DOI:
10.1039/D2NA00530A
(Review Article)
Nanoscale Adv., 2023,
5, 1527-1558
Functional nano-systems for transdermal drug delivery and skin therapy
Received
9th August 2022
, Accepted 27th November 2022
First published on 24th February 2023
Abstract
Transdermal drug delivery is one of the least intrusive and patient-friendly ways for therapeutic agent administration. Recently, functional nano-systems have been demonstrated as one of the most promising strategies to treat skin diseases by improving drug penetration across the skin barrier and achieving therapeutically effective drug concentrations in the target cutaneous tissues. Here, a brief review of functional nano-systems for promoting transdermal drug delivery is presented. The fundamentals of transdermal delivery, including skin biology and penetration routes, are introduced. The characteristics of functional nano-systems for facilitating transdermal drug delivery are elucidated. Moreover, the fabrication of various types of functional transdermal nano-systems is systematically presented. Multiple techniques for evaluating the transdermal capacities of nano-systems are illustrated. Finally, the advances in the applications of functional transdermal nano-systems for treating different skin diseases are summarized.
1. Introduction
Transdermal drug delivery is one of the least intrusive and patient-friendly ways for therapeutic agent administration. It can not only boost medication bioavailability by concentrating drug molecules in a particular skin region, but also limit the possibility of unforeseen adverse effects.1–3 Therefore, transdermal drug delivery is an appealing option for oral administration and an alternative to hypodermic injection. In the 1970s, the Food and Drug Administration (FDA) first authorized the transdermal patch to administer scopolamine for the treatment of motion sickness.4 Since then, various physical and chemical strategies of transdermal drug delivery systems (TDDs) have been developed with significant progress achieved. Physical methods include epidermal erosion, skin puncture devices using probes, high-frequency oscillating needle bundles, microneedle arrays, and high-velocity dry powder jets, whereas chemical methods include the use of penetration enhancers and prodrugs.5 However, physical methods and chemical methods possess their own drawbacks. Physical methods such as iontophoresis may lead to pain, a burning sensation, blister formation, and skin necrosis with increasing current strength or if applied for a longer period.6 Slight itching, irritation, and a burning sensation have been reported with sonophoresis.7 Microneedles may cause skin irritation or allergy in sensitive skin. Topical formulations such as pastes, creams, gels, oils and ointments8,9 have been developed with significant progress achieved but have poor permeation through the stratum corneum (SC) and require a high dose and repeated applications in a day, which may cause severe side effects like skin rashes and itching that lead to poor patient compliance for long term therapy.10 Moreover, the application of pro-drugs is another chemical technique to conquer the barrier function of the skin. The main disadvantage of this approach is that the pro-moiety is basically a redundant coarse stone, which, when released, may result in adverse effects.7 Thus, it is highly desired to develop new types of TDDs, improve drug penetration across the skin barrier and achieve therapeutically effective drug concentrations in the target cutaneous tissues.
Ever since the term “nanoparticles” was known to the scientific world from the 1970s, functional nano-systems have attracted great scientific interest and have always been the research hotspot in the dynamic interdisciplinary branch of science.11–13 Functional nano-systems typically have diameters ranging from 10 to 1000 nm in at least one dimension and are composed of different biocompatible materials, including natural or synthetic polymers and lipids.14 Due to their distinct characteristics, including small size and large specific surface area, high encapsulation capacity of both hydrophilic and lipophilic drugs and applicability for multiple administration routes, functional nano-systems can be used as superior drug delivery platforms, which can regulate the release rate, change the biodistribution and improve the bioavailability of the delivered drugs. As a result, functional nano-systems provide new concepts and opportunities for developing new TDDs. Recently, functional nano-systems have been attracted a great deal of interest for transdermal drug delivery, and plenty of research and significant achievements have been made. Various functional nano-systems, such as nanogels, polymeric nanoparticles, metallic nanoparticles, dendrimers, micelles, lipid nanoparticles and quantum dot nanocarriers, have been demonstrated to be an effective strategy to overcome the skin barrier, while causing no tissue harm and promoting transdermal drug delivery (Fig. 1).15–20 Therefore, a comprehensive depiction of the whole scene on functional nano-systems for transdermal drug delivery, from fundamentals to evaluations and various advanced applications, is desired.
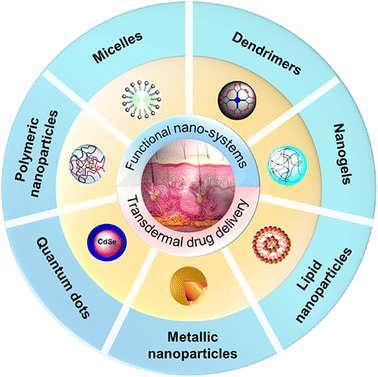 |
| Fig. 1 Schematic illustration of representative functional nano-systems for promoting transdermal drug delivery, including liposomes, quantum dots, hybrid NPs, dendrimers, micelles, ionic liquid-based NPs and polymeric nanogels. Adapted from ref. 19. Copyright 2016 John Wiley & Sons Ltd. Adapted from ref. 20. Copyright 2019 MDPI. | |
In this review, we highlight the recent progress on functional nano-systems for promoting transdermal drug delivery. First, the fundamentals of transdermal delivery, including skin biology and penetration routes, are introduced. Then, the characteristics of functional nano-systems for facilitating transdermal drug delivery are elucidated. Moreover, the fabrication of various types of functional transdermal nano-systems are systematically presented. Besides, multiple techniques for evaluating the transdermal capacities of nano-systems are illustrated. Finally, the advances in applications of functional transdermal nano-systems for treating different skin diseases are summarized. We present an overview of functional nano-systems for transdermal drug delivery covering from basic fundamentals to progress made for advanced therapeutic applications in recent years.
2. Fundamentals of transdermal delivery
2.1 Skin biology
As the biggest organ of the human body with simple accessibility, the skin is a prospective option for medication administration. Thus, it been extensively examined, and its biological structure is well understood.21 The skin structure can be categorized into three layers epidermis, dermis, and subcutaneous “fat” tissue (Fig. 2). The epidermis comprises two main layers: stratum corneum (SC), the viable epidermis (VE). The SC, with a thickness of 10–20 μm, consists of dead, flattened, keratin-rich cells (keratinocytes) and an intercellular lamellar lipid bilayer, which acts as the outer layer of the skin. The lipid layer primarily consists of ceramides (50%), cholesterol (25%), and other free fatty acids, and is estimated to be <100 nm wide, limiting passive diffusion to small, lipophilic molecules.21 The SC is characterized as having a ‘brick-and-mortar’ structure due to the long, flat, tile-like form of the corneocytes22 and responsible for the most of the skin's barrier function.23–26 The keratinocytes underlying the SC make up the VE, which is approximately 100–150 μm thick. These cells continually proliferate, pushing older cells to the surface where they undergo keratinization and programmed cell death, resulting in the formation of the SC.27 Keratinocytes can produce cytokines and chemokines to boost immune activity at the site of infection.28 Patrolling dendritic cells may be recruited as a result of this signaling.
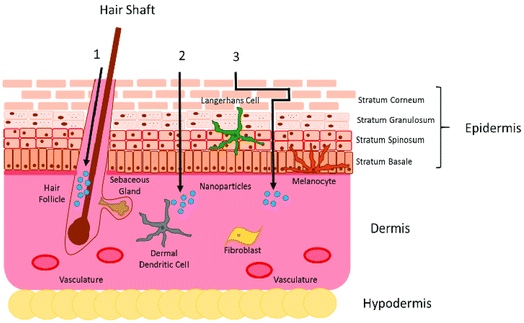 |
| Fig. 2 The skin biological structure and three distinct pathways: (A) transcellular, (B) intercellular and (C) appendageal routes. Adapted from ref. 26. Copyright 2016 licensee MDPI. | |
The dermis, which is ∼1200 μm thick, is on top of the skin's middle layer. It is made up of connective tissue like collagen, elastin, fibronectin, and glycosaminoglycan matrix, and it is dotted with blood and lymphatic vessels, nerve endings, hair follicles, sebaceous glands, and sweat glands.21,29 The hair follicles and sweat ducts form a direct connecting path from the dermis to the skin surface, bypassing the stratum corneum and henceforth involved in providing the appendageal route of skin permeation.29
The superficial fascia, or the loose connective and adipose tissue beneath the dermis that connects and supports the skin and muscle, is known as the subcutaneous “fat” tissue. It is composed of cells that contain large quantities of fat, making the cytoplasm lipoidal in character.30 The collagen between the fat cells provides the linkage of the epidermis and the dermis with the underlying structures of the skin.
2.2 Skin penetration routes
In accordance with the skin biological structure, the process of transdermal delivery can occur via three distinct pathways: (A) intercellular route, (B) transcellular route, and (C) appendageal route (Fig. 2). The intercellular route has been identified as the primary pathway, in which the penetrating nanocarriers cross the intercellular lipids by diffusion between the cells.31 The size as well as the mechanical characteristics of the nanocarriers must be considered for this pathway since they must be flexible.17 Rigid nanocarriers, like metal NPs, have been reported to have a limited ability to enter the SC via the intercellular pathway. It has been proposed that their lack of flexibility impedes their diffusion between cells.32 In contrast, highly flexible polymer-based nanocarriers have been shown to be able to penetrate into the SC. The transcellular route is the most direct and fast pathway for the nanocarriers to permeate the skin; however, this pathway is challenging as NPs must overcome both the lipophilic (cell membrane and the lipid matrix) and lipophobic barriers (inside the cells) within the skin cells.33 Functional nanocarriers with a high degree of amphiphilicity may be suitable options for overcoming such barriers. The follicular pathway is the most commonly postulated method for highly rigid nanocarriers to penetrate the skin. This approach addresses the administration of medications directly through hair follicles and glandular ducts. However, this route is severely constrained since hair follicles and glandular ducts account for just 0.1 and 0.01% of the entire skin surface area, respectively.34 Meanwhile, there is sufficient evidence that the size of the applied nanocarriers has an important impact on the penetration depth and selective targeting of certain compartments inside the hair follicle.35 Toll et al. showed that the penetration depth in terminal hair follicles increases when smaller NPs are used.36 Similarly, Alvarez Roman et al. demonstrated with animal models that ∼20 nm NPs penetrate deeper than polystyrene ∼200 nm particles.37 Furthermore, the accumulation of nanocarriers within the hair follicle canal can benefit from their depot activity, allowing for continuous drug release over time. Thus, a constant drug level in tissue could be achieved opening the way for transdermal delivery of active compounds to specific targets within the hair follicle.38,39 Taken together, each of the penetration routes represents a chance for nano-systems to overcome the skin barrier and the properties of the functional nano-systems must be fine-tuned to meet the requirements of the selected routes.
3. Characteristics of functional nano-systems for facilitating transdermal drug delivery
Functional nano-systems with the capacity to cross the skin offer an attractive option to facilitate transdermal drug delivery. It is worth noting that due to the unique physicochemical features of nano-systems, such as nanoscale effect, form, stiffness, thermo-responsiveness, surface charge, and pH-responsiveness, that can all impact skin penetration and interact with biological components to improve the transdermal capacity, the efficacy of nano-systems is more from their capacity to cross the skin, rather than acting as a skin surface depot. The representative characteristics of functional nano-systems that contribute to the facilitation of the transdermal drug delivery are discussed in detail in the following sections.
3.1 Nanometer effects
Among all the characteristics, the intrinsic nanometer effects are no doubt the most important factor. Specifically, the nanometer effects refer to the phenomenon that nanocarriers have size-dependent penetration capacities across the epidermal barriers.40–42 For example, the penetration of differently sized citrate-coated gold nanoparticles, with diameters of 15, 102, and 198 nm, were investigated, respectively. Smaller NPs can permeate deeper into the skin (fibrous layer and adipose tissues), whereas larger NPs gather mostly in the epidermis and dermis.40 Moreover, the reduction in the size of nanocarriers and the relative increase in the surface area of the nanocarriers may improve the function of bioactive compounds; when the size is reduced, the ability of functional nano-systems to interact with biological membranes is also enhanced, promoting their biological activity.43 Moreover, Wiraja et al. demonstrated framework nucleic acids (FNAs) with distinct shapes and sizes for topical applications. Skin histology reveals size-dependent penetration, with FNAs ≤75 nm effectively reaching the dermis layer. 17 nm-tetrahedral FNAs show the greatest penetration to 350 μm from the skin periphery.44
3.2 Hydrating effects
Apart from having a nano-meter effect, functional nano-systems show the hydration effect that can promote skin hydration and be useful in transdermal delivery applications. Generally, the skin hydration can promote the transdermal transport of both hydrophilic and hydrophobic drugs, while hydrophobic compounds can hardly penetrate and cause skin irritation in some situations.45,46 Thermo-responsive nano-systems, such as nanogels (NGs) and nano-capsules, have been demonstrated to induce skin hydration by releasing water in response to temperature change, which enhances the penetration of hydrophilic compounds, ranging from dyes to proteins.47 For instance, Giulbudagian et al. reported the unique properties of thermos-responsive nanogels (tNGs) acting as penetration enhancers to increase skin hydration.47 Three types of tNGs were synthesized by employing dendritic polyglycerol as a multifunctional crosslinker and poly(N-isopropylacrylamide) (PNIPAM), p(GME-co-EGE) and p(di(ethylene glycol) methyl ether methacrylate-co-oligo ethylene glycol methacrylate) p(DEGMA-co-OEGMA475) as three kinds of thermo-responsive polymers. These NGs were covalently tagged with indodicarbocyanine (IDCC) and loaded with fluorescein to study both the penetration of tNGs and the penetration of released fluorescein. The results showed that skin samples treated with NGs were swollen and the SC exhibited a disruption in the structure of its proteins and lipids as opposed to untreated skin samples (Fig. 3A–C). Furthermore, after adhering to the skin surface, lipid-based nanocarriers can alter skin moisturizing keratin and modify the structure of both SC lipids and keratin. Both keratin hydration and structural alterations to the lipids and keratin can weaken the SC's barrier function, allowing the medication to penetrate deeper into the skin.43
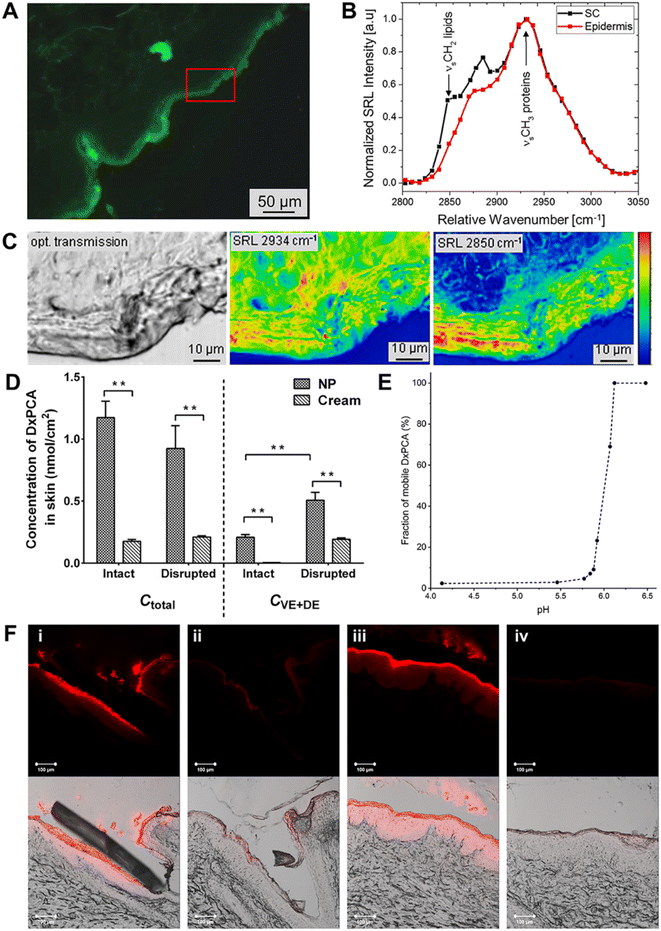 |
| Fig. 3 Illustration of Stimulated Raman-Loss (SRL) images of the skin distribution of proteins and lipids by treating with tNG, and pH-sensitive Eudragit® L 100 NPs for transdermal delivery. (A) Fluorescence microscopy image of the skin region for SRL (red frame). (B) SRL spectra of the SC and viable epidermis and (C) optical transmission image (left); distributions of proteins (middle) and lipids (right). (D) Differential permeation of DxPCA from NPs and cream in intact and barrier-disrupted porcine ear skin. (E) Release efficiency of DxPCA from NP dispersion calculated from simulated EPR spectra under different pH conditions. (F) Confocal laser scanning microscopy (CLSM) images of the hair follicles (HFs) (i and ii) and glabrous skin (iii and iv) of the intact skin after the application of Nile red (NR) loaded in NPs (i and iii) and cream (ii and iv). (A–C) Adapted from ref. 47. Copyright 2016 Elsevier. (D and E) Adapted from ref. 53. Copyright 2019 Elsevier. | |
3.3 pH-Responsiveness
Together with temperature, pH is one of the important stimulus-response mechanisms that has been widely applied to trigger drug release from nano-systems. Healthy skin is known to have an acidic surface with a pH range of 4.1 to 5.8. As the skin layers change, the pH of the deeper skin layers and hair follicles shifts to a near-neutral environment.48 In addition, skin illnesses (inflammations and epidermal lesions) can alter pH levels.49 Due to these pH changes in different skin layers and environments, pH-responsive nano-systems can exhibit physicochemical changes in various skin layers or diseased areas, which facilitate the transdermal drug delivery.50 Eudragits and chitosan (CS) are two pH-responsive polymers that have been used in therapeutics.51,52 For instance, Dong et al. designed pH-responsive NPs based on Eudragit L100 and loaded with spin-labeled dexamethasone (DxPCA). When pH was above 6, NPs showed higher skin permeation than commercial cream in both intact and barrier disrupted skin (Fig. 3D–F).53 Moreover, CS is a common natural pH-responsive polymer whose responsiveness arises from its amino groups, which undergoes protonation in an acidic environment, leading to repulsion of the positively charged groups followed by swelling. Sahu et al. developed a CS nanogel loaded with 5-fluorouracil (5-FU) to act against melanoma and demonstrated the triggered release of 5-FU in a slightly acidic microenvironment, resulting in selective drug accumulation at the melanoma site.52
3.4 Electrostatic charges
The surface charge can affect functional nano-system permeation and penetration on skin.54 Specifically, the surface of cells presents a negative charge mainly caused by sulfated proteoglycan molecules, which are responsible for cell proliferation, migration and movement.55 Thus the electrostatic interactions between the cell membrane and nano-systems can be achieved when nano-systems present a positive charge and therefore promote the transdermal permeation.41,56 Meantime, the negatively charged nano-systems also show high cellular uptake due to a non-specific process of nano-system adsorption on the cell membrane and to the formation of clusters of nano-systems.49 The adsorption of negatively charged NPs to positively charged sites occurs via electrostatic interaction neutralization and membrane bending, or cellular uptake via endocytosis.55 There is no change of the permeation of neutral nano-systems across the endothelial cell monolayer.55
4. Fabrication of functional transdermal nano-systems
With the above-mentioned characteristics, functional nano-systems hold great feasibility to improve transdermal delivery while lowering the undesirable side.57–59 In the following section, functional nano-systems including polymeric nanocarriers (polymeric NPs, polymeric micelles, and dendrimers), lipid-based NPs (solid lipid NPs and nanostructured lipid carriers), vesicular nanocarriers (liposomes, niosomes, transfersomes, and ethosomes), and inorganic NPs (metallic NPs, metallic oxide NPs, silica NPs, and quantum dots) are discussed. To offer a whole scene of the functional nano-systems for transdermal drug delivery, we have elaborately summarized their detailed information including the compositions and fabrication methods in Table 1 and Table 2 shows the advantages and disadvantages of these developed functional nano-systems.
Table 1 Summary of the composition and fabrication methods of functional nano-systems
Nano-systems |
Composition |
Characterization |
Fabrication methods |
Reference |
SLNs |
Solid lipids, surfactants, co-surfactants |
Shape: spherical |
Thin film hydration; hand shaking; reverse-phase evaporation; sonication; ether injection; pro-niosome method; freeze and thaw; dehydration; rehydration; bubble method; solvent injection-homogenization technique; microfluidics |
60–66
|
Size: 50–1000 nm |
Drug: amphotericin B |
NLCs |
Solid lipids, liquid lipids (oils), surfactants |
Shape: spherical |
High pressure homogenization (hot/cold); ultrasonication (probe/bath); solvent evaporation; solvent emulsification diffusion; microemulsion; supercritical fluid; spray drying; hot-melt extrusion (HME) methods |
29, 60, 67–76 |
Size: 120.9–185.2 nm |
Drug: simvastatin |
Liposomes |
Cholesterol, phospholipids |
Shape: spherical |
Reverse-phase evaporation; membrane extrusion; thin film hydration; sonication (probe/bath); ethanol injection; ether injection; freeze-thaw; micro-emulsification; microfluidics; heating methods |
77–92
|
Size: 25–5000 nm |
Drug: diflucortolone valerate |
Ethosomes |
Ethanol, phospholipids |
Shape: spherical |
Thin film hydration; vortex/sonication; ethanol injection; hot and cold methods |
25, 55, 93, 94 |
Drug: lipophilic or hydrophilic drugs |
Transfersomes |
Phospholipids, surfactant/edge activators, alcohol buffering agent |
Shape: spherical |
Thin film hydration |
95–101
|
Size: 90–140 nm |
Drug: meloxicam |
Niosomes |
Cholesterol, nonionic surfactant |
Shape: spherical |
Cold and hot method |
55, 102–113 |
Size: 100 to 2000 nm |
ISAsomes |
Inverse bicontinuous (Q2) and discontinuous hexagonal (H2) phases |
Shape: nonlamellar |
High-energy emulsification methods, microfluidization, high-pressure homogenization |
114–120
|
Size: 100–200 nm |
Drug: progesterone |
Polymeric NPs |
Natural/synthesis polymers |
Shape: spherical |
Emulsification/solvent diffusion; salting; nanoprecipitation; polymerization; dialysis; solvent evaporation; supercritical fluidization |
55, 123–131 |
Size: 10 to 1000 nm |
Drug: donepezil hydrochloride |
Polymeric micelles |
Polymers preferably, polyethylene glycol, aqueous solution, ligands (target molecules) |
Shape: spherical |
Chemical conjugation; physical entrapment through dialysis; emulsification; poly-ionic complexation |
23, 25, 79, 132–137 |
Size: 113.0 ± 34.0 nm, 498.7 ± 35.4 nm |
Drug: curcumin |
Dendrimers |
Core molecule, surface units, monomers |
Shape: spherical |
Divergent; convergent method |
60, 139–145 |
Size: 20 nm |
Metallic NPs |
Metal atom, ligands |
Shape: rod, spherical, triangular, spherical |
Microfluidics |
79, 109, 125, 148–166 |
Size: 2–100 nm |
Metallic oxide NPs |
Metal oxide |
Shape: non-spherical |
Green synthesis methods |
34, 168, 169 |
Size: <100 nm |
QDs |
Metalloid crystalline core, surface groups/polymer/peptide ligand/antibody |
Shape: spherical |
Oxidative cleavage, hydrothermal/solvothermal, microwave/ultrasonic-assisted, electrochemical, pulsed laser ablation, chemical vapor deposition, electron beam irradiation, controllable synthesis, carbonization |
54, 173, 174 |
Size: 20 nm |
Silica NPs |
Silica |
Shape: spherical |
A system chemical method |
41, 79, 170–172 |
Size: 0.05–2 nm |
Table 2 Advantages and disadvantages of nano-systems for transdermal drug delivery
Nano-systems |
Advantage |
Disadvantage |
Reference |
SLNs |
Feasibility in incorporation of lipophilic and hydrophilic drugs, producing on a large industrial scale, good biocompatibility and biodegradability |
No deeper penetration for SLNs, drug expulsion during storage cause by lipid polymorphism for SLNs, and risk of gelation for SLNs |
60–66
|
NLCs |
Better drug loading capacity, high permeation (NLCs: 86.35%, marketed formulation: 69.41%), prevention of drug expulsion during storage, better stability |
No deeper penetration |
29, 60, 67–76 |
Liposomes |
Encapsulation of amphiphilic drugs, low toxicity and biocompatibility (erythema and eschar formation value 0–1), improves localized delivery |
Require special storage conditions, higher production cost, poor permeation to the viable epidermis and dermis, poor physicochemical characteristics for long-term stability (storage at 4–6 °C, in a refrigerator and adjustment of the pH of the dispersions to pH values close to neutral) |
77–92
|
Niosomes, transfersomes, ethosomes |
Incorporation of both lipophilic and hydrophilic drugs, higher encapsulation efficiency, simple and inexpensive to manufacture compared with liposomes, the ability of intact transport across the SC due to their soft and malleable characteristics, enhanced penetration and permeation (niosomes: up to a depth of 120 nm) |
Skin irritation and toxicity (erythema and eschar formation value 0∼1) due to chemical excipients |
25, 55, 93–113 |
ISAsomes |
Better drug loading capacity, high permeation (NLCs: 48.57 × 10−2 ± 0.7 μg cm−2) |
Unknown toxicity and coalescence |
114–120
|
Polymeric NPs |
Targeted to a specific site, higher stability than lipid-based ones, tunable chemical and physical properties, existence of pH, enzymatic, hydrolysis, etc., sensitive properties when preferred appropriate polymers |
Mass production is difficult, unknown toxicity, and coalescence |
55, 123–131 |
Polymeric micelles |
Acts as penetration enhancers, increased solubility of highly lipophilic drugs, protecting drug from environmental conditions |
Useful only for lipophilic drugs, low drug-loading capacity, dependent on critical micellar concentration |
23, 25, 79, 132–137 |
Dendrimers |
Multifunctional surface modification, target specific drug delivery |
Not suitable for hydrophilic drugs, higher cost, toxicological (hemolysis rates >5%) |
60, 139–145 |
Metallic NPs |
Potential therapeutic and diagnostic tools, stability against oxidation, in vivo degradation, biocompatibility, bioavailability of entrapped drug targeted action, targeted drug action |
Scaling up process is difficult, the use of toxic NPs |
79, 109, 125, 148–166 |
Metallic oxide NPs |
Biocompatibility, stability, and targeted action, potential therapeutic and diagnostic tools |
Exhibited phototoxicity and cellular membrane damage |
34, 168, 169 |
QDs |
Biocompatibility, cellular targeting enhanced specificity, deliver a wide range of bioactive (430–720 nm), imaging tool |
Toxicity of heavy metals |
54, 173, 174 |
Silica NPs |
Biocompatibility, surface functionalization |
Potential toxicity of NPs |
41, 79, 170–172 |
4.1 Lipid carriers
Various types of lipid-based nano-systems, such as solid lipid NPs (SLNs), nanostructured lipid carriers (NLCs), and vesicular nanocarriers, are developed for transdermal drug delivery. They can alter SC barrier integrity by disrupting lipid bilayers, as well as increasing epidermal distribution of drug molecules and hair follicle targeting of lipid nanocarriers.
4.1.1 Lipid carriers.
4.1.1.1 SLNs.
The SLNs made of physiological and biodegradable lipids are widely employed transdermal nanocarriers.60,61 At room temperature, these lipids can create a solid lipophilic matrix into which hydrophilic or lipophilic drug molecules can be incorporated. Structurally, they are spherical and have an estimated mean particle size in the range of 50–1000 nm, with a narrow particle size distribution around the mean particle size.61 Various techniques, including hot and cold homogenization, solvent emulsification, evaporation, microemulsion, W/O/W double emulsion method, and microfluidics, are applied to fabricate SLNs.62–65 As shown in Fig. 4A and B, SLNs can be fabricated in a microchannel system by the liquid flow-focusing and gas displacing method (LFGDM). The size of the fabricated NPs can be tuned approximately in the range of 100–220 nm and 80–210 nm, respectively (Fig. 4C). Furthermore, due to small particle size and hence a larger surface area, these NPs make intimate contact with the superficial junction of corneocyte clusters and channels of the SC.60 This is especially crucial for improving drug accumulation and local drug depot development, which may be utilized for regulated drug administration over time. SLNs also have a specific occlusive characteristic, which may enhance the penetration of drugs through the SC by reducing trans-epidermal water loss. Therefore, SLNs are being extensively researched for therapeutic efficacy via the skin delivery route. Butani et al. designed topical amphotericin B-loaded SLNs to improve the therapeutic antifungal activity. The result demonstrated higher drug accumulation in the upper layers of the skin and minimize the diffusion of the drug from the dermis layer into the systemic circulation.66 The smallest particle sizes are observed for SLN dispersions with a low lipid content (up to 5%). Both the low concentration of the dispersed lipid and the low viscosity offer challenges for the subsequent dermal administration. In most cases, the incorporation of the SLN dispersion in an ointment or gel is necessary in order to achieve a formulation that can be administered to the skin. Besides, cycles of ultrasonication may later the size and stability of the dispersion. This is why sometimes scale-up of SLNs fails even though perfect initial lab results are achieved.
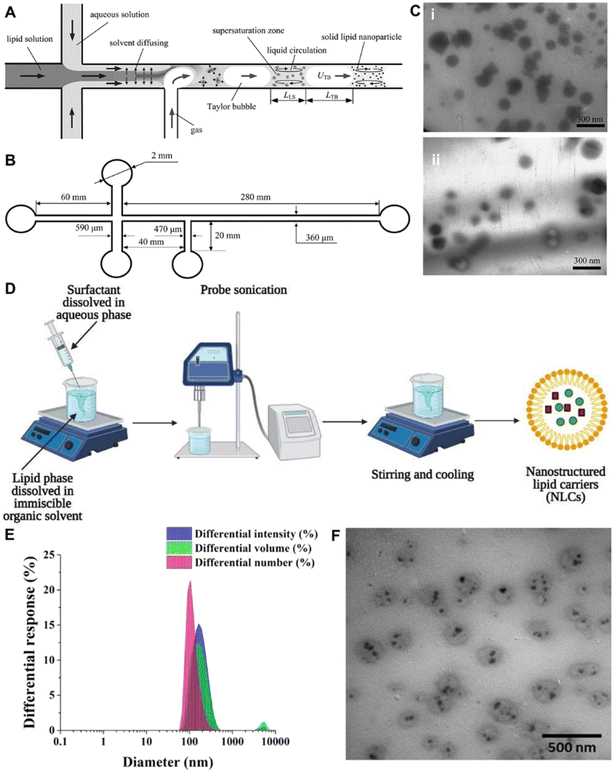 |
| Fig. 4 Preparation and characterization of SLNs and NLCs. (A and B) The preparation of SLNs by LFGDM by microfluidics. (C) Transmission electron microscopy (TEM) photographs of the fabricated SLNs. (D) Schematics of the preparation of NLCs via the emulsification-solvent evaporation technique. (E) Histograms showing the intensity-, volume-, and number-averaged hydrodynamic diameter distributions of simvastatin-loaded NLCs. (F) TEM image of simvastatin-loaded NLCs showing spherical or elliptical vesicles with smooth surfaces. (A–C) Adapted from ref. 64. Copyright 2009 Elsevier. (D) Adapted from ref. 71. Copyright 2021 Elsevier. (E and F) Adapted from ref. 72. Copyright 2021 Elsevier. | |
4.1.1.2 NLCs.
NLCs, as a new improved generation of lipid NPs, are presently being explored extensively.60 NLCs are produced by combining at least one liquid lipid (oil) with one or more solid lipids to form nano-capsules, where the liquid lipid phase can be embedded in a solid matrix or localized on the surface of solid particles (Fig. 4F).67,68 High-pressure homogenization,69 melt-emulsification,70 emulsification-solvent evaporation (Fig. 4D),71,72 ultra-sonication,73 solvent-diffusion,73 and spray drying techniques74 are used in the fabrication of NLCs. The normal weight ratio of solid lipid to liquid lipid ranges from 70
:
30 to 90
:
10.29 Therefore, several types of NLCs are obtained based on the technique of manufacturing and the content of the lipid blend. Moreover, using dynamic light scattering (DLS) and transmission electron microscopy (TEM), the characterized information of nano-systems based on the intensity, volume, number, and morphology can be obtained (Fig. 4E and F). The intensity-, volume- and number-averaged hydrodynamic diameters of NLCs are 185.2, 175.9 and 120.9 nm, respectively. NLCs have a spherical shape. Since, NLCs are smaller in size, they have better surface contact with the SC and consequently increase the number of active compounds penetrated through the skin. Moreover, nano-sized particles may closely attach to the skin surface, allowing for more regulated medication administration. Furthermore, since NLCs provide higher drug loading than SLNs, they can achieve a high drug concentration gradient on the skin surface to facilitate drug permeation.75,76 NLCs have been successfully utilized to deliver drugs via topical routes for improving drug permeation, skin hydration, controlled drug release, and drug stability. NLCs have a remarkably wide range of properties which make them useful for TDDs and have been well investigated to reduce the toxic side effects of the drugs and increase the efficacy of the treatment. However, because of the unpredictable gelation tendency and inherent low incorporation rates resulting from the crystalline structure of the solid lipid, the total number of commercialized NLC products on the market is still limited, which deserves further efforts in advancing the transformation of scientific research.
4.1.2 Vesicular nanocarriers.
4.1.2.1 Liposomes.
Liposomal systems have been demonstrated as one of the promising vesicular nanocarriers in terms of bypassing the epidermal barrier.77 Liposomes are lipid-based microscopic vesicles composed of one or more lipid bilayers arranged in a concentric fashion that enclose the same number of aqueous compartments as biological membranes. Liposomes are generally spherical in form and range in size from 25 to 5000 nm.78 Due to this unique structural composition, hydrophilic drugs can be loaded in the aqueous core while hydrophobic drugs can be loaded in the lipid bilayer (Fig. 5C).79,80 Thin-film hydration,81 reversed-phase evaporation,82 microfluidic methods (Fig. 5A–D),80,83,84 and solvent injection techniques85 are most commonly used conventional liposome preparation techniques. For instance, different types of micromixer cartridges are adopted to facilitate the fabrication of liposomes (Fig. 5A). Particularly, the toroidal mixer with planar geometry and the staggered herringbone micromixer with embossed chevrons employ centrifugal forces to encourage uniform mixing. In addition, the basic T-mixer has two entrances where the liquid is forced into the T-junction and subsequently produces liposomes. Moreover, a rapid single-step preparation method of liposomes using microfluidics is also developed (Fig. 5B). Several theories have been advanced regarding the mechanism of skin delivery via liposomes. Some of the prominent theories include intact vesicular skin penetration,86,87 adsorption effect,87 and the penetration of liposomes through the trans-appendageal route.69,88 However, some researchers have recently suggested that the permeation enhancement effect of liposomes is due to the interaction of liposomes with the skin lipid causing partial fluidization of the skin lipid and consequently delivering the drug to the deeper skin layers (below the SC layer).89–92 Although liposomes facilitate the delivery of drugs to target specific areas of the skin, their therapeutic effects would be overestimated if the toxicity is not considered seriously. Studies showed these liposomes could induce toxic effects like inflammation, myelosuppression, proliferation, and carcinogenesis. Therefore, to further demonstrate the potential of liposomes in transdermal drug delivery, a comprehensive assessment about the potential toxicity of liposomes is necessary.
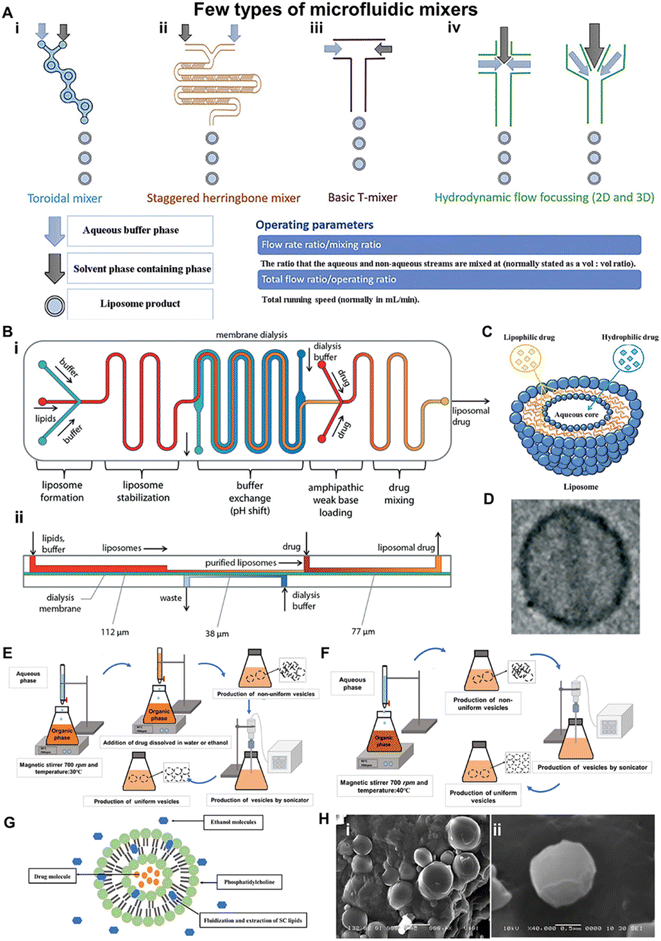 |
| Fig. 5 Preparation and characterization of liposomes and ethosomes for transdermal drug delivery. (A) Various design types of micromixer cartridges for producing liposomes. (i) A toroidal mixer with planar geometry employing centrifugal forces to encourage uniform mixing. (ii) The staggered herringbone micromixer with embossed chevrons. (iii) A basic T-mixer with two inlets where fluids are forced into a T junction and (iv) hydrodynamic flow focusing with three inlets where a central stream of solvent is focused by aqueous fluid streams either in 2D or 3D. (B) Rapid single-step preparation of liposomes using microfluidics. (i) Schematic of the fully integrated microfluidic device for remote loading of liposomal therapeutic nanomedicines in-line with liposome synthesis. (ii) Cross-sectional view of the microfluidic system, revealing the differing channel heights supporting each process step. (C) Schematics of the liposome structure and lipophilic or hydrophilic drug entrapment models. (D) A TEM image of a liposome with a size of 225.5 nm ± 44.8 nm. (E) Preparation of ethosomes by the cold method. (F) Preparation of an ethosome by the hot method. (G) Diagram of the ethosome and ethanol fluidization of SC lipids of skin. (H) Scanning electron microscope (SEM) image of ETC5 ethosmal suspension (i) × 4000 and (ii) × 40 000. (A) Adapted from ref. 83. Copyright 2020 Elsevier. (B and D) Adapted from ref. 84. Copyright 2014 The Royal Society of Chemistry. (C) Adapted from ref. 80. Copyright 2012 American Scientific Publishers. (E and F) Adapted from ref. 85. Copyright 2018 Bentham Science Publishers. (G) Adapted from ref. 105. Copyright 2013 Informa UK Ltd. | |
4.1.2.2 Niosomes.
Niosomes are non-ionic surfactant vesicular systems with size from 100 to 2000 nm.25 The surfactants used for forming niosomes are biodegradable, biocompatible, and non-immunogenic. Niosomes have higher encapsulation efficiency, improved chemical stability, enhanced penetration, and lower cost compared with liposomes.25 Another advantage is the straightforward approach for producing niosomes on big scale niosomes as versatile carrier systems can be administered through various routes, including transdermal delivery.93,94 Examples of transdermal drug delivery using niosomes are minoxidil and ellagic acid. Niosomes have been reported to enhance the residence time of these drugs in the SC and epidermis, while decreasing the systemic absorption of the drug and improving the penetration of the trapped substances across the skin.55 Although there are multiple advantages of the niosomal delivery system, stability is still an important issue since the drug may be hydrolyzed with the aqueous suspension of niosomes. Moreover, the problem of drug leakage from the entrapment site and aggregate formation of niosome may also occur. These all need to be considered when developing a niosomal delivery system for skin treatment.
4.1.2.3 Transfersomes.
Transfersomes are another vesicular system firstly designed in 1992 by Cevc et al.95 These transfersomes have at least one inner aqueous compartment that is surrounded by a lipid bilayer with appropriately tailored properties. Morphologically, transfersomes resemble lipid vesicles, liposomes, but functionally, they are sufficiently deformable to penetrate pores much smaller than their own size.96 Moreover, they are metastable, making the vesicle membrane ultraflexible and highly deformable. Due to their deformable characteristics, transfersomes can penetrate sufficiently through skin pores.97 Cevc et al. proposed that hydrophilic elastic vesicles squeezed into the narrow intercellular paths, causing the intercellular pathways to widen by 20–30 nm.98 The deformable transfersomes were then moved into skin layers through skin microchannels along the transdermal hydration gradient. After that, the transfersomes distributed between corneocytes via intercellular pathways and were found in the subcutaneous tissue.98,99 Despite the fact that the transfersomes' shape and volume adaptation changed transiently the size did not change after crossing the barriers.98 Therefore, the transfersomes are proposed as superior nanocarriers over the conventional lipid vesicles for the enhancement of drug permeation and vesicle–skin interactions.98,100 Furthermore, Duangjit et al. established that meloxicam-loaded transfersomes penetrated the skin by disrupting and fluidizing the SC lipids (acting as penetration enhancers).101 It is worth noting that the use of transfersomes is limited because oxidative degradation makes its predisposition. In addition, the formulation process of transfersomes is expensive without easily accessible.
4.1.2.4 Ethosomes.
Ethosomes are lipid vesicular systems embodying ethanol in relatively high concentrations for superior permeability.102 They are primarily made up of phospholipids, ethanol, and water (Fig. 5G).103 Structurally, ethosomal are composed of a phospholipid bilayer and an aqueous inner core that contains the entrapped active ingredient.104 Ethosomes can be prepared by two conventional methods including a hot method and cold method (Fig. 5E and F).103,105,106 The nano-systems were nearly uniform and of spherical shape as shown in Fig. 5H. Due to the high ethanol concentration, their soft and malleable properties allow them to cross the skin or membrane barrier and affect the degree of transdermal penetration.107 These ethosomal systems, unlike liposomes and transfersomes, can encapsulate either lipophilic or hydrophilic drugs and deliver them into systemic circulation under both occlusive108,109 and non-occlusive conditions.55 In addition, the size of ethosomes is smaller than that of a liposome when prepared under the same conditions, due to the high alcohol content. This reduction in size is due to ethanol imparting a net negative charge to the vesicle surface. Ethosomes have been shown to exhibit high encapsulation efficiency for a wide variety of molecules including lipophilic drugs due to the multi-layered vesicles and the presence of ethanol, which allows for better solubility of many drugs.110,111 Phospholipids and high concentration of ethanol in the ethosomes have created a synergistic mechanism for drug penetration into deeper layers of the skin in transdermal drug delivery.112 Ethanol increases the lipid fluidity of the ethosomal and skin lipid bilayers while decreasing the density of the highly ordered lipids of the SC. The soft flexible ethosome perturbed intercellular lipid layers of the SC penetrate into the deeper skin layers. After the ethosomes fuse with the lipid contents of the SC, the drug diffuses into deeper skin layers or systemic circulation.113 It is important to assess the skin tolerability and safety of topical ethosomes on intact as well as wounded, infected and damaged skin by in vitro and in vivo experiments. Besides, the phospholipid contents of ethosomes are prone to hydrolysis and trigger oxidation, which would lead to stability problems for ethosomes, and therefore an evaluation of the long-term stability of ethosomal systems is required.
4.1.2.5 ISAsomes.
Internally self-assembled somes or particles (ISAsomes) are non-lamellar structured lipid NPs, including cubosomes and hexosomes.114 ISAsomes are nano-self-assemblies, sharing common features with SLNs. Meanwhile, ISAsomes envelope internally non-lamellar liquid crystalline phases (inverse bicontinuous (Q2) and discontinuous hexagonal (H2) phases). They appear inside the kinetically stabilized dispersed particles. Non-lamellar liquid crystalline nanoparticles have typically sizes in the range of 100–200 nm.114,115 These nanosystems are typically formed in the presence of an efficient stabilizer by applying high-energy emulsification methods that include ultrasonication, microfluidization, and high-pressure homogenization.116–118 ISAsomes have unique lyotropic liquid crystalline nano-structural features, making them attractive as nanocarriers for transdermal drug delivery systems.119 They are able to encapsulate active ingredients, to interact with the skin strata after cutaneous administration and to release their content in a controlled fashion. Mohyeldin et al. reported the result that four progesterone-loaded nanocarriers, cubosomes, nanoliposomes, nanoemulsions and nanomicelles, to enhance progesterone penetration via the human abdominal skin.120 All nanocarriers exhibited higher transdermal flux relative to free progesterone in ex vivo skin permeation. Compared with other nanocarriers, cubosomes revealed a higher skin penetration with a transdermal steady flux of 48.57 × 10−2 ± 0.7 μg cm−2 h−1. Al-Zuhairy et al. developed a novel hexosome by mixing lipid-forming liquid crystals (LCs) with petrolatum, to allow for more medication penetration through the skin. In an in vitro skin permeation study, the mixture formulations dramatically enhanced drug skin concentrations compared to the white petrolatum.121 These results showed that ISAsomes will bring about enormous opportunities in transdermal drug delivery.
4.2 Polymeric nanocarriers
In recent years, an increasing number of polymeric nanocarriers for transdermal delivery have been developed.122 Nanocarriers fabricated from biodegradable polymers have the potential to overcome limitations associated with other lipid systems, such as lower drug loading and phase stability issues. Moreover, sustained and controlled release of the drug as well as specific targeting could be achieved over the physicochemical characters of these polymeric nanocarriers.123
4.2.1 Polymeric NPs.
Polymeric NPs range in size from 10 to 1000 nm and are made of biocompatible and low immunogenicity polymers. Drugs can be dissolved, entrapped, encapsulated, or attached in the polymeric matrix.55,124 The preparation of polymeric NPs can be achieved by nanoprecipitation (also called the solvent displacement method),125 various types of emulsification/solvent evaporation,65 and the salting out method.65 For instance, Karnik et al. synthesized poly(lactide-co-glycolide)-b-poly(ethylene glycol) (PLGA-PEG) NPs on a microfluidic platform by nanoprecipitation for a variety of biomedical applications (Fig. 6A and B).125 The prepared spherical nano-systems with diameters typically in the range of 10–50 nm were observed by using TEM (Fig. 6C). Biodegradable polymeric materials like gelatin, CS, albumin, hyaluronic acid (HA), and polylactic acid (PLA) are frequently used.126 One of the most widely investigated natural polymers is CS. The mucoadhesive property and availability of functional groups on the CS molecules permit the conjugation of ligands to promote site-specific targeted action.127 Besides, some of the other benefits of this polymer are biocompatibility, biodegradability, and maximal drug loading capacity.128Although CS has many enticing properties, its low stability limits its usage in pharmaceutical products.129 Some researchers have reported the use of more stable anionic polymers as well as the use of cross-linking agents combined with CS to address this difficulty. The prepared NPs have good stability and skin permeation. For example, Takeuchi et al. explored donepezil hydrochloride (DP)-loaded poly(lactic-co-glycolic acid) (PLGA)-CS core–shell NPs to treat osteoporosis.130 Compared to a DP solution, core–shell NPs improved both skin accumulation and delivery of DP into hair follicles. Moreover, synthetic polymers are also applied to form NPs. Compared to natural biodegradable polymers, the biodegradation process of synthetic polymers is significantly different, taking several weeks to a few years by enzymolysis or chemical and physical cues. For example, hydrophobic PLGA NPs with high skin permeability can be degraded in vivo by scission of ester linkages to produce glycolic acid and lactic acid in several weeks.131 Moreover, most of the polymeric NPs are generally water-soluble. They can present a surface modification (PEGylation) to achieve a selective accumulation.132 However, the limitations like one particular process or technique is not suitable for the encapsulation of all drugs, post-preparative steps, such as purification and preservation, incomplete or discontinuous films, and inadequate stability of certain active components still remain to be solved. Despite these technological challenges, nanoparticles have been showing great promise for the development of TDDs.
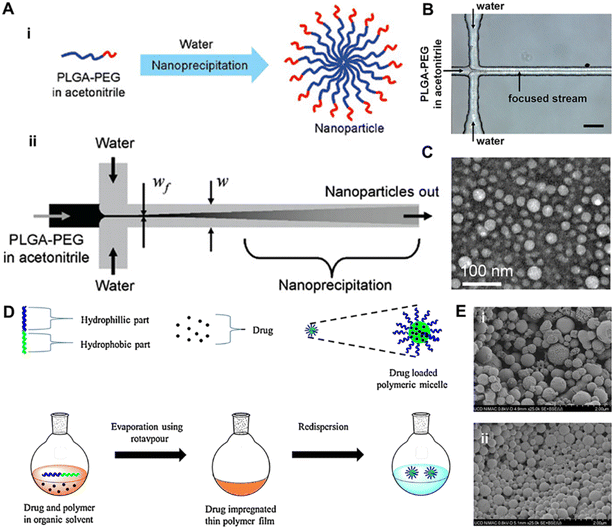 |
| Fig. 6 The synthesis and representation of polymeric nanoparticles and polymeric micelles. (A) The synthesis of polymeric nanoparticles using nanoprecipitation in a microfluidic device. (i) Nanoprecipitation of PLGA-PEG copolymers. (ii) The process of mixing can be carried out in a microfluidic device using hydrodynamic flow focusing, where the polymer stream is focused into a thin stream between two water streams with higher flow rates. Rapid mixing and nanoprecipitation occur due to the diffusion of the solvent out of the focused stream and diffusion of water into the focused stream. (B) A microfluidic device for hydrodynamic flow focusing of polymeric nanoparticles in water. Scale bar 50 μm. (C) TEM image of nanoparticles synthesized by nanoprecipitation of PLGA15K-PEG3.4K by hydrodynamic flow focusing showing a spherical PLGA core–PEG corona structure of the nanoparticles. (D) Solvent evaporation method/solution casting method. (E) Scanning electron microscopy images of polymeric micelles, (i) PLGA-Dex10 and (ii) PLGA-Dex10-curc. (A–C) Adapted from ref. 125. Copyright 2008 American Chemical Society. (D) Adapted from ref. 135. Copyright 2019 Elsevier. (E) Adapted from ref. 139. Copyright 2021, Springer Nature. | |
4.2.2 Polymeric micelles.
Polymeric micelles for transdermal delivery have gained great attention in recent years.133 To reduce surface-free energy in an aqueous medium, these micelles can self-assemble from amphiphilic block copolymers at/above specific polymer concentration (critical micelle concentration) forming a hydrophobic core and a hydrophilic shell.79 The hydrophobic core contributes to both drug solubility and the depot effect, whereas the hydrophilic shell contributes to stabilization.25,134 Moreover, micellar forms such as worm-like, dislike, or sheet are generated dependent on the number of copolymer blocks and their arrangement in the dispersion.23 The polymeric micelles can be generated by using a variety of processes, including the solvent evaporation method (solution casting method) (Fig. 6D), self-assembly method, and solid dispersion method.135–137 Moreover, as an important assay method, SEM can be used to evaluate the morphology of nano-systems. For instance, the results showed that the curcumin-functionalized PLGA-dextran micelles (PLGA-Dex10-curc) have much larger hydrodynamic diameters (498.7 ± 35.4 nm) in contrast with PLGA-dextran (PLGA-Dex10) (113.0 ± 34.0 nm). Meantime, the micelles also seem to have a rougher surface (Fig. 6E). This biocompatible, extremely stable colloidal system with enhanced drug solubilization and localized drug release to the targeted region keeps the polymeric micelles more intact under dermatological conditions, mainly psoriasis, burns, and acne. Polymeric micelles also have the other superiorities of polymeric nanocarriers such as tunable chemical and physical properties.25 However, they have some limitations including the dependence of critical micelle concentrations and low drug-loading capacity.25,138 Polymeric micelles are one of the most promising types of nano-systems for transdermal drug delivery. At present, numerous micelle-based formulations are being developed and are at various stages of clinical trials. We may expect the wide utilization of these nano-systems in practical applications in the near future.
4.2.3 Dendrimers.
Dendrimers are unimolecular, monodisperse, synthetic polymers (generally <15 nm) having symmetrically placed branching units that can from a spherical shape (Fig. 7B).140 These nanocarriers are made up of three parts, a core, internal dendritic branches, and peripheral surface that is functionalized with a land layered structure.141 Conventionally, dendrimers can be synthesized by convergent synthesis and divergent synthesis (Fig. 7A).142–144 For instance, PAMAM-G7 NPs have a spherical shape with a mean diameter size of 20 nm (Fig. 7C). Meanwhile, they also display a multi-layered-like structure, which has smaller spherical substructures. Dendrimers gained significant attention as polymeric skin permeation enhancers that can increase drug transport through the skin.142,145,146 Dendrimers promote drug penetration by increasing drug solubility and enhancing partitioning and diffusion of the drug molecules into the skin.60 The size and surface properties of dendrimers are critical for their dermal applications. The properties of dendrimers or dendrimer–cargo complexes can be modified as needed to enhance or inhibit their dermal penetration, as needed.142 However, there are still issues that need to be addressed before such systems can be translated to the clinic trials. For example, while the oligomeric and dendritic systems are attractive for clinical applications due to their well-defined structures, the syntheses of these molecules require tedious steps and therefore will be complex and costly for scaling up. In addition, the steric hindrance at the dendrimer periphery has thus far limited the molecules to relatively low generations, in turn limiting the degree of amplification that can be achieved.
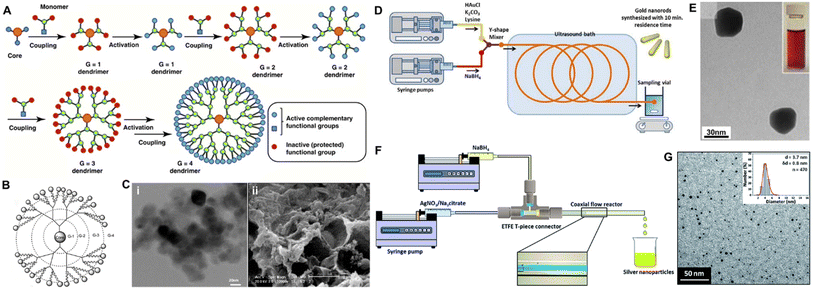 |
| Fig. 7 The synthesis and characterization of dendrimer and metallic NPs for transdermal delivery. (A) Process of forming a dendrimer using the divergent growth method. (B) Conceptional scheme of the PAMAM-G4 dendrimer. (C) Microscopic images of the dendrimer. (i) TEM image of PAMAM-G7; scale bar: 20 nm (ii) SEM image of PAMAM-G7; scale bar: 2 μm. (D) Microfluidic-mediated gold nanorod synthesis. (E) TEM image of gold nanostructures obtained. (F) Schematic of the coaxial flow reactor setup. Inset shows flow visualization of laminar flow inside the coaxial flow reactor with blue dye flowing through the inner tube and water flowing through the outer tube. (G) TEM image and size distributions of the prepared silver nanoparticles. (A) Adapted from ref. 143. Copyright 2016 Elsevier. (B) Adapted from ref. 141. Copyright 2018, MDPI. (C) Adapted from ref. 144. Copyright 2017, BMC Infectious Diseases. (D) Adapted from ref. 147. Copyright 2019 SAGE Publishing. (E) Adapted from ref. 148. Copyright 2012 The Royal Society of Chemistry. (F and G) Adapted from ref. 149. Copyright 2015 The Royal Society of Chemistry. | |
4.3 Inorganic NPs
4.3.1 Metallic NPs.
Metallic NPs possess specially designed structures in which drugs are encapsulated, and surface functionalized physicochemical properties such as higher surface-to-volume ratios, improved ionic conductivity, and aid in crossing barriers to target sites.150,151 These NPs may be generated using chemical synthesis techniques.79,149,152 Moreover, microfluidic technology has been used to facilitate the controllable production of metallic NPs (Fig. 7D and F). Silver or gold NPs are the most commonly employed metallic NPs in the skin delivery industry.153,154
Gold NPs (AuNPs) have been widely explored as promising candidates for transdermal therapeutic applications.155 AuNPs are available in a variety of colors based on their size (between 3 and 120 nm), the oscillations of conduction band electrons at appropriate wavelengths, form, and amount of aggregation. These colors include orange, blue, red, and yellow.156 The size and surface characteristics of AuNPs appear to have an important impact on intracellular uptake and cytotoxicity.157 These carriers can be produced using a variety of technologies, such as microfluidic method,158 and the use of a green chemistry route.159 For instance, anisotropic AuNPs can be fabricated in a Y-shaped channel. AuNPs with irregular shapes and polydispersity were obtained in the reaction channel due to adjustment of the concentration of shape modulating agents (Fig. 7E). They can be coupled with peptides/proteins, nucleic acid (NA) moieties, small drug molecules through either chemical conjugation (thiol derivative bonds and streptavidin linker), or physical interactions (charge and hydrophobic interactions).126 Over the past decade or so, AuNPs have increasingly been utilized in TDDs besides the conventional systemic administration. Recent research has shown that topically administered AuNPs may pass the skin barrier and reach the epidermis and even the dermis layer.160 Correspondingly, various skin-related applications have been proposed. Researchers loaded vascular endothelial growth factor (VEGF) onto negatively charged AuNPs through ionic interaction for skin penetration, stimulating angiogenesis in the dermis layer as verified through α-SMA immunostaining and vessel density.161,162 Likewise, AuNPs received significant attention as transdermal nanocarriers for NA moieties (siRNAs and antisense oligonucleotides), enabling facile and effective gene therapy. In diabetic mice, Randeria et al. demonstrated that ganglioside-monosialic acid 3 synthases (GM3S) targeted spherical nucleic acid (SNA) modulated keratinocyte migration, proliferation, and insulin-like growth factor-1 (IGF1) receptor activity to promote wound healing (Fig. 8A–C).111 Apart from therapeutic applications, the surface plasmon resonance phenomena of AuNPs makes it ideal for diagnostic applications as well (through fluorescence dequenching of molecular probes upon interaction with the target moiety). Using this technique, known as Nano-Flare of Au-based SNAs, small chemicals and mRNA targets, either extracellular or intracellular, can be used as sensors.163,164 Combining SNA skin penetration ability with its mRNA sensing Nano-Flare configuration, Yeo et al. investigated its utilization to monitor connective tissue growth factor (CTGF) mRNA expression of skin fibroblasts, for facile, biopsy-free detection of abnormal scarring (Fig. 8D).165 Besides, CTGF Nano-Flare reported specifically the presence of abnormal fibroblasts on the rabbit and modified ex vivo human skin (Fig. 8E).165 Apart from AuNPs, silver NPs (AgNPs) have emerged as attractive metallic NPs for transdermal therapeutic purposes. AgNPs are particulate dispersions or solid particles with diameters ranging from 1 to 100 nm.166,167 Synthesized AgNPs include spherical, truncated, and triangular with different diameters (2–100 nm) and a higher surface to volume ratio. These NPs are promising candidates for the treatment of bacterial infections due to their potent and broad-spectrum antibacterial properties.168 Although green synthesis of AgNPs is favorable compared to conventional chemical and physical procedures, its simplicity, cost, and time consumption should also be considered. Synthesis procedures using mediated fungus, bacteria, and other organisms are difficult since they involve the isolation and growth of the strains, which are intricate operations requiring several complicated steps. Also, these procedures are problematic in terms of maintaining the culture medium as well as the physical and chemical conditions. Plant extracts have recently been considered because of their ease of extraction, abundant availability, and their potential to eliminate the above-mentioned complicated procedures of cell culture maintenance.
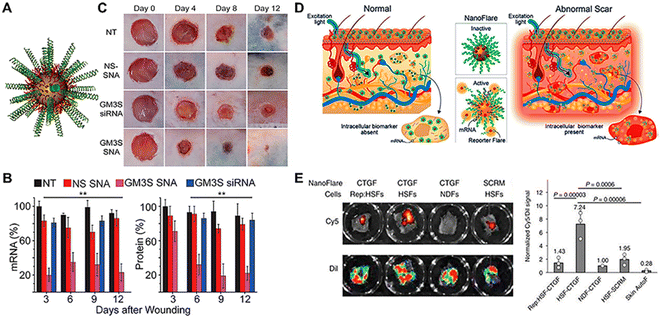 |
| Fig. 8 Topically applied Au-based SNAs for wound healing and early scar diagnosis. (A) Schematic of Au-SNA carrying GM3S siRNA and ethylene glycol (GM3S SNAs). (B) Efficacy of GM3S SNAs in down-regulating cutaneous GM3S mRNA and protein levels in an obese mice model. (C) GM3S SNAs prevent the delayed wound healing in the obese mouse. (D) Early, facile detection of abnormal scarring through topical application of SNA Nanoflare. (E) SNA Nanoflare targeting connective tissue growth factor (CTGF) mRNA revealed the presence of HSFs in ex vivo skin, relative to NDFs or drug-treated HSFs. Adapted from ref. 126. Copyright 2021 American Chemical Society. | |
4.3.2 Metallic oxide NPs.
Metal oxide NPs have gained popularity in the diagnosis and treatment of dermatologic illnesses, as well as in cosmetics, due to their broad spectrum of biological activity and unique physicochemical features.169,170 TiO2 and ZnO NPs are two of the most often utilized metallic oxide NPs. TiO2 NPs and ZnO NPs contributed well in dermatological situations due to their strong photostability and capacity to absorb UV radiation with wavelengths less than 365 and 380 nm, respectively.35 However, the absorption of UV radiation by metallic oxides gives rise to reactive oxygen species (ROS) that exhibited phototoxicity and cellular membrane damage in human keratinocytes which has limited their application.35 Metals such as Ag and Au are favored in the creation of NPs to overcome the aforementioned restrictions. These NPs form a rigid functionalized surface structure with drug entrapment inside the core. They are made in a variety of sizes with customizable surface properties. Besides, the mechanisms of toxicity of metallic oxide NPs still remain largely uncharted. Metal oxide NPs exhibit varying degrees of toxicity, which are noticed less in their bulk counterparts. Before being applied in clinic applications, an evaluation and assessment of the risks and adverse effects are required.
4.3.3 Silica NPs.
Silica NPs, which have a large surface area, well-defined size, customizable pore dimensions, high loading/encapsulation of desirable therapeutic molecules, and stimuli-responsivity, are another type of viable nanocarrier.79 The drugs can be loaded in the pores or on the surface of silica NPs, shielding from enzymes and other compounds in the environment. A sequence of chemical processes was used to create silica NPs with sizes ranging from 0.05 to 2 nm.171,172 Various skin-related uses of silica NPs have been widely investigated. Rancan et al. confirmed the influence of size and surface charge on the penetration capabilities of silica NPs in human skin explants pretreated with cyanoacrylate skin surface stripping (CSSS).41 The size of silica NPs is a crucial factor of translocation across the SC. Hirai and colleagues demonstrated skin penetration and cellular localization of well-dispersed amorphous nano-silica particles with a diameter of 70 nm using TEM. The results suggested that silica NPs can pass the epidermal barrier and are transferred to the lymph nodes after three days of topical treatment on mice in vivo.173 Before the realization of the use of silica NPs in clinical applications, several major challenges should be overcome, including improvement of drug loading, enhancing incorporation efficiency, spatial and temporal control of drug release, and highly efficient targeting of disease sites. A thorough understanding of the toxicity profile is not only a scientific concern but also a prerequisite for the clinical application of silica NPs. Nevertheless, by integrating silica NPs with other systems such as polymers and small molecule materials, it is expected that more strategic and scale-up production of “intelligent” and composite nano-reagents will be achieved.
4.3.4 Quantum dots (QDs).
QDs are nanoscale crystals constructed from semiconductor materials with a core, shell, and coated surfaces. The quantum effect of light absorption or emission is caused by the presence of semiconductors in the core (CdSe), and fluorescence effects and photostability are the functions of the shell (ZnS).54,79 Thus QDs have fluorescence features that allow them to be tracked inside the skin. Since Cd was discovered inside the liver and kidney after skin exposure, Tang et al. demonstrated QDs can penetrate and permeate through rat skin, while raising concerns about QD's toxicity.174 Wang et al. demonstrated that QDs can enter the skin, especially when it is injured or prepared with UVB rays, and Cd can diffuse into internal organs.175 For further development of QDs, researchers need to find commercially available and effective methods to fabricate various QDs. Multiple devices and applications can benefit from QDs because of their special optical and electrical properties. Promising strategies have been provided by elaborate designs of micro-structures and device structures for practical applications and future research. However, the research on QDs is still in its early stage compared to graphene. There is still a long way to go for extensive practical applications, and there is also a wide space of exploration for researchers.
5. Evaluating the transdermal capacities of nano-systems
In the past, the transdermal passage of nanoparticles, or the capacity of nanoparticles to promote the passage of drugs to the systemic circulation, is a rather controversial field.176 However, the evaluation of the enhanced transdermal drug delivery facilitated by functional nano-systems has been addressed using a variety of microscopic, spectroscopic, and structural methods. Label-based or label-free instrumental approaches can be used to characterize the characteristics of functional nano-systems and their penetration into the skin. Diffusion cell method, TEM/SEM, cryo-electron microscopy (Cryo-EM), soft X-ray spectromicroscopy, Raman microscopy, atomic force microscopy (AFM), surface enhanced Raman scattering (SERS), and Fourier transformed infrared (FTIR) and infrared (IR) microscopy are label-free methods. Meanwhile fluorescence spectroscopy (intensity-based, spectrally-resolved, time-resolved, and raster scanning-based correlation techniques), single-molecule fluorescence microscopy, and electron paramagnetic resonance (EPR) techniques are examples of label-based techniques. The sensitivity, specificity (information content), geographical resolution, and technological requirements of these approaches differ. This paragraph will provide an outline of the various techniques for evaluating the transdermal capacities of nano-systems.
5.1 Label-free technique
5.1.1 Diffusion cell method.
The diffusion cell method is the gold standard in the evaluation of TDDs, which can provide numbers of quantifiable data to support the capacity of NPs to cross the epidermal layer.177,178 For example, Özcan et al. designed lecithin–chitosan NPs to investigate the topical delivery of quercetin. In an in vitro percutaneous study, compared with the quercetin solution, NPs could significantly enhance the cumulative amounts of quercetin in the dermis and epidermis within 12 hours of application (P < 0.05), and the amounts of quercetin in the epidermis and dermis were 9.00 ± 0.40 μg and 3.31 ± 0.51 μg, respectively, which were 1.45 and 1.32 times those of the control formulation. In vivo, quercetin extracted from the epidermis and dermis was analyzed by HPLC at every sampling point. For the nanoparticles, the amounts of quercetin in the epidermis and dermis were 8.4 ± 1.53 μg and 2.21 ± 0.01 μg, respectively, which were 2.3 and 1.2 times those of the control formulation. The permeation behavior of quercetin in vivo was similar to that in vitro (P < 0.05). Özcan et al. synthesized diflucortolone valerate (DFV) loaded lecithin/chitosan NPs to evaluate if the in vitro accumulation of clobetasol-17-propionate would improve the efficacy in vivo.179 It produced a significantly higher amount of DFV in the SC + epidermis and dermis in comparison with the tested dosage forms (P < 0.05). The results indicated that these nanoparticles showed 6.33- and 5.79-fold higher retention compared with commercial cream for DFV in SC + epidermis and dermis, respectively.
5.1.2 TEM/SEM and AFM.
TEM, CryoEM, and SEM are routinely used to evaluate the shape and surface morphology of NPs, and also be utilized to investigate nanoparticle localization in skin tissue.122 TEM is sensitive to electron-dense spots in the material and offers good pictures of skin slices (human skin and murine skin), particularly for metallic or silica NPs with ultrastructural spatial resolution down to 0.1 nm.32,180 SEM, which employs a raster scan of an electron beam over a surface, in conjunction with an X-ray microanalyzer that is sensitive in the excited volume element, enables simultaneous viewing and elemental analysis of the specimen.32
5.1.3 AFM.
AFM “touches” the surface of the sample with a mechanical probe to characterize its morphology and record its mechanical properties.181 AFM provides information on height differences in the nm range. AFM has recently become an important tool for studying the micro/nanoscale properties of skin and nanosystems. For example, clusters of dispersed NPs (particle dimension: 37.5 nm width at half height) in the dermal collagen network were visualized in human skin cryosections using this technique.182 In addition, AFM can provide insights into the electrical properties of nanoscale data, leading to a better understanding of the mechanisms of how nanosystems interact with and influence skin electrical properties. This is because the change of charge is proportional to the contact surface potential. When the surface electric potential changes, the charge will change accordingly.
5.1.4 X-ray spectromicroscopy.
X-ray microscopy is a potent label-free technology that uses the resonant excitation of the target samples (polymers or drugs) in the soft and hard X-ray regimes.183 For example, the medication dexamethasone's ex vivo penetration profile in human skin can be achieved by using X-ray microscopic scanning of skin sections.184 The absorption of the drug (here at the oxygen K-edge at 530.6 eV) can be selectively measured and visualized in the image. The concentrations of dexamethasone as low as 1–2 μg cm−2 were detected in human skin.184
5.1.5 Vibrational spectroscopy and microscopy including IR, FTIR, Raman, and surface enhanced Raman scattering (SERS).
The vibrational states of molecular bonds can be reveled via the absorption in the infrared spectral region. Accordingly, information on molecular composition and structural organization of lipid lamellar phases becomes available. In vivo studies of nanocarrier penetration and drug release were carried by collecting various layers of the SC using the tape stripping technique. Combining FTIR185 and FTIR photoacoustic spectroscopy, the quantitative distribution patterns in the human SC can be obtained.186 Furthermore, FTIR measurements on isolated SC sheets can provide direct information on skin hydration, protein, and lipid composition as well as secondary protein structures.187 The lateral organization and conformational ordering of the lipids in the sample can be observed via the methylene scissoring and stretching modes.188 However, because of the significant IR absorption of water in vivo IR spectroscopy investigations are only sensitive to the epidermis. Since Raman-active bonds can vary their polarizability when excited with a certain frequency of light, Raman spectroscopy can enable selectivity to specific bonds. While the intensity of the Raman peak is directly proportional to the concentration of the respective molecule, non-invasive detection and quantification of Raman-active compounds in the skin are feasible in vivo.189,190 When employing Raman micro-spectroscopy, this label-free technology also delivers pictures and direct visualization (resolution down to ∼1 μm), together with chemical analysis, and it may be utilized for in vivo applications on human skin.191–193 For in vivo confocal Raman studies, water profiles, SC thickness, and penetration profiles of hydrophilic compounds with a depth resolution of 5 μm were described.191 By deconvolution with the known model spectra, the penetration profiles of Raman-active nanoparticles may be derived from the overlapping Raman spectra of the NPs and the skin compounds, such as cholesterol, ceramide, keratin, urea, water, and other skin compounds.193 Moreover, SERS is a sophisticated label-free imaging technique based on molecular vibrational spectroscopy with resolutions down to the submicrometer region, that have been applied for the detection of nanoparticles and drugs in the skin (porcine skin and murine skin).193,194
5.2 Label-based techniques
5.2.1 Fluorescence spectroscopy.
Fluorescence spectroscopy can be used to track skin penetration in vivo by exciting fluorophores connected to the nanocarrier (normally in the UV to near IR spectral range).195,196 Endogenous fluorophores (porphyrins, nicotinamide adenine dinucleotide (NADH), collagen, and elastin), fluoresce pharmaceuticals, and exogenous fluorophores are examples of these fluorophores. For example, the transport capacity of zinc(II) phthalocyanine (ZnPc)-loaded small NPs in the skin has been investigated using fluorescence spectroscopy (Fig. 9A–C). The distribution of ZnPc-NPs/(2-hydroxypropyl)-β-cyclodextrin (HPβCD) in different skin layers can be observed (Fig. 9C). Besides using fluorescence intensity or the emission wavelength as a readout, fluorescence lifetime and time-resolved anisotropy can be employed. Fluorescence lifetime is highly sensitive to the environment and therefore allows, among others, sensing of polarity, pH, calcium concentration, or biomolecular interaction. The analysis of time-resolved anisotropy provides insights into the molecular dynamics of the nanocarrier.197,198
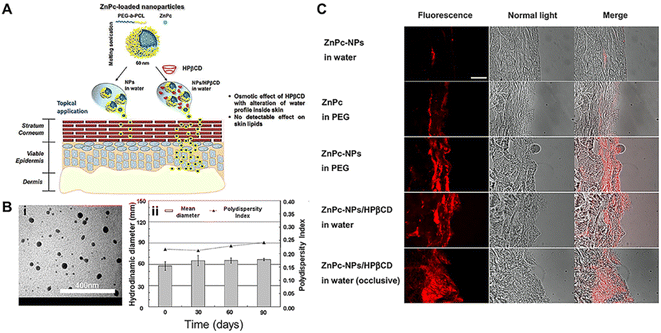 |
| Fig. 9 Preparation and evaluation of polymer NPs through the skin. (A) Poly(ethylene glycol)-poly (ε-caprolactone)-amphiphilic diblock copolymer (PEG-b-PCL) NPs coupled with (2-hydroxypropyl)-β-cyclodextrin (HPβCD) NPs as a novel vehicle for the transdermal delivery of highly lipophilic compounds. (B) The evaluation and characterization of NPs. (i) TEM image of ZnPc-NPs (0.2% theoretical loading) and (ii) colloidal properties of freeze dried ZnPc-NPs/HPβCD stored in the dark at 4 °C after dispersion in water. (C) Fluorescence images of skin sections after 24 h of permeation experiment. ZnPc red fluorescence can be observed. Merged pictures were obtained by superimposing brightfield and fluorescence images of the same area. Scale bar: 500 μm. Adapted from ref. 196. Copyright 2015 Elsevier. | |
5.2.2 Single molecule microscopy.
Studying fluorescent molecules in the skin at the single-molecule level is challenging. To circumvent this, single fluorescently labeled NPs are studied using multiphoton microscopy.199 Recently, a single particle tracking based approach was developed using ex vivo skin combined with tape stripping to visualize and determine the diffusion constants and spatial confinements of diffusion (penetration pathways) of a model drug in the SC with nanometer resolution at a depth of about 2–4 μm.200 This technique may also be used to apply nanocarriers and pharmaceuticals to the skin in vivo.
5.2.3 EPR spectroscopy.
EPR spectroscopy can detect unpaired electrons in paramagnetic molecules and can be subsequently utilized to investigate the ex vivo and in vivo penetration of hyperbranched nano-transporters or nano-structured lipid carriers in porcine skin using spin-labeled molecules as cargo.201 The change of the EPR signal within the skin is used to track the penetration. In addition, free radicals are spontaneously produced in organisms as they are essential for various metabolic processes. ROS, containing one or two unpaired electrons, may be overproduced under stress situations (nanoparticle uptake). Therefore, cytotoxicity and antioxidant status may be measured using EPR spectroscopy.202
6. Applications of transdermal functional nano-systems for treating skin diseases
As a novel strategy, functional nano-systems have improved the penetration of drugs across the SC, and enhanced target-specific transdermal drug delivery.92 The use of nano-systems in various skin diseases, such as infectious skin diseases and wound healing, skin inflammation, and skin cancer (atopic dermatitis and psoriasis), has resulted in better therapy.
6.1 Skin infection and wound healing
The skin, as the body's defensive barrier, is vulnerable to external micro-organism infection such as bacterial and fungal infection.
Fungi like Candida, Aspergillus, and Tinea can invade and colonize the dead tissue of the SC, leading to red, scaly, and itchy skin. Fungal infections can be superficial or invasive. Nano-systems have been found to be beneficial in the treatment of fungal skin diseases like tinea versicolor. Recently, novel SLNs were developed to release the antifungal agent Fluconazole (FLZ) for the treatment of Pityriasis Versicolor (PV), a type of fungal infection caused by Malassezia species in the SC.203 Two formulations of FLZ-SLN topical gels were explored, with release capacities of 50 and 80% of the encapsulated drug, respectively. A randomized controlled clinical trial (RCT) on 30 well-diagnosed PV patients was performed and the results were compared with the commercialized product Candistan®. The trial lasted 4 weeks, and the FLZ-SLNs were administered twice a day to the afflicted region. The results showed that by employing FLZ-SLNs, the cure rate for fungal infection can be increased to 99% after full therapy. While Candistan® could only eliminate 80% of the whole fungal infection (Fig. 10A–D).
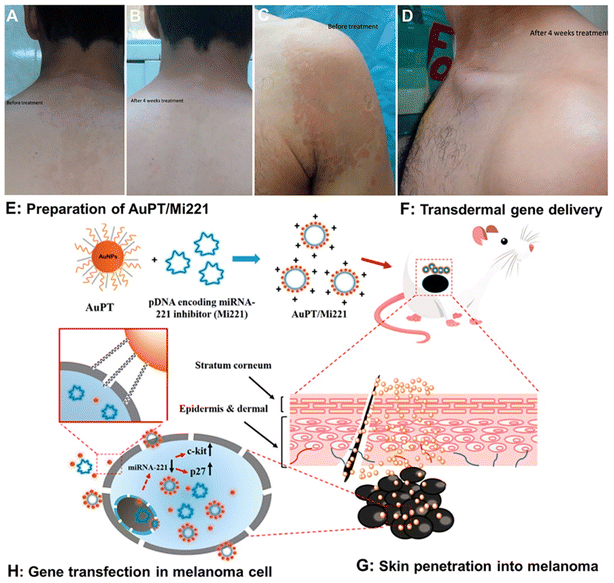 |
| Fig. 10 Illustration of the application of nano-systems in skin infection and skin cancer. (A) Before treatment, (B) after 4 weeks of treatment, (C) before treatment, and (D) after 4 weeks of treatment using FLZ-SLNs. The AuPT NP mediated skin cutaneous melanoma treatment consists of four different steps, including (E) preparation of AuPT/Mi221 nanocomplexes. (F) Topical application of AuPT/Mi221 and the skin penetration of AuPT/Mi221. (G) Skin penetration into melanoma, and (H) gene transfection of AuPT/Mi221 in melanoma cells for tumor therapy. (A–D) Adapted from ref. 203. Copyright 2017 Taylor & Francis Group. (E–H) Adapted from ref. 227. Copyright 2017 American Chemical Society. | |
Bacterial species such as Staphylococcus aureus (S. aureus) and streptococcus pyogenes can infiltrate either the intact skin (Impetigo and Cellulitis) or wound skin.204 Various functional nano-systems have been developed for topical drug delivery to the skin against bacterial infections. Salatin et al. designed a novel nano-platform that incorporates polymeric NPs in thermosensitive hydrogels, which offer distinct advantages such as improved tissue localization, minimized burst release, and controlled drug release.205 The results of the extensive ex vivo and in vivo experiments revealed the possible utility of the cephalexin nanohydrogel for transdermal drug delivery. Besides, the discovery of nanocarriers brings about new concepts for wound healing, since metal NPs or polymeric NPs might be coupled with dressing materials by means of coating or other methods. They have been intensively explored to serve as antimicrobial compounds or to supply endogenous molecules and bioactive compounds to promote the healing process as well as protecting the wound sites from infections. For instance, a CS-Au@2-mercapto-1-methylimidazole (MMT)/gelatin wound dressing was developed to treat multiple antibiotic-resistant bacteria related wound infections. In the study, CS and MMT capped AuNPs showed a synergistic effect and skin was close to full recovery after 16 days of treatment for a rabbit dorsal wound model.206 Moreover, a functional nano-system can overcome existing drug resistance mechanisms, including decreased uptake and increased efflux of the drug from the microbial cell, biofilm formation, and intracellular bacteria.207 Liu et al. developed surface-adaptive, pH-responsive mixed-shell polymeric micelles (MSPMs), which penetrate and accumulate in a staphylococcal biofilm to kill bacteria over the depth of the biofilm.208 As demonstrated for fluorescent Nile red loaded micelles using confocal-laser-scanning-microscopy, MSPMs could be demonstrated to penetrate and accumulate in a biofilm compared with single-shell-polymeric-micelles. The two-dimensional nano-system with atomical thickness and exceptional properties has demonstrated great promise as a therapeutic target for bacterial wound infection.209,210 For example, Zheng et al. designed a hybrid thermo-sensitive hydrogel (Cip-Ti3C2 TSG) by incorporating Cip-Ti3C2 nanocomposites into the network structure of Cip-loaded hydrogels to effectively trap and kill bacteria. In vitro antibacterial results showed that the CIP-Ti3C2 nanocomposites achieved high-efficiency sterilization (>99.99999%) (7.03 log
10) for the inhibition of methicillin-resistant Staphylococcus aureus (MRSA) by combining photothermal therapy with chemotherapy. In an MRSA-induced murine skin infection model, the hybrid hydrogel simultaneously achieved high-efficiency sterilization and long-term inhibition effects, avoiding the rebound of bacteria after photothermal therapy.211
6.2 Skin inflammation
The most prevalent relapsing and chronic inflammatory skin disorders in the world are atopic dermatitis (AD) and psoriasis.212 Many causes, including impaired skin barrier function, microbial colonization, diet, and other irritants, can trigger the inflammation.
AD is typically triggered during childhood with severe eczematous lesions. Corticosteroids and calcineurin inhibitors are considered as the gold standard to treat AD, and functional nano-systems can improve their transdermal penetration. For instance, with the aid of the hydrating effect, liposomes show efficient therapy of atopic dermatitis on the SC. Eroğlu et al. investigated betamethasone valerate (BMV) and diflucortolone valerate (DFV)-loaded liposomes and incorporated into CS gel. The developed liposome/NP gel formulation inhibited atopic dermatitis on paw in rats more effectively than commercial creams.213 Similarly, Asad et al. explored nano-systems for NLC-based gel to treat AD. The anti-inflammatory activity of NLC gel showed a rapid onset of action, as well as a prolonged duration of action as compared with a marketed gel.214
Psoriasis is a recurrent, chronic inflammatory condition marked by red heavily scaled plaques.215 A broad range of treatments involving systemic therapy, topical therapy, and phototherapy are conducted. However, these techniques can only alleviate the disease process and the therapeutic effects are limits. Therefore, to get enhanced treatment benefit, nano-systems are applied to work against psoriasis.216 For instance, SLNs based on trehalose monooleate were fabricated using a microemulsion technology and utilized to administer cyclosporin-A, which can reduce systemic side effects and increased the amount of the cyclosporin-A level in the SC and dermis compared to the control, and therefore promote the therapeutic effects.217 In a more recent study, Fathalla et al. prepared various liposomal and ethosomal preparations loaded with anthralin to enhance efficacy against psoriasis. The clinical efficacy and safety of liposomal and ethosomal Pluronic®F-127 gels were evaluated in patients having psoriasis (https://clinicaltrials.gov/identifier is NCT03348462). Safety was assessed by recording various adverse events. The results showed, at the baseline, that the patients had a median Psoriasis Area and Severity Index (PASI) of 3.4 for liposomes and 3.6 for ethosomes without a significant difference. After treatment, the mean PASI change was −68.66% and −81.84% for liposomes and ethosomes, respectively, with a significant difference in favor of ethosomes. No adverse effects were detected in both groups. Study findings suggest that anthralin ethosomes could serve as a potential treatment of psoriasis.218 In order to better promote the percutaneous absorption of a drug or make the drug arduous to diffuse into the skin by passive diffusion, hybrid systems such as nanoparticles and hydrogels, nanoparticles and microneedles gained favorable progress in enhancing the efficacy of nanomedicines.43,219,220 Moreover, Asad MI et al. developed a pH-sensitive polymeric NP loaded hydrogel to act against psoriasis. Compared with a free methotrexate (MTX) hydrogel and marketed cream, a significant decrease in the Psoriatic Area and Severity Index (PASI) score was observed from day 10 from the group of mice treated with the MTX-NP loaded hydrogel. An improvement in the normalcy of mice skin was observed.214 Chen et al. evaluated the effect of a triptolide-loaded liposome hydrogel patch (TP-LHP) combined with a microneedle array in percutaneous penetration and pharmacokinetics and pharmacodynamics.221 The pharmacokinetic results showed that TP-LHP assisted by microneedles yields plasma drug levels in line with the one-compartment open model. The results of the pharmacodynamic study indicated that TP-LHP can reduce the degree of joint swelling and inhibit the utterances of fetal liver kinase-1, hypoxia-inducible factor-1α in the synovium, and fetal liver tyrosine kinase-4.
6.3 Skin cancer
The three most common kinds of skin cancer are basal cell carcinoma, squamous cell carcinoma (SCC), and melanoma.126 Despite the fact that SCC is the most frequent skin cancer, malignant melanoma is the leading cause of mortality. Located in the bottom layer of the epidermis, melanoma has high metastasis and lethality.222 Chemotherapy, radiotherapy, or immunotherapy is currently used to treat melanoma; however these treatments face drawbacks like tumor reoccurrence and toxic side effects.223,224 Thus, functional nano-systems can act as an alternative for treating skin cancer.225 Usually, a functional nano-system after intravenous injection circulates throughout the body and accumulates in the tumor passively through an enhanced permeation and retention (EPR) effect.226 However, with TDDs, in comparison with the passive targeting method, there is a possibility for more targeted drug delivery to cells and tissues. For example, Niu et al. developed a cell-penetrating peptide and cationic poly(ethyleneimine) conjugated gold nanoparticle (AuPT) loaded pDNA system (AuPT/Mi221) to inhibit the encoding of miRNA-221. As an efficient carrier of pDNAs, AuPT improved the transfection efficiency and reversed the progression of melanoma (Fig. 10E–H).227 Moreover, Das et al. designed apigenin (Ap) (a dietary flavonoid) loaded in poly (lactic-co-glycolide) nanoparticles (NAp) as the therapeutic agent of skin melanoma, in which nano-apigenin targeted nuclear DNA and promoted the accumulation of ROS.228 Jiang et al. designed a paintable oligopeptide hydrogel with paclitaxel (PTX)-encapsulated cell-penetrating-peptide (CPP)-modified transfersomes (PTX-CTs) to enhance transdermal PTX delivery for topical melanoma treatment.229 Comparted with the PTX-CT solution, PTX-CTs/Gel can be painted as a patch on the skin above the melanoma with a prolonged retention time. Due to the superior deformability of the transfersomes, the PTX-CTs are able to efficiently extrude through the gaps in the SC into the epidermis where the melanoma cells are located, further enhancing the skin permeation. The PTX-CTs were shown to effectively slow the tumor growth in combination with the systemic chemotherapy using taxol, the commercial PTX formulation on the xenograft B10F16 melanoma mouse model (Table 3).
Table 3 The application of functional nano-systems for transdermal drug delivery and treatment of skin diseases
Skin disease |
Nano-system |
Composition |
Therapeutic effects |
Fungal infection |
SLNs |
Lipids, poloxamer 407, cremophor RH 40 |
The developed FLZ-SLN topical gels have a superior significant fast therapeutic index in the treatment of PV over commercially available Candistan® cream192 |
Bacterial infection |
Nano-hydrogel |
CS, cephalexin, pluronic®F-127 |
The promising data obtained from the comprehensive ex vivo and in vivo tests demonstrated the potential use of the cephalexin nano-hydrogel for topical drug delivery194 |
AuNPs |
CS, 2-mercapto-1-methylimidazole |
The in vivo rabbit wound healing model revealed that the CS-Au@MMT/gelatin dressing possesses significant antibacterial potential against methicillin-resistant S. aureus-associated wound infection195 |
Polymeric micelles |
Poly(ethylene glycol), poly(β-amino ester) |
Killing bacteria in their biofilm mode of growth, bypassing biofilm recalcitrance to antimicrobial penetration197 |
Two-dimensional functional nanomaterials |
Ti3C2 MXene |
Provides an example of efficient MXene-based antimicrobials to treat localized bacterial infections such as methicillin-resistant Staphylococcus aureus (MRSA)-induced skin abscess200 |
Atopic dermatitis |
Liposomes |
Betamethasone valerate, diflucortolone valerate |
TEWL and erythema measurement results showed that liposomes might be an effective and safe carrier for corticosteroids in skin disease treatment202 |
NLCs |
Liquid lipids (oils) |
The anti-inflammatory activity of NLC gel showed a rapid onset of action, as well as a prolonged duration of action as compared with the marketed gel203 |
SLNs |
Cyclosporin-A, trehalose monooleate |
SLNs based on trehalose monooleate were fabricated using the microemulsion technology and utilized to administer cyclosporin-A, which can reduce systemic side effects and increased the amount of the cyclosporin-A level in the SC and dermis compared to the control206 |
Psoriasis |
Polymeric NPs |
Polymers (E100), surfactants (PVA) |
In vivo results showed that psoriasis was induced in the mice model and successfully treated, which was also confirmed by H & E staining203 |
Ethosomes |
Pluronic®F-127, anthralin |
Anthralin ethosomes could serve as a potential treatment of psoriasis218 |
Liposome |
Lecithin, cholesterol, triptolide |
The combination of TP-LHP and microneedle technology could provide a safe and efficient administration method of triptolide for treating RA221 |
Skin cancer |
AuNPs |
Plasmid DNA, twin-arginine translocation peptide |
Penetrating peptide conjugated cationic gold nanoparticles offer a promising vehicle for both the skin penetration and transfection of pDNAs, possessing great potential in topical gene therapy227 |
Polymeric NPs |
Poly (lactic-co-glycolide), apigenin |
Therapeutic efficacy of NAp can be enhanced by PLGA nanoparticle formulations to have better ameliorative potential in combating skin melanoma228 |
Transfersomes |
Cell-penetrating-peptide, paclitaxel |
The PTX-CTs were shown to effectively slow the tumor growth in combination with the systemic chemotherapy using taxol, the commercial PTX formulation on a xenograft B10F16 melanoma mouse model229 |
7. Conclusion
Transdermal drug delivery is one of the least intrusive and patient-friendly ways for therapeutic agent administration. It can not only boost medication bioavailability by concentrating drug molecules in a particular skin region, but also limit the possibility of unforeseen adverse effects. Various formulations of TDDs have been developed, while their application is still limited due to the low drug distribution efficiency through the skin, which is mainly caused by the inherent skin barrier function. Recently, functional nano-systems as one of the most promising strategies to treating skin diseases have attracted a great deal of interest with significant progress achieved.
8. Outlook
The skin layer is the intrinsic barrier to impede the delivery of nanosystems into the deep skin tissue. Novel functional nano-systems open new perspectives for promoting transdermal drug delivery and treating skin diseases. Although significant achievements have been obtained, there is still a wide space of exploration for researchers.230–233 Here in this section, we offered a comprehensive outlook of formulation strategies point-by-point to further highlight the future directions of nanotechnology-based on skin therapy, including: (1) developing novel nano-systems with more sophisticated structures and shapes; (2) expanding the scope of materials used for developing novel nano-systems; (3) developing novel nano-systems with minimized toxicity effects; (4) executing more deeper and comprehensive investigation over the specific skin penetration mechanism for different nano-systems.
8.1 Developing novel nano-systems with more sophisticated structures and shapes
Available reports focus on size-dependent skin penetration of NPs, mainly through follicular pathways. However, advancements in developing different structured nano-systems have facilitated their applications in transdermal delivery.234 For instance, Tak et al. demonstrated that the skin penetrating capabilities of AgNPs show shape-dependence.234 Franz diffusion cell system was used in vitro to study the skin penetrating capabilities of AgNPs with different shapes. The amount of silver permeated through skin to the receptor cell at different time intervals was measured using inductively coupled plasma-mass spectrometry (ICP-MS). AgNPs showed shape-dependent permeation through skin. After 12 h the amount of silver penetrated from rod-shaped nanoparticles (RNPs), spherical nanoparticles (SNPs) and triangular nanoparticles (TNPs) through the unit area of skin was 1.82, 1.17 and 0.52 μg cm−2. An in vivo study using SKH-1 hairless mice showed varying skin penetration rates of differently shaped AgNPs in plasma-mass spectrometry (ICP-MS) analysis, when systemic circulating blood samples were analyzed after five days of treatment. RNPs showed the highest concentration in blood of 108.57 ± 5.43 ng mL−1, whereas the concentration of silver permeated from SNPs and TNPs in blood was 50.00 ± 2.50 ng mL−1 and 39.29 ± 1.96 ng mL−1, respectively. The consideration of the shape dependency of nanosystems in skin penetration capabilities could help develop an ideal topical agent with the highest efficacy and low systemic toxicity.
8.2 Expanding the scope of materials used for developing novel nano-systems
As the basic skeleton unit of nanocarriers, materials are one of the sources of unique functions of nanosystems.18,206,235 At present, nature provides us with a variety of materials, from metal to non-metal, and from organic to inorganic. For the reasonable choice of materials, it can make our designed work of art stand out. Drug resistant bacteria are a headache in the medical field. At present, researchers combine antibacterial materials with antibiotics/non-antibiotics to achieve dual antibacterial effects.236,237 For example, the interaction between the positive charge in the chitosan molecule and the negative charge in the microbial cell membrane leads to the leakage of bacterial proteins and other cell components, resulting in the antibacterial effect. The combination of the two will greatly improve the antibacterial effect.237,238 Chemical excipients such as ethanol and glutaraldehyde are often used in the formula, which may cause skin irritation, allergic reaction and dermatitis, and are widely criticized. Therefore, materials with good biocompatibility have attracted more attention. For example, Zhang et al. developed a new TDDS for the treatment of hypertension using atenolol (ATE) based on poly (acrylic acid) (PAA)-decorated three-dimensional (3D) flower-like MoS2 nanoparticles (PAA-MoS2 NPs) that respond to NIR laser irradiation. The skin irritation assay of PAA-MoS2 was performed by using a rabbit model. In vivo skin erythema index indicates that PAA-MoS2 can be applied safely in clinics without causing irritation.239
8.3 Developing novel nano-systems with minimized toxicity effects
At present, most investigations of functional transdermal nano-systems are at the pre-clinical stage and far from being applied in clinical trials, which leaves large space and deserves more deep and systematic studies. For instance, since nanomaterials display active interactions with the skin barrier, the assessment of nano-systems’ toxicity is very important. However, most research focuses on in vitro toxicity using the coculture system of human fibroblasts and keratinocytes. The methylthiazoltetrazole (MTT) test is commonly used to determine the toxicity of curcumin-Cs NPs when incubated with HaCaT keratinocytes.240 Biodegradable self-assembling gelatin/epigallocatechin gallate (EGCG) nanoparticles (GE NPs) were demonstrated for good biocompatibility. In this study, cell viability assays indicated that 10 μg mL−1 concentrations of EGCG or GE were non-toxic to human dermal fibroblast (WS1) cells.241 Moreover, Au-NPs with 5 nm at a concentration up to 200 mM were shown to be safe to keratinocytes, since materials with cell viability more than 80% are often considered as biocompatible.242 While cell-based in vitro research is important to determining the toxicity toward specific cells or tissues, in vivo experiments are essential to test their systemic toxicity for the organs and the whole body. Besides, chemical excipients such as ethanol are used in the formulation of nano-systems, which could cause skin irritation, an allergic reaction, and dermatitis. Therefore, the toxicity effects of nano-systems need to be evaluated by using rabbit and rat models. For example, the skin irritation assay of triptolide (TPL) loaded NPs was performed by using a rat model. The result showed that TPL-NPs can be applied safely in clinics without inducing irritation.243
8.4 Executing more in-depth and comprehensive investigation over the specific skin penetration mechanism for different nano-systems
The specific skin penetration mechanism for each type of nano-system deserves more in-depth and comprehensive investigation. Currently, the transdermal mechanism for some types of nano-systems has been revealed, but for most of the types, their specific mechanism remains unclear. The diffusion cell method is the gold standard in the evaluation of TDDs, which is the most commonly used.177,178 Patlolla et al. demonstrated size-dependent penetration by using TEM, with AuNPs (15 nm) effectively reaching a deeper region of the skin layer whereas larger particles were observed mainly in the epidermis and dermis. Moreover, one solution would be to label nano-systems with fluorescent dyes, which can be used to monitor the penetration process with the help of confocal imaging. For instance, the inclusion of the transactivating transcriptional activator (TAT) peptide on the surface of NPs enables better binding to the SC through its positively charged groups, enhancing NP occlusion and facilitating passage into the SC.244 Furthermore, while skin penetration of nano-systems can be achieved, the precise control over the penetration depth still needs investigation. The physicochemical properties of nano-systems and their composition may influence their penetration depth. For example, the different sizes of AuNPs are used to target different areas in the skin.40 In addition, when there is an incomplete formation of nanocarriers, it could lead to coalescence. Therefore, the composition and physicochemical properties of nano-systems should be elaborately modified in accordance with their specific therapeutic application.
Abbreviations
FDA | Food and Drug Administration |
TDDs | transdermal drug delivery systems |
NPs | nanoparticles |
CNTs | carbon nanotubes |
SC | stratum corneum |
NGs | nanogels |
tNGs | thermos-responsive nanogels |
PNIPAM | poly(N-isopropylacrylamide) |
IDCC | indodicarbocyanine |
SRL | Stimulated Raman-Loss |
CS | chitosan |
DxPCA | spin-labeled dexamethasone |
5-FU | 5-fluorouracil |
CLSM | Confocal laser scanning microscopic |
HFs | hair follicles |
NR | Nile red |
SLNs | solid lipid NPs |
NLCs | nanostructured lipid carriers |
HA | hyaluronic acid |
PLA | polylactic acid |
PLGA | poly(lactic-co-glycolic acid) |
AuNPs | Gold NPs |
NA | nucleic acid |
VEGF | vascular endothelial growth factor |
SNA | spherical nucleic acid |
IGF1 | insulin-like growth factor-1 |
GM3S | ganglioside-monosialic acid 3 synthases |
CTGF | connective tissue growth factor |
AgNPs | silver NPs |
ROS | reactive oxygen species |
TEM | transmission electron microscopy |
QDs | quantum dots |
CSSS | cyanoacrylate skin surface stripping |
SEM | scanning electron microscope |
Cryo-EM | cryo-electron microscopy |
AFM | atomic force microscopy |
SERS | surface enhanced Raman scattering |
FTIR | Fourier transformed infrared |
IR | infrared |
EPR | electron paramagnetic resonance |
AD | atopic dermatitis |
FLZ | Fluconazole |
PV | Pityriasis Versicolor |
RCT | randomized controlled clinical trial |
S. aureus
|
Staphylococcus aureus
|
BMV | betamethasone valerate |
DFV | diflucortolone valerate |
SCC | squamous cell carcinoma |
AuPT | gold nanoparticle |
AuPT/Mi221 | gold nanoparticle loaded pDNA system |
Ap | apigenin |
Nap | a dietary flavonoid loaded in poly (lactic-co-glycolide) nanoparticles |
PASI | Psoriatic Area and Severity Index |
DLS | dynamic light scattering |
LFGDM | liquid flow-focusing and gas displacing method |
PLGA-PEG | poly(lactide-co-glycolide)-b-poly(ethylene glycol) |
PLGA-Dex10 | poly(D, L-lactide-co-glycolide)-dextran |
PLGA-Dex10-curc | curcumin-functionalized PLGA-dextran micelles |
PAMAM-G7 | poly (amidoamine) |
NADH | nicotinamide adenine dinucleotide |
HPbCD | (2-hydroxypropyl)-β-cyclodextrin |
MMT | mercapto-1-methylimidazole |
MTX | methotrexate |
MTT | methyl thiazolyl tetrazolium |
TAT | transactivating transcriptional activator |
VE | viable epidermis |
FNAs | framework nucleic acids |
HPLC | High Performance Liquid Chromatography |
MSPM | mixed-shell polymeric micelles |
MRSA | methicillin-resistant Staphylococcus aureus |
PASI | Psoriasis Area and Severity Index |
MTX | methotrexate |
TP-LHP | triptolide-loaded liposome hydrogel patch |
EPR | enhanced permeation and retention |
PTX | paclitaxel |
CPP | cell-penetrating-peptide |
PTX-CTs | paclitaxel (PTX)-encapsulated cell-penetrating-peptide (CPP)-modified transfersomes |
EGCG | epigallocatechin gallate |
GE NPs | gelatin/epigallocatechin gallate (EGCG) nanoparticles |
WS1 | human dermal fibroblast |
TPL | triptolide |
ATE | atenolol |
PAA | poly (acrylic acid) |
3D | three-dimensional |
ICP-MS | plasma-mass spectrometry |
RNPs | rod-shaped nanoparticles |
SNPs | spherical nanoparticles |
TNPs | triangular nanoparticles |
ICP-MS | plasma-mass spectrometry |
LC | liquid crystals |
ISAsomes | Internally self-assembled somes or particles |
Author contributions
Liujun Liu: conceptualization and writing – original draft. Wenbin Zhao: conceptualization and writing – review & editing. Qingming Ma: conceptualization, writing – original draft, conceptualization, writing – review & editing, and supervision. Yang Gao: writing – original draft. Weijiang Wang: writing – original draft and writing – review & editing. Xuan Zhang: writing – original draft. Yunxia Dong: writing – review & editing. Tingting Zhang: writing – original draft. Yan Liang: writing – original draft. Shangcong Han: writing – original draft. Jie Cao: writing – original draft. Xinyu Wang: writing – original draft. Wentao Sun: writing – review & editing. Haifeng Ma: writing – review & editing. Yong Sun: writing – review & editing.
Conflicts of interest
There are no conflicts of interest to declare.
Acknowledgements
This study was supported by the National Natural Science Foundation of China (22008130), China Postdoctoral Science Foundation (2020M682124), Qingdao Science and Technology Demonstration and Guidance Project (21-1-4-rkjk-10-nsh), and Key technology research and industrialization demonstration projects of Qingdao (22-3-3-hygg-25-hy), and the financial support from The Shandong Consortium in the Yellow River Basin for Prevention, Treatment and Drug Development for Primary Diseases Related to Alcoholism.
References
- K. S. Paudel, M. Milewski, C. L. Swadley, N. K. Brogden, P. Ghosh and A. L. Stinchcomb, Challenges and opportunities in dermal/transdermal delivery, Ther. Delivery, 2010, 1(1), 109–131 CrossRef CAS PubMed
.
- S. Wright, M. Yelland, K. Heathcote, S. K. Ng and G. Wright, Fear of needles-nature and prevalence in general practice, Aust. Fam. Physician, 2009, 38(3), 172–176 Search PubMed
.
- C. M. McMurtry, R. Pillai Riddell, A. Taddio, N. Racine, G. J. Asmundson, M. Noel, C. T. Chambers and V. Shah, HelpinKids; Adults, T., Far From “Just a Poke”: Common Painful Needle Procedures and the Development of Needle Fear, Clin. J. Pain, 2015, 31(10 Suppl), S3–S11 CrossRef PubMed
.
- M. N. Pastore, Y. N. Kalia, M. Horstmann and M. S. Roberts, Transdermal patches: history, development and pharmacology, Br. J. Pharmacol., 2015, 172(9), 2179–2209 CrossRef CAS PubMed
.
- T. Waghule, V. K. Rapalli, S. Gorantla, R. N. Saha, S. K. Dubey, A. Puri and G. Singhvi, Nanostructured Lipid Carriers as Potential Drug Delivery Systems for Skin Disorders, Curr. Pharm. Des., 2020, 26(36), 4569–4579 CrossRef CAS PubMed
.
- S. M. Silva, L. Hu, J. J. Sousa, A. A. Pais and B. B. Michniak-Kohn, A combination of nonionic surfactants and iontophoresis to enhance the transdermal drug delivery of ondansetron HCl and diltiazem HCl, Eur. J. Pharm. Biopharm., 2012, 80(3), 663–673 CrossRef CAS PubMed
.
- M. Pradhan, S. Srivastava, D. Singh, S. Saraf, S. Saraf and M. R. Singh, Perspectives of Lipid-Based Drug Carrier Systems for Transdermal Delivery, Crit. Rev. Ther. Drug Carrier Syst., 2018, 35(4), 331–367 CrossRef PubMed
.
- V. H. S. Araujo, L. Delello Di Filippo, J. L. Duarte, L. Sposito, B. A. F. Camargo, P. B. da Silva and M. Chorilli, Exploiting solid lipid nanoparticles and nanostructured lipid carriers for drug delivery against cutaneous fungal infections, Crit. Rev. Microbiol., 2021, 47(1), 79–90 CrossRef CAS PubMed
.
- M. Sguizzato, E. Esposito and R. Cortesi, Lipid-Based Nanosystems as a Tool to Overcome Skin Barrier, Int. J. Mol. Sci., 2021, 22(15), 8319 CrossRef CAS PubMed
.
- M. Gupta, U. Agrawal and S. P. Vyas, Nanocarrier-based topical drug delivery for the treatment of skin diseases, Expert Opin. Drug Delivery, 2012, 9(7), 783–804 CrossRef CAS PubMed
.
- T. Qi, B. Chen, Z. Wang, H. Du, D. Liu, Q. Yin, B. Liu, Q. Zhang and Y. Wang, A pH-Activatable nanoparticle for dual-stage precisely mitochondria-targeted photodynamic anticancer therapy, Biomaterials, 2019, 213, 119219 CrossRef CAS PubMed
.
- Y. Hui, X. Yi, F. Hou, D. Wibowo, F. Zhang, D. Zhao, H. Gao and C. X. Zhao, Role of Nanoparticle Mechanical Properties in Cancer Drug Delivery, ACS Nano, 2019, 13(7), 7410–7424 CrossRef CAS PubMed
.
- G. Birrenbach and P. P. Speiser, Polymerized micelles and their use as adjuvants in immunology, J. Pharm. Sci., 1976, 65(12), 1763–1766 CrossRef CAS PubMed
.
- L. Yang, C. S. Kwan, L. L. Zhang, X. H. Li, Y. Han, K. C. F. Leung, Y. G. Yang and Z. F. Huang, Chiral Nanoparticle-Induced Enantioselective Amplification of Molecular Optical Activity, Adv. Funct. Mater., 2019, 29(8), 1807307 CrossRef
.
- A. Shchelokov, N. Palko, V. Potemkin, M. Grishina, R. Morozov, E. Korina, D. Uchaev, I. Krivtsov and O. Bol'shakov, Adsorption of Native Amino Acids on Nanocrystalline TiO2: Physical Chemistry, QSPR, and Theoretical
Modeling, Langmuir, 2019, 35(2), 538–550 CrossRef CAS PubMed
.
- F. Rancan, H. Volkmann, M. Giulbudagian, F. Schumacher, J. I. Stanko, B. Kleuser, U. Blume-Peytavi, M. Calderon and A. Vogt, Dermal Delivery of the High-Molecular-Weight Drug Tacrolimus by Means of Polyglycerol-Based Nanogels, Pharmaceutics, 2019, 11(8), 394 CrossRef CAS PubMed
.
- F. Rancan, M. Asadian-Birjand, S. Dogan, C. Graf, L. Cuellar, S. Lommatzsch, U. Blume-Peytavi, M. Calderon and A. Vogt, Effects of thermoresponsivity and softness on skin penetration and cellular uptake of polyglycerol-based nanogels, J. Controlled Release, 2016, 228, 159–169 CrossRef CAS PubMed
.
- N. Tiwari, E. R. Osorio-Blanco, A. Sonzogni, D. Esporrin-Ubieto, H. Wang and M. Calderon, Nanocarriers for Skin Applications: Where Do We Stand?, Angew. Chem., Int. Ed. Engl., 2022, 61(3), e202107960 CrossRef CAS PubMed
.
- E. Ratemi, A. Sultana Shaik, A. Al Faraj and R. Halwani, Alternative approaches for the treatment of airway diseases: focus on nanoparticle medicine, Clin. Exp. Allergy, 2016, 46(8), 1033–1042 CrossRef CAS PubMed
.
- A. Yau, J. Lee and Y. Chen, Nanomaterials for Protein Delivery in Anticancer Applications, Pharmaceutics, 2021, 13(2), 155 CrossRef CAS PubMed
.
- C. M. Schoellhammer, D. Blankschtein and R. Langer, Skin permeabilization for transdermal drug delivery: recent advances and future prospects, Expert Opin. Drug Delivery, 2014, 11(3), 393–407 CrossRef CAS PubMed
.
- B. E. Polat, D. Blankschtein and R. Langer, Low-frequency sonophoresis: application to the transdermal delivery of macromolecules and hydrophilic drugs, Expert Opin. Drug Delivery, 2010, 7(12), 1415–1432 CrossRef CAS PubMed
.
- A. Dahlan, H. O. Alpar, P. Stickings, D. Sesardic and S. Murdan, Transcutaneous immunisation assisted by low-frequency ultrasound, Int. J. Pharm., 2009, 368(1–2), 123–128 CrossRef CAS PubMed
.
- S. M. Bal, Z. Ding, E. van Riet, W. Jiskoot and J. A. Bouwstra, Advances in transcutaneous vaccine delivery: do all ways lead to Rome?, J. Controlled Release, 2010, 148(3), 266–282 CrossRef CAS PubMed
.
- E. Kahraman, S. Gungor and Y. Ozsoy, Potential enhancement and targeting strategies of polymeric and lipid-based nanocarriers in dermal drug delivery, Ther. Delivery, 2017, 8(11), 967–985 CrossRef CAS PubMed
.
- B. C. Palmer and L. A. DeLouise, Nanoparticle-Enabled Transdermal Drug Delivery Systems for Enhanced Dose Control and Tissue Targeting, Molecules, 2016, 21(12), 1719 CrossRef PubMed
.
- M. Ashokkumar, J. Lee, Y. Iida, K. Yasui, T. Kozuka, T. Tuziuti and A. Towata, Spatial distribution of acoustic cavitation bubbles at different ultrasound frequencies, Chemphyschem, 2010, 11(8), 1680–1684 CrossRef CAS PubMed
.
- C. Scarponi, F. Nasorri, F. Pavani, S. Madonna, R. Sestito, M. Simonacci, O. De Pita, A. Cavani and C. Albanesi, Low-frequency low-intensity ultrasounds do not influence the survival and immune functions of cultured keratinocytes and dendritic cells, J. Biomed. Biotechnol., 2009, 2009, 193260 Search PubMed
.
- S. Jain, N. Patel, M. K. Shah, P. Khatri and N. Vora, Recent Advances in Lipid-Based Vesicles and Particulate Carriers for Topical and Transdermal Application, J. Pharm. Sci., 2017, 106(2), 423–445 CrossRef CAS PubMed
.
- F. Erdo, N. Hashimoto, G. Karvaly, N. Nakamichi and Y. Kato, Critical evaluation and methodological positioning of the transdermal microdialysis technique. A review, J. Controlled Release, 2016, 233, 147–161 CrossRef CAS PubMed
.
- R. J. Scheuplein and I. H. Blank, Permeability of the skin, Physiol. Rev., 1971, 51(4), 702–747 CrossRef CAS PubMed
.
- B. Baroli, M. G. Ennas, F. Loffredo, M. Isola, R. Pinna and M. A. Lopez-Quintela, Penetration of metallic nanoparticles in human full-thickness skin, J. Invest. Dermatol., 2007, 127(7), 1701–1712 CrossRef CAS PubMed
.
- M. Schneider, F. Stracke, S. Hansen and U. F. Schaefer, Nanoparticles and their interactions with the dermal barrier, Dermatoendocrinol, 2009, 1(4), 197–206 CrossRef CAS PubMed
.
- R. J. Scheuplein, Mechanism of percutaneous absorption. II. Transient diffusion and the relative importance of various routes of skin penetration, J. Invest. Dermatol., 1967, 48(1), 79–88 CrossRef CAS PubMed
.
- K. Niska, E. Zielinska, M. W. Radomski and I. Inkielewicz-Stepniak, Metal nanoparticles in dermatology and cosmetology: interactions with human skin cells, Chem.-Biol. Interact., 2018, 295, 38–51 CrossRef CAS PubMed
.
- R. Toll, U. Jacobi, H. Richter, J. Lademann, H. Schaefer and U. Blume-Peytavi, Penetration profile of microspheres in follicular targeting of terminal hair follicles, J. Invest. Dermatol., 2004, 123(1), 168–176 CrossRef CAS PubMed
.
- R. Alvarez-Roman, A. Naik, Y. N. Kalia, R. H. Guy and H. Fessi, Skin penetration and distribution of polymeric nanoparticles, J. Controlled Release, 2004, 99(1), 53–62 CrossRef CAS PubMed
.
- A. S. Hoffman, The origins and evolution of “controlled” drug delivery systems, J. Controlled Release, 2008, 132(3), 153–163 CrossRef CAS PubMed
.
- A. Vogt, N. Mandt, J. Lademann, H. Schaefer and U. Blume-Peytavi, Follicular targeting–a promising tool in selective dermatotherapy, J. Invest. Dermatol. Symp. Proc., 2005, 10(3), 252–255 CrossRef PubMed
.
- G. Sonavane, K. Tomoda, A. Sano, H. Ohshima, H. Terada and K. Makino,
In vitro permeation of gold nanoparticles through rat skin and rat intestine: effect of particle size, Colloids Surf., B, 2008, 65(1), 1–10 CrossRef CAS PubMed
.
- F. Rancan, Q. Gao, C. Graf, S. Troppens, S. Hadam, S. Hackbarth, C. Kembuan, U. Blume-Peytavi, E. Ruhl, J. Lademann and A. Vogt, Skin penetration and cellular uptake of amorphous silica nanoparticles with variable size, surface functionalization, and colloidal stability, ACS Nano, 2012, 6(8), 6829–6842 CrossRef CAS PubMed
.
- J. E. Seto, B. E. Polat, R. F. Lopez, D. Blankschtein and R. Langer, Effects of ultrasound and sodium lauryl sulfate on the transdermal delivery of hydrophilic permeants: comparative in vitro studies with full-thickness and split-thickness pig and human skin, J. Controlled Release, 2010, 145(1), 26–32 CrossRef CAS PubMed
.
- M. Yang, Y. Gu, X. Tang, T. Wang and J. Liu, Advancement of Lipid-Based Nanocarriers and Combination Application with Physical Penetration Technique, Curr. Drug Delivery, 2019, 16(4), 312–324 CrossRef CAS PubMed
.
- C. Wiraja, Y. Zhu, D. C. S. Lio, D. C. Yeo, M. Xie, W. Fang, Q. Li, M. Zheng, M. Van Steensel, L. Wang, C. Fan and C. Xu, Framework nucleic acids as programmable carrier for transdermal drug delivery, Nat. Commun., 2019, 10(1), 1147 CrossRef PubMed
.
- M. E. Lane, Skin penetration enhancers, Int. J. Pharm., 2013, 447(1–2), 12–21 CrossRef CAS PubMed
.
- R. Gupta, B. S. Dwadasi, B. Rai and S. Mitragotri, Effect of Chemical Permeation Enhancers on Skin Permeability: In silico screening using Molecular Dynamics simulations, Sci. Rep., 2019, 9(1), 1456 CrossRef PubMed
.
- M. Giulbudagian, F. Rancan, A. Klossek, K. Yamamoto, J. Jurisch, V. C. Neto, P. Schrade, S. Bachmann, E. Ruhl, U. Blume-Peytavi, A. Vogt and M. Calderon, Correlation between the chemical composition of thermoresponsive nanogels and their interaction with the skin barrier, J. Controlled Release, 2016, 243, 323–332 CrossRef CAS PubMed
.
- M. Dimde, F. F. Sahle, V. Wycisk, D. Steinhilber, L. C. Camacho, K. Licha, J. Lademann and R. Haag, Synthesis and Validation of Functional Nanogels as pH-Sensors in the Hair Follicle, Macromol. Biosci., 2017, 17(10), 1600505 CrossRef PubMed
.
- M. H. Schmid-Wendtner and H. C. Korting, The pH of the skin surface and its impact on the barrier function, Skin Pharmacol. Physiol., 2006, 19(6), 296–302 CrossRef PubMed
.
- F. F. Sahle, C. Gerecke, B. Kleuser and R. Bodmeier, Formulation and comparative in vitro evaluation of various dexamethasone-loaded pH-sensitive polymeric nanoparticles intended for dermal applications, Int. J. Pharm., 2017, 516(1–2), 21–31 CrossRef CAS PubMed
.
- K. Thoma and K. Bechtold, Influence of aqueous coatings on the stability of enteric coated pellets and tablets, Eur. J. Pharm. Biopharm., 1999, 47(1), 39–50 CrossRef CAS PubMed
.
- P. Sahu, S. K. Kashaw, S. Sau, V. Kushwah, S. Jain, R. K. Agrawal and A. K. Iyer, pH Responsive 5-Fluorouracil Loaded Biocompatible Nanogels For Topical Chemotherapy of Aggressive Melanoma, Colloids Surf., B, 2019, 174, 232–245 CrossRef CAS PubMed
.
- P. Dong, F. F. Sahle, S. B. Lohan, S. Saeidpour, S. Albrecht, C. Teutloff, R. Bodmeier, M. Unbehauen, C. Wolff, R. Haag, J. Lademann, A. Patzelt, M. Schafer-Korting and M. C. Meinke, pH-sensitive Eudragit(R) L 100 nanoparticles promote cutaneous penetration and drug release on the skin, J. Controlled Release, 2019, 295, 214–222 CrossRef CAS PubMed
.
- F. Larese Filon, M. Mauro, G. Adami, M. Bovenzi and M. Crosera, Nanoparticles skin absorption: New aspects for a safety profile evaluation, Regul. Toxicol. Pharmacol., 2015, 72(2), 310–322 CrossRef CAS PubMed
.
-
O. Uchechi, J. D. N. Ogbonna and A. A. Attama, Nanoparticles for Dermal and Transdermal Drug Delivery, in Application of Nanotechnology in Drug Delivery, 2014 Search PubMed
.
- P. R. Leroueil, S. Hong, A. Mecke, J. R. Baker, B. G. Orr and M. M. Banaszak Holl, Nanoparticle interaction with biological membranes: does nanotechnology present a Janus face?, Acc. Chem. Res., 2007, 40(5), 335–342 CrossRef CAS PubMed
.
- P. V. Pople and K. K. Singh, Development and evaluation of colloidal modified nanolipid carrier: application to topical delivery of tacrolimus, Eur. J. Pharm. Biopharm., 2011, 79(1), 82–94 CrossRef CAS PubMed
.
- W. E. Bawarski, E. Chidlowsky, D. J. Bharali and S. A. Mousa, Emerging nanopharmaceuticals, Nanomedicine, 2008, 4(4), 273–282 CrossRef CAS PubMed
.
- L. Zhang, D. Pornpattananangku, C. M. Hu and C. M. Huang, Development of nanoparticles for antimicrobial drug delivery, Curr. Med. Chem., 2010, 17(6), 585–594 CrossRef CAS PubMed
.
- R. H. Muller, K. Mader and S. Gohla, Solid lipid nanoparticles (SLN) for controlled drug delivery – a review of the state of the art, Eur. J. Pharm. Biopharm., 2000, 50(1), 161–177 CrossRef CAS PubMed
.
- F. Nasiri, L. Faghfouri and M. Hamidi, Preparation, optimization, and in vitro characterization of alpha-tocopherol-loaded solid lipid nanoparticles (SLNs), Drug Dev. Ind. Pharm., 2020, 46(1), 159–171 CrossRef CAS PubMed
.
- K. M. Wolfgang Mehnert, Solid lipid nanoparticles production, characterization and applications, Adv. Drug Delivery Rev., 2001, 47(2–3), 165–196 CrossRef PubMed
.
- R. Parhi and P. Suresh, Preparation and characterization of solid lipid nanoparticles-a review, Curr. Drug Discovery Technol., 2012, 9(1), 2–16 CrossRef CAS PubMed
.
- J. Yun, S. Zhang, S. Shen, Z. Chen, K. Yao and J. Chen, Continuous production of solid lipid nanoparticles by liquid flow-focusing and gas displacing method in microchannels, Chem. Eng. Sci., 2009, 64(19), 4115–4122 CrossRef CAS
.
- Q. Ma and J. Xu, Green microfluidics in microchemical engineering for carbon neutrality, Chin. J. Chem. Eng., 2023, 53, 332–345 CrossRef
.
- D. Butani, C. Yewale and A. Misra, Topical Amphotericin B solid lipid nanoparticles: Design and development, Colloids Surf., B, 2016, 139, 17–24 CrossRef CAS PubMed
.
- J. Pardeike, A. Hommoss and R. H. Muller, Lipid nanoparticles (SLN, NLC) in cosmetic and pharmaceutical dermal products, Int. J. Pharm., 2009, 366(1–2), 170–184 CrossRef CAS PubMed
.
- C. L. Fang, S. A. Al-Suwayeh and J. Y. Fang, Nanostructured lipid carriers (NLCs) for drug delivery and targeting, Recent Pat. Nanotechnol., 2013, 7(1), 41–55 CrossRef CAS PubMed
.
- J. du Plessis, K. Egbaria, C. Ramachandran and N. Weiner, Topical delivery of liposomally encapsulated gamma-interferon, Antiviral Res., 1992, 18(3–4), 259–265 CrossRef CAS PubMed
.
- D. Muhindo, E. A. Ashour, M. Almutairi, P. H. Joshi and M. A. Repka, Continuous Production of Raloxifene Hydrochloride Loaded Nanostructured Lipid Carriers Using Hot-Melt Extrusion Technology, J. Drug Delivery Sci. Technol., 2021, 65 Search PubMed
.
- E. Gomaa, H. A. Fathi, N. G. Eissa and M. Elsabahy, Methods for preparation of nanostructured lipid carriers, Methods, 2022, 199, 3–8 CrossRef CAS PubMed
.
- H. A. Fathi, A. Allam, M. Elsabahy, G. Fetih and M. El-Badry, Nanostructured lipid carriers for improved oral delivery and prolonged antihyperlipidemic effect of simvastatin, Colloids Surf., B, 2018, 162, 236–245 CrossRef CAS PubMed
.
- N. Naseri, H. Valizadeh and P. Zakeri-Milani, Solid Lipid Nanoparticles and Nanostructured Lipid Carriers: Structure, Preparation and Application, Adv. Pharm. Bull., 2015, 5(3), 305–313 CrossRef CAS PubMed
.
- V. Mishra, K. K. Bansal, A. Verma, N. Yadav, S. Thakur, K. Sudhakar and J. M. Rosenholm, Solid Lipid Nanoparticles: Emerging Colloidal Nano Drug Delivery Systems, Pharmaceutics, 2018, 10(4), 191 CrossRef CAS PubMed
.
- B. Gaba, M. Fazil, S. Khan, A. Ali, S. Baboota and J. Ali, Nanostructured lipid carrier system for topical delivery of terbinafine hydrochloride, Bull. Fac. Pharm. Cairo Univ., 2015, 53(2), 147–159 Search PubMed
.
- A. A. Phatak and P. D. Chaudhari, Development and evaluation of Nanostructured Lipid Carrier (NLC) based topical delivery of an anti-inflammatory drug, J. Pharm. Res., 2013, 7(8), 677–685 CAS
.
- P. Kushwaha, S. Saxena and B. Shukla, A Recent Overview on Dermatological Applications of Liposomes, Recent Pat. Nanotechnol., 2021, 15(4), 310–321 CrossRef CAS PubMed
.
- G. J. Nohynek, E. K. Dufour and M. S. Roberts, Nanotechnology, cosmetics and the skin: is there a health risk?, Skin Pharmacol. Physiol., 2008, 21(3), 136–149 CrossRef CAS PubMed
.
- A. K. Ramanunny, S. Wadhwa, M. Gulati, S. K. Singh, B. Kapoor, H. Dureja, D. K. Chellappan, K. Anand, K. Dua, R. Khursheed, A. Awasthi, R. Kumar, J. Kaur, L. Corrie and N. K. Pandey, Nanocarriers for treatment of dermatological diseases: Principle, perspective and practices, Eur. J. Pharmacol., 2021, 890, 173691 CrossRef CAS PubMed
.
- A. Laouini, C. Jaafar-Maalej, I. Limayem-Blouza, S. Sfar, C. Charcosset and H. Fessi, Preparation, Characterization and Applications of Liposomes: State of the Art, J. Colloid Sci. Biotechnol., 2012, 1(2), 147–168 CrossRef CAS
.
- S. Jain, N. Patel, P. Madan and S. Lin, Quality by design approach for formulation, evaluation and statistical optimization of diclofenac-loaded ethosomes via transdermal route, Pharm. Dev. Technol., 2015, 20(4), 473–489 CrossRef CAS PubMed
.
- N. Parnami, T. Garg, G. Rath and A. K. Goyal, Development and characterization of nanocarriers for topical treatment of psoriasis by using combination therapy, Artif. Cells, Nanomed., Biotechnol., 2014, 42(6), 406–412 CrossRef CAS PubMed
.
- S. Shah, V. Dhawan, R. Holm, M. S. Nagarsenker and Y. Perrie, Liposomes: Advancements and innovation in the manufacturing process, Adv. Drug Delivery Rev., 2020, 154–155, 102–122 CrossRef CAS PubMed
.
- R. R. Hood, W. N. Vreeland and D. L. DeVoe, Microfluidic remote loading for rapid single-step liposomal drug preparation, Lab Chip, 2014, 14(17), 3359–3367 RSC
.
- S. K. Das, S. Chakraborty, C. Roy, R. Rajabalaya, A. W. Mohaimin, J. Khanam, A. Nanda and S. R. David, Ethosomes as Novel Vesicular Carrier: An Overview of the Principle, Preparation and its Applications, Curr. Drug Delivery, 2018, 15(6), 795–817 CrossRef CAS PubMed
.
- M. M. V. Gulasekharam, Liposomes – a selective drug delivery system for the topical route of administration I. Lotion dosage form, Life Sci., 1980, 26(18), 1473–1477 CrossRef PubMed
.
- M. Mezei and V. Gulasekharam, Liposomes–a selective drug delivery system for the topical route of administration: gel dosage form, J. Pharm. Pharmacol., 1982, 34(7), 473–474 CrossRef CAS PubMed
.
- G. M. El Maghraby, A. C. Williams and B. W. Barry, Can drug-bearing liposomes penetrate intact skin?, J. Pharm. Pharmacol., 2006, 58(4), 415–429 CrossRef CAS PubMed
.
- H. E. Hofland, J. A. Bouwstra, H. E. Bodde, F. Spies and H. E. Junginger, Interactions between liposomes and human stratum corneum in vitro: freeze fracture electron microscopical visualization and small angle X-ray scattering studies, Br. J. Dermatol., 1995, 132(6), 853–866 CrossRef CAS PubMed
.
- M. Kirjavainen, J. Mönkkönen, M. Saukkosaari, R. Valjakka-Koskela, J. Kiesvaara and A. Urtti, Phospholipids affect stratum corneum lipid bilayer fluidity and drug partitioning into the bilayers, J. Controlled Release, 1999, 58(2), 207–214 CrossRef CAS PubMed
.
- K. Merja, A. U., J. Ilpo, M. T. Suhonen, P. Petteri, V. Valjakka-Koskela, J. Kiesvaara and J. MtinkkiSnen, Interaction of liposomes with human skin in vitro lipid composition and structure, Biochim. Biophys. Acta, 1996, 1304(3), 179–189 Search PubMed
.
- S. Zellmer, W. Pfeil and J. Lasch, Interaction of phosphatidylcholine liposomes with the human stratum corneum, Biochim. Biophys. Acta, 1995, 1237(2), 176–182 CrossRef PubMed
.
- I. A. Alsarra, A. A. Bosela, S. M. Ahmed and G. M. Mahrous, Proniosomes as a drug carrier for transdermal delivery of ketorolac, Eur. J. Pharm. Biopharm., 2005, 59(3), 485–490 CrossRef CAS PubMed
.
- R. Muzzalupo, L. Tavano, R. Cassano, S. Trombino, T. Ferrarelli and N. Picci, A new approach for the evaluation of niosomes as effective transdermal drug delivery systems, Eur. J. Pharm. Biopharm., 2011, 79(1), 28–35 CrossRef CAS PubMed
.
- G. Cevc and G. Blume, Lipid vesicles penetrate into intact skin owing to the transdermal osmotic gradients and hydration force, Biochim. Biophys. Acta, 1992, 1104(1), 226–232 CrossRef CAS PubMed
.
- S. Jain, P. Jain, R. B. Umamaheshwari and N. K. Jain, Transfersomes–a novel vesicular carrier for enhanced transdermal delivery: development, characterization, and performance evaluation, Drug Dev. Ind. Pharm., 2003, 29(9), 1013–1026 CrossRef CAS PubMed
.
- A. Gupta, G. Aggarwal, S. Singla and R. Arora, Transfersomes: a novel vesicular carrier for enhanced transdermal delivery of sertraline: development, characterization, and performance evaluation, Sci. Pharm., 2012, 80(4), 1061–1080 CrossRef CAS PubMed
.
- G. Cevc, Lipid vesicles and other colloids as drug carriers on the skin, Adv. Drug Delivery Rev., 2004, 56(5), 675–711 CrossRef CAS PubMed
.
- E. K. Oh, S. E. Jin, J. K. Kim, J. S. Park, Y. Park and C. K. Kim, Retained topical delivery of 5-aminolevulinic acid using cationic ultradeformable liposomes for photodynamic therapy, Eur. J. Pharm. Sci., 2011, 44(1–2), 149–157 CrossRef CAS PubMed
.
- N. Dragicevic-Curic, S. Grafe, B. Gitter, S. Winter and A. Fahr, Surface charged temoporfin-loaded flexible vesicles: in vitro skin penetration studies and stability, Int. J. Pharm., 2010, 384(1–2), 100–108 CrossRef CAS PubMed
.
- S. Duangjit, P. Opanasopit, T. Rojanarata and T. Ngawhirunpat, Characterization and In Vitro Skin Permeation of Meloxicam-Loaded Liposomes versus Transfersomes, J. Drug Delivery, 2011, 2011, 418316 Search PubMed
.
- C. C. Mbah, P. F. Builders and A. A. Attama, Nanovesicular carriers as alternative drug delivery systems: ethosomes in focus, Expert Opin. Drug Delivery, 2014, 11(1), 45–59 CrossRef CAS PubMed
.
- S. K. Das, S. Chakraborty, C. Roy, R. Rajabalaya, A. W. Mohaimin, J. Khanam, A. Nanda and S. R. David, Ethosomes as Novel Vesicular Carrier: An Overview of the Principle, Preparation and its Applications, Curr. Drug Delivery, 2018, 15(6), 795–817 CrossRef CAS PubMed
.
- S. Wadhwa, V. Garg, M. Gulati, B. Kapoor, S. K. Singh and N. Mittal, Nanovesicles for Nanomedicine: Theory and Practices, Methods Mol. Biol., 2019, 2000, 1–17 CrossRef CAS PubMed
.
- S. S. Bhosale and A. M. Avachat, Design and development of ethosomal transdermal drug delivery system of valsartan with preclinical assessment in Wistar albino rats, J. Liposome Res., 2013, 23(2), 119–125 CrossRef CAS PubMed
.
- S. Jain, A. K. Tiwary, B. Sapra and N. K. Jain, Formulation and evaluation of ethosomes for transdermal delivery of lamivudine, AAPS PharmSciTech, 2007, 8(4), E111 CrossRef PubMed
.
- T. Serikawa, A. Kikuchi, S. Sugaya, N. Suzuki, H. Kikuchi and K. Tanaka,
In vitro and in vivo evaluation of novel cationic liposomes utilized for cancer gene therapy, J. Controlled Release, 2006, 113(3), 255–260 CrossRef CAS PubMed
.
- D. Paolino, G. Lucania, D. Mardente, F. Alhaique and M. Fresta, Ethosomes for skin delivery of ammonium glycyrrhizinate: in vitro percutaneous permeation through human skin and in vivo anti-inflammatory activity on human volunteers, J. Controlled Release, 2005, 106(1–2), 99–110 CrossRef CAS PubMed
.
- X. Q. Niu, D. P. Zhang, Q. Bian, X. F. Feng, H. Li, Y. F. Rao, Y. M. Shen, F. N. Geng, A. R. Yuan, X. Y. Ying and J. Q. Gao, Mechanism investigation of ethosomes transdermal permeation, Int. J. Pharm.: X, 2019, 1, 100027 CAS
.
- W. Mehnert and K. Mader, Solid lipid nanoparticles: production, characterization and applications, Adv. Drug Delivery Rev., 2001, 47(2–3), 165–196 CrossRef CAS PubMed
.
- E. Touitou, N. Dayan, L. Bergelson, B. Godin and M. Eliaz, Ethosomes — novel vesicular carriers for enhanced delivery:characterization and skin penetration properties, J. Controlled Release, 2000, 65(3), 403–418 CrossRef CAS PubMed
.
- R. H. Muller and C. M. Keck, Challenges and solutions for the delivery of biotech drugs–a review of drug nanocrystal technology and lipid nanoparticles, J. Biotechnol., 2004, 113(1–3), 151–170 CrossRef CAS PubMed
.
- F. Castelli, C. Puglia, M. G. Sarpietro, L. Rizza and F. Bonina, Characterization of indomethacin-loaded lipid nanoparticles by differential scanning calorimetry, Int. J. Pharm., 2005, 304(1–2), 231–238 CrossRef CAS PubMed
.
- A. Yaghmur and H. Mu, Recent advances in drug delivery applications of cubosomes, hexosomes, and solid lipid nanoparticles, Acta Pharm. Sin. B, 2021, 11(4), 871–885 CrossRef CAS PubMed
.
- A. Gordillo-Galeano and C. E. Mora-Huertas, Solid lipid nanoparticles and nanostructured lipid carriers: A review emphasizing on particle structure and drug release, Eur. J. Pharm. Biopharm., 2018, 133, 285–308 CrossRef CAS PubMed
.
- X. Shao, G. Bor, S. Al-Hosayni, S. Salentinig and A. Yaghmur, Structural characterization of self-assemblies of new omega-3 lipids: docosahexaenoic acid and docosapentaenoic acid monoglycerides, Phys. Chem. Chem. Phys., 2018, 20(37), 23928–23941 RSC
.
- I. D. M. Azmi, J. Ostergaard, S. Sturup, B. Gammelgaard, A. Urtti, S. M. Moghimi and A. Yaghmur, Cisplatin Encapsulation Generates Morphologically Different Multicompartments in the Internal Nanostructures of Nonlamellar Liquid-Crystalline Self-Assemblies, Langmuir, 2018, 34(22), 6570–6581 CrossRef CAS PubMed
.
- I. D. Azmi, P. P. Wibroe, L. P. Wu, A. I. Kazem, H. Amenitsch, S. M. Moghimi and A. Yaghmur, A structurally diverse library of safe-by-design citrem-phospholipid lamellar and non-lamellar liquid crystalline nano-assemblies, J. Controlled Release, 2016, 239, 1–9 CrossRef CAS PubMed
.
- L. Boge, K. Hallstensson, L. Ringstad, J. Johansson, T. Andersson, M. Davoudi, P. T. Larsson, M. Mahlapuu, J. Hakansson and M. Andersson, Cubosomes for topical delivery of the antimicrobial peptide LL-37, Eur. J. Pharm. Biopharm., 2019, 134, 60–67 CrossRef CAS PubMed
.
- S. M. Mohyeldin, M. M. Mehanna and N. A. Elgindy, Superiority of liquid crystalline cubic nanocarriers as hormonal transdermal vehicle: comparative human skin permeation-supported evidence, Expert Opin. Drug Delivery, 2016, 13(8), 1049–1064 CrossRef CAS PubMed
.
- S. A. S. Al-Zuhairy, W. R. Kadhum, M. Alhijjaj, M. M. Kadhim, A. S. Al-Janabi, A. W. Salman, H. K. R. Al-Sharifi and A. A. Khadom, Development and Evaluation of Biocompatible Topical Petrolatum-liquid Crystal Formulations with Enhanced Skin Permeation Properties, J. Oleo Sci., 2022, 71(3), 459–468 CrossRef CAS PubMed
.
- A. Vogt, C. Wischke, A. T. Neffe, N. Ma, U. Alexiev and A. Lendlein, Nanocarriers for drug delivery into and through the skin – Do existing technologies match clinical challenges?, J. Controlled Release, 2016, 242, 3–15 CrossRef CAS PubMed
.
- F. Rancan, U. Blume-Peytavi and A. Vogt, Utilization of biodegradable polymeric materials as delivery agents in dermatology, Clin., Cosmet. Invest. Dermatol., 2014, 7, 23–34 CrossRef PubMed
.
- C. P. Reis, R. J. Neufeld, A. J. Ribeiro and F. Veiga, Nanoencapsulation I. Methods for preparation of drug-loaded polymeric nanoparticles, Nanomedicine, 2006, 2(1), 8–21 CrossRef CAS PubMed
.
- R. Karnik, F. Gu, P. Basto, C. Cannizzaro, L. Dean, W. Kyei-Manu, R. Langer and O. C. Farokhzad, Microfluidic platform for controlled synthesis of polymeric nanoparticles, Nano Lett., 2008, 8(9), 2906–2912 CrossRef CAS PubMed
.
- M. Cui, C. Wiraja, S. W. T. Chew and C. Xu, Nanodelivery Systems for Topical Management of Skin Disorders, Mol. Pharm., 2021, 18(2), 491–505 CrossRef CAS PubMed
.
- J. U. Menon, P. Ravikumar, A. Pise, D. Gyawali, C. C. Hsia and K. T. Nguyen, Polymeric nanoparticles for pulmonary protein and DNA delivery, Acta Biomater., 2014, 10(6), 2643–2652 CrossRef CAS PubMed
.
- P. Rajitha, D. Gopinath, R. Biswas, M. Sabitha and R. Jayakumar, Chitosan nanoparticles in drug therapy of infectious and inflammatory diseases, Expert Opin. Drug Delivery, 2016, 13(8), 1177–1194 CrossRef CAS PubMed
.
- E. Szymanska and K. Winnicka, Stability of chitosan-a challenge for pharmaceutical and biomedical applications, Mar. Drugs, 2015, 13(4), 1819–1846 CrossRef CAS PubMed
.
- I. Takeuchi, T. Takeshita, T. Suzuki and K. Makino, Iontophoretic transdermal delivery using chitosan-coated PLGA nanoparticles for positively charged drugs, Colloids Surf., B, 2017, 160, 520–526 CrossRef CAS PubMed
.
- S. M. Bennett, M. Arumugam, S. Wilberforce, D. Enea, N. Rushton, X. C. Zhang, S. M. Best, R. E. Cameron and R. A. Brooks, The effect of particle size on the in vivo degradation of poly(D,L-lactide-co-glycolide)/alpha-tricalcium phosphate micro- and nanocomposites, Acta Biomater., 2016, 45, 340–348 CrossRef CAS PubMed
.
- M. Prieto, A. Y. Rwei, T. Alejo, T. Wei, M. T. Lopez-Franco, G. Mendoza, V. Sebastian, D. S. Kohane and M. Arruebo, Light-Emitting Photon-Upconversion Nanoparticles in the Generation of Transdermal Reactive-Oxygen Species, ACS Appl. Mater. Interfaces, 2017, 9(48), 41737–41747 CrossRef CAS PubMed
.
- D. Smejkalova, T. Muthny, K. Nesporova, M. Hermannova, E. Achbergerova, G. Huerta-Angeles, M. Svoboda, M. Cepa, V. Machalova, D. Luptakova and V. Velebny, Hyaluronan polymeric micelles for topical drug delivery, Carbohydr. Polym., 2017, 156, 86–96 CrossRef CAS PubMed
.
- V. P. Torchilin, Structure and design of polymeric surfactant-based drug delivery systems, J. Controlled Release, 2001, 73(2–3), 137–172 CrossRef CAS PubMed
.
- S. S. Kesharwani, S. Kaur, H. Tummala and A. T. Sangamwar, Multifunctional approaches utilizing polymeric micelles to circumvent multidrug resistant tumors, Colloids Surf., B, 2019, 173, 581–590 CrossRef CAS PubMed
.
- Y. Lu and K. Park, Polymeric micelles and alternative nanonized delivery vehicles for poorly soluble drugs, Int. J. Pharm., 2013, 453(1), 198–214 CrossRef CAS PubMed
.
- R. Thipparaboina, R. B. Chavan, D. Kumar, S. Modugula and N. R. Shastri, Micellar carriers for the delivery of multiple therapeutic agents, Colloids Surf., B, 2015, 135, 291–308 CrossRef CAS PubMed
.
- E. J. Cha, J. E. Kim and C. H. Ahn, Stabilized polymeric micelles by electrostatic interactions for drug delivery system, Eur. J. Pharm. Sci., 2009, 38(4), 341–346 CrossRef CAS PubMed
.
- C. H. N. Barros, D. W. Hiebner, S. Fulaz, S. Vitale, L. Quinn and E. Casey, Synthesis and self-assembly of curcumin-modified amphiphilic polymeric micelles with antibacterial activity, J. Nanobiotechnol., 2021, 19(1), 104 CrossRef CAS PubMed
.
- C. Dianzani, G. P. Zara, G. Maina, P. Pettazzoni, S. Pizzimenti, F. Rossi, C. L. Gigliotti, E. S. Ciamporcero, M. Daga and G. Barrera, Drug delivery nanoparticles in skin cancers, BioMed Res. Int., 2014, 2014, 895986 Search PubMed
.
- Z. Mhlwatika and B. A. Aderibigbe, Application of Dendrimers for the Treatment of Infectious Diseases, Molecules, 2018, 23(9), 2205 CrossRef PubMed
.
- K. Dave and V. V. Krishna Venuganti, Dendritic polymers for dermal drug delivery, Ther. Delivery, 2017, 8(12), 1077–1096 CrossRef CAS PubMed
.
- B. Gorain, M. Tekade, P. Kesharwani, A. K. Iyer, K. Kalia and R. K. Tekade, The use of nanoscaffolds and dendrimers in tissue engineering, Drug Discovery Today, 2017, 22(4), 652–664 CrossRef CAS PubMed
.
- M. Gholami, R. Mohammadi, M. Arzanlou, F. A. Dourbash, E. Kouhsari, G. Majidi, S. M. Mohseni and S. Nazari,
In vitro antibacterial activity of poly (amidoamine)-G7 dendrimer, BMC Infect. Dis., 2017, 17, 1–11 CrossRef PubMed
.
- V. V. Venuganti and O. P. Perumal, Poly(amidoamine) dendrimers as skin penetration enhancers: Influence of charge, generation, and concentration, J. Pharm. Sci., 2009, 98(7), 2345–2356 CrossRef CAS PubMed
.
- V. V. Venuganti, P. Sahdev, M. Hildreth, X. Guan and O. Perumal, Structure-skin permeability relationship of dendrimers, Pharm. Res., 2011, 28(9), 2246–2260 CrossRef CAS PubMed
.
- M. Rahman and E. Rebrov, Microreactors for Gold Nanoparticles Synthesis: From Faraday to Flow, Processes, 2014, 2(2), 466–493 CrossRef
.
- V. Sebastian, S. K. Lee, C. Zhou, M. F. Kraus, J. G. Fujimoto and K. F. Jensen, One-step continuous synthesis of biocompatible gold nanorods for optical coherence tomography, Chem. Commun., 2012, 48(53), 6654–6656 RSC
.
- R. Baber, L. Mazzei, N. T. K. Thanh and A. Gavriilidis, Synthesis of silver nanoparticles in a microfluidic coaxial flow reactor, RSC Adv., 2015, 5(116), 95585–95591 RSC
.
- P. K. Singh and A. Sachdeva, Modification of properties of polymer electrolyte by incorporation of titanium dioxide nanoparticles, Mol. Cryst. Liq. Cryst., 2019, 693(1), 97–106 CrossRef
.
- A. Sachdeva and P. K. Singh, Modification of properties of polymer electrolyte by incorporation of titanium dioxide nanoparticles, Mol. Cryst. Liq. Cryst., 2020, 693(1), 97–106 CrossRef
.
- N. N. Bonnia, M. S. Kamaruddin, M. H. Nawawi, S. Ratim, H. N. Azlina and E. S. Ali, Green Biosynthesis of Silver Nanoparticles Using ‘Polygonum Hydropiper’ and Study its Catalytic Degradation of Methylene Blue, Procedia Chem., 2016, 19, 594–602 CrossRef CAS
.
- J. Valdez, S. Bawage, I. Gomez and S. R. Singh, Facile and rapid detection of respiratory syncytial virus using metallic nanoparticles, J. Nanobiotechnol., 2016, 14, 13 CrossRef PubMed
.
- I. A. Adelere and A. Lateef, Novel Approach to the Green Synthesis of Metallic Nanoparticles: The use of agro-wastes, enzymes and pigments, Nanotechnol. Rev., 2016, 5(6), 567–587 CAS
.
- C. O. Silva, P. Rijo, J. Molpeceres, L. Ascensao, A. Roberto, A. S. Fernandes, R. Gomes, J. M. Pinto Coelho, A. Gabriel, P. Vieira and C. P. Reis, Bioproduction of gold nanoparticles for photothermal therapy, Ther. Delivery, 2016, 7(5), 287–304 CrossRef CAS PubMed
.
- W. Haiss, N. T. Thanh, J. Aveyard and D. G. Fernig, Determination of size and concentration of gold nanoparticles from UV-vis spectra, Anal. Chem., 2007, 79(11), 4215–4221 CrossRef CAS PubMed
.
- S. Rajeshkumar, Anticancer activity of eco-friendly gold nanoparticles against lung and liver cancer cells, J. Genet. Eng. Biotechnol., 2016, 14(1), 195–202 CrossRef CAS PubMed
.
- N. Shokoufi, B. Abbasgholi Nejad Asbaghi and A. Abbasi-Ahd, Microfluidic chip-photothermal lens microscopy for DNA hybridization assay using gold nanoparticles, Anal. Bioanal. Chem., 2019, 411(23), 6119–6128 CrossRef CAS PubMed
.
- A. H. Mota, P. Rijo, J. Molpeceres and C. P. Reis, Broad overview of engineering of functional nanosystems for skin delivery, Int. J. Pharm., 2017, 532(2), 710–728 CrossRef CAS PubMed
.
- F. L. Filon, M. Crosera, G. Adami, M. Bovenzi, F. Rossi and G. Maina, Human skin penetration of gold nanoparticles through intact and damaged skin, Nanotoxicology, 2011, 5(4), 493–501 CrossRef CAS PubMed
.
- I. Takeuchi, T. Suzuki and K. Makino, Skin permeability and transdermal delivery route of 50-nm indomethacin-loaded PLGA nanoparticles, Colloids Surf., B, 2017, 159, 312–317 CrossRef CAS PubMed
.
- P. S. Randeria, M. A. Seeger, X. Q. Wang, H. Wilson, D. Shipp, C. A. Mirkin and A. S. Paller, siRNA-based spherical nucleic acids reverse impaired wound healing in diabetic mice by ganglioside GM3 synthase knockdown, Proc. Natl. Acad. Sci. U. S. A., 2015, 112(18), 5573–5578 CrossRef CAS PubMed
.
- J. Wang and H. S. Zhou, Aptamer-based Au nanoparticles-enhanced surface plasmon resonance detection of small molecules, Anal. Chem., 2008, 80(18), 7174–7178 CrossRef CAS PubMed
.
- A. E. Prigodich, P. S. Randeria, W. E. Briley, N. J. Kim, W. L. Daniel, D. A. Giljohann and C. A. Mirkin, Multiplexed nanoflares: mRNA detection in live cells, Anal. Chem., 2012, 84(4), 2062–2066 CrossRef CAS PubMed
.
- D. C. Yeo, C. Wiraja, A. S. Paller, C. A. Mirkin and C. Xu, Abnormal scar identification with spherical-nucleic-acid technology, Nat. Biomed. Eng., 2018, 2(4), 227–238 CrossRef CAS PubMed
.
- H. H. Lara, E. N. Garza-Trevino, L. Ixtepan-Turrent and D. K. Singh, Silver nanoparticles are broad-spectrum bactericidal and virucidal compounds, J. Nanobiotechnol., 2011, 9, 30 CrossRef CAS PubMed
.
- E. K. Poulose and S. Prabhu, Silver nanoparticles: mechanism of antimicrobial action, synthesis, medical applications, and toxicity effects, Int. Nano Lett., 2012, 2, 32 CrossRef
.
- L. Rizzello and P. P. Pompa, Nanosilver-based antibacterial drugs and devices: mechanisms, methodological drawbacks, and guidelines, Chem. Soc. Rev., 2014, 43(5), 1501–1518 RSC
.
- S. Gupta, R. Bansal, S. Gupta, N. Jindal and A. Jindal, Nanocarriers and nanoparticles for skin care and dermatological treatments, Indian Dermatol. Online J., 2013, 4(4), 267–272 CrossRef PubMed
.
- J. R. Antonio, C. R. Antonio, I. L. Cardeal, J. M. Ballavenuto and J. R. Oliveira, Nanotechnology in dermatology, An. Bras. Dermatol., 2014, 89(1), 126–136 CrossRef PubMed
.
- X. D. Wang, Z. X. Shen, T. Sang, X. B. Cheng, M. F. Li, L. Y. Chen and Z. S. Wang, Preparation of spherical silica particles by Stober process with high concentration of tetra-ethyl-orthosilicate, J. Colloid Interface Sci., 2010, 341(1), 23–29 CrossRef CAS PubMed
.
- E. Bohn, W. Stöber and A. Fink, Controlled Growth of Monodisperse Silica Spheres in the Micron Size Range, J. Colloid Interface Sci., 1968, 26(1), 62–69 CrossRef
.
- T. Hirai, T. Yoshikawa, H. Nabeshi, T. Yoshida, T. Akase, Y. Yoshioka, N. Itoh and Y. Tsutsumi, Dermal absorption of amorphous nanosilica particles after topical exposure for three days, Pharmazie, 2012, 67(8), 742–743 CAS
.
- L. Tang, C. Zhang, G. Song, X. Jin and Z. Xu,
In vivo skin penetration and metabolic path of quantum dots, Sci. China: Life Sci., 2013, 56(2), 181–188 CrossRef CAS PubMed
.
- L. Wang, G. Qin, S. Geng, Y. Dai and J. Y. Wang, Preparation of zein conjugated quantum dots and their in vivo transdermal delivery capacity through nude mouse skin, J. Biomed. Nanotechnol., 2013, 9(3), 367–376 CrossRef CAS PubMed
.
- C. S. J. Campbell, L. R. Contreras-Rojas, M. B. Delgado-Charro and R. H. Guy, Objective assessment of nanoparticle disposition in mammalian skin after topical exposure, J. Controlled Release, 2012, 162(1), 201–207 CrossRef CAS PubMed
.
- J. D. Lee, J. Y. Kim, H. J. Jang, B. M. Lee and K. B. Kim, Percutaneous permeability of 1-phenoxy-2-propanol, a preservative in cosmetics, Regul. Toxicol. Pharmacol., 2019, 103, 56–62 CrossRef CAS PubMed
.
- P. Rajitha, P. Shammika, S. Aiswarya, A. Gopikrishnan, R. Jayakumar and M. Sabitha, Chaulmoogra oil based methotrexate loaded topical nanoemulsion for the treatment of psoriasis, J. Drug Delivery Sci. Technol., 2019, 49, 463–476 CrossRef CAS
.
- I. Özcan, E. Azizoglu, T. Senyigit, M. Ozyazici and O. Ozer, Enhanced dermal delivery of diflucortolone valerate using lecithin/chitosan nanoparticles: in vitro and in vivo evaluations, Int. J. Nanomed., 2013, 8, 461–475 CrossRef PubMed
.
- A. Ostrowski, D. Nordmeyer, A. Boreham, C. Holzhausen, L. Mundhenk, C. Graf, M. C. Meinke, A. Vogt, S. Hadam, J. Lademann, E. Ruhl, U. Alexiev and A. D. Gruber, Overview about the localization of nanoparticles in tissue and cellular context by different imaging techniques, Beilstein J. Nanotechnol., 2015, 6, 263–280 CrossRef PubMed
.
- B. Bhushan, Nanotribological and nanomechanical properties of skin with and without cream treatment using atomic force microscopy and nanoindentation, J. Colloid Interface Sci., 2012, 367, 1–33 CrossRef CAS PubMed
.
- C. A. Grant, P. C. Twigg, R. Baker and D. J. Tobin, Tattoo ink nanoparticles in skin tissue and fibroblasts, Beilstein J. Nanotechnol., 2015, 6, 1183–1191 CrossRef CAS PubMed
.
- J. Raabe, G. Tzvetkov, U. Flechsig, M. Boge, A. Jaggi, B. Sarafimov, M. G. Vernooij, T. Huthwelker, H. Ade, D. Kilcoyne, T. Tyliszczak, R. H. Fink and C. Quitmann, PolLux: a new facility for soft x-ray spectromicroscopy at the Swiss Light Source, Rev. Sci. Instrum., 2008, 79(11), 113704 CrossRef CAS PubMed
.
- K. Yamamoto, R. Flesch, T. Ohigashi, S. Hedtrich, A. Klossek, P. Patoka, G. Ulrich, S. Ahlberg, F. Rancan, A. Vogt, U. Blume-Peytavi, P. Schrade, S. Bachmann, M. Schafer-Korting, N. Kosugi and E. Ruhl, Selective Probing of the Penetration of Dexamethasone into Human Skin by Soft X-ray Spectromicroscopy, Anal. Chem., 2015, 87(12), 6173–6179 CrossRef CAS PubMed
.
- P. L. Honeywell-Nguyen, G. S. Gooris and J. A. Bouwstra, Quantitative assessment of the transport of elastic and rigid vesicle components and a model drug from these vesicle formulations into human skin in vivo, J. Invest. Dermatol., 2004, 123(5), 902–910 CrossRef CAS PubMed
.
- B. D. Hanh, R. H. H. Neubert, S. Wartewig and J. Lasch, Penetration of compounds through human stratum corneum as studied by Fourier transform infrared photoacoustic spectroscopy, J. Controlled Release, 2000, 70(3), 393–398 CrossRef PubMed
.
- M. Witting, A. Boreham, R. Brodwolf, K. Vavrova, U. Alexiev, W. Friess and S. Hedtrich, Interactions of hyaluronic Acid with the skin and implications for the dermal delivery of biomacromolecules, Mol. Pharm., 2015, 12(5), 1391–1401 CrossRef CAS PubMed
.
- J. Caussin, G. S. Gooris and J. A. Bouwstra, FTIR studies show lipophilic moisturizers to interact with stratum corneum lipids, rendering the more densely packed, Biochim. Biophys. Acta, 2008, 1778(6), 1517–1524 CrossRef CAS PubMed
.
- T. R. Hata, T. A. Scholz, I. V. Ermakov, R. W. McClane, F. Khachik, W. Gellermann and L. K. Pershing, Non-invasive raman spectroscopic detection of carotenoids in human skin, J. Invest. Dermatol., 2000, 115(3), 441–448 CrossRef CAS PubMed
.
- M. C. Meinke, R. Muller, A. Bechtel, S. F. Haag, M. E. Darvin, S. B. Lohan, F. Ismaeel and J. Lademann, Evaluation of carotenoids and reactive oxygen species in human skin after UV irradiation: a critical comparison between in vivo and ex vivo investigations, Exp. Dermatol., 2015, 24(3), 194–197 CrossRef CAS PubMed
.
- P. J. Caspers, G. W. Lucassen, E. A. Carter, H. A. Bruining and G. J. Puppels,
In vivo confocal Raman microspectroscopy of the skin: noninvasive determination of molecular concentration profiles, J. Invest. Dermatol., 2001, 116(3), 434–442 CrossRef CAS PubMed
.
- P. D. Pudney, M. Melot, P. J. Caspers, A. Van Der Pol and G. J. Puppels, An in vivo confocal Raman study of the delivery of trans retinol to the skin, Appl. Spectrosc., 2007, 61(8), 804–811 CrossRef CAS PubMed
.
- Y. Zhu, C. S. Choe, S. Ahlberg, M. C. Meinke, U. Alexiev, J. Lademann and M. E. Darvin, Penetration of silver nanoparticles into porcine skin ex vivo using fluorescence lifetime imaging microscopy, Raman microscopy, and surface-enhanced Raman scattering microscopy, J. Biomed. Opt., 2015, 20(5), 051006 CrossRef PubMed
.
- C. L. Evans, E. O. Potma, M. Puoris'haag, D. Cote, C. P. Lin and X. S. Xie, Chemical imaging of tissue in vivo with video-rate coherent anti-Stokes Raman scattering microscopy, Proc. Natl. Acad. Sci. U. S. A., 2005, 102(46), 16807–16812 CrossRef CAS PubMed
.
- M. Panjehpour, C. E. Julius, M. N. Phan, T. Vo-Dinh and S. Overholt, Laser-induced fluorescence spectroscopy for in vivo diagnosis of non-melanoma skin cancers, Lasers Surg. Med., 2002, 31(5), 367–373 CrossRef PubMed
.
- C. Conte, G. Costabile, I. d'Angelo, M. Pannico, P. Musto, G. Grassia, A. Ialenti, P. Tirino, A. Miro, F. Ungaro and F. Quaglia, Skin transport of PEGylated poly(epsilon-caprolactone) nanoparticles assisted by (2-hydroxypropyl)-beta-cyclodextrin, J. Colloid Interface Sci., 2015, 454, 112–120 CrossRef CAS PubMed
.
- A. Boreham, M. Pfaff, E. Fleige, R. Haag and U. Alexiev, Nanodynamics of dendritic core-multishell nanocarriers, Langmuir, 2014, 30(6), 1686–1695 CrossRef CAS PubMed
.
- A. Boreham, J. Pikkemaat, P. Volz, R. Brodwolf, C. Kuehne, K. Licha, R. Haag, J. Dernedde and U. Alexiev, Detecting and Quantifying Biomolecular Interactions of a Dendritic Polyglycerol Sulfate Nanoparticle Using Fluorescence Lifetime Measurements, Molecules, 2015, 21(1), E22 CrossRef PubMed
.
- F. Stracke, B. Weiss, C. M. Lehr, K. Konig, U. F. Schaefer and M. Schneider, Multiphoton microscopy for the investigation of dermal penetration of nanoparticle-borne drugs, J. Invest. Dermatol., 2006, 126(10), 2224–2233 CrossRef CAS PubMed
.
- P. Volz, A. Boreham, A. Wolf, T. Y. Kim, J. Balke, J. Frombach, S. Hadam, Z. Afraz, F. Rancan, U. Blume-Peytavi, A. Vogt and U. Alexiev, Application of single molecule fluorescence microscopy to characterize the penetration of a large amphiphilic molecule in the stratum corneum of human skin, Int. J. Mol. Sci., 2015, 16(4), 6960–6977 CrossRef CAS PubMed
.
- S. B. Lohan, N. Icken, C. Teutloff, S. Saeidpour, R. Bittl, J. Lademann, E. Fleige, R. Haag, S. F. Haag and M. C. Meinke, Investigation of cutaneous penetration properties of stearic acid loaded to dendritic core-multi-shell (CMS) nanocarriers, Int. J. Pharm., 2016, 501(1–2), 271–277 CrossRef CAS PubMed
.
- S. Lohan, A.-C. Lauer, S. Arndt, A. Friedrich, K. Tscherch, S. Haag, M. Darvin, H. Vollert, A. Kleemann, I. Gersonde, N. Groth, J. Lademann, S. Rohn and M. Meinke, Determination of the Antioxidant Status of the Skin by In Vivo-Electron Paramagnetic Resonance (EPR) Spectroscopy, Cosmetics, 2015, 2(3), 286–301 CrossRef CAS
.
- S. El-Housiny, M. A. Shams Eldeen, Y. A. El-Attar, H. A. Salem, D. Attia, E. R. Bendas and M. A. El-Nabarawi, Fluconazole-loaded solid lipid nanoparticles topical gel for treatment of pityriasis versicolor: formulation and clinical study, Drug Delivery, 2018, 25(1), 78–90 CrossRef CAS PubMed
.
- S. M. Iovino, K. D. Krantz, D. M. Blanco, J. A. Fernandez, N. Ocampo, A. Najafi, B. Memarzadeh, C. Celeri, D. Debabov, B. Khosrovi and M. Anderson, NVC-422 topical gel for the treatment
of impetigo, Int. J. Clin. Exp. Pathol., 2011, 4(6), 587–595 CAS
.
- S. Salatin, F. Lotfipour and M. Jelvehgari, Preparation and characterization of a novel thermosensitive and bioadhesive cephalexin nanohydrogel: a promising platform for topical antibacterial delivery, Expert Opin. Drug Delivery, 2020, 17(6), 881–893 CrossRef CAS PubMed
.
- B. Lu, H. Ye, S. Shang, Q. Xiong, K. Yu, Q. Li, Y. Xiao, F. Dai and G. Lan, Novel wound dressing with chitosan gold nanoparticles capped with a small molecule for effective treatment of multiantibiotic-resistant bacterial infections, Nanotechnology, 2018, 29(42), 425603 CrossRef PubMed
.
- R. Y. Pelgrift and A. J. Friedman, Nanotechnology as a therapeutic tool to combat microbial resistance, Adv. Drug Delivery Rev., 2013, 65(13–14), 1803–1815 CrossRef CAS PubMed
.
- Y. Liu, H. J. Busscher, B. Zhao, Y. Li, Z. Zhang, H. C. van der Mei, Y. Ren and L. Shi, Surface-Adaptive, Antimicrobially Loaded, Micellar Nanocarriers with Enhanced Penetration and Killing Efficiency in Staphylococcal Biofilms, ACS Nano, 2016, 10(4), 4779–4789 CrossRef CAS PubMed
.
- C. Feng, J. Ouyang, Z. Tang, N. Kong, Y. Liu, L. Fu, X. Ji, T. Xie, O. C. Farokhzad and W. Tao, Germanene-Based Theranostic Materials for Surgical Adjuvant Treatment: Inhibiting Tumor Recurrence and Wound Infection, Matter, 2020, 3(1), 127–144 CrossRef
.
- J. Fu, T. Liu, X. Feng, Y. Zhou, M. Chen, W. Wang, Y. Zhao, C. Lu, G. Quan, J. Cai, X. Pan and C. Wu, A Perfect Pair: Stabilized Black Phosphorous Nanosheets Engineering with Antimicrobial Peptides for Robust Multidrug Resistant Bacteria Eradication, Adv. Healthcare Mater., 2022, 11(10), e2101846 CrossRef PubMed
.
- Y. Zheng, Y. Yan, L. Lin, Q. He, H. Hu, R. Luo, D. Xian, J. Wu, Y. Shi, F. Zeng, C. Wu, G. Quan and C. Lu, Titanium carbide MXene-based hybrid hydrogel for chemo-photothermal combinational treatment of localized bacterial infection, Acta Biomater., 2022, 142, 113–123 CrossRef CAS PubMed
.
- C. E. Griffiths, P. van de Kerkhof and M. Czarnecka-Operacz, Psoriasis and Atopic Dermatitis, Dermatol. Ther., 2017, 7(Suppl 1), 31–41 CrossRef PubMed
.
- I. Eroğlu, E. Azizoglu, M. Ozyazici, M. Nenni, H. Gurer Orhan, S. Ozbal, I. Tekmen, I. Ertam, I. Unal and O. Ozer, Effective topical delivery systems for corticosteroids: dermatological and histological evaluations, Drug Delivery, 2016, 23(5), 1502–1513 Search PubMed
.
- M. I. Asad, D. Khan, A. U. Rehman, A. Elaissari and N. Ahmed, Development and In Vitro/In Vivo Evaluation of pH-Sensitive Polymeric Nanoparticles Loaded Hydrogel for the Management of Psoriasis, Nanomaterials, 2021, 11(12), 3433 CrossRef CAS PubMed
.
- K. Vanaja, R. H. Shobha Rani and S. Sacchidananda, Formulation and Clinical Evaluation Of Ultradeformable Liposomes in the Topical Treatment of Psoriasis, Clin. Res. Regul. Aff., 2008, 25(1), 41–52 CrossRef
.
- P. Negi, I. Sharma, C. Hemrajani, C. Rathore, A. Bisht, K. Raza and O. P. Katare, Thymoquinone-loaded lipid vesicles: a promising nanomedicine for psoriasis, BMC Complementary Altern. Med., 2019, 19(1), 334 CrossRef CAS PubMed
.
- S. Trombino, R. Russo, S. Mellace, G. P. Varano, A. S. Laganà, F. Marcucci and R. Cassano, Solid lipid nanoparticles made of trehalose monooleate for cyclosporin-A topic release, J. Drug Delivery Sci. Technol., 2019, 49, 563–569 CrossRef CAS
.
- D. Fathalla, E. M. K. Youssef and G. M. Soliman, Liposomal and Ethosomal Gels for the Topical Delivery of Anthralin: Preparation, Comparative Evaluation and Clinical Assessment in Psoriatic Patients, Pharmaceutics, 2020, 12(5), 446 CrossRef CAS PubMed
.
- J. Yu, J. Wang, Y. Zhang, G. Chen, W. Mao, Y. Ye, A. R. Kahkoska, J. B. Buse, R. Langer and Z. Gu, Glucose-responsive insulin patch for the regulation of blood glucose in mice and minipigs, Nat. Biomed. Eng., 2020, 4(5), 499–506 CrossRef CAS PubMed
.
- J. Yu, Y. Zhang, Y. Ye, R. DiSanto, W. Sun, D. Ranson, F. S. Ligler, J. B. Buse and Z. Gu, Microneedle-array patches loaded with hypoxia-sensitive vesicles provide fast glucose-responsive insulin delivery, Proc. Natl. Acad. Sci. U. S. A., 2015, 112(27), 8260–8265 CrossRef CAS PubMed
.
- G. Chen, B. Hao, D. Ju, M. Liu, H. Zhao, Z. Du and J. Xia, Pharmacokinetic and pharmacodynamic study of triptolide-loaded liposome hydrogel patch under microneedles on rats with collagen-induced arthritis, Acta Pharm. Sin. B, 2015, 5(6), 569–576 CrossRef PubMed
.
- A. E. Krausz, B. L. Adler, V. Cabral, M. Navati, J. Doerner, R. A. Charafeddine, D. Chandra, H. Liang, L. Gunther, A. Clendaniel, S. Harper, J. M. Friedman, J. D. Nosanchuk and A. J. Friedman, Curcumin-encapsulated nanoparticles as innovative antimicrobial and wound healing agent, Nanomedicine, 2015, 11(1), 195–206 CrossRef CAS PubMed
.
- A. Vyas, S. K. Das, D. Singh, A. Sonker, B. Gidwani, V. Jain and M. Singh, Recent Nanoparticulate Approaches of Drug Delivery for Skin Cancer, Trends Appl. Sci. Res., 2012, 7(8), 620–635 CrossRef
.
-
E. Joseph and G. Singhvi, Multifunctional nanocrystals for cancer therapy: a potential nanocarrier, in Nanomaterials for Drug Delivery and Therapy, 2019, pp. 91–116 Search PubMed
.
- D. Bei, J. Meng and B. B. Youan, Engineering nanomedicines for improved melanoma therapy: progress and promises, Nanomedicine, 2010, 5(9), 1385–1399 CrossRef CAS PubMed
.
- S. Barua and S. Mitragotri, Challenges associated with Penetration of Nanoparticles across Cell and Tissue Barriers: A Review of Current Status and Future Prospects, Nano Today, 2014, 9(2), 223–243 CrossRef CAS PubMed
.
- J. Niu, Y. Chu, Y. F. Huang, Y. S. Chong, Z. H. Jiang, Z. W. Mao, L. H. Peng and J. Q. Gao, Transdermal Gene Delivery by Functional Peptide-Conjugated Cationic Gold Nanoparticle Reverses the Progression and Metastasis of Cutaneous Melanoma, ACS Appl. Mater. Interfaces, 2017, 9(11), 9388–9401 CrossRef CAS PubMed
.
- S. Das, J. Das, A. Samadder, A. Paul and A. R. Khuda-Bukhsh, Strategic formulation of apigenin-loaded PLGA nanoparticles for intracellular trafficking, DNA targeting and improved therapeutic effects in skin melanoma in vitro, Toxicol. Lett., 2013, 223(2), 124–138 CrossRef CAS PubMed
.
- T. Jiang, T. Wang, T. Li, Y. Ma, S. Shen, B. He and R. Mo, Enhanced Transdermal Drug Delivery by Transfersome-Embedded Oligopeptide Hydrogel for Topical Chemotherapy of Melanoma, ACS Nano, 2018, 12(10), 9693–9701 CrossRef CAS PubMed
.
- K. Jin, Z. Luo, B. Zhang and Z. Pang, Biomimetic nanoparticles for inflammation targeting, Acta Pharm. Sin. B, 2018, 8(1), 23–33 CrossRef PubMed
.
- Y. Shi, X. Feng, L. Lin, J. Wang, J. Chi, B. Wu, G. Zhou, F. Yu, Q. Xu, D. Liu, G. Quan, C. Lu, X. Pan, J. Cai and C. Wu, Virus-inspired surface-nanoengineered antimicrobial liposome: A potential system to simultaneously achieve high activity and selectivity, Bioact. Mater., 2021, 6(10), 3207–3217 CrossRef CAS PubMed
.
- L. Chen, W. Hong, W. Ren, T. Xu, Z. Qian and Z. He, Recent progress in targeted delivery vectors based on biomimetic nanoparticles, Signal Transduction Targeted Ther., 2021, 6(1), 225 CrossRef PubMed
.
- C. M. Hu, R. H. Fang, J. Copp, B. T. Luk and L. Zhang, A biomimetic nanosponge that absorbs pore-forming toxins, Nat. Nanotechnol., 2013, 8(5), 336–340 CrossRef CAS PubMed
.
- Y. K. Tak, S. Pal, P. K. Naoghare, S. Rangasamy and J. M. Song, Shape-Dependent Skin Penetration of Silver Nanoparticles: Does It Really Matter?, Sci. Rep., 2015, 5, 16908 CrossRef CAS PubMed
.
- A. Mohandas, T. R. Nimal, V. Das, S. A. Shankarappa, R. Biswas and R. Jayakumar, Drug loaded bi-layered sponge for wound management in hyperfibrinolytic conditions, J. Mater. Chem. B, 2015, 3(28), 5795–5805 RSC
.
- L. J. Liu, Q. M. Ma, S. N. Wang, Y. Gao, C. R. Zhu, W. B. Zhao, W. T. Sun, H. F. Ma and Y. Sun, Efficient epidermal delivery of antibiotics by self-assembled lecithin/chitosan nanoparticles for enhanced therapy on epidermal bacterial infections, Int. J. Biol. Macromol., 2022, 218, 568–579 CrossRef CAS PubMed
.
- Z. Sobhani, S. M. Samani, H. Montaseri and E. Khezri, Nanoparticles of Chitosan Loaded Ciprofloxacin: Fabrication and Antimicrobial Activity, Adv. Pharm. Bull., 2017, 7(3), 427–432 CrossRef CAS PubMed
.
- F. Y. Alqahtani, F. S. Aleanizy, E. El Tahir, B. T. Alquadeib, I. A. Alsarra, J. S. Alanazi and H. G. Abdelhady, Preparation, characterization, and antibacterial activity of diclofenac-loaded chitosan nanoparticles, Saudi Pharm. J., 2019, 27(1), 82–87 CrossRef PubMed
.
- K. Zhang, Y. L. Zhuang, J. W. Li, X. C. Liu and S. H. He, Poly(Acrylic Acid)-Modified MoS2 Nanoparticle-Based Transdermal Atenolol Delivery of Atenolol, Int. J. Nanomed., 2020, 15, 5517–5526 CrossRef CAS PubMed
.
- Q. Feng, Y. Liu, J. Huang, K. Chen, J. Huang and K. Xiao, Uptake, distribution, clearance, and toxicity of iron oxide nanoparticles with different sizes and coatings, Sci. Rep., 2018, 8(1), 2082 CrossRef PubMed
.
- V. J. Drew, H. Y. Huang, Z. H. Tsai, H. H. Tsai and C. L. Tseng, Preparation of gelatin/epigallocatechin gallate self-assembly nanoparticles for transdermal drug delivery, J. Polym. Res., 2017, 24(11), 1–10 CAS
.
- Y. Huang, F. Yu, Y. S. Park, J. Wang, M. C. Shin, H. S. Chung and V. C. Yang, Co-administration of protein drugs with gold nanoparticles to enable percutaneous delivery, Biomaterials, 2010, 31(34), 9086–9091 CrossRef CAS PubMed
.
- Y. Gu, M. Yang, X. Tang, T. Wang, D. Yang, G. Zhai and J. Liu, Lipid nanoparticles loading triptolide for transdermal delivery: mechanisms of penetration enhancement and transport properties, J. Nanobiotechnol., 2018, 16(1), 68 CrossRef PubMed
.
- R. R. Patlolla, P. R. Desai, K. Belay and M. S. Singh, Translocation of cell penetrating peptide engrafted nanoparticles across skin layers, Biomaterials, 2010, 31(21), 5598–5607 CrossRef CAS PubMed
.
Footnote |
† L. Liu and W. Zhao contributed equally to this work. |
|
This journal is © The Royal Society of Chemistry 2023 |