DOI:
10.1039/D2TA00225F
(Paper)
J. Mater. Chem. A, 2022,
10, 9137-9149
Comprehensive physicochemical and photovoltaic analysis of different Zn substitutes (Mn, Mg, Fe, Ni, Co, Ba, Sr) in CZTS-inspired thin film solar cells†
Received
10th January 2022
, Accepted 10th March 2022
First published on 11th March 2022
Abstract
The relatively stagnant efficiency of Cu2ZnSnS4 (CZTS) kesterite thin film solar cells has led to the exploration of alternative materials based on the kesterite structure. The unavoidable formation of Cu–Zn disorder-related defects and Sn-related deep defects such as SnZn and its cluster in CZTS prompt various attempts to substitute Zn. However, the underlying principles behind the selection of the cation substitutes remain unclear since most studies have been performed using different synthetic strategies. In this study, CXTS (X = Zn, Mn, Mg, Ni, Fe, Co, Ba, Sr) thin films are synthesized by a facile spray pyrolysis and sulfurization method, and their physical properties and device performance are compared. It is found that a majority of the compounds form a tetragonal structure (kesterite or stannite); however, Mg + CTS and Ni + CTS are unstable in their quaternary structure and form a mixture of secondary phases, while CBaTS and CSrTS form trigonal structures. From UV-Vis spectroscopy, it is found that CMnTS, CBaTS and CSrTS exhibit steep and clear absorption edges, which make them promising solar cell absorbers. Meanwhile, high carrier concentrations (>1018 cm−3) are observed for the compounds with transition metal substitutes (Mn, Mg, Ni, Fe, Co). Promising photovoltaic responses are observed in CMnTS, Mg + CTS, CBaTS and CSrTS, with CBaTS having the highest device performance possibly due to the lower band tailing, as observed from the photoluminescence and external quantum efficiency measurements. From these findings, correlations among the suitable cation substitutes for kesterite-inspired compounds are discussed and a guide for screening different cation substitutes for Zn in alternative I2-II-IV-VI4 solar cells is provided.
1. Introduction
Cu2ZnSnS4 (CZTS) is a promising candidate for low-cost and environmentally friendly thin-film solar cells, but its highest recorded efficiency is 12.6% (ref. 2 and 3) (13% for certified active area measurement4 and 13.5% with 5% Ag substitution5), which is still considerably lower than the theoretical Shockley–Queisser limit of 32.4% for CZTS.6 Although notable progress has been made in achieving a CZTS solar cell with an efficiency of around 12%, especially using various solution process strategies,7–12 the main issue originating from the high open circuit voltage (Voc) deficit still stands. Several previous studies have analyzed and reviewed the progress and performance limiting factors in kesterite.13–20 In earlier studies, CuZn antisite defects have been suggested as the main culprit for the Voc deficit (including in high quality single crystal (CZTSSe).21–28 However, recent theoretical and experimental works have found that Sn-related defects might be more influential to the Voc deficit problem.29–33 Even though Sn can be replaced by other elements, there is a limited option for Sn replacement (mainly Ge).34–41 Another alternative is to replace Zn, which forms the Sn-related deep defects SnZn. Replacing Zn with Cd10,42–47 has shown promising improvement, which is correlated to a decrease in CuZn antisite defects and the suppression of deep defects, resulting in a better carrier lifetime, sharper absorption band edge and Voc improvement. Unfortunately, Cd is not an ideal substitute due to its toxicity. There are several other possible Zn substitutes that satisfy the octet rule and are less toxic and more abundant. Table 1 lists all of the possible cations with the +2 oxidation state from different cation groups, such as alkaline earth metals, transition metals, post-transition metals, and rare-earth metals. From the list, seven cations can be considered suitable as environmentally friendly substitutes for Zn, such as Mn, Ni, Fe, Co, Mg, Sr, and Ba. All of these cations have +2 oxidation state as their stable states and have different cation radii with Zn/Cu in the 4-fold coordination.48,49 Based on the abundance (atom fraction) of the chemical elements in Earth's upper continental crust data from the United States geological survey (USGS),50–52 these elements are considered abundant (more than 10 atoms of element per 106 atoms of Si) and are not categorized as precious or rare-earth metals. According to the Agency for Toxic Substances and Disease Registry (ATSDR),53 these elements have little to no health effect after low levels of exposure (except for Ba in certain soluble compounds). The last criterion is their stability as quaternary compounds, which have been reported by several theoretical calculation or experimental findings. Several quaternary compounds (i.e., Cu2FeSnS4 (CFTS),83,84 Cu2MnSnS4 (CMnTS),85–88 Cu2SrSnS4 (CSrTS) and Cu2BaSn(S,Se)4 (CBaTS)65,66,89–92 have shown promising photovoltaic performance. As the morphology and chemical composition of the kesterite-inspired compounds often depend on the synthesis approaches, it is hard to deduce a conclusive comparison and selection criteria based on the reported data, which were synthesized and characterized using different approaches. Therefore, this study aims to comprehensively compare the cation substitute compounds based on the facile spray pyrolysis technique to fabricate CXTS (X = Mn, Mg, Ni, Fe, Co, Ba, Sr). The structural, phases, morphology and optoelectronic properties are correlated with the photovoltaic performances and their electronic configuration. Several design considerations were also drawn and summarized from this study, which may serve as a guide to designing alternative I2-II-IV-VI4 absorbers.
Table 1 List of possible cation substitutes for Zn in Cu2ZnSnS4
Cations with +2 oxidation state |
Electronic configuration |
Criteria |
Ref. |
Earth-abundant50–52 |
Non-toxic53 |
Quaternary chalcogenide |
Metastable. Toxic as soluble compounds. May induce a health hazard. |
Alkaline earth metals (group IIA) |
Beryllium (Be) |
[He]2s2 |
✓ |
✗ |
✓a |
54 and 55 |
Magnesium (Mg) |
[Ne]3s2 |
✓ |
✓ |
✓ |
56–58 |
Calcium (Ca) |
[Ar]4s2 |
✓ |
✓ |
✗ |
56 |
Strontium (Sr) |
[Kr]5s2 |
✓ |
✓ |
✓ |
59–62 |
Barium (Ba) |
[Xe]6s2 |
✓ |
✓b |
✓ |
63–66 |
Radium (Ra) |
[Rn]7s2 |
✗ |
✗ |
✗ |
|
Transition metals |
Manganese (Mn) |
[Ar]4s23d5 |
✓ |
✓ |
✓ |
67–70 |
Iron (Fe) |
[Ar]4s23d6 |
✓ |
✓ |
✓ |
71–73 |
Cobalt (Co) |
[Ar]4s23d7 |
✓ |
✓c |
✓ |
73–76 |
Nickel (Ni) |
[Ar]4s23d8 |
✓ |
✓c |
✓ |
77 and 78 |
Copper (Cu) |
[Ar]4s13d10 |
✓ |
✓ |
✓(as Cu+) |
|
Zinc (Zn) |
[Ar]4s23d10 |
✓ |
✓ |
✓ |
|
Palladium (Pd) |
[Kr]4d10 |
✗ |
✓ |
✗ |
|
Cadmium (Cd) |
[Kr]5s24d10 |
✗ |
✗ |
✓ |
79–81 |
Platinum (Pt) |
[Xe]6s14f145d9 |
✗ |
✓ |
✗ |
|
Mercury (Hg) |
[Xe]6s24f145d10 |
✗ |
✗ |
✗ |
|
Copernicium (Cn) |
[Rn]7s25f146d10 |
✗ |
✗ |
✗ |
|
Post-transition metals |
Germanium (Ge) |
[Ar]4s23d104p2 |
✗ |
✓ |
✓(as Ge4+) |
61, 68 and 82 |
Tin (Sn) |
[Kr]5s24d105p2 |
✓ |
✓ |
✓(as Sn4+) |
|
Lead (Pb) |
[Xe]6s24f145d106p2 |
✓ |
✗ |
✗ |
|
Polonium (Po) |
[Xe]6s24f145d106p4 |
✗ |
✗ |
✗ |
|
Lanthanide |
Europium (Eu) |
[Xe]6s24f7 |
✗ |
✓ |
✗ |
|
Actinides |
Nobelium (No) |
[Rn]7s25f14 |
✗ |
✗ |
✗ |
|
2. Experimental section
2.1 Cu2XSnS4 thin film preparation
The terms “Cu2XSnS4” (X = Zn, Mn, Mg, Ni, Fe, Co, Ba, Sr) in this study refer to the compounds based on the precursor solution, and do not reflect the final composition and phases in the films. The precursor solution was prepared by dissolving copper chloride dihydrate (CuCl2·2H2O, 0.072 M), tin chloride dihydrate (SnCl2·2H2O, 0.036 M) and thiourea (CH4N2S, 0.64 M) into 50 ml D.I. water. Following that, divalent cation (0.036 M) was added which either zinc chloride (ZnCl2), manganese chloride tetrahydrate (MnCl2·4H2O), magnesium chloride hexahydrate (MgCl2·6H2O), nickel chloride (NiCl2), iron(II) chloride (FeCl2), cobalt(II) chloride hexahydrate (CoCl2·6H2O), strontium chloride hexahydrate (SrCl2·6H2O) or barium chloride dihydrate (BaCl2·2H2O). The chemical precursors were purchased from Sigma Aldrich with a purity of 99.99%. There are two types of precursors made, the stoichiometric and non-stoichiometric ratios. Deviations in the non-stoichiometric ratio had Cu-poor (Cu/(Zn + Sn) = 0.75) and X-rich (X/Sn = 1.2) composition. The stoichiometric samples were used to perform the thin film characterizations, while the non-stoichiometry samples were focused on the device analysis. Dilute HCl (0.1 mol) was added to adjust the pH value to 2. It is to ensure solution stability specially to prevent SnO2 precipitation during the spraying process.93,94 Mo-coated glass substrates were cleaned using Alconox® detergent, ethanol, isopropyl alcohol (IPA) and D.I. water in an ultrasonic bath. The precursor was sprayed on substrates, which were heated. The hot plate temperature (450 °C = 280 °C substrate temperature), gas pressure (∼20 psi), and solution flow rate (1.6 ml min−1) were the spray parameters used to obtain the 1 μm thin film. The as-deposited films were sulfurized at 600 °C for 40 min in a two-zone tube furnace. The sulfur source (300 mg) in another zone of the furnace was heated at lower temperature (200 °C). The tube was filled with Ar gas (∼300 mBar).
2.3 Cu2XSnS4 device fabrication
The solar cell devices were fabricated with a Mo/CXTS/CdS/ITO/Ag-paste configuration. Chemical bath deposition (CBD) of the CdS buffer layer was operated at 80 °C for 8 min. Cd(SO4) (0.015 M), CH4N2S (0.75 M) and NH4OH (28–30%) were used to fabricate the CdS. Following that, the Sn:InO (ITO) window layers were sputtered on top of CdS with a thickness of around 100–200 nm. The devices were subjected to post-annealing treatment at 300 °C for 10 min in an Ar atmosphere (∼300 mBar). After that, the samples were manually scribed to make a grid with each square having an area of 0.16 cm2 (active area of 0.15 cm2). Finally, the Ag paste was put on top to act as an electrode.
2.4 Thin films and device characterizations
Scanning electron microscopy (SEM) and energy dispersive X-ray spectroscopy (EDS) characterizations were performed by FESEM (FESEM, JOEL, JSM-7600F). X-ray diffraction (XRD) patterns were collected by Bruker D8 Advance with Cu Kα (1.5406 Å) as the XRD source. Raman scattering spectra were measured in a backscattering configuration using Horiba Jobin-Yvon FHR-640 and Horiba Jobin-Yvon iHR-320 monochromators, both coupled with CCD detectors. The former monochromator was used together with a solid-state YAG:Nd (532 nm) laser as the excitation sources. The iHR-320 monochromator was optimized to the NIR range, and was used with a 785 nm diode laser. All spectra were normalized to the position of the main peak of monocrystalline Si at 520 cm−1. Absorption properties of the films were conducted on a Shimadzu UV-3600 apparatus with a scanning range from 400 nm to 1400 nm. An in-house built set-up was used for all of the calibrated photoluminescence measurements. For excitation, monochromatic lights of diode lasers (405 and 663 nm) were used. The beam diameter was measured with a CCD-camera, and the Gaussian spot size radius of 1.33 mm was used for probing the samples. The photon flux was set to between 1.7 × 1017 to 0.9 × 1017 photons cm−2 s−1, which is equivalent to the flux of an AM1.5 sun spectrum of 1.4 to 2 eV, respectively. For the intensity correction, the laser power was calibrated with a power meter. Hall measurements were performed on exfoliated samples (exfoliated from Mo to quartz substrate) in the parallel dipole line AC Hall setup.95,96 Device characterization, such as current density–voltage (J–V) and external quantum efficiency (EQE), were performed in a Xe-based solar simulator with Keithley 2612A and PVE300 from Bentham, respectively.
3. Results and discussions
3.1 Morphology and chemical composition of CXTS
Fig. 1 shows the cross-section SEM images of the CXTS thin films. CZTS and CMnTS have similar morphology, forming a dense film made of mostly grains equivalent to their thickness. Meanwhile, Mg + CTS and Ni + CTS films consist of small grains with an evident layered structure for Mg + CTS. CCoTS and CFeTS films exhibit a dense film with a mixture of large and small grains. In addition, the adhesion between the films and Mo substrate are poor as observed from the voids in the interface. Lastly, CBaTS and CSrTS exhibit the largest average grain sizes (Fig. S1†) compared to the other cations, even though the large grains in CSrTS are not uniformly distributed (Fig. S2†). This result is consistent with the EDS measurement, as shown in Table 2. Based on the standard deviations of elemental compositions, most of the films show lateral uniformity and homogenous chemical composition, except for Mg + CTS and CSrTS. These two compounds showed higher standard deviation than the other substitutes, which could be related to the distinct layered and non-uniform morphology in Mg + CTS and CSrTS, respectively. Further examination of CSrTS by EDS revealed that the large grain phase belongs to the CSrTS quaternary phase. Meanwhile, the small grains did not contain Sr (Fig. S3†), thus indicating the formation of binary or ternary secondary phases consisting of only Cu–Sn–S. These small grains were also observed in Mg + CTS and Ni + CTS, which might also be attributed to the formation of similar secondary phases. Deviations from the initial stoichiometric composition of the precursor were observed in all compounds to various extents, as seen from the cations' ratio. This phenomenon is commonly found during the formation of quaternary compounds. It is related to the high synthesis temperature and low partial pressure of binary sulfides, which results in cation loss, as reported in the case of CZTS.97 Further optimization on the sulfurization process for each compound needs to be done to have better control of the layer's composition.
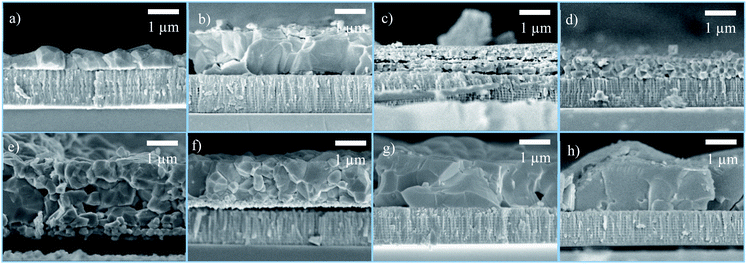 |
| Fig. 1 Cross-sectional SEM images of the CXTS films: (a) CZTS, (b) CMnTS, (c) Mg + CTS, (d) Ni + CTS, (e) CFeTS, (f) CCoTS, (g) CBaTS and (h) CSrTS. | |
Table 2 Elemental composition of CXTS from energy dispersive spectroscopy measurementsa
|
Elemental composition (at%) |
Elemental ratios |
Cu |
X |
Sn |
S |
Cu/(X + Sn) |
(X)/Sn |
(Cu + X + Sn)/S |
The elemental composition is calculated as an average of five different sampling areas from the plane-view SEM after sulfurization, and the error is the standard deviation. Elemental ratios are based on the average values elemental composition. |
CZTS |
23.2 ± 0.1 |
11.6 ± 0.2 |
13.8 ± 0.5 |
51.4 ± 0.6 |
0.91 |
0.84 |
0.94 |
CMnTS |
23.1 ± 0.6 |
11.4 ± 0.2 |
12.5 ± 0.4 |
53.0 ± 1.0 |
0.97 |
0.91 |
0.89 |
CFeTS |
25.1 ± 0.5 |
12.2 ± 0.3 |
12.5 ± 0.3 |
50.1 ± 0.6 |
1.02 |
0.97 |
0.99 |
CCoTS |
24.8 ± 0.4 |
10.2 ± 0.2 |
13.2 ± 0.1 |
51.8 ± 0.4 |
1.06 |
0.78 |
0.93 |
CBaTS |
24.1 ± 0.1 |
13.0 ± 0.2 |
11.2 ± 0.4 |
51.7 ± 0.2 |
0.99 |
1.17 |
0.93 |
CSrTS |
20.0 ± 5.8 |
12.7 ± 5.0 |
15.6 ± 1.1 |
52.5 ± 2.1 |
0.76 |
0.88 |
0.91 |
Mg + CTS |
20.8 ± 0.6 |
14.8 ± 2.4 |
11.5 ± 0.3 |
52.9 ± 1.6 |
0.80 |
1.29 |
0.89 |
Ni + CTS |
22.8 ± 0.7 |
11.7 ± 0.4 |
14.0 ± 0.5 |
51.5 ± 0.3 |
0.89 |
0.84 |
0.94 |
3.2 Crystal structure and phase analysis of the CXTS thin films
Fig. 2 exhibits the XRD pattern and Raman scattering spectra of the CXTS films. The Bragg reflections at 2θ = 40.5°, which corresponds to Mo substrate, are evident in all samples. The XRD data from the CXTS films exhibit two distinct characteristics, which can be classified into two groups of crystal system. The lower number of diffraction planes in CZTS, CMnTS, Mg + CTS, Ni + CTS, CFeTS and CCoTS indicates a higher symmetry system. These films exhibit similar strong diffraction peaks (reflections around 28° and 48°), which is common for crystal system compounds derived from a zinc blended structure, including ZnS and Cu2SnS3.98–100 Meanwhile, the higher number of diffraction planes in CBaTS and CSrTS indicates a lower symmetry system. All of the analyzed thin films can be categorized into the tetragonal group for the higher symmetry system, and the trigonal group for the lower symmetry system. These categorizations are based on the identification of their individual diffractograms with reference diffractograms and theoretical predictions.54,56,61,62,101
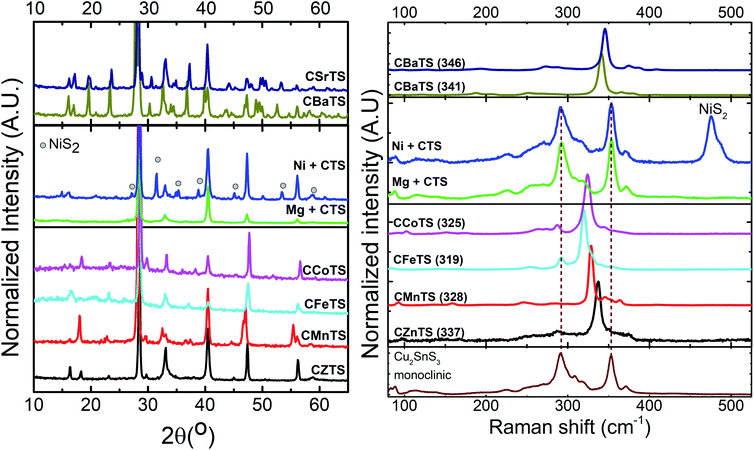 |
| Fig. 2 X-ray diffraction patterns (left) and Raman scattering spectra measured under 532 nm excitation wavelength (right) for the CXTS films. Numbers indicate the position of the most intense Raman peak of the quaternary phase. The reference Raman spectra of monoclinic Cu2SnS3 is added for convenience.1 | |
An individual investigation was conducted on each film from the tetragonal group (Fig. S4†). The CZTS, CMnTS, CFeTS and CCoTS peaks correspond to their respective tetragonal peaks in the database (JCPDS 026-0575 for CZTS, JCPDS 051-0757 for CMnTS, JCPDS 044-1476 for CFeTS and JCPDS 026-0513 for CCoTS).102,103 A systematic peak shift from the smallest cation (Co) to the largest (Mn) are observed, indicating lattice expansion due to the different ionic sizes (Fig. S5†). A peak shift is commonly found in the substitution study due to the lattice strain introduced by elements with different ionic sizes.104–106 The main peaks of Ni + CTS correspond to its quaternary compound (JCPDS 026-0552) with an obvious presence of the NiS2 phase (grey circles).107
To further identify and confirm the formation of the quaternary and/or secondary phases, all of the stoichiometric thin films were also examined by Raman spectroscopy. Fig. 2b shows the average spectra measured in different points of the thin films under 532 nm excitation wavelength, and similar spectra measured under 785 nm are presented in Fig. S6.† Analysis of the obtained Raman spectra and comparison with the previously published results21,76,86,108–113 allowed us to conclude that for most of the analyzed compounds, the quaternary phase is the dominant one. Furthermore, there was no or only a residual amount of the secondary phases present in the thin films. This is expected of the Mg + CTS and Ni + CTS compounds, for which the Raman scattering analysis exhibits only the mixture of ternary and binary secondary phases,1,107 in accordance with the XRD results.
The general shape of the obtained Raman spectra of all analyzed compounds allowed for a conclusion about the common characteristic features for the quaternaries. Independent of the crystalline structure, the spectra were characterized by one intense peak (its position is indicated in the brackets close to each compound in the left panel of Fig. 2) and a series of peaks with lower intensity. In accordance with the previous analysis in the case of the quaternary compounds crystallized with a tetragonal Bravais lattice (CZTS, CMnTS, CFeTS and CCoTS), the most intense Raman peak is assigned to the anion vibrations.21,108,110,111,113 This suggests an insignificant influence of the changes in the cation sub-lattice to the position of the main Raman peak, which is in line with the presented results. Slightly higher changes are expected in the peaks with lower intensity, and the main ones can be seen in the range of 240–300 cm−1 (see Fig. S7†). Nevertheless, the general shape of the spectra of the tetragonal quaternary compounds is quite similar even for the low intensity peaks, as seen from Fig. S7.† This, however, is not true for the two compounds crystallized with the trigonal lattice (CBaTS and CSrTS). The lower symmetry of these two compounds also results in a higher amount of expected Raman peaks (up to 138 peaks can be seen in the Raman spectra, taking into account the LO/TO splitting and the irreducible representation for the space group P31).114 As a result, the general redistribution and the appearance of the peaks with low intensity for the trigonal compounds are significantly different from those of the tetragonal compounds. However, a notable similarity can be found between the Raman spectra of CBaTS and CSrTS with only a small blue shift of the peaks of the latter, which is in accordance with the smaller size of the Sr cation and expected shorter interatomic bounds.
Several remarks can be deducted from these quaternary compound formations. In this study, Mg + CTS and Ni + CTS do not form a stable quaternary phase, while CBaTS and CSrTS form a trigonal shape instead of tetragonal. First, the unsuccessful fabrication of Mg + CTS seems to be related to the lack of a d-orbital in Mg. Although the d-orbital of Zn does not play an important role in the conduction and valence band edge formation in CZTS, it still contributes to the density of states formation.115 A similar case for the remaining cations in this study (Fe, Co, Mn, Ba and Sr), their d-orbital also contributes to the density of states formation of CFeTS, CCoTS, CMnTS, CBaTS and CSrTS.54,61,64,116,117 Even in CMnTS, the Mn d-orbital plays a more important role as it hybridizes with the s and p-orbitals to form a conduction band minimum (CBM).118 Second, in the case of the Ni + CTS formation, there is a high similarity between its secondary phase and quaternary phase structure, which tends to make the phase separation easily occur. Ni + CTS has been shown (theoretically and experimentally) to have a stable quaternary P
2c polytype cubic phase, which has a similar cubic phase with its secondary phase NiS2.73,78,119–122 Thus, it may be more difficult to obtain the quaternary compound and reaction kinetics during the growth of Ni + CTS must be carefully controlled to obtain a pure quaternary compound.123 In addition, Ni has the smallest ionic radius in 4-fold coordination among the transition metal substitutes, which can increase the likelihood of Ni leaving the lattice.49 Lastly, as observed in CBaTS and CSrTS, it emphasizes the importance of the ionic radius difference between the substitute and the other host cations (Cu and Sn in this study). If the ionic size difference is too large, a different structure will be formed.
3.3 Optoelectronic properties of the CXTS thin films
Fig. 3 shows the absorption spectra for the CXTS samples by UV-Vis spectroscopy. The spectra of the samples are classified into 4 figures based on the similarity in the absorption spectra shape, i.e., CZTS and CMnTS, Mg + CTS and Ni + CTS, CFeTS and CCoTS, CBaTS and CSrTS. The partially filled orbital configuration of CFeTS and CCoTS might have different absorption profiles compared to the half-filled orbital CMnTS and fully-filled orbital CZTS.124 Meanwhile, Mg + CTS and Ni + CTS are similar as they possess multiple absorption edges, which correspond to multiple band gaps due to the formed mixture of the secondary phases, including the formation of different polymorphs of the Cu–Sn–S system.1,98 CBaTS and CSrTS have absorption edges at low wavelength. However, CSrTS exhibits a prominent low band gap absorption, which can be attributed to the small grains of the Cu2SnS3 phase found in the EDS analysis. CZTS, CMnTS, CBaTS and CSrTS show steep absorption edges, while the rest have more gradual absorption edges.
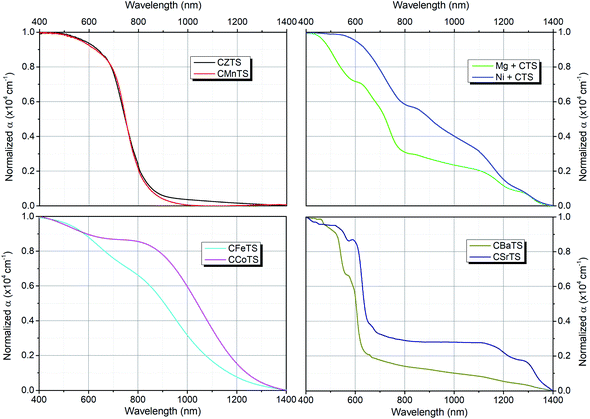 |
| Fig. 3 Normalized absorption spectra for the CXTS films. | |
From the absorption curve, the band gaps of these films were extracted based on Tauc plot (Fig. S8†)125 and are tabulated in Table 3. The band gaps of CZTS, CMnTS, CFeTS, CSrTS and CBaTS are comparable with the previous findings.60,62,66,87,126–129 However, the CSrTS sample also exhibits two smaller inflection points, which can be attributed to the band gap of the Cu2SnS3 secondary phase (∼0.92–0.95 eV).1,130 In Mg + CTS and Ni + CTS, these correspond to the ternary phases' band gaps, orthorhombic Cu3SnS4 (1.57 eV)130 and monoclinic Cu2SnS3 (1.10 and ∼1.00 eV).1,130 As for CCoTS, the band gap is estimated to be at a relatively smaller value than the reported values around 1.4–1.6 eV,75,76,131 which might be due to severe band tailing for CCoTS in this study.
Table 3 Summary of the CXTS thin film characterization
Sample |
Structure |
Secondary phases |
Band gap (eV) |
Morphology |
Hole concentration (cm−3) |
Hole mobility (cm2 V−1 s−1) |
CZTS |
Tetragonal (kesterite) I![[4 with combining macron]](https://www.rsc.org/images/entities/char_0034_0304.gif) |
Cu3SnS4 |
1.60 |
Grain size ≈ thickness |
2.55 × 1015 |
0.923 |
CMnTS |
Tetragonal (stannite) I 2m |
SnSx, Mn–S |
1.60 |
Grain size ≈ thickness |
2.56 × 1019 |
0.501 |
CFeTS |
Tetragonal (stannite) I 2m |
— |
1.20 |
Poor adhesion with Mo (MoS2) |
1.91 × 1019 |
1.107 |
CCoTS |
Tetragonal (stannite) I 2m |
— |
1.10 |
Poor adhesion with Mo (MoS2) |
2.95 × 1021 |
0.466 |
CBaTS |
Trigonal P31 |
— |
2.00 |
Large grains, grain size ≈ thickness |
6.83 × 1015 |
2.134 |
CSrTS |
Trigonal P3221 |
Cu–Sn–S phases |
1.88 |
Large grains, non-uniform |
2.14 × 1018 |
2.762 |
Mg + CTS |
— |
Orthorhombic Cu3SnS4, monoclinic Cu2SnS3 |
1.57 |
Small grains, non-uniform (layered) |
2.92 × 1018 |
1.083 |
Ni + CTS |
— |
NiS2(cubic), monoclinic Cu2SnS3 |
1.10 |
Small grains |
1.97 × 1019 |
1.579 |
In terms of the electronic properties, all films possess p-type conductivity based on the Hall measurement. The carrier concentration and mobility of these films are tabulated in Table 3. In terms of the carrier concentration, CZTS and CBaTS have similar values around 1015 cm−3, which is suitable for the solar cell material.132 However, the carrier concentration of the other films are >1018 cm−3, which have been shown to possess poor photovoltaic performances.86,133 The high concentration for Mg + CTS, Ni + CTS and CSrTS may be attributed to the Cu2SnS3 secondary phase.98 In terms of mobility, all of the films have mobility around 0.5–2.7 cm2 V−1 s−1, where CBaTS and CSrTS have the highest mobility.
The transition metal candidates (Fe, Ni, Co and Mn) exhibit high carrier concentration, which seems to be related to their multivalent properties. All of these metals possess multiple oxidation states due to their partially filled d-orbitals. Mn is expected to be more stable as the only one with half-filled 3d5-orbitals,134 while the rest of the substitutes possess a partially filled orbital.135 The compound could even be more stable and show potential in suppressing defects if the elemental substitute has a fully filled orbital as observed in Cd.44,46,47 The unpaired electrons in the partially filled orbital contributes to the high carrier concentration either as a free electron or in traps. As for CBaTS and CSrTS, the different crystal structures result in a higher band gap and lower carrier concentration. However, the conducting secondary phase for CSrTS in this study increases the carrier concentration significantly.
3.4 Device characterization and analysis of the CXTS solar cells
Following the thin film studies, solar cell devices (Mo/CXTS/CdS/i-ZnO/ITO/Ag-paste) were fabricated and measured to further understand the effect of different cations on the photovoltaic performance. The devices were fabricated under several different conditions, such as stoichiometric and non-stoichiometric ratios (Cu-poor, X-rich), without post-annealing and post-ITO deposition annealing. The non-stoichiometric ratio and post-annealing after ITO depositions are a commonly reported strategy in boosting the CZTS performance.93,136–139 Fig. S9† shows the statistical solar cell parameters of the non-stoichiometric (Cu-poor and X-rich) CXTS devices with and without post annealing. The non-filled and striped box represent solar cell devices without and with post-device annealing, respectively.
The CXTS devices with the non-stoichiometric condition perform better than the stoichiometric one, especially Voc (Fig. S10†). CMnTS, Mg + CTS, CBaTS and CSrTS show noticeable photovoltaic responses. On the other hand, devices with and without post annealing show that Ni + CTS, CFeTS and CCoTS does not produce photovoltaic response, even though some literature reported photovoltaic response for these three compounds.77,83,140,141 This finding is also in line with the absorption edges gradient of the thin films where the four substitutes with steep slope show photovoltaic performance. Several reasons can be attributed to this finding. First, the Ni + CTS quaternary compound does not seem to form and there are prominent NiS2 secondary phases in this study. Second, the morphologies of CFeTS and CCoTS suffer from the poor adhesion with Mo, which creates an issue for the photovoltaic device. Lastly, in the reported literature, the evident photovoltaic performances for these three compounds are observed in different device architectures, such as using different buffer layers (Bi2S3 for CFeTS83) and in a sensitized solar cell structure (ZnO/ZnS nanorods141). The post-device annealing also exhibits improvement for all films, except for CBaTS. Redistributions of the cations and anions were commonly observed as the effect of these post-device annealing events. As CBaTS does not seem to suffer from a disordered structure like kesterite CZTS,61,142 it is possible that the post-device annealing disrupts the structure, instead of improving it.
The J–V characteristics of the respective CXTS “champion” cells after annealing under one sun illumination is shown in Fig. 4a. Ni + CTS, CFeTS and CCoTS displayed an ohmic response, which illustrates the non-diode response from these materials. Among these cells, CZTS as a reference performs the best with an efficiency of 5.66%. CBaTS exhibits the second best performing cells, and is the best among the substitutes with an efficiency of 1.06%. The low Jsc for CBaTS is expected due to its high band gap, but the Voc is quite low with respect to the band gap. In comparison with the literature, the highest efficiency CBaTS is achieved at 1.7% with Voc being ∼0.2 V higher than in this study.66 The Voc deficit might be due to the unoptimized cation ratio, deposition parameters or buffer layer (CdS might not have the best band alignment) and possible secondary phase. Nevertheless, this result is comparable with the highest reported, and further optimization is expected to improve the performance. The efficiency of CMnTS (η = 0.56%) is also not far behind the record in the literature (η = 0.91%).87 In fact, the Voc and fill factor in this study is slightly higher than the record, while the decreased efficiency is governed by the lower Jsc, which can be improved by thickness optimization. It should be noted that the photovoltaic responses of Mg + CTS and CSrTS are most likely attributed to the CTS secondary phases. In general, the poor performances for some of these devices can be attributed to the suboptimal morphology, high carrier concentration, low mobility and secondary phases of the films.
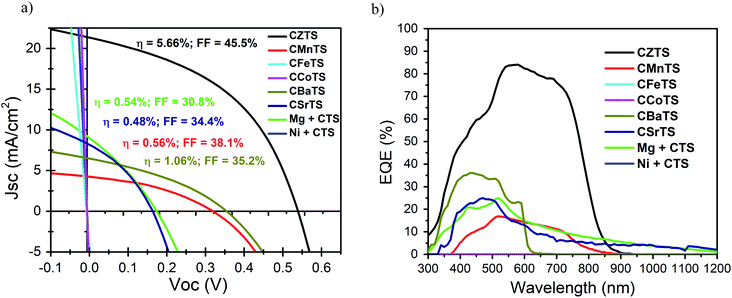 |
| Fig. 4 (a) J–V curve for the “champion” cell from each of the CXTS films. (b) External quantum efficiency for the CXTS solar cells with their respective extracted band gap and Jsc. Each CXTS film (represented by different colors) has two EQE responses; the solid line represents the post annealed film response and dashed line represents the response for the film without post annealing. | |
To further understand the charge collection of these films, external quantum efficiency measurements were performed on the CXTS devices. From Fig. 4b, only CZTS, CMnTS, Mg + CTS, CBaTS and CSrTS show quantum efficiency signals, which is consistent with the photovoltaic response. A similar trend with their respective photovoltaic performances is also observed. The EQEs of Mg + CTS and CSrTS show extended gradual decreasing slopes, indicating the presence of low band gap CTS secondary phases. The sharp drop around 600 nm for CBaTS indicates low band fluctuation and tailing, which is an issue in CZTS,143,144 while its relatively high band gap makes this compound promising for the semitransparent and tandem applications.
A photoluminescence study was also performed on the five samples, which exhibited the photovoltaic response (Fig. S11†). Table 4 tabulates the extracted parameters from the PL response, details on the parameter extraction can be found in a previous study.145 All of the measurements were performed at room temperature, and were spectrally corrected by using a commercially calibrated halogen lamp with a known spectrum and absolutely calibrated with the help of a power meter. All of the absorbers were passivated with 50 nm of the CdS layer by chemical bath deposition. The PL measurements, except for Sr and Ba substituted CXTS samples, were carried out at room temperature with a 663 nm wavelength diode laser as the excitation source. Since the band gaps of the Sr and Ba-substituted CXTS samples are relatively higher, a blue laser (405 nm) was used as the excitation source. Although the excitation wavelength of the laser is higher than the bandgap of CdS, the CdS thickness is negligible, and the absorption of the passivated layer is nearly negligible in the band gap region of the absorber. The external radiative efficiency (ERE) deduced from the calibrated PL spectrum was then used to determine the QFLS,
QFLS = VSQoc + (KT/q)ln(ERE) |
where

and
VSQoc = 0.93(
EPL/
q) − 0.176 V.
145
Table 4 Extracted parameters from the photoluminescence for the samples with a photovoltaic responsea
|
E g (eV) |
ERE (%) |
E PL (eV) |
V oc[SQ] (eV) |
QFLS (eV) |
QFLS loss (eV) |
V oc (eV) |
QFLS-Voc (eV) |
ERE = external radiative efficiency, Eg = band gap from UV-Vis, EPL = photoluminescence peak, Voc[SQ] = Voc based on the Shockley Quisser limit from the EPL, QFLS = quasi Fermi level splitting. |
CZTS |
1.6 |
1.904 × 10−4 |
1.260 |
0.996 |
0.667 |
0.329 |
0.581 |
0.086 |
CMnTS |
1.6 |
2.697 × 10−5 |
1.357 |
1.085 |
0.707 |
0.378 |
0.345 |
0.362 |
CBaTS |
2 |
7.74 × 10−3 |
1.133 |
0.878 |
0.641 |
0.237 |
0.380 |
0.261 |
CSrTS |
0.92, 0.95, 1.88 |
2.74 × 10−3 |
1.155 |
0.898 |
0.635 |
0.263 |
0.181 |
0.454 |
Mg + CTS |
1, 1.1, 1.57 |
3.920 × 10−5 |
0.920 |
0.678 |
0.310 |
0.368 |
0.191 |
0.119 |
CZTS and CMnTS exhibit well defined single peaks in their PL spectra (1.26 and 1.357 eV, respectively), with the EPL value closer to their respective Eg. This indicates that the PL response is dominated by the quaternary compounds without significant secondary phase contributions in comparison with the other compounds. The significant differences between the Eg and EPL in these two compounds highlight the poor performances due to recombination losses. The EPL, which are less than Eg, may indicate radiative recombination via deep tail states (band-tail to band-tail recombination).146 It is also possible that the free-to-bound transition occurs due to the acceptor state recombination.147 In conjunction with the low mobility, these findings also imply the lower carrier lifetime issue. Meanwhile, the spectral responses of the Mg + CTS, CBaTS and CSrTS films seem to be dominated by their secondary phases or deep defects (Fig. S11†), which explains the very high carrier concentration and poor performances of these devices. Mg + CTS, which forms a lower bandgap Cu–Sn–S secondary phase in this study, shows EPL around the band gap of Cu–Sn–S. As for CBaTS and CSrTS, the PL peak from their respective quaternary compounds was found to be very close to their Eg at the high energy level. However, there are other more dominant broad peaks at lower energy levels. These can be related to the secondary phases with a lower band gap or deep defects in these structures, which is often found to generate high density of bulk recombination centers.89 The ERE and QFLS loss in CBaTS and CSrTS are more promising than CZTS. The ERE is defined as a ratio between the emitted photon flux and excitation flux, which means that the quality of the radiative emission in these two films seems to be better than CZTS in this study.148 The QFLS loss is quantified as the difference between the Voc[SQ] and the QFLS. This can be related to the non-radiative recombination quality of the film,149 which shows that CBaTS and CSrTS have fewer non-radiation recombinations than the other films. The last parameter is the difference between QFLS and the devices' Voc, which can indicate the recombination at the interface.150,151 The results show that the four cation substitutes showing photovoltaic responses have more detrimental interface properties than CZTS. This indicates the need for alternative buffer layers, as some of these compounds have a larger band gap than CZTS, which makes CdS an unsuitable choice. Interface treatment might also be required to improve the device performance of these films. A previous study reported that the combination of CBaTS or CSrTS with a CdS buffer layer is not optimal because the conduction bands of CBTS and CSTS lie at significantly higher energy levels than in CIGS and CZTS. Therefore, although CBaTS and CSrTS are promising alternatives due to less recombination near the band edge based on PL and EQE, the deep defects and interface recombination require further optimization and alternative device structure to achieve good power conversion efficiency.
4. Conclusion
In summary, investigation on the effect of different cation substitutes for Zn in CZTS has been performed using the spray pyrolysis method, including the emerging material CBaTS and CSrTS thin films. Initial screenings of candidates based on their abundance, toxicity and stability predictions as quaternary compounds resulted in seven potential candidates (Mn, Mg, Ni, Fe, Co, Ba and Sr).
After performing both thin film and solar cell device characterizations on various CXTS compounds, the following interpretations and design considerations can be concluded:
(a) The cation substitute must have d-orbitals to replace Zn and form a stable quaternary compound, as observed with the unsuccessful attempt to form Mg + CTS.
(b) The partially filled 3d-orbital of Fe, Ni, Co and Mn in this study increased the carrier concentration due to possible oxidation state changes and additional free carriers.
(c) The large ionic difference between the substitute and the host Cu and Sn may result in different quaternary crystal structures and optoelectronic properties. The change might also be a beneficial one, as observed in the sharp EQE for CBaTS.
(d) For substitutes that have similar structures between their secondary phases and quaternary phase, such as Ni + CTS, the fabrication needs to be done in a more controlled method to achieve a pure quaternary compound.
We found a notable photovoltaic response for CZTS, CMnTS, CBaTS, and CSrTS, and an ohmic response for Ni + CTS, CFeTS and CCoTS. This correlates with the large grain size and sharper UV-Vis and EQE band edges in the first four compounds. On the other hand, the last 3 compounds without a PV effect do not form a quaternary compound, do not show any PL emission under 1 sun excitation, and/or have a small grain size and poor interface with Mo. This suggests that more specific synthesis parameters or post-synthesis treatments should be applied in the case of the Ni, Fe and Co substituent elements.
The photovoltaic response and the formation of a stable quaternary compound for CMnTS, CBaTS and CSrTS show the potential as emerging kesterite-inspired PV materials. The photoluminescence results also highlight the positive indication that CBaTS and CSrTS reduce defects near the band edges, but their deep defects and interface recombination have to be resolved. Finally, further optimization pathways should be considered to improve the photovoltaic response of these alternative emerging absorbers, such as finding the optimized stoichiometric ratio (e.g., the cations ratio) for each compound, optimizing the annealing parameters and/or different device configurations (e.g., different buffer layers).
Conflicts of interest
There are no conflicts to declare.
Acknowledgements
The authors acknowledge funding support from the CREATE Programme under the Campus for Research Excellence and Technological Enterprise (CREATE), which is supported by the National Research Foundation, Prime Minister's Office, Singapore; and Ministry of Education (MOE) Tier 2 Project (MOE2016-T2-1-030). Support by the Luxembourgish Fonds National de la Recherche FNR in the framework of the project CASK is acknowledged. The authors from IREC belong to the SEMS (Solar Energy Materials and Systems) Consolidated Research Group of the “Generalitat de Catalunya” (ref. 2017 SGR 862), and are grateful to the European Regional Development Funds (ERDF, FEDER Programa Competitivitat de Catalunya 2007–2013). M. G. acknowledges financial support from the Spanish Ministry of Science, Innovation and Universities within the Juan de la Cierva fellowship (IJC2018-038199-I).
References
- F. Oliva, L. Arqués, L. Acebo, M. Guc, Y. Sánchez, X. Alcobé, A. Pérez-Rodríguez, E. Saucedo and V. Izquierdo-Roca, J. Mater. Chem. A, 2017, 5, 23863–23871 RSC.
- W. Wang, M. T. Winkler, O. Gunawan, T. Gokmen, T. K. Todorov, Y. Zhu and D. B. Mitzi, Adv. Energy Mater., 2014, 4, 1301465 CrossRef.
- D.-H. Son, S.-H. Kim, S.-Y. Kim, Y.-I. Kim, J.-H. Sim, S.-N. Park, D.-H. Jeon, D.-K. Hwang, S.-J. Sung and J.-K. Kang, J. Mater. Chem. A, 2019, 7, 25279–25289 RSC.
- Y. Gong, Y. Zhang, Q. Zhu, Y. Zhou, R. Qiu, C. Niu, W. Yan, W. Huang and H. Xin, Energy Environ. Sci., 2021, 14, 2369–2380 RSC.
- Y. Gong, R. Qiu, C. Niu, J. Fu, E. Jedlicka, R. Giridharagopal, Q. Zhu, Y. Zhou, W. Yan, S. Yu, J. Jiang, S. Wu, D. S. Ginger, W. Huang and H. Xin, Adv. Funct. Mater., 2021, 31, 2101927 CrossRef CAS.
- W. Ki and H. W. Hillhouse, Adv. Energy Mater., 2011, 1, 732–735 CrossRef CAS.
- Y. Sun, P. Qiu, W. Yu, J. Li, H. Guo, L. Wu, H. Luo, R. Meng, Y. Zhang and S. Liu, Adv. Mater., 2021, 33, 2104330 CrossRef CAS PubMed.
- X. Xu, L. Guo, J. Zhou, B. Duan, D. Li, J. Shi, H. Wu, Y. Luo and Q. Meng, Adv. Energy Mater., 2021, 11, 2102298 CrossRef CAS.
- Y. Zhao, X. Zhao, D. Kou, W. Zhou, Z. Zhou, S. Yuan, Y. Qi, Z. Zheng and S. Wu, ACS Appl. Mater. Interfaces, 2021, 13, 795–805 CrossRef CAS PubMed.
- Z. Su, G. Liang, P. Fan, J. Luo, Z. Zheng, Z. Xie, W. Wang, S. Chen, J. Hu, Y. Wei, C. Yan, J. Huang, X. Hao and F. Liu, Adv. Mater., 2020, 32, 2000121 CrossRef CAS PubMed.
- Y. Gong, Y. Zhang, E. Jedlicka, R. Giridharagopal, J. A. Clark, W. Yan, C. Niu, R. Qiu, J. Jiang, S. Yu, S. Wu, H. W. Hillhouse, D. S. Ginger, W. Huang and H. Xin, Sci. China Mater., 2021, 64, 52–60 CrossRef CAS.
- J. Li, Y. Huang, J. Huang, G. Liang, Y. Zhang, G. Rey, F. Guo, Z. Su, H. Zhu, L. Cai, K. Sun, Y. Sun, F. Liu, S. Chen, X. Hao, Y. Mai and M. A. Green, Adv. Mater., 2020, 32, 2005268 CrossRef PubMed.
- S. K. Wallace, D. B. Mitzi and A. Walsh, ACS Energy Lett., 2017, 2, 776–779 CrossRef CAS.
- S. Hadke, M. Huang, C. Chen, Y. F. Tay, S. Chen, J. Tang and L. Wong, Chem. Rev., 2021 DOI:10.1021/acs.chemrev.1c00301.
- K. Pal, P. Singh, A. Bhaduri and K. B. Thapa, Sol. Energy Mater. Sol. Cells, 2019, 196, 138–156 CrossRef CAS.
- F. Liu, S. Wu, Y. Zhang, X. Hao and L. Ding, Sci. Bulletin, 2020, 65, 698–701 CrossRef CAS.
- M. Ravindiran and C. Praveenkumar, Renew. Sustain. Energy Rev., 2018, 94, 317–329 CrossRef CAS.
- S. Giraldo, Z. Jehl, M. Placidi, V. Izquierdo-Roca, A. Pérez-Rodríguez and E. Saucedo, Adv. Mater., 2019, 31, 1806692 CrossRef PubMed.
- M. Baid, A. Hashmi, B. Jain, A. K. Singh, M. A. B. H. Susan and M. Aleksandrova, Opt. Quant. Electron., 2021, 53, 656 CrossRef CAS.
- M. Kumar, A. Dubey, N. Adhikari, S. Venkatesan and Q. Qiao, Energy Environ. Sci., 2015, 8, 3134–3159 RSC.
- M. Guc, S. Levcenko, I. V. Bodnar, V. Izquierdo-Roca, X. Fontane, L. V. Volkova, E. Arushanov and A. Pérez-Rodríguez, Sci. Rep., 2016, 6, 19414 CrossRef CAS PubMed.
- S. Levcenko, V. E. Tezlevan, E. Arushanov, S. Schorr and T. Unold, Phys. Rev. B, 2012, 86(4), 045206 CrossRef.
- A. Nagaoka, T. Masuda, S. Yasui, T. Taniyama and Y. Nose, Jpn. J. Appl. Phys., 2018, 57, 101201 CrossRef.
- S. Podsiadlo, M. Bialoglowski, M. Fadaghi, W. Gebicki, C. Jastrzebski, E. Zero, D. Trzybinski and K. Wozniak, Cryst. Res. Technol., 2015, 50, 690–694 CrossRef CAS.
- K. Rudisch, A. Davydova, C. Platzer-Björkman and J. Scragg, J. Appl. Phys., 2018, 123, 161558 CrossRef.
- A. Ritscher, M. Hoelzel and M. Lerch, J. Solid State Chem., 2016, 238, 68–73 CrossRef CAS.
- G. Rey, A. Redinger, J. Sendler, T. P. Weiss, M. Thevenin, M. Guennou, B. El Adib and S. Siebentritt, Appl. Phys. Lett., 2014, 105, 112106 CrossRef.
- T. Gokmen, O. Gunawan, T. K. Todorov and D. B. Mitzi, Appl. Phys. Lett., 2013, 103, 103506 CrossRef.
- S. Kim, J.-S. Park and A. Walsh, ACS Energy Lett., 2018, 3, 496–500 CrossRef CAS.
- Y. Gong, Y. Zhang, E. Jedlicka, R. Giridharagopal, J. A. Clark, W. Yan, C. Niu, R. Qiu, J. Jiang, S. Yu, S. Wu, H. W. Hillhouse, D. S. Ginger, W. Huang and H. Xin, Sci. China Mater., 2020, 64, 52–60 CrossRef.
- J. S. Park, S. Kim, Z. Xie and A. Walsh, Nat. Rev. Mater., 2018, 3, 194–210 CrossRef CAS.
- S. Giraldo, Z. Jehl, M. Placidi, V. Izquierdo-Roca, A. Pérez-Rodríguez and E. Saucedo, Adv. Mater., 2019, 31, 1806692 CrossRef PubMed.
- S. Kim, J. A. Márquez, T. Unold and A. Walsh, Energy Environ. Sci., 2020, 13, 1481–1491 RSC.
- S. Kim, K. M. Kim, H. Tampo, H. Shibata and S. Niki, Appl. Phys. Express, 2016, 9, 102301 CrossRef.
- I. Kim, K. Kim, Y. Oh, K. Woo, G. Cao, S. Jeong and J. Moon, Chem. Mater., 2014, 26, 3957–3965 CrossRef CAS.
- A. Collord and H. Hillhouse, Chem. Mater., 2016, 28, 2067–2073 CrossRef CAS.
- K. Tsuji, T. Maeda and T. Wada, Jpn. J. Appl. Phys., 2018, 57, 08RC21 CrossRef.
- S. Levcenko, M. Guc, C. Merschjann, G. Gurieva, S. Schorr, M. Lux-Steiner and E. Arushanov, Phys. Status Solidi C, 2013, 10, 1079–1081 CrossRef CAS.
- L. Choubrac, M. Bär, X. Kozina, R. Felix, R. G. Wilks, G. Brammertz, S. Levcenko, L. Arzel, N. Barreau and S. Harel, ACS Appl. Energy Mater., 2020, 3, 5830–5839 CrossRef CAS.
- T. Schnabel, M. Seboui, A. Bauer, L. Choubrac, L. Arzel, S. Harel, N. Barreau and E. Ahlswede, RSC Adv., 2017, 7, 40105–40110 RSC.
- R. B. Wexler, G. S. Gautam and E. A. Carter, J. Mater. Chem. A, 2021, 9, 9882–9897 RSC.
- Z. Su, J. M. R. Tan, X. Li, X. Zeng, S. K. Batabyal and L. H. Wong, Adv. Energy Mater., 2015, 5(19), 1500682 CrossRef.
- R. Sun, D. Zhuang, M. Zhao, Q. Gong, M. Scarpulla, Y. Wei, G. Ren and Y. Wu, Sol. Energy Mater. Sol. Cells, 2018, 174, 494–498 CrossRef CAS.
- C. Yan, K. Sun, J. Huang, S. Johnston, F. Liu, B. P. Veettil, K. Sun, A. Pu, F. Zhou, J. A. Stride, M. A. Green and X. Hao, ACS Energy Lett., 2017, 2, 930–936 CrossRef CAS.
- S. H. Hadke, S. Levcenko, S. Lie, C. J. Hages, J. A. Márquez, T. Unold and L. H. Wong, Adv. Energy Mater., 2018, 8, 1802540 CrossRef.
- S. Hadke, W. Chen, J. M. R. Tan, M. Guc, V. Izquierdo-Roca, G.-M. Rignanese, G. Hautier and L. H. Wong, J. Mater. Chem. A, 2019, 7, 26927–26933 RSC.
- S. Hadke, S. Levcenko, G. Sai Gautam, C. J. Hages, J. A. Márquez, V. Izquierdo-Roca, E. A. Carter, T. Unold and L. H. Wong, Adv. Energy Mater., 2019, 9, 1902509 CrossRef CAS.
- N. N. Greenwood and A. Earnshaw, Chemistry of the Elements, Elsevier, 2012 Search PubMed.
- R. Shannon, Acta Crystallogr. A, 1976, 32, 751–767 CrossRef.
- F. W. Clarke and H. S. Washington, The Composition of the Earth's Crust, US Government Printing Office, 1924 Search PubMed.
- G. B. Haxel, J. B. Hedrick and G. J. Orris, Rare Earth Elements—Critical Resources for High Technology, 2002, https://pubs.usgs.gov/fs/2002/fs087-02 Search PubMed.
- USGS, Commodity Statistics Information, https://minerals.usgs.gov/minerals/pubs/commodity/.
- Substance Listing - Letter A|Toxic Substance Portal|ATSDR, 2021, https://wwwn.cdc.gov/TSP/substances/SubstanceAZ.aspx Search PubMed.
- R. Chen and C. Persson, J. Appl. Phys., 2017, 121, 203104 CrossRef.
- L. Weston and C. Stampfl, Phys. Rev. Mater., 2018, 2, 085407 CrossRef CAS.
- G. Zhong, K. Tse, Y. Zhang, X. Li, L. Huang, C. Yang, J. Zhu, Z. Zeng, Z. Zhang and X. Xiao, Thin Solid Films, 2016, 603, 224–229 CrossRef CAS.
- G. Yang, X. Zhai, Y. Li, B. Yao, Z. Ding, R. Deng, H. Zhao, L. Zhang and Z. Zhang, Mater. Lett., 2019, 242, 58–61 CrossRef CAS.
- M. Wei, Q. Du, R. Wang, G. Jiang, W. Liu and C. Zhu, Chem. Lett., 2014, 43, 1149–1151 CrossRef CAS.
- C. L. Teske, Z. Anorg. Allg. Chem., 1976, 419, 67–76 CrossRef CAS.
- Z. Tong, J. Yuan, J. Chen, A. Wu, W. Huang, C. Han, Q. Cai, C. Ma, Y. Liu, L. Fang and Z. Liu, Mater. Lett., 2019, 237, 130–133 CrossRef CAS.
- T. Zhu, W. P. Huhn, G. C. Wessler, D. Shin, B. Saparov, D. B. Mitzi and V. Blum, Chem. Mater., 2017, 29, 7868–7879 CrossRef CAS.
- F. Hong, W. Lin, W. Meng and Y. Yan, Phys. Chem. Chem. Phys., 2016, 18, 4828–4834 RSC.
- D. Shin, E. Ngaboyamahina, Y. Zhou, J. T. Glass and D. B. Mitzi, J. Phys. Chem. Lett., 2016, 7, 4554–4561 CrossRef CAS PubMed.
- Z. Xiao, W. Meng, J. V. Li and Y. Yan, ACS Energy Lett., 2016, 29–35, DOI:10.1021/acsenergylett.6b00577.
- D. Shin, T. Zhu, X. Huang, O. Gunawan, V. Blum and D. B. Mitzi, Adv. Mater., 2017, 29(24), 1606945 CrossRef PubMed.
- Z. Chen, K. Sun, Z. Su, F. Liu, D. Tang, H. Xiao, L. Shi, L. Jiang, X. Hao and Y. Lai, ACS Appl. Energy Mater., 2018, 1, 3420–3427 CrossRef CAS.
- T. Fries, Y. Shapira, F. Palacio, M. C. Morón, G. J. McIntyre, R. Kershaw, A. Wold and E. J. McNiff, Phys. Rev. B, 1997, 56, 5424–5431 CrossRef CAS.
- L. Guen and W. S. Glaunsinger, J. Solid State Chem., 1980, 35, 10–21 CrossRef CAS.
- X. Liang, P. Guo, G. Wang, R. Deng, D. Pan and X. Wei, RSC Adv., 2012, 2, 5044 RSC.
- L. Chen, H. Deng, J. Tao, H. Cao, L. Huang, L. Sun, P. Yang and J. Chu, RSC Adv., 2015, 5, 84295–84302 RSC.
- G. P. Bernardini, D. Borrini, A. Caneschi, F. Di Benedetto, D. Gatteschi, S. Ristori and M. Romanelli, Phys. Chem. Miner., 2000, 27, 453–461 CrossRef CAS.
- V. S. Rusakov, N. I. Chistyakova, I. A. Burkovsky, A. M. Gapochka, T. L. Evstigneeva and S. Schorr, J. Phys. Conf. Ser., 2010, 217, 012038 CrossRef.
- Y. Cui, R. Deng, G. Wang and D. Pan, J. Mater. Chem., 2012, 22, 23136–23140 RSC.
- B. Murali, M. Madhuri and S. Krupanidhi, Cryst. Growth Des., 2014, 14, 3685–3691 CrossRef CAS.
- A. Ghosh, A. Biswas, R. Thangavel and G. Udayabhanu, RSC Adv., 2016, 6, 96025–96034 RSC.
- F. Ozel, E. Aslan, B. Istanbullu, O. Akay and I. Hatay Patir, Appl. Catal. B Environ., 2016, 198, 67–73 CrossRef CAS.
- S. Rondiya, N. Wadnerkar, Y. Jadhav, S. Jadkar, S. Haram and M. Kabir, Chem. Mater., 2017, 29, 3133–3142 CrossRef CAS.
- C. L. Yang, Y. H. Chen, M. Lin, S. L. Wu, L. Li, W. C. Liu, X. S. Wu and F. M. Zhang, Mater. Lett., 2016, 166, 101–104 CrossRef CAS.
- Y. Cui, G. Wang and D. Pan, J. Mater. Chem., 2012, 22, 12471–12473 RSC.
- M. Rouchdi, E. Salmani, N. Hassanain and A. Mzerd, Opt. Quant. Electron., 2017, 49(4), 1–12 Search PubMed.
- Q. Zhang, H. Deng, L. Chen, J. Tao, J. Yu, P. Yang and J. Chu, Mater. Lett., 2017, 193, 206–209 CrossRef CAS.
- D. B. Khadka and J. Kim, J. Phys. Chem. C, 2015, 119, 1706–1713 CrossRef CAS.
- S. Chatterjee and A. J. Pal, Sol. Energy Mater. Sol. Cells, 2017, 160, 233–240 CrossRef CAS.
- C. Dong, G. Y. Ashebir, J. Qi, J. Chen, Z. Wan, W. Chen and M. Wang, Mater. Lett., 2018, 214, 287–289 CrossRef CAS.
- A. Le Donne, S. Marchionna, M. Acciarri, F. Cernuschi and S. Binetti, Sol. Energy, 2017, 149, 125–131 CrossRef CAS.
- R. R. Prabhakar, S. Zhenghua, Z. Xin, T. Baikie, L. S. Woei, S. Shukla, S. K. Batabyal, O. Gunawan and L. H. Wong, Sol. Energy Mater. Sol. Cells, 2016, 157, 867–873 CrossRef CAS.
- J. Yu, H. Deng, Q. Zhang, J. Tao, L. Sun, P. Yang and J. Chu, Mater. Lett., 2018, 233, 111–114 CrossRef CAS.
- S. Marchionna, A. Le Donne, M. Merlini, S. Binetti, M. Acciarri and F. Cernuschi, J. Alloys Compd., 2017, 693, 95–102 CrossRef CAS.
- A. Crovetto, Z. Xing, M. Fischer, R. Nielsen, C. N. Savory, T. Rindzevicius, N. Stenger, D. O. Scanlon, I. Chorkendorff and P. C. K. Vesborg, ACS Appl. Mater. Interfaces, 2020, 12, 50446–50454 CrossRef CAS PubMed.
- H. Xiao, Z. Chen, K. Sun, C. Yan, J. Xiao, L. Jiang, X. Hao, Y. Lai and F. Liu, Thin Solid Films, 2020, 697, 137828 CrossRef CAS.
- J. Ge and Y. Yan, J. Mater. Chem. C, 2017, 5, 6406–6419 RSC.
- H. Guo, C. Ma, Z. Chen, X. Jia, Q. Cang, N. Yuan and J. Ding, Sol. Energy, 2019, 181, 301–307 CrossRef CAS.
- T. H. Nguyen, W. Septina, S. Fujikawa, F. Jiang, T. Harada and S. Ikeda, RSC Adv., 2015, 5, 77565–77571 RSC.
- X. Zeng, K. F. Tai, T. Zhang, C. W. J. Ho, X. Chen, A. Huan, T. C. Sum and L. H. Wong, Sol. Energy Mater. Sol. Cells, 2014, 124, 55–60 CrossRef CAS.
-
(a) T. Gokmen and O. Gunawan, Hall Measurement System with Rotary Magnet, US Pat., 9041389, International Business Machines Corp., 2015 Search PubMed;
(b) Rotating magnetic Field Hall measurement system, US Pat.App. 14/682696, 14/748495, 2014 Search PubMed.
- O. Gunawan, Y. Virgus and K. F. Tai, Appl. Phys. Lett., 2015, 106, 062407 CrossRef.
- J. J. Scragg, T. Ericson, T. Kubart, M. Edoff and C. Platzer-Björkman, Chem. Mater., 2011, 23, 4625–4633 CrossRef CAS.
- A. C. Lokhande, R. B. V. Chalapathy, M. He, E. Jo, M. Gang, S. A. Pawar, C. D. Lokhande and J. H. Kim, Sol. Energy Mater. Sol. Cells, 2016, 153, 84–107 CrossRef CAS.
- W. Li, J. Chen, C. Yan and X. Hao, J. Alloys Compd., 2015, 632, 178–184 CrossRef CAS.
- X. Zeng, S. S. Pramana, S. K. Batabyal, S. G. Mhaisalkar, X. Chen and K. B. Jinesh, Phys. Chem. Chem. Phys., 2013, 15, 6763–6768 RSC.
- C. Wang, S. Chen, J.-H. Yang, L. Lang, H.-J. Xiang, X.-G. Gong, A. Walsh and S.-H. Wei, Chem. Mater., 2014, 26, 3411–3417 CrossRef CAS.
- L. Kaupmees, M. Altosaar, O. Volobujeva, T. Raadik, M. Grossberg, M. Danilson, E. Mellikov and P. Barvinschi, Adv. Mater. Sci. Eng., 2012, 2012, 11 Search PubMed.
- K.-L. Huang, C.-H. Huang, W.-T. Lin, Y.-S. Fu and T.-F. Guo, J. Alloys Compd., 2015, 646, 1015–1022 CrossRef CAS.
- Y. Zhu, Y. Liu, G. Ren, X. Tan, M. Yu, Y. H. Lin, C. W. Nan, A. Marcelli, T. Hu and W. Xu, Inorg. Chem., 2018, 57, 6051–6056 CrossRef CAS PubMed.
- Q. Zhang, H. Deng, L. Chen, L. Yu, J. Tao, L. Sun, P. Yang and J. Chu, J. Alloys Compd., 2017, 695, 482–488 CrossRef CAS.
- K.-L. Ou, J.-C. Fan, J.-K. Chen, C.-C. Huang, L.-Y. Chen, J.-H. Ho and J.-Y. Chang, J. Mater. Chem., 2012, 22, 14667–14673 RSC.
- M. S. Faber, M. A. Lukowski, Q. Ding, N. S. Kaiser and S. Jin, J. Phys. Chem. C Nanomater. Interfaces, 2014, 118, 21347–21356 CrossRef CAS PubMed.
- X. Fontané, V. Izquierdo-Roca, E. Saucedo, S. Schorr, V. O. Yukhymchuk, M. Y. Valakh, A. Pérez-Rodríguez and J. R. Morante, J. Alloys Compd., 2012, 539, 190–194 CrossRef.
- L. Chen, H. Deng, J. Tao, W. Zhou, L. Sun, F. Yue, P. Yang and J. Chu, J. Alloys Compd., 2015, 640, 23–28 CrossRef CAS.
- C. Rincón, M. Quintero, E. Moreno, C. Power, E. Quintero, J. Henao, M. Macías, G. Delgado, R. Tovar and M. Morocoima, Solid State Commun., 2011, 151, 947–951 CrossRef.
- M. Himmrich and H. Haeuseler, Spectrochim. Acta Part A Mol. Spectrosc., 1991, 47, 933–942 CrossRef.
- J. Zhong, Q. Wang and W. Cai, Mater. Lett., 2015, 150, 69–72 CrossRef CAS.
- S. Schorr, G. Gurieva, M. Guc, M. Dimitrievska, A. Pérez-Rodríguez, V. Izquierdo-Roca, C. S. Schnohr, J. Kim, W. Jo and J. M. Merino, J. Phys. Energy, 2019, 2, 012002 CrossRef.
- J. Ge, P. Koirala, C. R. Grice, P. J. Roland, Y. Yu, X. Tan, R. J. Ellingson, R. W. Collins and Y. Yan, Adv. Energy Mater., 2016, 1601803, DOI:10.1002/aenm.201601803.
- C. Persson, J. Appl. Phys., 2010, 107, 053710 CrossRef.
- A. Ghosh, R. Thangavel and M. Rajagopalan, Energy Environ. Focus, 2014, 3, 142–151 CrossRef.
- S. Palaz, H. Unver, G. Ugur, A. M. Mamedov and E. Ozbay, IOP Conf. Ser. Mater. Sci. Eng., 2017, 175, 012014 CrossRef.
- T. Fukushima, K. Yamauchi and S. Picozzi, Phys. Rev. B, 2010, 82 Search PubMed.
- T.-X. Wang, Y.-G. Li, H.-R. Liu, H. Li and S.-X. Chen, Mater. Lett., 2014, 124, 148–150 CrossRef CAS.
- A. Kamble, K. Mokurala, A. Gupta, S. Mallick and P. Bhargava, Mater. Lett., 2014, 137, 440–443 CrossRef CAS.
- F. Ozel, J. Alloys Compd., 2016, 657, 157–162 CrossRef CAS.
- S. Sarkar, B. Das, P. R. Midya, G. C. Das and K. K. Chattopadhyay, Mater. Lett., 2015, 152, 155–158 CrossRef CAS.
- S. Chen, A. Walsh, X.-G. Gong and S.-H. Wei, Adv. Mater., 2013, 25, 1522–1539 CrossRef CAS PubMed.
- D. Shin, B. Saparov and D. B. Mitzi, Adv. Energy Mater., 2017, 7, 1602366 CrossRef.
- A. Murphy, Sol. Energy Mater. Sol. Cells, 2007, 91, 1326–1337 CrossRef CAS.
- A. Nagoya, R. Asahi and G. Kresse, J. Phys. Condens. Matter, 2011, 23, 404203 CrossRef CAS PubMed.
- H. Katagiri, K. Jimbo, W. S. Maw, K. Oishi, M. Yamazaki, H. Araki and A. Takeuchi, Thin Solid Films, 2009, 517, 2455–2460 CrossRef CAS.
- D. B. Mitzi, O. Gunawan, T. K. Todorov, K. Wang and S. Guha, Sol. Energy Mater. Sol. Cells, 2011, 95, 1421–1436 CrossRef CAS.
- P. Baláž, M. Baláž, M. J. Sayagués, A. Eliyas, N. G. Kostova, M. Kaňuchová, E. Dutková and A. Zorkovská, Crystals, 2017, 7, 367 CrossRef.
- P. A. Fernandes, P. M. P. Salomé and A. F. d. Cunha, J. Phys. D: Appl. Phys., 2010, 43, 215403 CrossRef.
- M. Krishnaiah, R. K. Mishra, S. G. Seo, S. H. Jin and J. T. Park, Ceram. Int., 2019, 45, 12399–12405 CrossRef CAS.
- K. F. Tai, O. Gunawan, M. Kuwahara, S. Chen, S. G. Mhaisalkar, C. H. A. Huan and D. B. Mitzi, Adv. Energy Mater., 2016, 6(3), 1501609 CrossRef.
- J. Dziewior and W. Schmid, Appl. Phys. Lett., 1977, 31, 346–348 CrossRef CAS.
- S. Lie, J. M. Rui Tan, W. Li, S. W. Leow, Y. F. Tay, D. M. Bishop, O. Gunawan and L. H. Wong, J. Mater. Chem. A, 2018, 6, 1540–1550 RSC.
- C. E. Housecroft and A. G. Sharpe, Inorganic Chemistry, Pearson Prentice Hall, 2005 Search PubMed.
- S. Lie, S. W. Leow, D. M. Bishop, M. Guc, V. Izquierdo-Roca, O. Gunawan and L. H. Wong, ACS Appl. Mater. Interfaces, 2019, 11, 25824–25832 CrossRef CAS PubMed.
- G.-X. Liang, Y.-D. Luo, J.-G. Hu, X.-Y. Chen, Y. Zeng, Z.-H. Su, J.-T. Luo and P. Fan, Surf. Coat. Technol., 2019, 358, 762–764 CrossRef CAS.
- Z. Su, G. Liang, P. Fan, J. Luo, Z. Zheng, Z. Xie, W. Wang, S. Chen, J. Hu and Y. Wei, Adv. Mater., 2020, 32, 2000121 CrossRef CAS PubMed.
- S. Lie, W. Li, S. W. Leow, D. M. Bishop, O. Gunawan and L. Helena Wong, Sol. RRL, 2020, 4, 1900521 CrossRef CAS.
- X. Meng, H. Deng, J. He, L. Sun, P. Yang and J. Chu, Mater. Lett., 2015, 151, 61–63 CrossRef CAS.
- A. Ghosh, D. K. Chaudhary, A. Biswas, R. Thangavel and G. Udayabhanu, RSC Adv., 2016, 6, 115204–115212 RSC.
- D. Shin, B. Saparov, T. Zhu, W. P. Huhn, V. Blum and D. B. Mitzi, Chem. Mater., 2016, 28, 4771–4780 CrossRef CAS.
- G. Rey, G. Larramona, S. Bourdais, C. Choné, B. Delatouche, A. Jacob, G. Dennler and S. Siebentritt, Sol. Energy Mater. Sol. Cells, 2018, 179, 142–151 CrossRef CAS.
- M. Kumar, A. Dubey, N. Adhikari, S. Venkatesan and Q. Qiao, Energy Environ. Sci., 2015, 8, 3134–3159 RSC.
- S. Siebentritt, T. P. Weiss, M. Sood, M. H. Wolter, A. Lomuscio and O. Ramirez, J. Phys. Mater., 2021, 4, 042010 CrossRef CAS.
- G. Rey, T. Weiss, J. Sendler, A. Finger, C. Spindler, F. Werner, M. Melchiorre, M. Hala, M. Guennou and S. Siebentritt, Sol. Energy Mater. Sol. Cells, 2016, 151, 131–138 CrossRef CAS.
- S. Levcenko, V. Tezlevan, E. Arushanov, S. Schorr and T. Unold, Phys. Rev. B, 2012, 86, 045206 CrossRef.
- M. A. Green, Prog. Photovoltaics Res. Appl., 2012, 20, 472–476 CrossRef CAS.
- P. Caprioglio, M. Stolterfoht, C. M. Wolff, T. Unold, B. Rech, S. Albrecht and D. Neher, Adv. Energy Mater., 2019, 9, 1901631 CrossRef.
- M. Sood, A. Urbaniak, C. Kameni Boumenou, T. P. Weiss, H. Elanzeery, F. Babbe, F. Werner, M. Melchiorre and S. Siebentritt, Prog. Photovoltaics Res. Appl., 2022, 30(3), 263–275 CrossRef.
- M. Sood, A. Urbaniak, C. K. Boumenou, T. Weiss, H. Elanzeery, F. Babbe, F. Werner, M. Melchiorre and S. Siebentritt, arXiv:2103.15616, 2021.
Footnote |
† Electronic supplementary information (ESI) available: Plan-view SEM images of the CXTS films; grain size distribution for the CXTS thin films; local EDS measurement for CSrTS; XRD pattern of the CXTS films with each respective reference patterns; normalized XRD peaks for CXTS compounds at 27° to 30°; Raman spectra under 785 nm excitation wavelength; Raman scattering spectra of CXTS compounds with the dominant quaternary phase measured under 532 nm; Tauc plot based on UV-Vis spectroscopy; device parameters for non-stoichiometric CXTS; device parameters for stoichiometric CXTS; photoluminescence spectra of CZTS, CMnTS, Mg + CTS, CBaTS and CSrTS. See DOI: 10.1039/d2ta00225f |
|
This journal is © The Royal Society of Chemistry 2022 |