-dissolution, which results in transient blocking and unblocking of the pores that can be observed as oscillations in the ionic current.58 This opens the possibility of using these nanopores as single-molecule sensors.56 Unwin and coworkers have shown the synthesis of metal–organic framework (MOF) crystals and other organic crystals with unusual polymorphs (Fig. 3b).60,61 The ability to apply different voltages across the nanopipette and vary the opening size of the nanopipettes offer possibilities for precise control of crystallization. Wang and coworkers have demonstrated the control of the nucleation and growth of one insulin crystal at the tip of the nanopipette by actively controlling the mass transport within it (Fig. 3c). The simultaneous electrical and optical measurement complements each other in observing the different stages of the crystal nucleation and growth.62
4. Perspectives
Single-entity and nanoelectrochemistry approaches have begun to reveal new information about the kinetics and mechanism of electrochemical nucleation processes. A deeper molecular insight into the nucleation process at the electrode will further positively impact the development of technologically important processes involving phase transition. Below, we summarize our perspectives on electrochemical nucleation studies, including open questions about electrochemical nucleation, and the potential development needed to answer the questions. The open questions include:
(1) What are the molecular and atomic structures of the nucleation sites?
(2) What are the dynamics of the nucleation sites, the nucleus, and the pre-nucleus?
(3) What are the roles of classical nucleation theory vs. non-classical theory in different electrochemical phase transition systems?
(4) How do the interfacial solvent structure and ion distribution affect nucleation?
We believe new analytical methods and measurement tools are needed to address these open questions. It is envisioned that multi-microscopic, spectroscopic, and electrochemical imaging can start to elucidate the nature of the nucleation sites and provide a holistic picture of the nucleation mechanism. Note that these approaches have started to reveal new knowledge about the active sites in electrocatalysis.40,63–66 In addition, while various analytical techniques have been applied to reveal different aspects of the nucleation, translating these measurements to the conditions relevant to the electrochemical interface, or in situ, is needed. Therefore, further development of in situ methods with a higher spatial and temporal resolution while minimizing the interference to the electrochemical system is envisioned to test different nucleation mechanisms, e.g., classical, atomistic, and non-classical nucleation theories, and their relevance in different nucleation systems. The high-quality experimental data will in turn help the development of a more refined theoretical framework.46 For example, in situ TEM methods, including the use of graphene membranes, have enabled the imaging of the growth and dissolution of nanoparticles with atomic resolution.67–69 Combining these approaches with electrochemical control and measurement is promising in revealing the dynamics of the nucleation sites on the electrode surface.70 In addition, high-speed atomic force microscopy (HS-AFM), which has observed increasing use in imaging the dynamics of proteins and biomolecules,71 seems suitable to capture the electrochemical nucleation processes in situ. Additionally, developing simplified but well-characterized model systems that are amenable to computation study is critical in fully unleashing the power of computation to provide detailed molecular information in electrochemical nucleation processes.72,73 Moreover, the application of sophisticated statistical analysis, including exploring the spatial and temporal correlation in the data, can reveal hidden mechanisms in complex and high-dimensional data,35 which have been recently demonstrated in the discovery of non-random nucleation in electrochemical roughening on Pt.74
Finally, developing new technology based on the concept of electrochemical phase transition is also expected to gain increasing interest. These include assembling complex structures and nano-devices in electrochemical additive manufacturing,75,76 and developing analytical sensors using phase transition.77 In addition, designing interfaces and interphases that control nucleation (e.g., uniform nucleation in batteries, mitigate or re-direct gas bubble nucleation) can benefit from the fundamental understanding of electrochemical nucleation.
Author contributions
All authors contributed equally to the work.
Conflicts of interest
The authors have no conflicts of interest to declare.
Acknowledgements
Acknowledgment is made to the Donors of the American Chemical Society Petroleum Research Fund for support of this research (61155-DNI5). The authors also acknowledge research support from The Welch Foundation (F-2158-20230405) and UT Austin CNS Catalyst Award.
References
- X. Zhao, H. Ren and L. Luo, Gas Bubbles in Electrochemical Gas Evolution Reactions, Langmuir, 2019, 35, 5392–5408 CrossRef CAS PubMed.
- A. Angulo, P. van der Linde, H. Gardeniers, M. Modestino and D. Fernández Rivas, Influence of Bubbles on the Energy Conversion Efficiency of Electrochemical Reactors, Joule, 2020, 4, 555–579 CrossRef CAS.
- G. Luo, Y. Yuan, D.-Y. Li, N. Li and G.-H. Yuan, Current Transition of Nucleation and Growth under Diffusion-Controlled Electrocrystallization: A Brief Review, Coatings, 2022, 12, 1195 CrossRef CAS.
- Z. Luo, X. Qiu, C. Liu, S. Li, C. Wang, G. Zou, H. Hou and X. Ji, Interfacial Challenges Towards Stable Li Metal Anode, Nano Energy, 2021, 79, 105507 CrossRef CAS.
- X. Xia, C.-F. Du, S. Zhong, Y. Jiang, H. Yu, W. Sun, H. Pan, X. Rui and Y. Yu, Homogeneous Na Deposition Enabling High-Energy Na-Metal Batteries, Adv. Funct. Mater., 2022, 32, 2110280 CrossRef CAS.
- P. Liu and D. Mitlin, Emerging Potassium Metal Anodes: Perspectives on Control of the Electrochemical Interfaces, Acc. Chem. Res., 2020, 53, 1161–1175 CrossRef CAS PubMed.
- C. Wei, L. Tan, Y. Zhang, K. Zhang, B. Xi, S. Xiong, J. Feng and Y. Qian, Covalent Organic Frameworks and Their Derivatives for Better Metal Anodes in Rechargeable Batteries, ACS Nano, 2021, 15, 12741–12767 CrossRef CAS PubMed.
- X. Yan, L. Lin, Q. Chen, Q. Xie, B. Qu, L. Wang and D.-L. Peng, Multifunctional Roles of Carbon-Based Hosts for Li-Metal Anodes: A Review, Carbon Energy, 2021, 3, 303–329 CrossRef CAS.
- A. R. Zeradjanin, P. Narangoda, I. Spanos, J. Masa and R. Schlögl, How to Minimise Destabilising Effect of Gas Bubbles on Water Splitting Electrocatalysts?, Curr. Opin. Electrochem., 2021, 30, 100797 CrossRef CAS.
- S. A. Kukushkin and A. V. Osipov, New Phase Formation on Solid Surfaces and Thin Film Condensation, Prog. Surf. Sci., 1996, 51, 1–107 CrossRef CAS.
- F. Wang, V. N. Richards, S. P. Shields and W. E. Buhro, Kinetics and Mechanisms of Aggregative Nanocrystal Growth, Chem. Mater., 2014, 26, 5–21 CrossRef CAS.
-
L. R. F. Allen, J. Bard and H. S. White, in Electrochemical Methods: Fundamentals and Applications, ed. S. Volk, Wiley, Hoboken, NJ, 3rd edn, 2022 Search PubMed.
- L. Xu, H.-W. Liang, Y. Yang and S.-H. Yu, Stability and Reactivity: Positive and Negative Aspects for Nanoparticle Processing, Chem. Rev., 2018, 118, 3209–3250 CrossRef CAS PubMed.
- D. K. Pattadar and F. P. Zamborini, Effect of Size, Coverage, and Dispersity on the Potential-Controlled Ostwald Ripening of Metal Nanoparticles, Langmuir, 2019, 35, 16416–16426 CrossRef CAS PubMed.
- P. L. Redmond, A. J. Hallock and L. E. Brus, Electrochemical Ostwald Ripening of Colloidal Ag Particles on Conductive Substrates, Nano Lett., 2005, 5, 131–135 CrossRef CAS PubMed.
- M. Volmer and A. Weber, Keimbildung in Übersättigten Gebilden, Z. Phys. Chem., 1926, 119U, 277–301 CrossRef.
- Y. B. Zeldovich, On the Theory of New Phase Formation: Cavitation, Acta Physicochim. URSS, 1943, 18, 1–22 CAS.
- S. Toschev and I. Markov, An Experimental Study of Non-Steady State Nucleation, Ber. Bunsenges. Phys. Chem., 2010, 73, 184–188 Search PubMed.
- E. Budewski, W. Bostanoff, T. Vitanoff, Z. Stoinoff, A. Kotzewa and R. Kaischew, Zweidimensionale Keimbildung Und Ausbreitung Von Monoatomaren Schichten an Versetzungsfreien (100)-Flächen Von Silbereinkristallen, Phys. Status Solidi
B, 1966, 13, 577–588 CrossRef.
- E. Budewski, W. Bostanoff, T. Witanoff, Z. Stoinoff, A. Kotzewa and R. Kaischew, Keimbildungserscheinungen an Versetzungsfreien (100)-Flächen Von Silbereinkristallen, Electrochim. Acta, 1966, 11, 1697–1707 CrossRef.
- E. Budewski, W. Bostanoff, T. Vitanoff, Z. Stoinoff, A. Kotzewa and R. Kaischew, Phys. Status Solidi B, 1966, 13, 577–588 CrossRef.
- S. R. German, M. A. Edwards, H. Ren and H. S. White, Critical Nuclei Size, Rate, and Activation Energy of H2 Gas Nucleation, J. Am. Chem. Soc., 2018, 140, 4047–4053 CrossRef CAS PubMed.
- B. J. Murray, Q. Li, J. T. Newberg, J. C. Hemminger and R. M. Penner, Silver Oxide Microwires: Electrodeposition and Observation of Reversible Resistance Modulation Upon Exposure to Ammonia Vapor, Chem. Mater., 2005, 17, 6611–6618 CrossRef CAS.
- R. M. Stiger, S. Gorer, B. Craft and R. M. Penner, Investigations of Electrochemical Silver Nanocrystal Growth on Hydrogen-Terminated Silicon(100), Langmuir, 1999, 15, 790–798 CrossRef CAS.
- A. Milchev, Electrochemical Phase Formation: Classical and Atomistic Theoretical Models, Nanoscale, 2016, 8, 13867–13872 RSC.
-
E. Budevski and W. Lorenz, Initial Stages of Bulk Phase Formation, VCH, Weinheim ; New York, 1996 Search PubMed.
- D. Erdemir, A. Y. Lee and A. S. Myerson, Nucleation of Crystals from Solution: Classical and Two-Step Models, Acc. Chem. Res., 2009, 42, 621–629 CrossRef CAS PubMed.
- S. Karthika, T. K. Radhakrishnan and P. Kalaichelvi, A Review of Classical and Non-classical Nucleation Theories, Cryst. Growth Des., 2016, 16, 6663–6681 CrossRef CAS.
- P. J. M. Smeets, A. R. Finney, W. J. E. M. Habraken, F. Nudelman, H. Friedrich, J. Laven, J. J. De Yoreo, P. M. Rodger and N. A. J. M. Sommerdijk, A Classical View on Non-classical Nucleation, Proc. Natl. Acad. Sci. U. S. A., 2017, 114, E7882–E7890 CrossRef CAS.
- J. V. Zoval, R. M. Stiger, P. R. Biernacki and R. M. Penner, Electrochemical Deposition of Silver Nanocrystallites on the Atomically Smooth Graphite Basal Plane, J. Phys. Chem., 1996, 100, 837–844 CrossRef CAS.
- R. M. Penner, Mesoscopic Metal Particles and Wires by Electrodeposition, J. Phys. Chem. B, 2002, 106, 3339–3353 CrossRef CAS.
- A. Radisic, P. M. Vereecken, J. B. Hannon, P. C. Searson and F. M. Ross, Quantifying Electrochemical Nucleation and Growth of Nanoscale Clusters Using Real-Time Kinetic Data, Nano Lett., 2006, 6, 238–242 CrossRef CAS PubMed.
- C. Cao, B. Shyam, J. Wang, M. F. Toney and H.-G. Steinrück, Shedding X-Ray Light on the Interfacial Electrochemistry of Silicon Anodes for Li-Ion Batteries, Acc. Chem. Res., 2019, 52, 2673–2683 CrossRef CAS PubMed.
- E. R. White, S. B. Singer, V. Augustyn, W. A. Hubbard, M. Mecklenburg, B. Dunn and B. C. Regan,
In Situ Transmission Electron Microscopy of Lead Dendrites and Lead Ions in Aqueous Solution, ACS Nano, 2012, 6, 6308–6317 CrossRef CAS PubMed.
- M. A. Edwards, H. S. White and H. Ren, Voltammetric Determination of the Stochastic Formation Rate and Geometry of Individual H2, N2, and O2 Bubble Nuclei, ACS Nano, 2019, 13, 6330–6340 CrossRef CAS PubMed.
- M. Y. Abyaneh and M. Fleischmann, The Role of Nucleation and of Overlap in Electrocrystallisation Reactions, Electrochim. Acta, 1982, 27, 1513–1518 CrossRef CAS.
- M. Y. Abyaneh and M. Fleischmann, Extracting Nucleation Rates from Current–Time Transients: Further Comments, J. Electroanal. Chem., 2002, 530, 123–125 CrossRef CAS.
- R. L. Deutscher and S. Fletcher, Nucleation on Active Sites: Part V. The Theory of Nucleation Rate Dispersion, J. Electroanal. Chem. Interfacial
Electrochem., 1990, 277, 1–18 CrossRef CAS.
- W. Obretenov, M. Höpfner, W. J. Lorenz, E. Budevski, G. Staikov and H. Siegenthaler, Characterization of the Surface Structure of Silver Single Crystal Electrodes by Ex Situ and In Situ STM, Surf. Sci., 1992, 271, 191–200 CrossRef CAS.
- L. A. Baker, Perspective and Prospectus on Single-Entity Electrochemistry, J. Am. Chem. Soc., 2018, 140, 15549–15559 CrossRef CAS PubMed.
- L. Luo and H. S. White, Electrogeneration of Single Nanobubbles at Sub-50-nm-Radius Platinum Nanodisk Electrodes, Langmuir, 2013, 29, 11169–11175 CrossRef CAS PubMed.
- Q. Chen, H. S. Wiedenroth, S. R. German and H. S. White, Electrochemical Nucleation of Stable N2 Nanobubbles at Pt Nanoelectrodes, J. Am. Chem. Soc., 2015, 137, 12064–12069 CrossRef CAS PubMed.
- H. Ren, S. R. German, M. A. Edwards, Q. Chen and H. S. White, Electrochemical Generation of Individual O2 Nanobubbles Via H2O2 Oxidation, J. Phys. Chem. Lett., 2017, 8, 2450–2454 CrossRef CAS PubMed.
- H. Ren, M. A. Edwards, Y. Wang and H. S. White, Electrochemically Controlled Nucleation of Single CO2 Nanobubbles Via Formate Oxidation at Pt Nanoelectrodes, J. Phys. Chem. Lett., 2020, 11, 1291–1296 CrossRef CAS PubMed.
- J. Velmurugan, J.-M. Noël and M. V. Mirkin, Nucleation and Growth of Mercury on Pt Nanoelectrodes at Different Overpotentials, Chem. Sci., 2013, 5, 189–194 RSC.
- N. J. Vitti, P. Majumdar and H. S. White, Critical Nucleus Size and Activation Energy of Ag Nucleation by Electrochemical Observation of Isolated Nucleation Events, Langmuir, 2023, 39, 1173–1180 CrossRef CAS PubMed.
- B. Blount, K. Kilner, H. Hu, D. Gohmann, E. Gordon, Y. Wang and H. Ren, Electrochemically Induced Nucleation of a Nanoscopic Ionic Solid, J. Phys. Chem. C, 2020, 124, 17413–17417 CrossRef CAS.
- N. Ebejer, A. G. Güell, S. C. S. Lai, K. McKelvey, M. E. Snowden and P. R. Unwin, Scanning Electrochemical Cell Microscopy: A Versatile Technique for Nanoscale Electrochemistry and Functional Imaging, Annu. Rev. Anal. Chem., 2013, 6, 329–351 CrossRef CAS PubMed.
- C. L. Bentley, M. Kang and P. R. Unwin, Nanoscale Structure Dynamics within Electrocatalytic Materials, J. Am. Chem. Soc., 2017, 139, 16813–16821 CrossRef CAS PubMed.
- J. Y. Y. Sze, S. Kumar, A. P. Ivanov, S.-H. Oh and J. B. Edel, Fine Tuning of Nanopipettes Using Atomic Layer Deposition for Single Molecule Sensing, Analyst, 2015, 140, 4828–4834 RSC.
- Y. Wang, E. Gordon and H. Ren, Mapping the Nucleation of H2 Bubbles on Polycrystalline Pt Via Scanning Electrochemical Cell Microscopy, J. Phys. Chem. Lett., 2019, 10, 3887–3892 CrossRef CAS PubMed.
- X. Deng, Y. Shan, X. Meng, Z. Yu, X. Lu, Y. Ma, J. Zhao, D. Qiu, X. Zhang, Y. Liu and Q. Chen, Direct Measuring of Single–Heterogeneous Bubble Nucleation Mediated by Surface Topology, Proc. Natl. Acad. Sci. U. S. A., 2022, 119, e2205827119 CrossRef CAS PubMed.
- Y. Liu, C. Jin, Y. Liu, K. H. Ruiz, H. Ren, Y. Fan, H. S. White and Q. Chen, Visualization and Quantification of Electrochemical H2 Bubble Nucleation at Pt, Au, and MoS2 Substrates, ACS Sens., 2021, 6, 355–363 CrossRef CAS PubMed.
- N. S. Georgescu, D. A. Robinson and H. S. White, Effect of Nonuniform Mass Transport on Nanobubble Nucleation at Individual Pt Nanoparticles, J. Phys. Chem. C, 2021, 125, 19724–19732 CrossRef CAS.
- S. C. S. Lai, R. A. Lazenby, P. M. Kirkman and P. R. Unwin, Nucleation, Aggregative Growth and Detachment of Metal Nanoparticles During Electrodeposition at Electrode Surfaces, Chem. Sci., 2015, 6, 1126–1138 RSC.
- M. R. Powell, M. Sullivan, I. Vlassiouk, D. Constantin, O. Sudre, C. C. Martens, R. S. Eisenberg and Z. S. Siwy, Nanoprecipitation-assisted Ion Current Oscillations, Nat. Nanotechnol., 2008, 3, 51–57 CrossRef CAS PubMed.
- E. T. Acar, P. Hinkle and Z. S. Siwy, Concentration-Polarization-Induced Precipitation and Ionic Current Oscillations with Tunable Frequency, J. Phys. Chem. C, 2018, 122, 3648–3654 CrossRef CAS.
- L. Innes, M. R. Powell, I. Vlassiouk, C. Martens and Z. S. Siwy, Precipitation-Induced Voltage-Dependent Ion Current Fluctuations in Conical Nanopores, J. Phys. Chem. C, 2010, 114, 8126–8134 CrossRef CAS.
- Z. S. Siwy, M. R. Powell, A. Petrov, E. Kalman, C. Trautmann and R. S. Eisenberg, Calcium-Induced Voltage Gating in Single Conical Nanopores, Nano Lett., 2006, 6, 1729–1734 CrossRef CAS PubMed.
- P. D. Morris, I. J. McPherson, M. A. Edwards, R. J. Kashtiban, R. I. Walton and P. R. Unwin, Electric Field-Controlled Synthesis and Characterisation of Single Metal–Organic-Framework (MOF) Nanoparticles, Angew. Chem., Int. Ed. Engl., 2020, 59, 19696–19701 CrossRef CAS PubMed.
- F. M. Maddar, D. Perry and P. R. Unwin, Confined Crystallization of Organic Materials in Nanopipettes: Tracking the Early Stages of Crystal Growth and Making Seeds for Unusual Polymorphs, Cryst. Growth Des., 2017, 17, 6565–6571 CrossRef CAS.
- Y. Li, M. Kvetny, M. Bowen, W. Brown, D. Wang and G. Wang, Method to Directly Measure and Actively Control a Single Nucleation-Crystal Growth Process, Cryst. Growth Des., 2019, 19, 2470–2475 CrossRef CAS.
- C. Santana Santos, B. N. Jaato, I. Sanjuán, W. Schuhmann and C. Andronescu,
Operando Scanning Electrochemical Probe Microscopy During Electrocatalysis, Chem. Rev., 2023, 123, 4972–5019 CrossRef CAS PubMed.
- C. L. Bentley, M. Kang, F. M. Maddar, F. Li, M. Walker, J. Zhang and P. R. Unwin, Electrochemical Maps and Movies of the Hydrogen Evolution Reaction on Natural Crystals of Molybdenite (MoS2): Basal Vs. Edge Plane Activity, Chem. Sci., 2017, 8, 6583–6593 RSC.
- R. G. Mariano, M. Kang, O. J. Wahab, I. J. McPherson, J. A. Rabinowitz, P. R. Unwin and M. W. Kanan, Microstructural Origin of Locally Enhanced CO2 Electroreduction Activity on Gold, Nat. Mater., 2021, 20, 1000–1006 CrossRef CAS PubMed.
- J. T. Mefford, A. R. Akbashev, M. Kang, C. L. Bentley, W. E. Gent, H. D. Deng, D. H. Alsem, Y.-S. Yu, N. J. Salmon, D. A. Shapiro, P. R. Unwin and W. C. Chueh, Correlative Operando Microscopy of Oxygen Evolution Electrocatalysts, Nature, 2021, 593, 67–73 CrossRef CAS PubMed.
- X. Ye, M. R. Jones, L. B. Frechette, Q. Chen, A. S. Powers, P. Ercius, G. Dunn, G. M. Rotskoff, S. C. Nguyen, V. P. Adiga, A. Zettl, E. Rabani, P. L. Geissler and A. P. Alivisatos, Single-Particle Mapping of Nonequilibrium Nanocrystal Transformations, Science, 2016, 354, 874–877 CrossRef CAS PubMed.
- I. A. Moreno-Hernandez, M. F. Crook, V. Jamali and A. P. Alivisatos, Recent Advances in the Study of Colloidal Nanocrystals Enabled by In Situ Liquid-Phase Transmission Electron Microscopy, MRS Bull., 2022, 47, 305–313 CrossRef.
- M. F. Crook, I. A. Moreno-Hernandez, J. C. Ondry, J. Ciston, K. C. Bustillo, A. Vargas and A. P. Alivisatos, EELS Studies of Cerium Electrolyte Reveal Substantial Solute Concentration Effects in Graphene Liquid Cells, J. Am. Chem. Soc., 2023, 145, 6648–6657 CrossRef CAS PubMed.
- I. A. Moreno-Hernandez, M. F. Crook, J. C. Ondry and A. P. Alivisatos, Redox Mediated Control of Electrochemical Potential in Liquid Cell Electron Microscopy, J. Am. Chem. Soc., 2021, 143, 12082–12089 CrossRef CAS PubMed.
- T. Ando, High-Speed Atomic Force Microscopy and Its Future Prospects, Biophys. Rev., 2018, 10, 285–292 CrossRef CAS PubMed.
- E. D. Gadea, Y. A. Perez Sirkin, V. Molinero and D. A. Scherlis, Electrochemically Generated Nanobubbles: Invariance of the Current with Respect to Electrode Size and Potential, J. Phys. Chem. Lett., 2020, 11, 6573–6579 CrossRef CAS PubMed.
- Y. A. Perez Sirkin, E. D. Gadea, D. A. Scherlis and V. Molinero, Mechanisms of Nucleation and Stationary States of Electrochemically Generated Nanobubbles, J. Am. Chem. Soc., 2019, 141, 10801–10811 CrossRef CAS PubMed.
- M. J. Rost, L. Jacobse and M. T. M. Koper, Non-Random Island Nucleation in the Electrochemical Roughening on Pt(111), Angew. Chem., Int. Ed., 2023, e202216376 CAS.
- A. N. Patel, K. McKelvey and P. R. Unwin, Nanoscale Electrochemical Patterning Reveals the Active Sites for Catechol Oxidation at Graphite Surfaces, J. Am. Chem. Soc., 2012, 134, 20246–20249 CrossRef CAS PubMed.
- J. Hengsteler, B. Mandal, C. van Nisselroy, G. P. S. Lau, T. Schlotter, T. Zambelli and D. Momotenko, Bringing Electrochemical Three-Dimensional Printing to the Nanoscale, Nano Lett., 2021, 21, 9093–9101 CrossRef CAS PubMed.
- R. Ranaweera, C. Ghafari and L. Luo, Bubble-Nucleation-Based Method for the Selective and Sensitive Electrochemical Detection of Surfactants, Anal. Chem., 2019, 91, 7744–7748 CrossRef CAS PubMed.
|
This journal is © The Royal Society of Chemistry 2023 |