DOI:
10.1039/D1TA08254J
(Review Article)
J. Mater. Chem. A, 2022,
10, 5771-5791
Synchrotron-radiation spectroscopic identification towards diverse local environments of single-atom catalysts
Received
25th September 2021
, Accepted 27th November 2021
First published on 29th November 2021
Abstract
Single-atom catalysts (SACs) that can achieve maximum atom utilization and provide well-defined active sites in energy conversion reactions have attracted much attention in the past decade. In general, the local structures of active sites in SACs largely determine their catalytic activity and selectivity. Therefore, identifying the local environments of active sites in specific SACs is essential to understand their catalytic functions in various reactions. Recent studies have demonstrated that synchrotron-radiation spectroscopic characterization methods possess unparalleled capacity in the identification of local environments of SACs due to their wide energy regulation window and high resolution. In this review, we aim to discuss the practical applications of some ex/in situ synchrotron-radiation spectroscopic techniques in recently reported advanced SACs. The experimental analyses from various techniques, such as X-ray absorption spectroscopy (XAS), photoemission spectroscopy (PES) and Fourier-transform infrared spectroscopy (FTIR), are highlighted. The built structure–activity relationships towards identified active sites are also discussed. Finally, a summary of spectral imputs and related significance is made and the research perspectives are suggested.
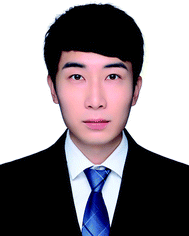 Sicong Qiao | Sicong Qiao received his bachelor's degree from Lanzhou University in 2020. He is currently a PhD student at University of Science and Technology of China. His research interests include the structural regulation of electrocatalysts and synchrotron-radiation characterizations. |
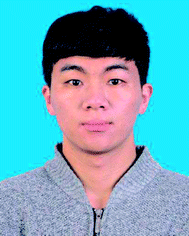 Qun He | Qun He received his PhD degree from University of Science and Technology of China in 2020. He is currently a postdoctoral researcher at University of Science and Technology of China. His current research interests include the controllable synthesis of nanomaterials for energy conversion and catalytic mechanism studies using in situ characterization techniques. |
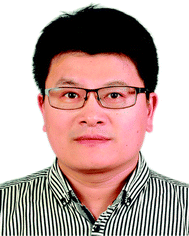 Li Song | Li Song received his PhD in 2006 from Institute of Physics, Chinese Academy of Sciences (advisor Prof. SiShen Xie). He was promoted to professor at University of Science and Technology of China in 2012. He has published about 300 co-authored papers and been cited over 25 000 times in total with H-index 82. His research is focusing on the combination of synchrotron radiation multi-techniques (XAS, SR-XRD, SR-PES and SR-FTIR) via setting up online test platforms, together with the development of data processing method, and applying for the key scientific issues in dynamic reactions and related energy devices. |
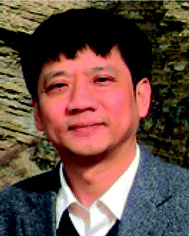 Shiqiang Wei | Shiqiang Wei received his PhD in 1992 from University of Science and Technology of China (advisors Prof. Qiwu Wang and Prof. Chengzong Yang). He was promoted to professor at University of Science and Technology of China in 1999. He was awarded the National Funds for Distinguished Young youths, and the Ministry of science and technology “973” project and National Key Basic Research Program of China. He has been engaged in establishment of X-ray absorption fine structure spectroscopy (XAFS) techniques with surface-sensitive, real-time and in situ measurement, and XAFS applications in energy conversion materials. |
1. Introduction
With the rapid development of world-wide industrialization and frequent human activity, the global consumption of fossil fuels is ever-increasing; inevitably, the shortage of natural resources and environmental problems, such as ocean acidification, greenhouse effect, etc., have happened.1,2 To mitigate these problems, many scientists call to increase the proportions of clean and sustainable solar, hydrogen, wind and other energy sources in modern energy supply systems.3–5 However, these energy sources are intermittent, largely hampering their practical applications. To address this issue, many researchers have proposed to develop efficient energy conversion and storage devices based on electrochemistry, photochemistry or thermochemistry in the past decades.6,7 Generally, the design and operation of these energy devices involve some typical catalytic reactions, i.e., hydrogen evolution reaction (HER), hydrogen oxidation reaction (HOR), oxygen evolution reaction (OER), oxygen reduction reaction (ORR), carbon dioxide reduction reaction (CO2RR), Fischer–Tropsch synthesis (FTS), etc.8–13 These reactions with complicated and sluggish reaction pathways are hard to achieve superior performances to meet the demands of energy devices. For example, the four concerted proton–electron transfer process involved anodic OER just possesses low capacity for oxygen formation, thereby limiting the rate of cathodic hydrogen production in water splitting.14,15 In addition, FTS for industrial coal-to-liquid and gas-to-liquid processes has huge challenges in product selectivity control.13 Therefore, exploiting high-activity catalysts is essential to promote the applications of energy devices.16–18
Traditional heterogeneous solid catalysts feature metal nanoparticles (NPs) and clusters that are composed of distinct amounts of metal atoms, and only a small fraction of sites located at surface, edge, step and corner are exposed to the reactants for catalysis.19,20 Therefore, the atomic efficiency of traditional solid catalysts is very low, causing huge waste of metals, especially some noble metals.21 Meanwhile, traditional catalysts endow the mechanism study with great challenges due to the presence of complex sites in them. Hence, the atomization strategy to decrease the size of metal particles/clusters into single atom to build single-atom catalysts (SACs) should be a promising way to inherit the advantages from both homogeneous and heterogeneous catalysts, such as maximum atomic utilization, high activity and stability.22–24 However, significantly increased surface free energy with downsizing particles leads to the severe segregation of metal atoms in fabrication and employment processes, which is the culprit of low loading and instability of SACs.25,26 Regarding this issue, some researchers found that appropriate supports with diverse anchoring and adsorption sites can prevent the sintering of single atoms (SAs) efficiently (Fig. 1).27 It is also found that supports can work as the modifiers to influence the electronic configurations of foreign atoms through the strong metal-support interaction (SMSI).28 Among different support materials, defect-rich nanocarbons, such as carbon nanotubes, graphene and carbon nitride, have been widely discussed.29–31 Intrinsic edges and holes, doped heteroatoms and surface function groups in/on nanocarbons strengthen the binding of metal atoms via chemical coordination.32–36 Metal compounds including oxides, nitrides, sulfides, phosphides, hydroxides, etc., are also promising supports for SACs.37 The existing cation/anion vacancies and large lattice voids in them can stabilize foreign atoms effectively, rendering obtained catalysts with excellent performances in various reactions.38,39 In addition, atomic replacement between host and foreign atoms can also achieve the incorporation of single atoms.40 Analogously, introducing isolated atoms into traditional metallic catalysts is also feasible, and these catalysts are generally known as single atom alloys (SAAs).41,42 SACs can also be derived from some porous materials including metal–organic frameworks (MOFs), covalent organic frameworks (COFs) and zeolitic imidazole frameworks (ZIFs).43–45 The ordered atomic arrangements, large atomic distance, and rich coordination atoms in them ensure the monodispersion of metal atoms after thermal pyrolysis.46–48
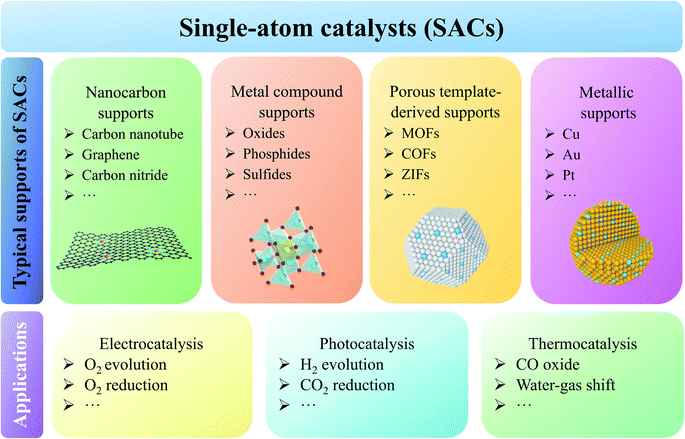 |
| Fig. 1 Comprehensive description of typical supports and applications of SACs. | |
Based on the above analyses, it is easily found that the flexible collocation between active metal atoms and supports results in the formation of SACs with diverse local environments. Different environments endow SACs with distinct properties, thus meeting a series of reactions.49 It is proved that the local cooperation between atomic Sn sites and adjacent oxygen vacancies in CuO contributes to higher CO2-to-methanol selectivity and activity owing to more stable configuration of adsorbed COOH.50 Moreover, some works demonstrated that the adjustment of ligand elementary species and coordination number can not only regulate the properties of metal sites, but also change the active centers. Tang et al. clarified that elements (N or O) in the first and second coordination spheres have a deep impact on the performance of Co SACs in acidic ORR.51 Changing Co–N tetra-coordination of prevalent CoN4 moieties to both Co–O and Co–N di-coordination for CoNOC moieties, the acidic ORR selectivity is tailored from 4e− to 2e− pathway while catalytic sites from isolated Co to C adjacent to the coordinated O. Li et al. reported a mixed catalyst containing both tetra-coordinate Zn–N4 and unsaturated Zn–N3 sites.52 And these unsaturated atoms result in the lower oxidation state of Zn and more stable COOH* adsorption, contributing to the robust CO2RR activity. On the other hand, high-loading SACs attract much attention and need to be explored to meet the demands of practical applications.53–55 Benefiting from the strong chelation and self-assemble effect of amine-functionalized graphene quantum dots (GQDs-NH2), the concentrations of isolated Ir and Ni sites can reach 41.6 wt% and 15.3 wt%, respectively.56 Meanwhile, unveiling the unique coordinate configurations influenced by the increasing metal coverage is fundamental for tracking the activity origin and optimizing design strategies of SACs. For instance, Xiong et al. demonstrated achieving a remarkable Fe loading ratio value of 30 wt% in single-atomic-site (SAS) catalysts would decrease the coordinate number of Fe with appropriate ∼2, which takes charge of the effective epoxidation of styrene.57 These results elucidate that unveiling the atomic-level structures has great significance in cognizing the catalytic behaviors of SACs.
To unveil the local structures of SACs, traditional light source-based spectral characterization methods, such as X-ray photoelectron spectroscopy (XPS), ultraviolet photoelectron spectroscopy (UPS), Raman, Fourier-transform infrared spectroscopy (FTIR), etc., are adopted in most works.58 Although many valuable results have been obtained based on these methods, it is still desired to use higher resolution methods to uncover the unknown or uncertain.59 In addition, traditional characterization methods in analyzing SACs face huge challenges. One challenge is that SACs generally have much less atomic concentrations than their nanoparticles and clusters. Another challenge is the smallest size disables some traditional structure characterization methods including X-ray diffraction (XRD), angle-resolved photoemission spectroscopy (ARPES), etc. As known, high-quality light source is one of the most important parts to determine the spectral signal-to-noise ratio (SNR) and resolution. In this regard, synchrotron-radiation light with high collimation, high resolution and continuously adjustable energy, performs huge advantages relative to traditional light sources in multiply conditions.60 The coupled X-ray absorption spectroscopy (XAS), including X-ray adsorption near-edge structure (XANES) and extended X-ray absorption fine structure (EXAFS), can be obtained in one test to analyze both electronic and coordinate structures of SACs. This advantage has been proved by many reported SACs-related works.61,62 Moreover, it is worth noting that XANES and EXAFS measurements can be applied under working conditions to provide reality-close information for the in-depth cognition of activity origins and catalytic mechanisms of SACs. Besides XANES and EXAFS, soft XAS is also efficient in the detection of chemical states, orbital hybridization and atom-specific local environments of SACs.63–65
It is also demonstrated that establishing traditional characterization methods based on synchrotron-radiation light can improve their data quality and reliability to a large extent. For instance, synchrotron radiation XPS, one of the most important surface-sensitive characterization methods, can obtain the surface information of catalysts with high energy resolution.66 Moreover, synchrotron-radiation near-ambient pressure X-ray photoelectron spectroscopy (NAP-XPS) or ambient pressure XPS (AP-XPS) is available for monitoring superficial valence states excluding the influence of ultrahigh vacuum (UHV).67 Analogous to XPS, UPS is another surface-sensitive method which can probe the electronic levels and valence bands of materials near Fermi level (EF).68,69 The work functions detected by UPS represent the energetic barrier for donating electrons from the EF of catalysts to the vacuum level or adsorbed molecules.70 Generally, synchrotron-radiation XPS and UPS are collectively denoted as synchrotron radiation photoemission spectroscopy (SRPES) because of their identical principles with photoelectric effect.71,72 In addition, in situ/operando synchrotron-radiation Fourier-transform infrared spectroscopy (SR-FTIR) is performed to certify the phase transition and identify the active intermediates.73 Experimental reports have demonstrated that SR-FTIR can show exponential signal intensification with respect to traditional FTIR.74 In conclusion, it is certain that synchrotron-radiation spectral methods are powerful in the identification of local environments of SACs. Hence in this review, we aim to summarize the advancements of multifarious synchrotron-radiation methods in parsing the local environments of SACs. These methods include XAS, SRPES and SR-FTIR (Fig. 2). The usage of in situ/operando synchrotron-radiation methods is expected to track the dynamic evolution of catalytically active sites at the beginning, proceeding and ending of reactions, understand the structure–property relationships, and provide the guideline for SACs designs. Finally, the capabilities and limitations of these methods are discussed for the demands of further development.
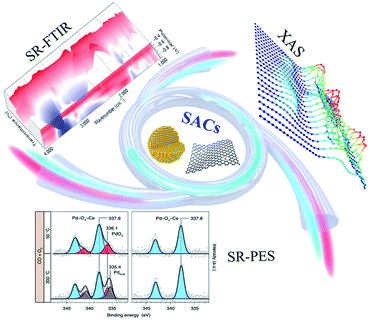 |
| Fig. 2 Schematic illustration of characterization results obtained by typical synchrotron-radiation techniques for SACs. Reprinted with permission from ref. 73. Copyright 2021, Springer Nature. Reprinted with permission from ref. 138. Copyright 2021, Springer Nature. | |
2. Synchrotron-radiation spectroscopies towards SACs
2.1 XAS
XAS is a synchrotron-radiation element-specific technique based on motivating electrons from the core to unoccupied energy levels by X-ray, thus offering help for unveiling electronic and coordinate structures of catalysts.75 When the X-ray passes through materials, the attenuated intensity is quantified by the absorption coefficient according to Beer's law.76 The relation between absorption coefficient and incident X-ray energy is influenced by elementary chemical states and local environments, which can be divided into pre-edge, near-edge and extended-edge regions.77 Pre-edge and near-edge regions are sensitive to oxidation states and electronic levels of detected elements, hence together referred to XANES.78,79 With further increasing X-ray energy, electrons will be excited to continuum states in vacuum.80 Ejected and back-scattered photoelectrons surrounding the absorbing atom lead to oscillation reflecting the coordination number and bond length in extended-edge region, which is denoted as EXAFS.81,82 Wavelet transform EXAFS (WT-EXAFS) spectra, which has high resolution for both k and R spaces, provide more intuitive comparison in bond length to identify distinct coordinated configurations.83,84 And through the least-squares fitting of Fourier transform EXAFS (FT-EXAFS), accurate values about coordinate number and bond length can be obtained.85,86 Besides, soft XAS has unique advantages in investigating orbital hybridization between metal and light ligand elements by employing soft X-ray as the excited light source.87
Different from techniques focusing on superficial chemical environments like XPS, XAS implies total statistical results of catalyst bulks owing to superior penetration depth of X-ray, which makes visualizing operando and in situ variation of serving SACs possible (Fig. 3a and b).88,89 Tracking the evolution of local environments including optimization of chemical states and bonding forms has attracted broad attention by in situ XAS under applied outfields. In this part, we are going to present more detailed discussions on identifying SACs with diverse local environments by XAS.
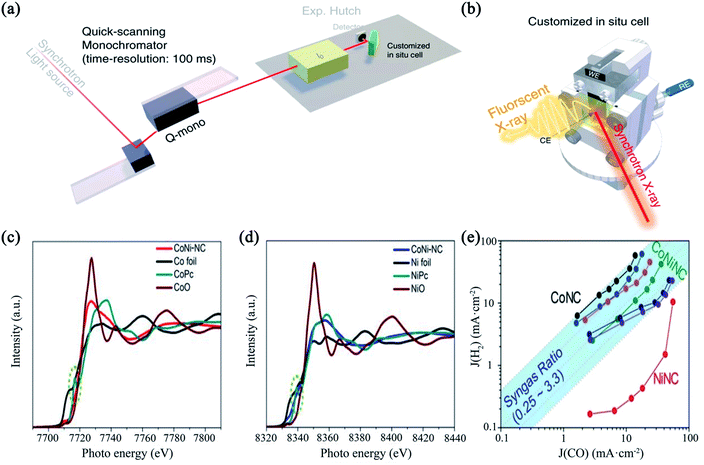 |
| Fig. 3 (a) The schematic diagram of a kind of setup for operando time-resolved XAS experiments; (b) the cartoon representation of customized operando XAS cell. Reprinted with permission from ref. 88. Copyright 2020, Springer Nature. (c, d) Co and Ni K-edge spectra of CoNi-NC and references; (e) the corresponding current densities of CO and H2. Reprinted with permission from ref. 91. Copyright 2020, WILEY-VCH Verlag GmbH & Co. KGaA, Weinheim. | |
2.1.1 XANES.
XANES can be used to evaluate elementary electronic structures directly and charge transfer indirectly, both of which are deeply connected with local environments of SAs.90 Typically, the electronic transition peaks of metals in pre-edge region can provide fingerprint identification of coordinate information. For instance, He et al. synthesized a dual-site SAC (denoted as CoNi-NC) for electrochemical conversion of CO2 to syngas.91 Obtained from Co and Ni K-edge XANES profiles, the valence states of Co and Ni are between 0 and +2, excluding the existence of metallic Co and Ni (Fig. 3c and d). It is worth noting that weak signals in pre-edge region are corresponding to the 1s → 4pz transition of Co and Ni, which are fingerprint descriptors for square-planar M-N4 moieties. Meanwhile, the reduced intensities compared to Co- and Ni-phthalocyanine suggest the distorted D4h symmetry in CoNi-NC. Then coupling XPS and EXAFS spectra, the single-atom configuration with dominant tetra-pyridinic-N coordination is confirmed. The Co–N4 and Ni–N4 act as HER and CO2RR sites respectively, which opens up the possibility of efficient production of syngas (Fig. 3e). Moreover, the electronic interaction regulated by metal compound supports through metal-nonmetal-metal bonds can be investigated by XANES. Shan et al. paid attention to short-range ordered Ir SAs integrated into cobalt oxide spinel (marked as Ir0.06Co2.94O4) synthesized by ion exchange–pyrolysis procedure (Fig. 4a).92 XANES spectra show that Ir in Ir0.06Co2.94O4 has a high valence state of about 4+, which acts as superior acidic OER sites (Fig. 4b and c). An increasing oxidation state of Co induced by electronic transfer after Ir substituting is proved through Co K-edge XANES (Fig. 4d).
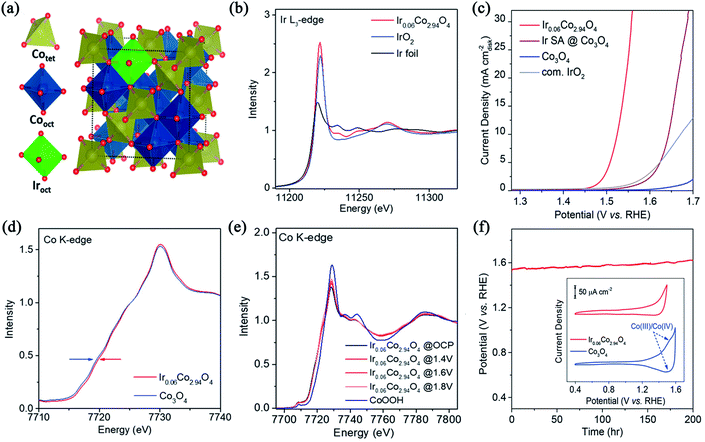 |
| Fig. 4 (a) Structural model of Ir-substituted cobalt spinel oxide in a polyhedral representation; (b) Ir L3-edge spectra of Ir0.06Co2.94O4, IrO2 and Ir foil; (c) polarization curves in O2-saturated 0.1 M HClO4; (d) Co K-edge spectrums of Ir0.06Co2.94O4 and Co3O4 support; (e) in situ Co K-edge spectra under several potentials; (f) chronopotentiometric curve of Ir0.06Co2.94O4 at 10 mA cm−2, the insert is cyclic voltammetry (CV) tests. Reprinted with permission from ref. 92. Copyright 2021, American Chemical Society. | |
In situ and operando XANES methods play a vital role in connecting local electronic environments of the target elements with reactivity and stability of SACs to conduct mechanism analysis.93 In the above-mentioned Shan's work, in situ Co K-edge XANES spectra were recorded to observe Co oxidation states under OER condition.92 Similar shape and intensities of white line suggest no obvious phase transformation under different applied potentials (Fig. 4e), in accordance with the results of in situ Raman spectra. These conclusions confirm Ir species can restrain the oxidation from Co3O4 to high-valence and easy-leaching CoOx(OH)y, which renders slighter dissolution of surface Co species than Co3O4 support and the long-term stability for over 200 h (Fig. 4f).94 Otherwise, the reactive evolution of local environments, such as reversible clustering of SAs, has a strong impact on catalytic performances, which can be specialized by the linear combination fitting (LCF) of in situ and operando XANES spectra. Maurer et al. fabricated Pt single-site catalyst (Pt-SS) through the redispersion of a traditional Pt/CeO2 catalyst in the hydrothermal oxidizing treatment.95 Using original Pt-SS and Pt nanoparticles (Pt-NP) as references for transiently recorded operando XANES spectra, obvious reduction in Pt-SS is observed when CO conversion set in, while absence in Pt-NP (Fig. 5a). Complementary to in situ infrared (IR) spectroscopy and DFT calculations, operando and in situ high-energy-resolution fluorescence detected XANES (HERFD-XANES) spectra were performed to track the formation, fate and consequence of Pt-SS. A dramatic decrease in intensity up to 150 °C arises followed by a shift of the white line towards lower adsorption energy (Fig. 5b), which certifies the valence of Pt changes accompanied by CO adsorption. To interpret the intermediate structural states, LCF was performed for operando spectra using the references obtained from the multivariate curve resolution alternating least squares algorithm (MCR-ALS) of in situ spectra.96,97 Four reference compounds containing Pt4+, Pt2+, more reduced Ptδ+–CO and Pt cluster-like PtXδ+–CO are proposed and quantificationally assigned to HERFD-XANES experimental spectra verified by ab initio calculations using FDMNES code (Fig. 5d–f).98,99 Ultimately, the relationship between fractions of references and applied temperatures indicates that formed tiny PtXδ+ entities from the breaking of Pt–O–Ce bonds work as real active sites for CO conversion (Fig. 5c).
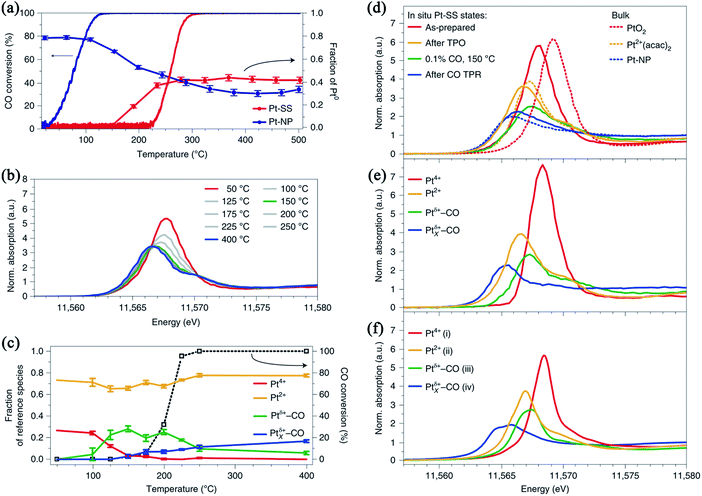 |
| Fig. 5 (a) CO conversion and LCF results of transiently recorded spectra during heating with 5 K min−1 in 1000 ppm CO and 10% O2 in He; (b) operando HERFD-XANES spectra of Pt-SS recorded at different temperatures in the gas mixture including 1000 ppm CO, 10% O2 and He; (c) catalytic activity during the HERFD-XANES experiments and fraction of Pt species obtained by LCF using references proposed by MCR-ALS; (d) in situ experimental spectra under the treatment of temperature-programmed reduction (TPR) and temperature-programmed oxidation (TPO); (e) spectra of MCR-ALS-derived references; (f) FDMNES-calculated spectra. Reprinted with permission from ref. 95. Copyright 2020, Springer Nature. | |
Thus, positive oxide states of metal atoms confirmed by XANES are indirect proofs to exclude the presence of metallic phase.100 The contrast of elementary electronic structures before and after introducing SAs is proceeded to specialize electron transfer and SMSI by ex situ XANES spectra.101,102 On the other hand, visualizing the dynamic changes of oxidation states is crucial to investigating the evolution of single-atom environments and impact of relevant adsorbed molecules under serving conditions, which can be achieved by in situ and operando XANES spectra cooperating with IR, Raman, theoretical calculation, etc.103 In general, synchrotron-radiation XANES takes momentous effect in unveiling redox-involved reaction mechanisms.
2.1.2 EXAFS.
EXAFS studies contribute to analyzing local coordinate configurations including bond length and ligancy of elements, thus giving detailed descriptions of structural models for SACs. It is reported that the catalyst with Ni SAs immobilized on N-doped hollow carbon spheres (Ni SACs/N–C) acts as an electrochemical sensor providing the real-time monitoring for cellular NO with a nanomolar detection limit (Fig. 6a).104 Least-squares fitting of FT-EXAFS spectrum exhibits only one dominant Ni–N peak with tetra coordination (Fig. 6b), suggesting a highly dispersed Ni–N4 architecture. Theoretically calculated spectrum employing the model of Ni–N4 immobilized on graphene is most consistent with the experimental one (Fig. 6c), which further manifests the geometrical aspects of Ni SACs/N–C. Moreover, it is well known that the performances of SACs can be rationally optimized by tailoring the metal atoms,105,106 adjacent coordinative dopants,107,108 and metal loading.109,110 Thus the impacts of unique coordinate structures and increasing metal loading on structure–property relation have been discussed extensively. Tang et al. prepared a new kind of Mo SAC (Mo1/OSG-H) with the loading ratio reaching 13.47 wt% by MgO-templated pyrolysis combined with glucose-chelating and defect-trapping effects.111 Owing to the heterogeneity induced by such a high Mo loading amount, EXAFS fitting with both Mo–O3S–C and mixture (Mo–O4–C and MoS2) models disables to give reasonable results. However, comparing to Na2MoO4 and MoS2 references, the features of Mo–O and Mo–S bonds can be identified unambiguously from FT-EXAFS and WT-EXAFS, which suggests a mixed coordination of Mo–O and Mo–S moieties in isolated Mo atoms anchored on oxygen, sulfur co-doped graphene (Fig. 6d and e). Benefiting from this novel structure and impressive metal concentration, Mo1/OSG-H exhibits the remarkable activity and selectivity over 95% towards 2e− ORR pathway (Fig. 6f). Besides classical coordination with heteroatoms like N, O, S, etc., metal–metal bonds can also take effect in trapping and immobilizing isolated atoms. For instance, Liu et al. achieved the transformation from RuO2 aggregates to Ru SAs (Ru1/MAFO) arising from anti-Ostwald ripening promoted by a strong covalent metal–support interaction (CMSI).112 EXAFS spectrum of Ru1/MAFO-900 reveals Ru–O and Ru–Fe scattering contributions (Fig. 7a), and the absence of Ru–Ru and Ru–O–Ru unambiguously evidences high dispersion of Ru atoms. Then combining multiply characterization methods, it is proved that the presence of Ru–Fe indicates CMSI between Ru SAs and FeOx can trap and stabilize Ru atoms after high-temperature calcination at 900 °C, which also contributes to the excellent performances for N2O decomposition (Fig. 7b and c).
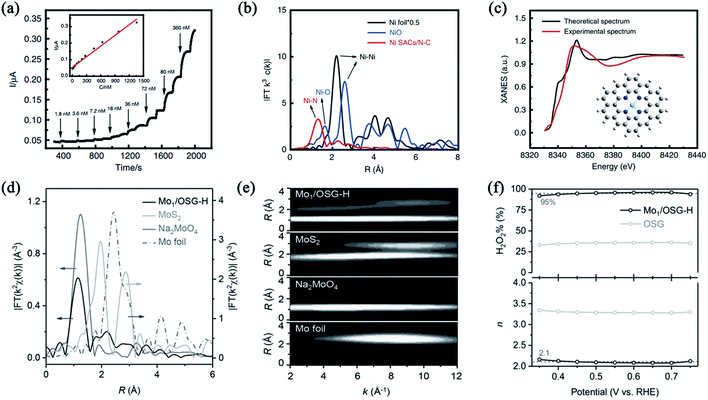 |
| Fig. 6 (a) Amperometric response of the Ni SACs/N–C-based stretchable sensor to successive addition of NO at +0.80 V, the inset is calibration curve; (b) Ni K-edge FT-EXAFS spectra of Ni SACs/N–C, NiO and Ni foil; (c) experimental and calculated spectrums, inset is proposed Ni–N4 architecture. Reprinted with permission from ref. 104. Copyright 2020, Springer Nature. (d, e) FT- and WT-EXAFS curves of the Mo K edge; (f) calculated electron transfer number (n) and H2O2 selectivity (H2O2%) of Mo1/OSG-H. Reprinted with permission from ref. 111. Copyright 2020, WILEY-VCH Verlag GmbH & Co. KGaA, Weinheim. | |
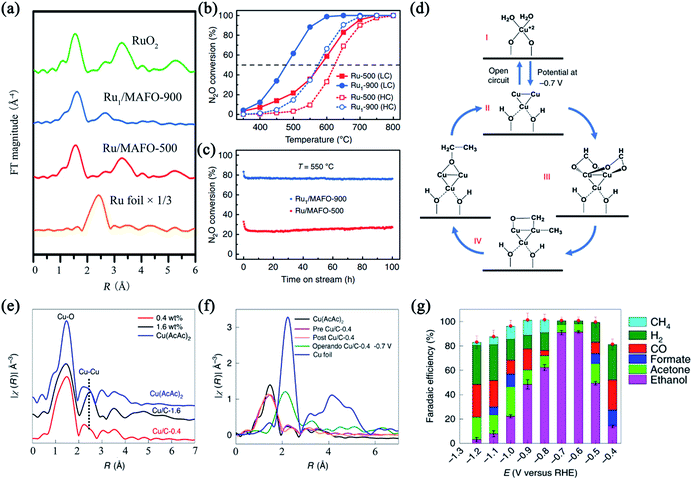 |
| Fig. 7 (a) Ru K-edge spectra of Ru1/MAFO, RuO2 and Ru foil; (b) N2O conversion at low (1000 ppm N2O, solid symbol) and high (20 vol% N2O, open symbol) concentrations; (c) N2O conversion stability measurement in low concentration tested at 550 °C. Reprinted with permission from ref. 112. Copyright 2020, Springer Nature. (d) The hypothesized CO2-to-ethanol reaction mechanism; (e) Cu K-edge spectra of Cu/C-0.4, Cu/C-1.6 and Cu foil; (f) ex situ and operando spectra, pre and post Cu/C-0.4 refer to the catalyst measured before and after CO2RR respectively; (g) products' species and faradaic efficiency (FE) while employing Cu/C-0.4. Reprinted with permission from ref. 113. Copyright 2020, Springer Nature. | |
In situ and operando EXAFS spectra also pave a way for observing the dynamic reconstruction of local structures and formation of real catalytic active sites of SACs, which helpfully operates mechanism analysis. Xu et al. synthesized a carbon-supported atomically dispersed Cu catalyst by amalgamated Cu–Li and subsequent leaching away Li.113Ex situ EXAFS spectra demonstrate single Cu is coordinated with four oxygen atoms and no Cu–Cu bond appears while the loading ratio is inferior to 0.4 wt% (Fig. 7e), agreeing well with the LCF of XANES. It's also found that Cu keeps atomically dispersed after a 16 h chronoamperometric run at −0.7 V for Cu/C-0.4. Then operando EXAFS spectra of Cu/C-0.4 were collected. Once the cell voltage was applied at −0.7 V, Cu first shell coordination switched from Cu–O to mainly Cu–Cu, indicating the formation of clustering Cu entities (marked as Cun, where n = 3 or 4) that act as catalytically active sites for CO2RR (Fig. 7f and g). Meanwhile, the re-oxidation of Cun clusters to Cu2+ SAs occurs in the CO2-saturated electrolyte as soon as the voltage shuts down according to ex situ EXAFS. Incorporating DFT calculations, the CO2-to-ethanol paths including a reversible transformation between Cu clusters and SAs was proposed in Fig. 7c. Moreover, some comparatively slighter changes in local environments are also associated with catalytic activities, such as shrinking or stretching of chemical bonds. Shang et al. designed a single-atom Inδ+–N4 catalyst (In-SAs/NC) through wet-impregnation and pyrolytic processes, the structure of which is confirmed by ex situ quantitative EXAFS (Fig. 8a).114 WT-EXAFS spectrum shows strong signal for In–N instead of In–In (Fig. 8d). Then potential-dependent in situ XAFS was conducted to observe the change of In–N bonds (Fig. 8b). It is analyzed that In–N bond length becomes shorter at −0.65 V than without potential, taking charge for high catalytic activity and selectivity of CO2RR (Fig. 8c).
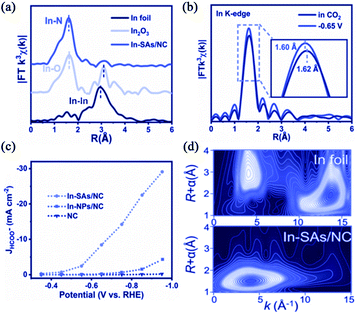 |
| Fig. 8 (a) In K-edge spectra of In-SAs/NC, In2O3 and In foil; (b) in situ spectra of In-SAs/NC; (c) the current density of HCOO− in CO2RR; (d) WT-EXAFS spectrums. Reprinted with permission from ref. 114. Copyright 2020, WILEY-VCH GmbH. | |
Therefore, EXAFS analysis is a mighty method to estimate dispersion degrees and distinguish the local environments of object elements by quantitative fit, especially for SACs.115 However, ex situ EXAFS isn't sensitive to some reversible or surrounding-involved processes. In this case, in situ and operando EXAFS spectra are essential for conducting studies of catalytic mechanisms because of convenience to tracking the changes of bond length and coordinate features of metal SAs, which is closely connected with the self-optimization of catalysts. Combining in situ or operando XANES and EXAFS, a detailed reaction mechanism can be postulated.116
2.1.3 Soft XAS.
The feature of relatively lower energy makes soft XAS sensitive to investigating the bond types and orbital hybridization of light elements.117 Especially for some classical SACs with nanocarbon supports, ligand elements like C, N, O, S play a vital role in catalytic reactivity.118 Zhang et al. obtained a HP-FeN4 catalyst with high-purity pyrrole-type FeN4 sites synthesized by pyrolysis under NH3 atmosphere.119 Traditional FeN4 sites were also fabricated analogically except under Ar atmosphere. Two typical features in C K-edge soft XAS of HP-FeN4 and FeN4 are assigned to the 1s core electrons of C into the πC
C* and σC–C* respectively, which is similar to highly graphitized carbon (Fig. 9a left). Spontaneously, N K-edge soft XAS can also be divided into two regions containing 1s electrons into the π* and σ* (Fig. 9a right). The transition from 1s to π* presents obvious resonances ascribed to C–N–C portion of pyridinic N (peak a) and pyrrolic/graphitic type N-groups (peak b). To distinguish the coordinated N entities, peak a and b are fitted and the results confirm the existence of high-purity Fe–pyrrolic N sites in HP-FeN4, which is entirely different from Fe–pyridinic N sites in FeN4 (Fig. 9b and c). It means that neighboring N configuration around Fe changes from pyridinic to pyrrolic N with the assistance of NH3, inducing preferable O2 adsorption energy and superior activity for four-electron ORR (Fig. 9d). Besides aiming at ligand elements, soft XAS also contributes to analyzing species and distribution of transition elements through their L and M edges. It is reported that the Mn–O3N1 configuration anchored on porous carbon (Mn–O3N1/PC) was designed through a dissolution-and-carbonization method.120 Mo L3 soft XAS of Mn–O3N1/PC is similar to MnO reference in both total electron yield (TEY) and partial fluorescence yield (PFY) modes (Fig. 9e). This suggests the oxidation state of +2 and uniform dispersion of Mn, because TEY detects signals within 5–10 nm thickness of the surface while PFY within up to 100 nm thickness of the bulk.
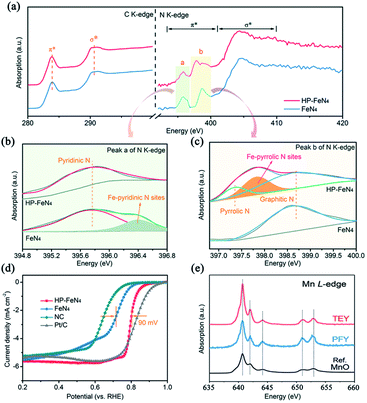 |
| Fig. 9 (a) C K-edge and N K-edge spectra of HP-FeN4 and FeN4; (b, c) deconvolution features of peak a (b) and b (c); (d) ORR polarization curves. Reproduced from ref. 119 with permission from the Royal Society of Chemistry. (e) Mn L-edge TEY and PFY spectra of Mn–O3N1/PC and MnO. Reprinted with permission from ref. 120. Copyright 2021, American Chemical Society. | |
It's worth noting that there are some researches concentrating on the influence of valence and spin states on catalytic performance by in situ and operando soft XAS spectra. For example, Zhou et al. tracked the voltage- and time-proportional valence state transition of Co accompanied by spontaneous delithiation in Li2Co2O4 under OER conditions by operando O K-edge soft XAS and ICP-OES.121 Zheng et al. confirmed the formation of high-valence Ni4+ under low overpotential conditions during pH-neutral OER using in situ Ni L-edge soft XAS spectra.122 However, the studies about in situ and operando soft XAS investigating on SACs are still lacking to the best of our knowledge, especially for light ligand elements. One possible reason is that soft XAS can only reflect the collective effect of C, N, O, etc., while only a small portion of them are coordinated with center atoms, which makes the vibration too weak to detect.123
In summary, XAS spectra have powerful ability in discussing local characters including oxidation states, bonding forms and orbital hybridization. For SACs, the cationic feature and absence of metal–metal bonds deduced by XANES and EXAFS respectively are essential to confirm atomic dispersion. The puzzle about the metal–ligand interaction can be answered partially by soft XAS. Meanwhile, tracking outfield- and time-dependent vibration of SACs becomes possible owing to the development of in situ and operando XAS spectra. The identification towards local environments of catalysts is not restricted to the initial and final states any more, thereby contributing to understanding the evolution of active sites and conducting mechanism studies. However, some specific challenges still need to be overcome to get much more novel phenomena. For example, the sensitivity and detection limit should be optimized for the sake of receiving subtle responses, such as the change of ligand elements and low-loading model SACs. The differential spectral method is another practical way to visualize these variations.124,125
2.2 SRPES
PES is a conventional, convenient and widely used method to evaluate the valence and species of target elements. Different from XAS detecting transmission light or fluorescence, PES receives signals from outgoing electrons that have shorter the mean free path due to inelastic collision.126 Thus PES is sensitive to surface-involved systems, such as SAs immobilized on the surface of supports. Although most traditional XPS and UPS measurements are finished on lab-based devices, synchrotron-radiation light source can obtain more high-quality data at the same beamline due to the adjustable incident energy, high brightness, etc.127
Recently, quasi in situ synchrotron-radiation high-resolution XPS spectra referring to treating samples with distinct atmosphere and temperature in a reaction cell firstly, then transferring to the analysis chamber under UHV without exposure to air, is proposed for catalytic studies.128–130 Although some valuable results have been obtained through quasi in situ XPS, it is worth noticing that this cannot avoid the influence of UHV on materials while testing. On the other hand, SACs-participating heterogeneous catalysis usually occurs on the solid–gas or solid–liquid interfaces. Authentic surface chemistry is a key descriptor for understanding catalytic performance.131 Hence, synchrotron-radiation NAP-XPS attracts much attention in investigating gas-involved processes and achieving in situ and operando tests, which are indispensable to understand paths of specially requested reactions (Fig. 10a).132,133 Herein, synchrotron-radiation NAP-XPS and UPS were summarized to highlight the value for applying to SACs. Other issues solved by SRPES such as describing longitudinal distribution of elements are also noteworthy.134
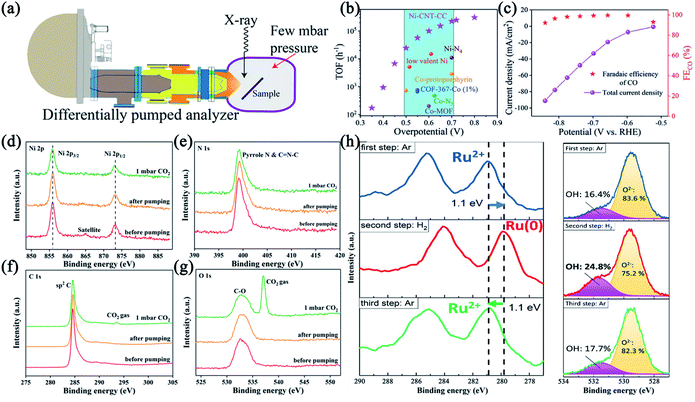 |
| Fig. 10 (a) A schematic representation for NAP-XPS setup. Reprinted with permission from ref. 133. Copyright 2018, WILEY-VCH Verlag GmbH & Co. KGaA, Weinheim. (b) The TOF of Ni-CNT-CC compared with those of other state-of-the-art CO2-to-CO reduction catalysts; (c) CO FE (symbol) and total current density (line + symbol); (d–g) high-resolution Ni 2p (d), N 1s (e), C 1s (f) and O 1s (g) spectra of Ni-CNT-CC before and after exposing to 1 mbar CO2. Reprinted with permission from ref. 135. Copyright 2020, WILEY-VCH Verlag GmbH & Co. KGaA, Weinheim. (h) Operando Ru 3d (left) and O 1s (right) spectra of Ru/MgO (1 1 1) at 350 °C under switching between 1 mbar Ar and 1 mbar H2. Reprinted with permission from ref. 137. Copyright 2021, American Chemical Society. | |
2.2.1 NAP-XPS.
As mentioned above, NAP-XPS builds the relation between local environments of SACs and interfaces surrounded by reactants. Liu et al. linked Ni2+ compound to carbon nanotubes (Ni-CNT-CC) as a well-defined single-atom Ni–N4 model catalyst for efficient CO2RR (Fig. 10b and c).135 Almost the same intensity and shape in Ni 2p, O 1s, N 1s and C 1s NAP-XPS before and after exposing to 1 mbar CO2 elucidates that CO2 did not chemically interact with Ni2+ sites of Ni-CNT-CC (Fig. 10d–g). Combining operando Ni K-edge XANES, operando Raman spectra and electrochemical kinetics studies, reversible formed Ni+ is certified as catalytically active centers for CO2 activation.
Moreover, NAP-XPS is naturally appropriate for in situ and operando thermocatalytic conditions owing to the convertible atmosphere and temperature, so that tracking the dynamic evolution of local environments of active center atoms induced by gas reactants.136 And unveiling these changes of coordinate types usually contributes to identifying real catalytic active species. For example, Wu et al. put forward a frustrated Lewis pair (FLP) mechanism of Ru anchored on stable MgO (111) facet for reversible hydrogen spillover based on operando AP-XPS combining in situ diffuse reflectance infrared Fourier transform spectroscopy (DRIFTS) and in situ XANES spectra.137 According to Ru 3d and O 1s XPS, after removing RuO2 under H2, Ru valence is +2 under Ar (Fig. 10h). Then switching to H2 atmosphere, Ru is reduced to Ru(0) accompanied by a significant increase of OH in O 1s spectrum. The increase can be totally counteracted once the flowing gas is switched to Ar again, suggesting a reversible H2 storage-release process. Under the circumstances, it is envisaged that half the reversible process is that Ru2+ acting as a Lewis acid leads to the cleavage of H2 and formation of Ru–H hydride that is converted to a proton progressively along with the reduction of Ru, while O2− near Ru sites as a Lewis base receives migrant proton to form OH−. Muravev et al. compared the interface dynamics of two single-atom Pd/CeO2 catalysts fabricated by conventional impregnation marked as 1PdRods and one-step flame spray pyrolysis (FSP) as 1PdFSP respectively for low-temperature CO oxidation.138In situ NAP-XPS demonstrates the appearance of small PdOx clusters at 336.1 eV and even metallic Pd at 335.4 eV in 1PdRods while absence in 1PdFSP under 2 mbar pressure conditions including CO and O2, showing the structural stability owing to stronger metal-support interactions (MSIs) by FSP (Fig. 11a and b). As a contrast, 5PdFSP and 5PdRods with higher Pd surface density are obtained to exclude the effect of Pd loading. Lower emerging temperature and content of metallic Pd in 5PdFSP are accord with mentioned conclusions, corroborated by in situ DRIFTS simultaneously. To understand MSIs and reducibility of the Pd–CeO2 interfaces, in situ synchrotron-radiation resonant photoelectron spectroscopy (RPES), with low excitation energies allowing visualizing elementary electronic states in the outermost layers at a depth less than ∼10 Å, was performed to track the variation of Ce3+ concentration by analyzing the Ce 4d–4f transition.139,140 As shown in Fig. 11c and d, slightly higher Ce3+ ratio in 1PdFSP than 1PdRods and the bare FSP support suggests higher concentration of oxygen vacancies and surface reducibility induced by Pd doping. The high oxygen mobility at the Pd–CeO2 interface supplies sufficient oxygen to stable cationic Pd.
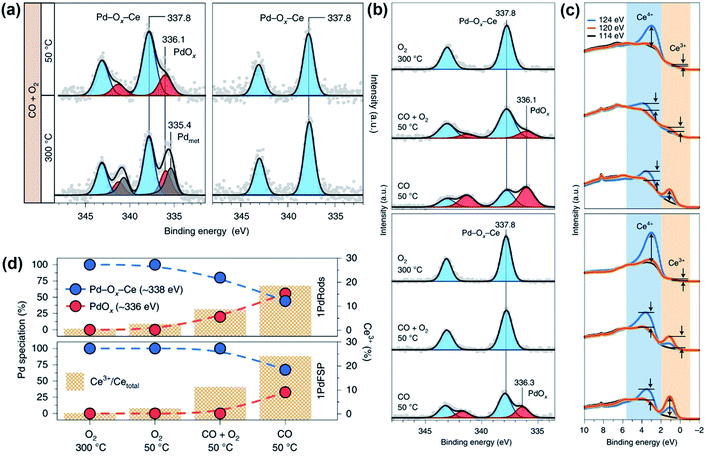 |
| Fig. 11 (a) In situ Pd 3d spectra of 1PdRods (left) and 1PdFSP (right) in 2 mbar mixed gas with CO : O2 = 1 : 1 at applied temperature; (b, c) in situ XPS (b) and RPES (c) of 1PdRods (top) and 1PdFSP (bottom) in 0.1 mbar reactants; (d) Pd and Ce oxidation state derived from XPS and RPES data. Reprinted with permission from ref. 138. Copyright 2021, Springer Nature. | |
In a word, ex/in situ and operando NAP-XPS for SACs are promising in conducting analysis of components with diverse local environments and mechanism studies of gas-involved interfacial processes. Meanwhile, NAP-XPS has developed a lot in gas pressure region and equipment design. Laboratory-based NAP-XPS systems have been used.141 However, it should be highlighted that entropy of the gas phase of even 0.1–1.0 Torr can give rise to massive reconstruction of some catalysts, the influence of which cannot be estimated.142 To reveal authentic surface chemistry of atmospheric processes, measurements in higher pressure even near 1 atm have to be exploited. On the other hand, current NAP-XPS characterizations mainly focus on pressure and temperature fields in gas phase catalysis. The applications of other outfields including electricity, magnetism and illumination need to be exploited for SACs-involved reactions.
2.2.2 UPS.
UPS aims at evaluating electronic binding strength and flowing direction of catalysts. Although most UPS experiments apply ultraviolet light source generated by the de-excitation of excited atoms or ions (mostly helium I and helium II), synchrotron radiation light with broad wave band also covers the ultraviolet energy region.143,144 The work function (Φ), which represents the energy difference between Fermi and vacuum level, can be calculated according to the formula Φ = hν − (Ecutoff − EF), while hν, Ecutoff, EF refers to incident light energy, secondary electron cutoff energy and Fermi energy.145 Gao et al. demonstrated Co SAs immobilized on the partially oxidized graphene nanosheets (Co1–G) with [Ru(bpy)3]Cl2 as a visible-light absorber for photocatalytic CO2 conversion.146 According to UPS with the excitation photon energy of 168.5 eV, the work function of Co1–G is 4.9 eV referenced to the EF = 0 determined from Au (Fig. 12a). And it is reported that the highest occupied molecular orbital (HOMO) and lowest unoccupied molecular orbital (LUMO) energy levels of [Ru(bpy)3]Cl2 are 5.68 and 3.19 eV respectively with respect to the vacuum level.147 Thus electrons transfer from LUMO of [Ru(bpy)3]Cl2 to the Fermi level of Co1–G for the subsequent reduction of CO2 (Fig. 12c). Meanwhile, the energy gap near 0 eV between the EF and VB edge exhibited by the valence-band (VB) spectrum signifies the zero-bandgap feature of Co1–G (Fig. 12b). Wang et al. claimed Pt SACs (PtSACs/C) synthesized by the electroplating strategy with the assistance of graphene oxide membrane (GOM) blocking the diffuse and aggregation of Pt ions in a H-cell.148 The work function and energy gap between the EF and VB edge of PtSACs/C are 4.2 eV and ∼0 eV respectively with the incident photon energy of 40.0 eV (Fig. 12d and e), also suggesting the zero-bandgap trait. Combining UV-vis diffuse reflectance spectrum, the photocatalytic HER process is illustrated in Fig. 12f.
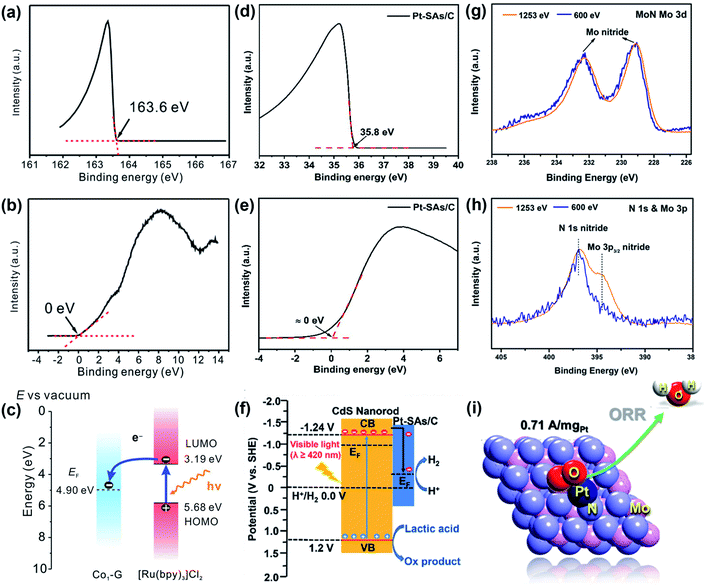 |
| Fig. 12 (a, b) Secondary electron cutoff spectrum (a) and VB spectrum (b) of Co1-G; (c) schematic energy-level diagram. Reprinted with permission from ref. 146. Copyright 2018, WILEY-VCH Verlag GmbH & Co. KGaA, Weinheim. (d, e) Secondary electron cutoff spectrum(d) and VB spectrum (e) of PtSAs/C; (f) the photocatalytic mechanism. Reproduced from ref. 148 with permission from the Royal Society of Chemistry. (g, h) Mo 3d (g) and N 1s (h) spectra of MoN at photon energies of 1253 and 600 eV; (i) optimized structure for Pt supported on MoN. Reprinted with permission from ref. 150. Copyright 2020, American Chemical Society. | |
So benefit from getting information of total catalysts rather than individual elements, the ability of getting and losing electrons of SACs can be evaluated by UPS straightly, which is closely connected with local environments and plays a significant role in unravelling the reactive mechanisms. Otherwise like XPS, the impact of UHV on the interface of catalysts cannot be ignored.
2.2.3 Depth detection of elementary distribution by SRPES.
It is comprehensible that X-ray with different wavenumbers has element-specific detection sensitivity and depth of penetration. It means the difference of elementary distribution between surface and sub-surface can be described by SRPES, in which there is hidden information about local coordinate environments.149 For instance, Li et al. achieved atomically dispersed Pt anchored on face-centered cubic(fcc)-structured MoN by the wet-impregnation and NH3-activation method.150 SRPES was employed to understand the difference between bulk and surface of MoN with photon energies of 1253 and 600 eV. Although Mo 3d spectrum keeps invariable at both conditions (Fig. 12g), the shoulder peak representing Mo 3p almost disappears in the N 1s and Mo 3p spectra at 600 eV (Fig. 12h), indicating N atoms are enriched on the surface. Hence Pt SAs tend to be coordinated with surficial N rather than Mo, agreeing well with EXAFS results (Fig. 12i). But the studies about the depth detection model for SACs are still scarce as far as we know. The fact is that statistical signals coming from both bulk and surface are obtained while employing high-energy X-ray as incident light, which hinders the extraction of valuable responses.
In brief, SRPES points at electronic states of target elements as a surface-sensitive technique, which is complementary to XAS for SACs. On account of the bother of UHV, synchrotron-radiation NAP-XAP or AP-XPS with the controllable atmosphere and temperature develops flourishingly, especially in the thermocatalytic field. In situ and operando NAP-XPS are employed to track the pressure- and temperature-dependent relationships between local environments of SACs and gas reactants in real time, disclosing the reactive steps farther. On the other hand, synchrotron-radiation UPS incorporating UV-vis diffuse reflectance spectra and DFT calculations gives detailed information of the electronic flowing direction. And the adjustable energy feature of synchrotron radiation light makes depth detection of elementary distribution possible. However, it should not be ignored that rigorous service conditions of PES confine the applications of other outfields, which need to be optimized.
2.3 SR-FTIR
SR-FTIR is a significant part of synchrotron-radiation methodologies on account of the convenience for observing intermediates and adsorbates in catalytic processes.151 Compared with conventional light sources, the focused beam of infrared light generated by synchrotron radiation is smaller in size and brighter, significantly optimizing the SNR.152 Moreover, the superior expansibility of SR-FTIR renders it meeting various demands involving in situ, operando, low-temperature, etc. (Fig. 13a)153
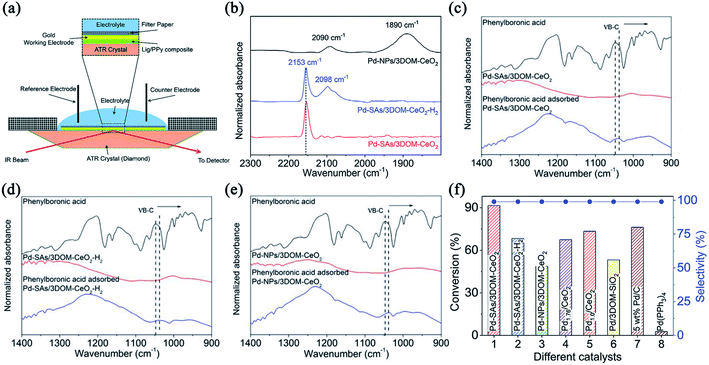 |
| Fig. 13 (a) Schematic representation of the in situ FTIR setup. Reproduced from ref. 153 with permission from the Royal Society of Chemistry. (b) CO-probing spectra of Pd-SAs/3DOM-CeO2, Pd-SAs/3DOM-CeO2-H2 and Pd-NPs/3DOM-CeO2; (c–e) FTIR spectra of phenylboronic acid adsorbed on these catalysts; (f) catalytic performance toward the Suzuki reaction of iodobenzene and phenylboronic acid. Reprinted with permission from ref. 154. Copyright 2020, WILEY-VCH GmbH. | |
Generally, local environments of catalysts have a deep influence on probing molecular bonding types and vibration modes. So the structural features of SACs can be deduced from adsorption behaviours. For instance, synchrotron-radiation CO-probing DRIFTS results of Pd SAs, H2-treated Pd SAs and Pd NPs denoted as Pd-SAs/3DOM-CeO2, Pd-SAs/3DOM-CeO2-H2 and Pd-NPs/3DOM-CeO2 respectively, were contrasted by Tao and coworkers.154 The sharp peak at 2153 cm−1 belongs to strhing variation of linear-adsorbed CO on Pd SAs, indicating atomic dispersion in Pd-SAs/3DOMCeO2 (Fig. 13b). An obvious shift of this sharp peak and another broad peak assigned to the linear-adsorbed CO on Pd clusters are observed, suggesting the clustering and reduction of Pd after H2 treatment. Distinctly, two broad bands located at 1890 and 2090 cm−1 are accord with the threefold-hollow-adsorbed CO and minor linear-adsorbed CO on Pd NPs of Pd-NPs/3DOM-CeO2 respectively. Then to insight into the positive role of Pd SAs, IR spectra were collected after the chemical adsorption of phenylboronic acid. The B–C stretching vibration peak at ∼1050 cm−1 exhibits more downshifts in Pd-SAs/3DOM-CeO2 than other two references, indicating that high-valence isolated Pd sites are more competent to activate phenylboronic acid in transmetalation step of Suzuki coupling reactions (Fig. 13c–e). Pd-SAs/3DOM-CeO2 exhibits superior conversion efficiency and selectivity towards Suzuki reactions, surpassing Pd NPs (Fig. 13f).
Moreover, SR-FTIR method can approach the requirements of in situ and operando methods.155,156 They are able to provide fingerprint identification of real-time active groups, which is of great concern in the hypothesis of reactive pathways for SACs. It is reported that the adsorbed OOH, O and OH intermediates detected by in situ SR-FTIR spectra present in the Cu-catalytic ORR reaction, which agreed well with the proposed mechanism.157 On the other hand, central metal atoms and neighbouring ligand atoms usually take synergetic effect in catalytic reactions. This effect can be distinguished by in situ and operando SR-FTIR, helpfully understanding the relation between local environments and catalytic performances. For instance, Su et al. reported isolated single-atom Ni supported on MOFs-derived carbon nitride (Ni1–NC) through ion-exchange and node-confined procedures.158 Two new absorption bands at 908 and 3465 cm−1 in operando SR-FTIR spectra are assigned to surface adsorbed O intermediate species and O–H from adsorbed water molecules respectively at the applied potentials (Fig. 14a and b). The relation between intensity and voltages was plotted in Fig. 14c. The rising part represents the evident accumulation of adsorbed O intermediates and adsorbed H2O species at the solid–liquid interfaces (SLEIs) under the preliminary voltages, promoting the rate-determining step of O–O dissociation in ORR to some extent. Keeping decreasing potentials versus RHE, the intensity at 908 cm−1 reaches an approximate saturation plateau while ∼3465 cm−1 presents a decline, elucidating the synergistic effect between hydroxyl on N/C sites adjacent to the Ni active centers and crucial adsorbed O intermediates promoted by Ni1N2 active sites. In isotope-labeling operando SR-FTIR with 18O2 and H216O, the shift of O–Ni and unchanged O–H confirm they originate from O2 reactants and H2O in electrolyte respectively (Fig. 14d–f). Operando XAFS and SR-FTIR analyses confirmed the formation of near-free isolated-zigzag Ni1(2−δ)+N2 active sites, which provide excellent ORR activity and durability by facilitating the adsorption and dissociation of O2 into crucial *O intermediates (Fig. 14g–i).
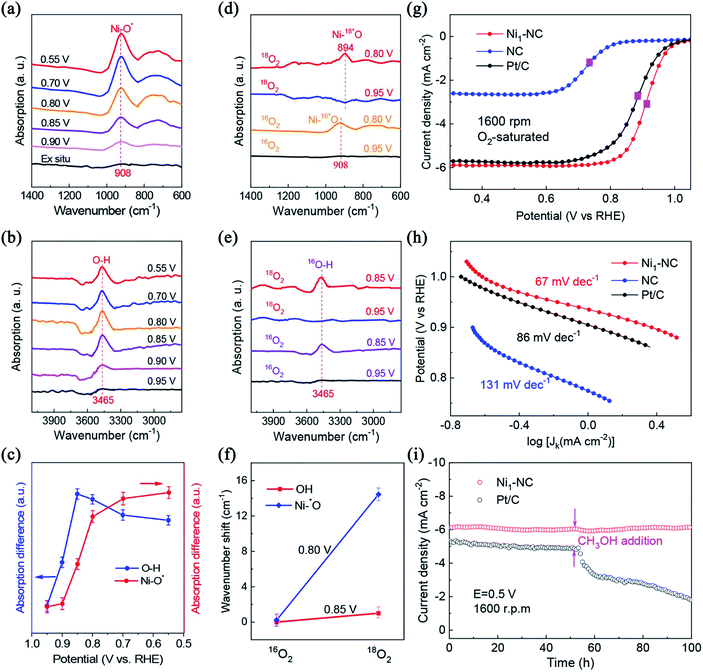 |
| Fig. 14 (a, b) Operando IR measurements of Ni1–NC during the ORR process; (c) signals at 3465 and 908 cm−1versus the potential; (d, e) isotope-labeling operando spectroscopy for Ni1–NC under various potentials; (f) the corresponding wavenumber shift; (g) linear sweep voltammograms (LSVs) at 1600 rpm; (h) Tafel plots; (i) methanol tolerance tests at 0.5 V versus RHE. Reprinted with permission from ref. 158. Copyright 2020, American Chemical Society. | |
Besides, SR-FTIR spectra fit other peculiar testing demands including low-temperature reflection and variable angle reflection.74 For instance, Ye et al. precisely tuned the number of Fe atoms to fabricate Fe SAs, Fe2 and Fe3 clusters anchored on N-doped carbon marked as Fex–N–C by pre-encapsulation and pyrolysis schedules.159 In low-temperature FTIR spectroscopies (LT-FTIR) after exposing catalysts to O2 for 10 min to ensure complete O2 adsorption, broad peaks located at 1200–1350 cm−1 for Fe1–N–C and 800–1000 cm−1 for Fe2 and Fe3–N–C are attributed to the vibrations of adsorbed superoxo-like (akin to Fe–O–O) and peroxo-like (akin to Fe–O–O–Fe) O2 species respectively. This indicates that the number of Fe atoms in clusters can really alter O2 adsorption configurations, in consistence with theoretical calculation results.
Conclusively, the adsorption-dissociation behaviors are regulated extensively by well-defined isolated metal sites with diverse local environments. In situ and operando identification of reactive configurations is vital to put forward accurate elementary reaction pathways of SACs. Specific requirements like low temperature and molecular probing are also able to be approached by SR-FTIR. However, the semi-quantitative character of IR blocks the progressive utilization in plotting the formula between the accumulation of intermediates and catalytic performance. Meanwhile, the peak intensity and width are related to not only the concentrations but also the vibration type of the bonds. The overlaps in wavenumbers of some bonds are also inseparable and inevitable.
3. Summary and perspective
Coupled microscopy and spectroscopy methods, a thorough characterization system for SACs has been built in the past decade. The combination of synchrotron radiation-based multitechniques (SRMS) has proved to be powerful in identifying the diverse local environments of isolated sites.160 This work summarized the advantages and limitations of existing synchrotron-radiation measurements including XAS, SRPES and SR-FTIR. It is worth noting that aforesaid methods cannot replace each other. The reasonable reaction mechanisms are only proposed by the comprehensive knowledge and analysis of all experimental and theoretical results. Based on this, respective roles of synchrotron-radiation tests acting in the studies of SACs and relevant outlooks are described as following.
3.1 Oxidation states
Although the dispersion degree of confined metal atoms should be evaluated straightly by the high-angle annular dark-field scanning transmission electron microscopy (HAADF-STEM), the elementary electropositivity confirmed by bulk-sensitive XANES and surface-sensitive XPS is also efficient. Meanwhile, ex situ XANES spectra detecting beginning and ending states of center and assistant atoms have the ability in deciphering partial mechanisms concerned with irreversible redox activation, electronic interaction and corrosion resistance, etc.92 On the other hand, the valence change of center atoms is strongly related to the redox-involved reaction performance of SACs. Some reversible or instantaneous intermediate states can only be investigated by in situ/operando XANES and NAP-XPS. The flourishing development of computational analysis of in situ XAS results offers the elaborate explanation of the evolution of isolated sites influenced by various outfields.95Operando NAP-XPS is naturally suitable to deal with gas-involved courses through building the relationship between gas molecules and active sites, for thermocatalysis in particularly.
3.2 Coordinate configurations
There is no doubt that the coordinated atoms and bonding types have a deep impact on catalytic activity when SAs are acknowledged as the reactive origin. EXAFS has unique advantages in recognizing the local coordinate environments. The coordinate number and bond length are obtained by EXAFS fitting to distinguish diverse local environments. Moreover, EXAFS satisfies the demands of in situ and operando measurements that are performed to unravel the evolution of chemical environments and structure–property relationship of SACs. Besides, depth detection of elementary distribution by SRPES can give a longitudinal distribution of atomic concentrations in a certain depth range, hinting the bonding modes between metal and ligand atoms.150
3.3 Support coupling effect
The electronic structures and stability of catalytically active sites are regulated by miscellaneous well-defined supports. Understanding the electronic metal–support interaction is of great concern in conducting mechanism researches. For classical structure of that transition metal is bonded with C, N, O, S, etc., soft XAS takes effect in the investigation of orbital hybridization and support coupling effect. Kinds of ligands such as pyridinic N, pyrrolic N and graphitic N, can be distinguished by the peak fitting of soft XAS, which is deeply connected with the adsorption energy of specific intermediates.119
3.4 Intermediates and adsorption behaviors
The interaction between reagents and adsorbed sites was deduced indirectly from the change of oxidation states by XANES and NAP-XPS before. However, the direct observation of adsorption kinds on SACs can only be achieved by in situ and operando SR-FTIR in the family of synchrotron-radiation techniques. The evolution of intermediate states is illustrated by their unique stretching or bending vibration. In addition, UPS explains the interactions between reagents and catalysts through the gaining/losing-electron ability evaluated by the work function.
Despite the unprecedented progress has been achieved in synchrotron-radiation ex/in situ and operando studies, there are multiple factors hindering more comprehensive acknowledgement of SACs-catalytic processes. The aspects that need to be further modified for synchrotron-radiation techniques are listed as following to expect to gain a coherent picture of the structure–property relationship for SACs in the not-too-distant future.
(1) The equipment covering detector, in situ cell, etc. and their relevant installation interval should be standardized and optimized to decrease the time of collecting spectra and increase SNR. For example, low background of XAS provides more possibility to identify slight changes in oxidation states and coordinate environments, or increase the sensitivity for some single-atom model catalysts with trace metal. Especially for soft XAS, the lack of in situ and operando measurements in SACs makes it imperative to design reasonable metrical procedures. For NAP-XPS, the applied atmosphere pressure region should be extended to weaken the near-ambient influence on catalytic structures.
(2) The more convincing data processing methods should be proposed combing theoretical calculation. As shown in chapter two, the XAS analysis is not limited to the contrast of the white line in XANES and least-square fitting in EXAFS any more. The more reasonable structural models are built by coupling of experimental and theoretical spectra. Linear combination fitting (LCF) results using advisable reference spectra can match well with the experimental results, which gives an interpretation of the evolution of probed catalytically active atoms.95 And the species of ligands can be elucidated by resolving adsorption bands in soft XAS spectra. Thus it can be realized that there is considerable progress in handling methods of XAS. And we have reasons to believe that well-disposed XAS will play a more crucial role in the characterization of SACs.
(3) Extending the kind of outfields that can be performed under work conditions is impending. Restricted by the features of diverse techniques, synchrotron-radiation methods usually have their own comfort zones for outfields applying to in situ and operando measurements. For example, NAP-XPS is naturally suitable for thermocatalytic reactions while the employment in electrocatalysis and photocatalysis is relatively fewer for SACs. In situ soft XAS has already been performed for bulk catalysts in electrocatalytic processes, but not for SACs.
(4) The characterization of SACs usually needs a comprehensive system encompassing multiple unique techniques. Thus, more powerful synchrotron-radiation methods need to be exploited for SACs to enrich this characterization family. Synchrotron-radiation X-ray emission spectroscopy (XES) relying on the ionization of electrons and detecting the intensity of the fluorescence at the maintained incident X-ray photon energy, is potential in this case. Valence-to-core XES (VTC-XES) of 3d transition metals can be used to distinguish neighboring ligand atoms of SACs such as C, N, O, S, etc.161 For example, it is reported that the transitions of local environments from isolated Mo–O species to partially carburized MoCxOy and totally carburized MoCx occur during methane dehydroaromatization (MDA), confirmed by characteristic Kβ′′ peak of Mo oxide (Fig. 15a).162 On the other hand, the typical long-range disorder property disables XRD and small-angle X-ray scattering (SAXS) for investigating the SACs under the static state. But in situ/operando XRD and SAXS take effect in tracking the dynamic evolution of isolated sites and supports, which is efficient for evaluating catalytic stability and unveiling clustering processes. For instance, Liu et al. chose single-site Co in a well-defined brookite TiO2 (210) nanorod (Co-TiO2) as a model catalyst for OER. In situ synchrotron-radiation XRD (SRXRD) shows no phase transition for TiO2 support and metal segregation for Co (Fig. 15d), suggesting the good stability.163 And it is reported that obvious agglomeration can be observed through a signal enhancement in the q range around 0.1 Å−1 in operando grazing-incidence SAXS (GISAXS) while calcinating Pt clusters (Pt24/SiO2/Si) from 50 to 200 °C in H2 (Fig. 15b and c).164 Thus it is convincing that SAXS can play a vital part in identifying real sites in the processes of reduction and clustering of SACs.165,166 However, the applications of XES, XRD and SAXS are still scarce in SACs to the best of our knowledge.
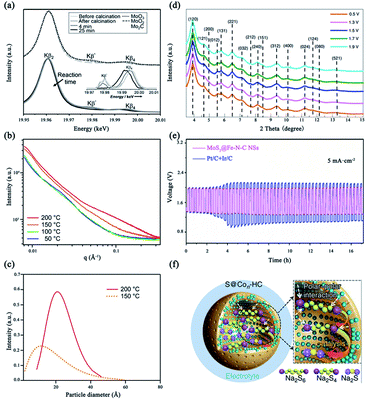 |
| Fig. 15 (a) Bottom: Kβ emission bands recorded before and after calcination, and after quenching of the MDA reaction at 4 min and 25 min; the Inset is background-removed VTC-XES; top: spectra of MoO3, Mo2C, and MoO2 references. Reprinted with permission from ref. 162. Copyright 2016, Wiley-VCH Verlag GmbH & Co. KGaA. (b) GISAXS intensity change of Pt24/SiO2/Si during the heating cycle from 50 to 200 °C in H2; (c) fitted particle size distributions. Reprinted with permission from ref. 164. Copyright 2017, American Chemical Society. (d) In situ SRXRD patterns of Co–TiO2 nanorod catalysts under the OER conditions. Reprinted with permission from ref. 163. Copyright 2021, Springer Nature. (e) The stability curve of ZABs. Reprinted with permission from ref. 170. Copyright 2021, National Academy of Sciences. (f) Schematic illustrations of electrode reaction mechanism. Reprinted with permission from ref. 171. Copyright 2018, Springer Nature. | |
It is worth noting that the applications of SACs have been extended to the whole courses of energy-storage and conversion. Recently, SACs in metal-air and lithium/sodium-sulfur batteries, proton exchange membrane full cells (PEMFC), etc., have attracted great attention.167–169 And synchrotron-radiation spectroscopies also take effect in these conditions. Yan et al. put forward a facile strategy for crafting wrinkled MoS2/N-doped carbon core/shell nanospheres interfaced with single Fe atoms for wearable, high-capacity, and cycling-stability aqueous zinc-air battery (ZABs) (Fig. 15e).170 The Fe–N4 configuration is confirmed by the fitting of FT-EXAFS. Zhang et al. employed sulfur encapsulated Co SAs and clusters anchored on hollow carbon nanospheres (S@Con-HC) as the cathode of room-temperature sodium-sulfur battery (RT-Na/S).171 The transition among S, Na2Sx, Na2S4, Na2S2 and Na2S is observed by in situ SRXRD, suggesting the electrocatalytic effects of atomic cobalt (Fig. 15f).
In summary, the development of SACs is tightly connected with progressive synchrotron-radiation ex/in situ and operando spectroscopies. Particularly, an unambiguous picture of the structure–activity relationship of SACs will be built by tighter combination of multiple in situ and operando synchrotron-radiation technologies. And the further developments of them have to reconcile the depth and breadth. That is, it is paratactic for optimizing data quality of one method and extending the characterization families of SACs. Furthermore, the catalytic performances are usually tailored by electronic interactions, geometric effects, local coordinate configurations, etc. The comprehensive cognitions from synchrotron-radiation spectroscopies, electron microscopies and other important methods are instructive for SACs in either tracking the origin of activities or providing guidelines for optimization designs.
Conflicts of interest
There are no conflicts to declare.
Acknowledgements
This program was financially supported by National Key R & D Program of China (2017YFA0402800, 2020YFA0405800), NSFC (12135012, U1932201), CAS International Partnership Program (211134KYSB20190063), CAS Collaborative Innovation Program of Hefei Science Center (2019HSC-CIP002), The University Synergy Innovation Program of Anhui Province (GXXT-2020-002) and China Postdoctoral Science Foundation (BX20200322, 2020M682009). We thank the Shanghai Synchrotron Radiation Facility (14W1 and 14B1, SSRF), the Beijing Synchrotron Radiation Facility (1W1B, 4W1B and 4B9A, BSRF), the Hefei Synchrotron Radiation Facility (Infrared Spectroscopy and Microspectroscopy, MCD-A and MCD-B Soochow Beamline for Energy Materials, Photoemission and Catalysis/Surface Science Endstations at NSRL) for kind help.
References
- S. C. Doney, V. J. Fabry, R. A. Feely and J. A. Kleypas, Annu. Rev. Mar. Sci., 2009, 1, 169–192 CrossRef PubMed.
- U. Büntgen, P. J. Krusic, A. Piermattei, D. A. Coomes, J. Esper, V. S. Myglan, A. V. Kirdyanov, J. J. Camarero, A. Crivellaro and C. Körner, Nat. Commun., 2019, 10, 2171 CrossRef PubMed.
- P. Veers, K. Dykes, E. Lantz, S. Barth, C. L. Bottasso, O. Carlson, A. Clifton, J. Green, P. Green, H. Holttinen, D. Laird, V. Lehtomäki, J. K. Lundquist, J. Manwell, M. Marquis, C. Meneveau, P. Moriarty, X. Munduate, M. Muskulus, J. Naughton, L. Pao, J. Paquette, J. Peinke, A. Robertson, J. Sanz Rodrigo, A. M. Sempreviva, J. C. Smith, A. Tuohy and R. Wiser, Science, 2019, 366, eaau2027 CrossRef CAS PubMed.
- Y. Zhao, C. Ding, J. Zhu, W. Qin, X. Tao, F. Fan, R. Li and C. Li, Angew. Chem., Int. Ed., 2020, 132, 9740–9745 CrossRef.
- R. F. Service, Science, 2019, 365, 108 CrossRef CAS PubMed.
- C. Wang, S. Chen, H. Xie, S. Wei, C. Wu and L. Song, Adv. Energy Mater., 2019, 9, 1802977 CrossRef.
- R. Liu and C. Streb, Adv. Energy Mater., 2021, 11, 2101120, DOI:10.1002/aenm.202101120.
- Z. Kou, W. Zang, W. Pei, L. Zheng, S. Zhou, S. Zhang, L. Zhang and J. Wang, J. Mater. Chem. A, 2020, 8, 3071–3082 RSC.
- Y. Zhou, Z. Xie, J. Jiang, J. Wang, X. Song, Q. He, W. Ding and Z. Wei, Nat. Catal., 2020, 3, 454–462 CrossRef CAS.
- N. Zhang, X. Feng, D. Rao, X. Deng, L. Cai, B. Qiu, R. Long, Y. Xiong, Y. Lu and Y. Chai, Nat. Commun., 2020, 11, 4066 CrossRef CAS PubMed.
- M. Luo, Z. Zhao, Y. Zhang, Y. Sun, Y. Xing, F. Lv, Y. Yang, X. Zhang, S. Hwang, Y. Qin, J. Y. Ma, F. Lin, D. Su, G. Lu and S. Guo, Nature, 2019, 574, 81–85 CrossRef CAS PubMed.
- S. Cao, Y. Wang, B. Zhu, G. Xie, J. Yu and J. R. Gong, J. Mater. Chem. A, 2020, 8, 7671–7676 RSC.
- X. Pan, F. Jiao, D. Miao and X. Bao, Chem. Rev., 2021, 121, 6588–6609 CrossRef CAS PubMed.
- H. Jiang, Q. He, X. Li, X. Su, Y. Zhang, S. Chen, S. Zhang, G. Zhang, J. Jiang, Y. Luo, P. M. Ajayan and L. Song, Adv. Mater., 2019, 31, 1805127 CrossRef PubMed.
- H. Wang, Z. N. Chen, D. Wu, M. Cao, F. Sun, H. Zhang, H. You, W. Zhuang and R. Cao, J. Am. Chem. Soc., 2021, 143, 4639–4645 CrossRef CAS PubMed.
- D. Liu, X. Li, S. Chen, H. Yan, C. Wang, C. Wu, Y. A. Haleem, S. Duan, J. Lu, B. Ge, P. M. Ajayan, Y. Luo, J. Jiang and L. Song, Nat. Energy, 2019, 4, 512–518 CrossRef CAS.
- S. Yang, Q. He, C. Wang, H. Jiang, C. Wu, Y. Zhang, T. Zhou, Y. Zhou and L. Song, J. Mater. Chem. A, 2018, 6, 11281–11287 RSC.
- A. Wang, J. Li and T. Zhang, Nat. Rev. Chem., 2018, 2, 65–81 CrossRef CAS.
- Z. Zeng, Y. Su, X. Quan, W. Choi, G. Zhang, N. Liu, B. Kim, S. Chen, H. Yu and S. Zhang, Nano Energy, 2020, 69, 104409 CrossRef CAS.
- L. Zhang, R. Long, Y. Zhang, D. Duan, Y. Xiong, Y. Zhang and Y. Bi, Angew. Chem., Int. Ed., 2020, 59, 6224–6229 CrossRef CAS PubMed.
- H. Jin, X. Liu, S. Chen, A. Vasileff, L. Li, Y. Jiao, L. Song, Y. Zheng and S. Z. Qiao, ACS Energy Lett., 2019, 4, 805–810 CrossRef CAS.
- Y. Shang, X. Xu, B. Gao, S. Wang and X. Duan, Chem. Soc. Rev., 2021, 50, 5281–5322 RSC.
- P. Zhou, F. Lv, N. Li, Y. Zhang, Z. Mu, Y. Tang, J. Lai, Y. Chao, M. Luo, F. Lin, J. Zhou, D. Su and S. Guo, Nano Energy, 2019, 56, 127–137 CrossRef CAS.
- W. H. Lai, L. F. Zhang, W. B. Hua, S. Indris, Z. C. Yan, Z. Hu, B. Zhang, Y. Liu, L. Wang, M. Liu, R. Liu, Y. X. Wang, J. Z. Wang, Z. Hu, H. K. Liu, S. L. Chou and S. X. Dou, Angew. Chem., Int. Ed., 2019, 58, 11868–11873 CrossRef CAS PubMed.
- X. Zheng, J. Tang, A. Gallo, J. A. Garrido Torres, X. Yu, C. J. Athanitis, E. M. Been, P. Ercius, H. Mao, S. C. Fakra, C. Song, R. C. Davis, J. A. Reimer, J. Vinson, M. Bajdich and Y. Cui, Proc. Natl. Acad. Sci. U. S. A., 2021, 118, e2101817118 CrossRef CAS PubMed.
- H. Y. Jeong, M. Balamurugan, V. S. K. Choutipalli, E. S. Jeong, V. Subramanian, U. Sim and K. T. Nam, J. Mater. Chem. A, 2019, 7, 10651–10661 RSC.
- W. Xiong, H. Li, H. Wang, J. Yi, H. You, S. Zhang, Y. Hou, M. Cao, T. Zhang and R. Cao, Small, 2020, 16, 2003943 CrossRef CAS PubMed.
- B. Han, Y. Gao, Y. Huang, W. Xi, J. Xu, J. Luo, H. Qi, Y. Ren, X. Liu, B. Qiao and T. Zhang, Angew. Chem., Int. Ed., 2020, 132, 11922–11927 CrossRef.
- T. Zhang, X. Han, H. Yang, A. Han, E. Hu, Y. Li, X. Yang, L. Wang, J. Liu and B. Liu, Angew. Chem., Int. Ed., 2020, 132, 12153–12159 CrossRef.
- B. Chen, T. Wang, S. Zhao, J. Tan, N. Zhao, S. P. Jiang, Q. Zhang, G. Zhou and H. M. Cheng, Adv. Mater., 2021, 33, 2007090 CrossRef CAS PubMed.
- X. Xiao, Y. Gao, L. Zhang, J. Zhang, Q. Zhang, Q. Li, H. Bao, J. Zhou, S. Miao, N. Chen, J. Wang, B. Jiang, C. Tian and H. Fu, Adv. Mater., 2020, 32, 2003082 CrossRef CAS PubMed.
- H. Wang, Q. Wang, Y. Cheng, K. Li, Y. Yao, Q. Zhang, C. Dong, P. Wang, U. Schwingenschlögl, W. Yang and X. X. Zhang, Nano Lett., 2012, 12, 141–144 CrossRef CAS PubMed.
- T. He, S. Chen, B. Ni, Y. Gong, Z. Wu, L. Song, L. Gu, W. Hu and X. Wang, Angew. Chem., Int. Ed., 2018, 130, 3551–3556 CrossRef.
- P. Zhou, N. Li, Y. Chao, W. Zhang, F. Lv, K. Wang, W. Yang, P. Gao and S. Guo, Angew. Chem., Int. Ed., 2019, 58, 14184–14188 CrossRef CAS PubMed.
- J. Han, H. Bao, J. Q. Wang, L. Zheng, S. Sun, Z. L. Wang and C. Sun, Appl. Catal., B, 2021, 280, 119411 CrossRef CAS.
- J. Gao, H. b. Yang, X. Huang, S. F. Hung, W. Cai, C. Jia, S. Miao, H. M. Chen, X. Yang, Y. Huang, T. Zhang and B. Liu, Chem, 2020, 6, 658–674 CAS.
- K. Qi, M. Chhowalla and D. Voiry, Mater. Today, 2020, 40, 173–192 CrossRef CAS.
- Q. He, Y. Wan, H. Jiang, Z. Pan, C. Wu, M. Wang, X. Wu, B. Ye, P. M. Ajayan and L. Song, ACS Energy Lett., 2018, 3, 1373–1380 CrossRef CAS.
- J. Zhang, X. Wu, W. C. Cheong, W. Chen, R. Lin, J. Li, L. Zheng, W. Yan, L. Gu, C. Chen, Q. Peng, D. Wang and Y. Li, Nat. Commun., 2018, 9, 1002 CrossRef PubMed.
- Y. Lu, T. Liu, C. L. Dong, Y. C. Huang, Y. Li, J. Chen, Y. Zou and S. Wang, Adv. Mater., 2021, 33, 2007056 CrossRef CAS PubMed.
- J. Mao, J. Yin, J. Pei, D. Wang and Y. Li, Nano Today, 2020, 34, 100917 CrossRef CAS.
- S. Luo, L. Zhang, Y. Liao, L. Li, Q. Yang, X. Wu, X. Wu, D. He, C. He, W. Chen, Q. Wu, M. Li, E. J. M. Hensen and Z. Quan, Adv. Mater., 2021, 33, 2008508 CrossRef CAS PubMed.
- A. M. Abdel Mageed, B. Rungtaweevoranit, M. Parlinska-Wojtan, X. Pei, O. M. Yaghi and R. J. Behm, J. Am. Chem. Soc., 2019, 141, 5201–5210 CrossRef CAS PubMed.
- Y. Zhu, X. Yang, C. Peng, C. Priest, Y. Mei and G. Wu, Small, 2021, 17, 2005148 CrossRef CAS PubMed.
- J. Ban, X. Wen, H. Xu, Z. Wang, X. Liu, G. Cao, G. Shao and J. Hu, Adv. Funct. Mater., 2021, 31, 2010472 CrossRef CAS.
- Z. Song, L. Zhang, K. Doyle-Davis, X. Fu, J. L. Luo and X. Sun, Adv. Energy Mater., 2020, 10, 2001561 CrossRef CAS.
- P. Peng, L. Shi, F. Huo, C. Mi, X. Wu, S. Zhang and Z. Xiang, Sci. Adv., 2019, 5, eaaw2322 CrossRef CAS PubMed.
- Y. Zhang, L. Jiao, W. Yang, C. Xie and H. L. Jiang, Angew. Chem., Int. Ed., 2021, 60, 7607–7611 CrossRef CAS PubMed.
- J. Hulva, M. Meier, R. Bliem, Z. Jakub, F. Kraushofer, M. Schmid, U. Diebold, C. Franchini and G. S. Parkinson, Science, 2021, 371, 375–379 CrossRef CAS PubMed.
- W. Guo, S. Liu, X. Tan, R. Wu, X. Yan, C. Chen, Q. Zhu, L. Zheng, J. Ma, J. Zhang, Y. Huang, X. Sun and B. Han, Angew. Chem., Int. Ed., 2021, 60, 21979 CrossRef CAS PubMed.
- C. Tang, L. Chen, H. Li, L. Li, Y. Jiao, Y. Zheng, H. Xu, K. Davey and S. Z. Qiao, J. Am. Chem. Soc., 2021, 143, 7819–7827 CrossRef CAS PubMed.
- S. Li, S. Zhao, X. Lu, M. Ceccato, X. M. Hu, A. Roldan, J. Catalano, M. Liu, T. Skrydstrup and K. Daasbjerg, Angew. Chem., Int. Ed., 2021, 60, 22826–22832 CrossRef CAS PubMed.
- Q. Wang, X. Huang, Z. L. Zhao, M. Wang, B. Xiang, J. Li, Z. Feng, H. Xu and M. Gu, J. Am. Chem. Soc., 2020, 142, 7425–7433 CrossRef CAS PubMed.
- L. S. Zhang, X. H. Jiang, Z. A. Zhong, L. Tian, Q. Sun, Y. T. Cui, X. Lu, J. P. Zou and S. L. Luo, Angew. Chem., Int. Ed., 2021, 60, 21751–21755 CrossRef CAS PubMed.
- S. Wang, P. Zhou, L. Zhou, F. Lv, Y. Sun, Q. Zhang, L. Gu, H. Yang and S. Guo, Nano Lett., 2021, 21, 4262–4269 CrossRef CAS PubMed.
- C. Xia, Y. Qiu, Y. Xia, P. Zhu, G. King, X. Zhang, Z. Wu, J. Y. T. Kim, D. A. Cullen, D. Zheng, P. Li, M. Shakouri, E. Heredia, P. Cui, H. N. Alshareef, Y. Hu and H. Wang, Nat. Chem., 2021, 13, 887–894 CrossRef CAS PubMed.
- Y. Xiong, W. Sun, P. Xin, W. Chen, X. Zheng, W. Yan, L. Zheng, J. Dong, J. Zhang, D. Wang and Y. Li, Adv. Mater., 2020, 32, 2000896 CrossRef CAS PubMed.
- N. Zhang, X. Zhang, L. Tao, P. Jiang, C. Ye, R. Lin, Z. Huang, A. Li, D. Pang, H. Yan, Y. Wang, P. Xu, S. An, Q. Zhang, L. Liu, S. Du, X. Han, D. Wang and Y. Li, Angew. Chem., Int. Ed., 2021, 60, 6170–6176 CrossRef CAS PubMed.
- W. Chen, C. Xie, Y. Wang, Y. Zou, C. L. Dong, Y. C. Huang, Z. Xiao, Z. Wei, S. Du, C. Chen, B. Zhou, J. Ma and S. Wang, Chem, 2020, 6, 2974–2993 CAS.
- K. Codling, Rep. Prog. Phys., 1973, 36, 541–624 CrossRef CAS.
- D. D. Babu, Y. Huang, G. Anandhababu, X. Wang, R. Si, M. Wu, Q. Li, Y. Wang and J. Yao, J. Mater. Chem. A, 2019, 7, 8376–8383 RSC.
- Q. He, J. H. Lee, D. Liu, Y. Liu, Z. Lin, Z. Xie, S. Hwang, S. Kattel, L. Song and J. G. Chen, Adv. Funct. Mater., 2020, 30, 2000407 CrossRef CAS.
- W. Xu, D. Cao, O. A. Moses, B. Sheng, C. Wu, H. Shou, X. Wu, S. Chen and L. Song, Nano Res., 2021, 14, 4534–4540 CrossRef CAS.
- J. Wan, Z. Zhao, H. Shang, B. Peng, W. Chen, J. Pei, L. Zheng, J. Dong, R. Cao, R. Sarangi, Z. Jiang, D. Zhou, Z. Zhuang, J. Zhang, D. Wang and Y. Li, J. Am. Chem. Soc., 2020, 142, 8431–8439 CrossRef CAS PubMed.
- A. Beheshti Askari, M. al Samarai, N. Hiraoka, H. Ishii, L. Tillmann, M. Muhler and S. DeBeer, Nanoscale, 2020, 12, 15185–15192 RSC.
- V. Pramhaas, M. Roiaz, N. Bosio, M. Corva, C. Rameshan, E. Vesselli, H. Grönbeck and G. Rupprechter, ACS Catal., 2021, 11, 208–214 CrossRef CAS PubMed.
- N. J. Divins, I. Angurell, C. Escudero, V. Pérez-Dieste and J. Llorca, Science, 2014, 346, 620 CrossRef CAS PubMed.
- S. Zhu, X. Li, X. Jiao, W. Shao, L. Li, X. Zu, J. Hu, J. Zhu, W. Yan, C. Wang, Y. Sun and Y. Xie, Nano Lett., 2021, 21, 2324–2331 CrossRef CAS PubMed.
- S. Béchu, M. Ralaiarisoa, A. Etcheberry and P. Schulz, Adv. Energy Mater., 2020, 10, 1904007 CrossRef.
- Y. Shi, Z. R. Ma, Y. Y. Xiao, Y. C. Yin, W. M. Huang, Z. C. Huang, Y. Z. Zheng, F. Y. Mu, R. Huang, G. Y. Shi, Y. Y. Sun, X. H. Xia and W. Chen, Nat. Commun., 2021, 12, 3021 CrossRef PubMed.
- J. Ma, C. Gao, J. Low, D. Liu, X. Lian, H. Zhang, H. Jin, X. Zheng, C. Wang, R. Long, H. Ji, J. Zhu and Y. Xiong, J. Phys. Chem. C, 2021, 125, 5542–5548 CrossRef CAS.
- J. Xu, Z. Ju, W. Zhang, Y. Pan, J. Zhu, J. Mao, X. Zheng, H. Fu, M. Yuan, H. Chen and R. Li, Angew. Chem., Int. Ed., 2021, 133, 8787–8791 CrossRef.
- C. Lv, L. Zhong, H. Liu, Z. Fang, C. Yan, M. Chen, Y. Kong, C. Lee, D. Liu, S. Li, J. Liu, L. Song, G. Chen, Q. Yan and G. Yu, Nature Sustain., 2021, 4, 868–876 CrossRef.
- C. Hu, X. Wang, Z. Qi and C. Li, Infrared Phys. Technol., 2020, 105, 103200 CrossRef CAS.
- F. de Groot, Chem. Rev., 2001, 101, 1779–1808 CrossRef CAS PubMed.
- M. Wang, L. Árnadóttir, Z. J. Xu and Z. Feng, Nano-Micro Lett., 2019, 11, 47 CrossRef CAS PubMed.
- W. Qu, X. Liu, J. Chen, Y. Dong, X. Tang and Y. Chen, Nat. Commun., 2020, 11, 1532 CrossRef CAS PubMed.
- G. Wang, C.-T. He, R. Huang, J. Mao, D. Wang and Y. Li, J. Am. Chem. Soc., 2020, 142, 19339–19345 CrossRef CAS PubMed.
- L. Jiao, R. Zhang, G. Wan, W. Yang, X. Wan, H. Zhou, J. Shui, S. H. Yu and H. L. Jiang, Nat. Commun., 2020, 11, 2831 CrossRef CAS PubMed.
- J. J. Rehr, J. J. Kas, F. D. Vila, M. P. Prange and K. Jorissen, Phys. Chem. Chem. Phys., 2010, 12, 5503–5513 RSC.
- Y. Chen, R. Gao, S. Ji, H. Li, K. Tang, P. Jiang, H. Hu, Z. Zhang, H. Hao, Q. Qu, X. Liang, W. Chen, J. Dong, D. Wang and Y. Li, Angew. Chem., Int. Ed., 2021, 60, 3212–3221 CrossRef CAS PubMed.
- P. Fornasini and R. Grisenti, J. Synchrotron Radiat., 2015, 22, 1242–1257 CrossRef CAS PubMed.
- Z. Yang, Y. Wang, M. Zhu, Z. Li, W. Chen, W. Wei, T. Yuan, Y. Qu, Q. Xu, C. Zhao, X. Wang, P. Li, Y. Li, Y. Wu and Y. Li, ACS Catal., 2019, 9, 2158–2163 CrossRef CAS.
- H. Zhang, W. Zhou, T. Chen, B. Y. Guan, Z. Li and X. W. Lou, Energy Environ. Sci., 2018, 11, 1980–1984 RSC.
- H. Su, W. Zhou, W. Zhou, Y. Li, L. Zheng, H. Zhang, M. Liu, X. Zhang, X. Sun, Y. Xu, F. Hu, J. Zhang, T. Hu, Q. Liu and S. Wei, Nat. Commun., 2021, 12, 6118 CrossRef CAS PubMed.
- H. Gu, X. Liu, X. Liu, C. Ling, K. Wei, G. Zhan, Y. Guo and L. Zhang, Nat. Commun., 2021, 12, 5422 CrossRef CAS PubMed.
- D. Cao, D. Liu, S. Chen, O. A. Moses, X. Chen, W. Xu, C. Wu, L. Zheng, S. Chu, H. Jiang, C. Wang, B. Ge, X. Wu, J. Zhang and L. Song, Energy Environ. Sci., 2021, 14, 906–915 RSC.
- S. C. Lin, C. C. Chang, S. Y. Chiu, H. T. Pai, T. Y. Liao, C. S. Hsu, W. H. Chiang, M. K. Tsai and H. M. Chen, Nat. Commun., 2020, 11, 3525 CrossRef CAS PubMed.
- C. Genovese, M. E. Schuster, E. K. Gibson, D. Gianolio, V. Posligua, R. Grau Crespo, G. Cibin, P. P. Wells, D. Garai, V. Solokha, S. Krick Calderon, J. J. Velasco Velez, C. Ampelli, S. Perathoner, G. Held, G. Centi and R. Arrigo, Nat. Commun., 2018, 9, 935 CrossRef PubMed.
- F. Wang, J. Ma, S. Xin, Q. Wang, J. Xu, C. Zhang, H. He and X. Cheng Zeng, Nat. Commun., 2020, 11, 529 CrossRef CAS PubMed.
- Q. He, D. Liu, J. H. Lee, Y. Liu, Z. Xie, S. Hwang, S. Kattel, L. Song and J. G. Chen, Angew. Chem., Int. Ed., 2020, 59, 3033–3037 CrossRef CAS PubMed.
- J. Shan, C. Ye, S. Chen, T. Sun, Y. Jiao, L. Liu, C. Zhu, L. Song, Y. Han, M. Jaroniec, Y. Zhu, Y. Zheng and S. Z. Qiao, J. Am. Chem. Soc., 2021, 143, 5201–5211 CrossRef CAS PubMed.
- H. Kim, D. Shin, W. Yang, D. H. Won, H. S. Oh, M. W. Chung, D. Jeong, S. H. Kim, K. H. Chae, J. Y. Ryu, J. Lee, S. J. Cho, J. Seo, H. Kim and C. H. Choi, J. Am. Chem. Soc., 2021, 143, 925–933 CrossRef CAS PubMed.
- M. Bajdich, M. García-Mota, A. Vojvodic, J. K. Nørskov and A. T. Bell, J. Am. Chem. Soc., 2013, 135, 13521–13530 CrossRef CAS PubMed.
- F. Maurer, J. Jelic, J. Wang, A. Gänzler, P. Dolcet, C. Wöll, Y. Wang, F. Studt, M. Casapu and J. D. Grunwaldt, Nat. Catal., 2020, 3, 824–833 CrossRef CAS.
- A. de Juan, J. Jaumot and R. Tauler, Anal. Methods, 2014, 6, 4964–4976 RSC.
- J. Jaumot, A. de Juan and R. Tauler, Chemom. Intell. Lab. Syst., 2015, 140, 1–12 CrossRef CAS.
- A. L. Ankudinov, J. J. Rehr, J. J. Low and S. R. Bare, J. Chem. Phys., 2002, 116, 1911–1919 CrossRef CAS.
- O. Bunău and Y. Joly, J. Phys.: Condens. Matter, 2009, 21, 345501 CrossRef PubMed.
- M. Xiao, J. Zhu, S. Li, G. Li, W. Liu, Y. P. Deng, Z. Bai, L. Ma, M. Feng, T. Wu, D. Su, J. Lu, A. Yu and Z. Chen, ACS Catal., 2021, 11, 8837–8846 CrossRef CAS.
- D. Liu, Q. He, S. Ding and L. Song, Adv. Energy Mater., 2020, 10, 2001482 CrossRef CAS.
- S. Li, M. Dong, J. Yang, X. Cheng, X. Shen, S. Liu, Z. Q. Wang, X. Q. Gong, H. Liu and B. Han, Nat. Commun., 2021, 12, 584 CrossRef CAS PubMed.
- J. Feng, H. Gao, L. Zheng, Z. Chen, S. Zeng, C. Jiang, H. Dong, L. Liu, S. Zhang and X. Zhang, Nat. Commun., 2020, 11, 4341 CrossRef PubMed.
- M. Zhou, Y. Jiang, G. Wang, W. Wu, W. Chen, P. Yu, Y. Lin, J. Mao and L. Mao, Nat. Commun., 2020, 11, 3188 CrossRef CAS PubMed.
- Y. Zhou, X. Tao, G. Chen, R. Lu, D. Wang, M.-X. Chen, E. Jin, J. Yang, H.-W. Liang, Y. Zhao, X. Feng, A. Narita and K. Müllen, Nat. Commun., 2020, 11, 5892 CrossRef CAS PubMed.
- S. Feng, X. Song, Y. Liu, X. Lin, L. Yan, S. Liu, W. Dong, X. Yang, Z. Jiang and Y. Ding, Nat. Commun., 2019, 10, 5281 CrossRef PubMed.
- J. Liu, C. Cao, X. Liu, L. Zheng, X. Yu, Q. Zhang, L. Gu, R. Qi and W. Song, Angew. Chem., Int. Ed., 2021, 60, 15248–15253 CrossRef CAS PubMed.
- H. Jing, Z. Zhao, C. Zhang, W. Liu, D. Wu, C. Zhu, C. Hao, J. Zhang and Y. Shi, Nano Res., 2021, 14, 4025–4032 CrossRef CAS.
- A. Kumar, X. Liu, J. Lee, B. Debnath, A. R. Jadhav, X. Shao, V. Q. Bui, Y. Hwang, Y. Liu, M. G. Kim and H. Lee, Energy Environ. Sci., 2021 10.1039/d1ee02603h.
- X. Chen, J. Wan, J. Wang, Q. Zhang, L. Gu, L. Zheng, N. Wang and R. Yu, Adv. Mater., 2021, 2104764 CrossRef CAS PubMed.
- C. Tang, Y. Jiao, B. Shi, J. N. Liu, Z. Xie, X. Chen, Q. Zhang and S. Z. Qiao, Angew. Chem., Int. Ed., 2020, 59, 9171–9176 CrossRef CAS PubMed.
- K. Liu, X. Zhao, G. Ren, T. Yang, Y. Ren, A. F. Lee, Y. Su, X. Pan, J. Zhang, Z. Chen, J. Yang, X. Liu, T. Zhou, W. Xi, J. Luo, C. Zeng, H. Matsumoto, W. Liu, Q. Jiang, K. Wilson, A. Wang, B. Qiao, W. Li and T. Zhang, Nat. Commun., 2020, 11, 1263 CrossRef CAS PubMed.
- H. Xu, D. Rebollar, H. He, L. Chong, Y. Liu, C. Liu, C. J. Sun, T. Li, J. V. Muntean, R. E. Winans, D. J. Liu and T. Xu, Nat. Energy, 2020, 5, 623–632 CrossRef CAS.
- H. Shang, T. Wang, J. Pei, Z. Jiang, D. Zhou, Y. Wang, H. Li, J. Dong, Z. Zhuang, W. Chen, D. Wang, J. Zhang and Y. Li, Angew. Chem., Int. Ed., 2020, 59, 22465–22469 CrossRef CAS PubMed.
- J. Xu, X. Zheng, Z. Feng, Z. Lu, Z. Zhang, W. Huang, Y. Li, D. Vuckovic, Y. Li, S. Dai, G. Chen, K. Wang, H. Wang, J. K. Chen, W. Mitch and Y. Cui, Nat. Sustain., 2021, 4, 233–241 CrossRef PubMed.
- X. Li, Y. Zeng, C. W. Tung, Y. R. Lu, S. Baskaran, S. F. Hung, S. Wang, C. Q. Xu, J. Wang, T. S. Chan, H. M. Chen, J. Jiang, Q. Yu, Y. Huang, J. Li, T. Zhang and B. Liu, ACS Catal., 2021, 11, 7292–7301 CrossRef CAS.
- L. Cao, Q. Luo, W. Liu, Y. Lin, X. Liu, Y. Cao, W. Zhang, Y. Wu, J. Yang, T. Yao and S. Wei, Nat. Catal., 2019, 2, 134–141 CrossRef CAS.
- Y. Pan, Y. Chen, K. Wu, Z. Chen, S. Liu, X. Cao, W.-C. Cheong, T. Meng, J. Luo, L. Zheng, C. Liu, D. Wang, Q. Peng, J. Li and C. Chen, Nat. Commun., 2019, 10, 4290 CrossRef PubMed.
- N. Zhang, T. Zhou, M. Chen, H. Feng, R. Yuan, C. a. Zhong, W. Yan, Y. Tian, X. Wu, W. Chu, C. Wu and Y. Xie, Energy Environ. Sci., 2020, 13, 111–118 RSC.
- L. Han, M. Hou, P. Ou, H. Cheng, Z. Ren, Z. Liang, J. A. Boscoboinik, A. Hunt, I. Waluyo, S. Zhang, L. Zhuo, J. Song, X. Liu, J. Luo and H. L. Xin, ACS Catal., 2021, 11, 509–516 CrossRef CAS.
- J. Zhou, L. Zhang, Y. C. Huang, C. L. Dong, H. J. Lin, C. T. Chen, L. H. Tjeng and Z. Hu, Nat. Commun., 2020, 11, 1984 CrossRef CAS PubMed.
- X. Zheng, B. Zhang, P. De Luna, Y. Liang, R. Comin, O. Voznyy, L. Han, F. P. García de Arquer, M. Liu, C. T. Dinh, T. Regier, J. J. Dynes, S. He, H. L. Xin, H. Peng, D. Prendergast, X. Du and E. H. Sargent, Nat. Chem., 2018, 10, 149–154 CrossRef CAS PubMed.
- J. Li and J. Gong, Energy Environ. Sci., 2020, 13, 3748–3779 RSC.
- S. Y. Chang, A. Uehara, S. G. Booth, K. Ignatyev, J. F. W. Mosselmans, R. A. W. Dryfe and S. L. M. Schroeder, RSC Adv., 2015, 5, 6912–6918 RSC.
- S. Fang, X. Zhu, X. Liu, J. Gu, W. Liu, D. Wang, W. Zhang, Y. Lin, J. Lu, S. Wei, Y. Li and T. Yao, Nat. Commun., 2020, 11, 1029 CrossRef CAS PubMed.
- X. Li, S. Wang, L. Li, Y. Sun and Y. Xie, J. Am. Chem. Soc., 2020, 142, 9567–9581 CAS.
- J. Cored, A. García-Ortiz, S. Iborra, M. J. Climent, L. Liu, C. H. Chuang, T. S. Chan, C. Escudero, P. Concepción and A. Corma, J. Am. Chem. Soc., 2019, 141, 19304–19311 CrossRef CAS PubMed.
- L. Han, L. Zhang, H. Wu, H. Zu, P. Cui, J. Guo, R. Guo, J. Ye, J. Zhu, X. Zheng, L. Yang, Y. Zhong, S. Liang and L. Wang, Adv. Sci., 2019, 6, 1900006 CrossRef PubMed.
- X. Zu, Y. Zhao, X. Li, R. Chen, W. Shao, Z. Wang, J. Hu, J. Zhu, Y. Pan, Y. Sun and Y. Xie, Angew. Chem., Int. Ed., 2021, 133, 13959–13965 CrossRef.
- L. Cao, W. Liu, Q. Luo, R. Yin, B. Wang, J. Weissenrieder, M. Soldemo, H. Yan, Y. Lin, Z. Sun, C. Ma, W. Zhang, S. Chen, H. Wang, Q. Guan, T. Yao, S. Wei, J. Yang and J. Lu, Nature, 2019, 565, 631–635 CrossRef CAS PubMed.
- L. Nguyen, F. F. Tao, Y. Tang, J. Dou and X. J. Bao, Chem. Rev., 2019, 119, 6822–6905 CrossRef PubMed.
- M. G. Sibi, D. Verma, H. C. Setiyadi, M. K. Khan, N. Karanwal, S. K. Kwak, K. Y. Chung, J. H. Park, D. Han, K. W. Nam and J. Kim, ACS Catal., 2021, 11, 8382–8398 CrossRef CAS.
- K. Roy, L. Artiglia and J. A. van Bokhoven, ChemCatChem, 2018, 10, 666–682 CrossRef CAS.
- D. Cao, O. A. Moses, B. Sheng, S. Chen, H. Pan, L. Wu, H. Shou, W. Xu, D. Li, L. Zheng, S. Chu, C. Hu, D. Liu, S. Wei, X. Zheng, Z. Qi, X. Wu, J. Zhang and L. Song, Sci. Bull., 2021, 66, 553–561 CrossRef CAS.
- S. Liu, H. B. Yang, S. F. Hung, J. Ding, W. Cai, L. Liu, J. Gao, X. Li, X. Ren, Z. Kuang, Y. Huang, T. Zhang and B. Liu, Angew. Chem., Int. Ed., 2020, 59, 798–803 CrossRef CAS PubMed.
- A. C. Schilling, K. Groden, J. P. Simonovis, A. Hunt, R. T. Hannagan, V. Çınar, J. S. McEwen, E. C. H. Sykes and I. Waluyo, ACS Catal., 2020, 10, 4215–4226 CrossRef CAS.
- S. Wu, K. Y. Tseng, R. Kato, T. S. Wu, A. Large, Y. K. Peng, W. Xiang, H. Fang, J. Mo, I. Wilkinson, Y. L. Soo, G. Held, K. Suenaga, T. Li, H. Y. T. Chen and S. C. E. Tsang, J. Am. Chem. Soc., 2021, 143, 9105–9112 CrossRef CAS PubMed.
- V. Muravev, G. Spezzati, Y. Q. Su, A. Parastaev, F. K. Chiang, A. Longo, C. Escudero, N. Kosinov and E. J. M. Hensen, Nat. Catal., 2021, 4, 469–478 CrossRef CAS.
- G. N. Vayssilov, Y. Lykhach, A. Migani, T. Staudt, G. P. Petrova, N. Tsud, T. Skála, A. Bruix, F. Illas, K. C. Prince, V. r. Matolín, K. M. Neyman and J. Libuda, Nat. Mater., 2011, 10, 310–315 CrossRef CAS PubMed.
- A. Neitzel, A. Figueroba, Y. Lykhach, T. Skála, M. Vorokhta, N. Tsud, S. Mehl, K. Ševčíková, K. C. Prince, K. M. Neyman, V. Matolín and J. Libuda, J. Phys. Chem. C, 2016, 120, 9852–9862 CrossRef CAS.
- Y. Ma, Y. Ren, Y. Zhou, W. Liu, W. Baaziz, O. Ersen, C. Pham Huu, M. Greiner, W. Chu, A. Wang, T. Zhang and Y. Liu, Angew. Chem., Int. Ed., 2020, 59, 21613–21619 CrossRef CAS PubMed.
- F. Tao, S. Dag, L. W. Wang, Z. Liu, D. R. Butcher, H. Bluhm, M. Salmeron and G. A. Somorjai, Science, 2010, 327, 850 CrossRef CAS PubMed.
- J. Yang, W. Li, D. Wang and Y. Li, Adv. Mater., 2020, 32, 2003300 CrossRef CAS PubMed.
- X. Li, Y. Sun, J. Xu, Y. Shao, J. Wu, X. Xu, Y. Pan, H. Ju, J. Zhu and Y. Xie, Nat. Energy, 2019, 4, 690–699 CrossRef CAS.
- M. Xiao, L. Gao, Y. Wang, X. Wang, J. Zhu, Z. Jin, C. Liu, H. Chen, G. Li, J. Ge, Q. He, Z. Wu, Z. Chen and W. Xing, J. Am. Chem. Soc., 2019, 141, 19800–19806 CrossRef CAS PubMed.
- C. Gao, S. Chen, Y. Wang, J. Wang, X. Zheng, J. Zhu, L. Song, W. Zhang and Y. Xiong, Adv. Mater., 2018, 30, 1704624 CrossRef PubMed.
- C. Gao, Q. Meng, K. Zhao, H. Yin, D. Wang, J. Guo, S. Zhao, L. Chang, M. He, Q. Li, H. Zhao, X. Huang, Y. Gao and Z. Tang, Adv. Mater., 2016, 28, 6485–6490 CrossRef CAS PubMed.
- Z. Wang, J. Yang, J. Gan, W. Chen, F. Zhou, X. Zhou, Z. Yu, J. Zhu, X. Duan and Y. Wu, J. Mater. Chem. A, 2020, 8, 10755–10760 RSC.
- F. Tao, M. E. Grass, Y. Zhang, D. R. Butcher, J. R. Renzas, Z. Liu, J. Y. Chung, B. S. Mun, M. Salmeron and G. A. Somorjai, Science, 2008, 322, 932 CrossRef CAS PubMed.
- S. Li, J. Liu, Z. Yin, P. Ren, L. Lin, Y. Gong, C. Yang, X. Zheng, R. Cao, S. Yao, Y. Deng, X. Liu, L. Gu, W. Zhou, J. Zhu, X. Wen, B. Xu and D. Ma, ACS Catal., 2020, 10, 907–913 CrossRef CAS.
- Y. Lu, J. Wang, L. Yu, L. Kovarik, X. Zhang, A. S. Hoffman, A. Gallo, S. R. Bare, D. Sokaras, T. Kroll, V. Dagle, H. Xin and A. M. Karim, Nat. Catal., 2019, 2, 149–156 CrossRef CAS.
- W. Duncan and G. Williams, Appl. Opt., 1983, 22, 2914–2923 CrossRef CAS PubMed.
- F. N. Ajjan, M. J. Jafari, T. Rębiś, T. Ederth and O. Inganäs, J. Mater. Chem. A, 2015, 3, 12927–12937 RSC.
- X. Tao, R. Long, D. Wu, Y. Hu, G. Qiu, Z. Qi, B. Li, R. Jiang and Y. Xiong, Small, 2020, 16, 2001782 CrossRef CAS PubMed.
- C. Wu, S. Ding, D. Liu, D. Li, S. Chen, H. Wang, Z. Qi, B. Ge and L. Song, Research, 2020, 2020, 5860712 CAS.
- W. Zhou, H. Su, Z. Wang, F. Yu, W. Wang, X. Chen and Q. Liu, J. Mater. Chem. A, 2021, 9, 1127–1133 RSC.
- H. Shang, X. Zhou, J. Dong, A. Li, X. Zhao, Q. Liu, Y. Lin, J. Pei, Z. Li, Z. Jiang, D. Zhou, L. Zheng, Y. Wang, J. Zhou, Z. Yang, R. Cao, R. Sarangi, T. Sun, X. Yang, X. Zheng, W. Yan, Z. Zhuang, J. Li, W. Chen, D. Wang, J. Zhang and Y. Li, Nat. Commun., 2020, 11, 3049 CrossRef CAS PubMed.
- H. Su, W. Zhou, H. Zhang, W. Zhou, X. Zhao, Y. Li, M. Liu, W. Cheng and Q. Liu, J. Am. Chem. Soc., 2020, 142, 12306–12313 CrossRef CAS PubMed.
- W. Ye, S. Chen, Y. Lin, L. Yang, S. Chen, X. Zheng, Z. Qi, C. Wang, R. Long, M. Chen, J. Zhu, P. Gao, L. Song, J. Jiang and Y. Xiong, Chem, 2019, 5, 2865–2878 CAS.
- D. Cao, H. Shou, S. Chen and L. Song, Curr. Opin. Electrochem., 2021, 30, 100788 CrossRef CAS.
- S. G. Han, D. D. Ma and Q. L. Zhu, Small Methods, 2021, 5, 2100102 CrossRef CAS.
- I. Lezcano-González, R. Oord, M. Rovezzi, P. Glatzel, S. W. Botchway, B. M. Weckhuysen and A. M. J. A. C. Beale, Angew. Chem., Int. Ed., 2016, 128, 5301–5305 CrossRef.
- C. Liu, J. Qian, Y. Ye, H. Zhou, C. J. Sun, C. Sheehan, Z. Zhang, G. Wan, Y.-S. Liu, J. Guo, S. Li, H. Shin, S. Hwang, T. B. Gunnoe, W. A. Goddard and S. Zhang, Nat. Catal., 2021, 4, 36–45 CrossRef CAS.
- Y. Dai, T. J. Gorey, S. L. Anderson, S. Lee, S. Lee, S. Seifert and R. E. Winans, J. Phys. Chem. C, 2017, 121, 361 CrossRef CAS.
- J. Timoshenko, A. Halder, B. Yang, S. Seifert, M. J. Pellin, S. Vajda and A. I. Frenkel, J. Phys. Chem. C, 2018, 122, 21686 CrossRef CAS.
- L. Fang, S. Seifert, R. E. Winans and T. Li, Small Methods, 2021, 5, 2001194 CrossRef CAS.
- B. Chen, X. Zhong, G. Zhou, N. Zhao and H. M. Cheng, Adv. Mater., 2021, 2105812 CrossRef PubMed.
- X. Wang, Y. Li, Y. Wang, H. Zhang, Z. Jin, X. Yang, Z. Shi, L. Liang, Z. Wu, Z. Jiang, W. Zhang, C. Liu, W. Xing and J. Ge, Proc. Natl. Acad. Sci. U. S. A., 2021, 118, e2107332118 CrossRef CAS PubMed.
- X. Cheng, J. Yang, W. Yan, Y. Han, X. Qu, S. Yin, C. Chen, R. Ji, Y. Li, G. Li, G. Li, Y. Jiang and S. Sun, Energy Environ. Sci., 2021, 14, 5958–5967 RSC.
- Y. Yan, S. Liang, X. Wang, M. Zhang, S.-M. Hao, X. Cui, Z. Li and Z. Lin, Proc. Natl. Acad. Sci. U. S. A., 2021, 118, e2110036118 CrossRef CAS PubMed.
- B. W. Zhang, T. Sheng, Y. D. Liu, Y. X. Wang, L. Zhang, W. H. Lai, L. Wang, J. Yang, Q. F. Gu, S. L. Chou, H. K. Liu and S. X. Dou, Nat. Commun., 2018, 9, 4082 CrossRef PubMed.
Footnote |
† These authors contributed equally to this work. |
|
This journal is © The Royal Society of Chemistry 2022 |