DOI:
10.1039/D2QO00736C
(Research Article)
Org. Chem. Front., 2022,
9, 4654-4662
α-Vinyl azide–cysteine click coupling reaction enabled bioorthogonal peptide/protein modification†
Received
7th May 2022
, Accepted 7th July 2022
First published on 11th July 2022
Abstract
α-Alkyl and α-aryl vinyl azides were found to be able to couple with cysteine-derived alkyl thiols chemoselectively under mild conditions, providing the corresponding β-ketosulfides with simultaneous extrusion of N2 and ammonia. This reaction was developed into an effective chemical platform for peptide and protein modification, which is completely cysteine selective and highly bioorthogonal, that avoids the interference from other native residues. The in situ formed ketone group can react specifically with alkoxyamines or hydrazines and therefore can serve as a versatile handle for a second modification. The modification of bovine serum albumin (BSA) with a dansyl fluorescent probe and the labeling of the genetically encoded fluorescent protein YPet-ECFP with biotin have been accomplished successfully through this platform.
Chemical modifications of peptides or proteins, or alternatively bioconjugations, have been proved and continue to be a powerful means to construct bioconjugates with improved or desired properties and have thus been widely used not only in chemical biology to study cellular processes but also in the medical industry to prepare biopharmaceuticals.1 Current trends in chemical modification emphasize on chemoselectivity, site-specificity and bioorthogonality.2 Cysteine, a proteogenic amino acid that is relatively less abundant yet ubiquitously distributed among mammalian proteins, has a number of distinct chemical properties related to the characteristic sulfhydryl group. Collectively, these merits make cysteine arguably the most popular endogenous residue for protein bioconjugation, and a myriad of methods for selective cysteine ligation have been developed over the years.3 Conventional cysteine modification reactions include disulfide bond formation,4 SN2 alkylation,5 SNAr (hetero)arylation6 and the most practiced conjugate addition to an array of electrophilic alkenes and alkynes.7 Recently, a number of creative methods have appeared, such as transition metal mediated cysteine arylation, alkenation, allyation and borylation,8 hypervalent iodine and alkynyl dibenzothiophenium reagent mediated cysteine functionalizations,9 light, strain or proximity promoted thiol–ene/yne reactions,10 and others.11 Overall, from the mechanistic perspective, the majority of the aforementioned modification methods involve the coupling of the nucleophilic cysteine thiol group with a particular electrophile under certain conditions, which can potentially be interfered by other biological nucleophiles (Fig. 1a). Disulfide exchange and retro-Michael addition also do harm to the stability, homogeneity and structural integrity of the relevant bioconjugates. On the other hand, thiol ene/yne reactions of inactivated alkenes/alkynes usually proceed with exclusive chemical or site selectivity to give robust products, but their applications are quite restricted probably because of the slow kinetics or the requirement for radical initiators (Fig. 1b).10 Therefore, there is an unmet need for a chemoselective and bioorthogonal chemical cysteine-modifying protocol that can deliver robust bioconjugates. To this end, we report such a protocol based on an α-vinyl azide–cysteine click coupling reaction that affords stable β-ketosulfide adducts through a radical C–S bond forming event (Fig. 1c).
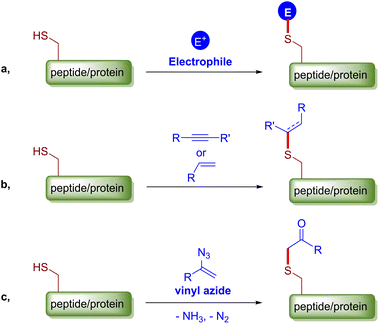 |
| Fig. 1 Chemical modification of a peptide or protein at the cysteine residue. | |
The reaction of aryl thiol with α-aryl vinyl azide to give a β-ketosulfide derivative was initially noted by Montevecchi and co-workers in 1997.12 Recently, our group reinvestigated this coupling process and expanded the scope of azides to α-alkyl vinyl azides.13 This thioether forming transformation takes place smoothly under benign conditions, without the need for extra additives and releasing ammonia and nitrogen as the only by-products. In addition, an aryl thiyl radical initiated radical-chain process was proposed and this process should tolerate aqueous medium due to its radical nature. These features prompted us to envisage that this reaction could evolve into an ideal platform for cysteine modification, based on the assumption that alkyl thiol is also able to participate in this radical event (Fig. 1c). To examine this premise, cysteine-derived alkyl mercaptan 2a was reacted with α-phenyl vinyl azide 1a (Table 1). To our delight, when mixed and stirred with 10 fold of 1a in THF at 40 °C under air, the cysteine derivative 2a disappeared in 30 min, as shown by TLC, and the desired β-ketosulfide 3aa was isolated in 77% yield (entry 1). An additional compound was obtained and identified as 4a, an oxidative homo-coupling product of 2a. Cysteine containing dipeptide 2b reacted similarly with 1a to deliver conjugate 3ab in 61% yield (entry 2). Furthermore, thioethers 3ac–an were obtained successfully from the coupling of 1a with the corresponding dipeptides 2c–n, indicating the good compatibility of this reaction with various proteogenic side chains such as thioether, hydroxyl, phenol, indole, and primary amide groups (entries 3–14). Tripeptide 2o with a cysteine residue in the middle was also a good substrate for this coupling reaction, affording adduct 3ao in 61% isolated yield (entry 15). Vinyl azide 1b, carrying a strong electron donating pivalamido group at the para position of the phenyl ring, reacted quickly with protected dipeptides 2b and 2l and tripeptide 2p to give 3bb, 3bl and 3bp, respectively. The relatively lower yields (30–40%) observed for these three reactions might be attributed to the use of a much less amount (2.0 vs. 10 equiv.) of vinyl azide (entries 16–18). With the restoration of the amount of vinyl azide to 10 equiv., the recovery in yields (50% for 3bj and 61% for 3bk) was observed for the reactions of 1b with 2j and 2k (entries 19 and 20). Notably, although the reaction time varied broadly from less than 1 h to as long as 8 h, the yields were constantly moderate to good, and in all cases, the dimerization of the related alkyl thiols via S–S bond formation was more or less detected, which is a detrimental sideway that competes with the desired C–S bond forming event for the cysteine sulfhydryl group.
Table 1 Coupling reaction of cysteine derivatives with α-aryl vinyl azidesa
After realizing and evaluating the ligation of cysteine derivatives with α-aryl vinyl azides, we then turned to the reaction of the more stable and hence less reactive α-alkyl vinyl azides. (Benzo)imidazole substituted vinyl azides 1c–j were readily obtained and reacted with 2a under the above conditions. The reactions did occur, but we encountered considerable difficulty in separating the thioether products from the corresponding vinyl azides through silica gel column chromatography. To avoid this annoying problem, an excess of cysteine 2a was employed to ensure complete consumption of vinyl azides, and the outcomes are presented in Table 2. Vinyl azides 1c–f, each carrying an imidazole moiety, all coupled successfully with alkyl thiol 2a to give rise to the related conjugates 3ca–3fa in high yields (entries 1–4). The lower reactivity of alkyl vinyl azides was evidenced by the much longer reaction time needed for their full conversions (20–24 h). With their benzoimidazole congeners 1g–j, even their corresponding adducts 3g–j were collected in quantitative yields (entries 5–8, 90–99% yield). Two equivalents of 2a were found to be capable of consuming the alkyl vinyl azide in all instances.
Table 2 Coupling reaction of BocNHCysOMe with α-alkyl vinyl azidesa
With this robust thioether-forming protocol in hand, our efforts were directed to evaluate its feasibility in selective cysteine modification employing phosphate buffered saline (PBS) as the solvent or cosolvent. Commercially available glutathione (GSH, 2q), a cysteine-containing natural peptide antioxidant, was used as the model substrate (Table 3). In an open vial, a solution of vinyl azide 1a (2.0 equiv.) in THF and a solution of glutathione (1.0 equiv.) in PBS (pH 7.4) were mixed and stirred at 40 °C. GSH 2q disappeared in 4 h and the coupling product 3aq was isolated in 75% yield through reverse phase column chromatography (entry 1). Without exception, α-aryl vinyl azides 1k–m were all proven to be competent agents for cysteine ligation, furnishing the corresponding conjugates 3kq–mq efficiently in longer reaction times (entries 2–4). Aliphatic vinyl azides 1n and 1i also demonstrated substantial potential in cysteine ligation (entries 5 and 6). For vinyl azides 1e, 1f and 1o–q that can dissolve well in water, the coupling reactions were conducted in PBS buffer without the use of THF as the co-solvent. For these reactions, the yields were determined from their LC-mass spectra (entries 7–12). In PBS buffer, both imidazolyl vinyl azides 1e and 1f underwent this thiyl radical initiated process faster than parallel events in THF–PBS cosolvents, affording 3eq and 3fq in 71% and 88% LC-MS yields, respectively (entries 7 and 8). The benzoimidazolium functionalized homologues 1o and 1p also efficiently modified GSH at cysteine with high yields in PBS (entries 9 and 10). Glycopeptide 3qq was constructed via the modification of GSH with 1q, a glucoside functionalized with a vinyl azide moiety at the anomeric position (entry 11). As shown in entry 12, the use of water to replace PBS as the reaction medium is feasible as well, albeit with a slight decrease in the yield. Masking the thiol group with trityl protection resulted in no reaction with 1a under the standard conditions even if stirred in air for 24 hours, indicating the inertness of the free amino and carboxyl groups toward this vinyl azide. At this point, the effectiveness of this reaction for selective chemical modification at cysteine has been firmly established. It is worth noting that in 2017, Chiba and colleagues realized site-specific modification at the cysteine residue in peptides and proteins using 2-azidoacrylates.14 Although 2-azidoacrylates also fall in the class of vinyl azides, they serve as Michael acceptors and undergo 1,4-addition with free cysteinyl thiols, a mechanism totally different from the radical chain process for the present method.
Table 3 Bioconjugation of glutathione with α-vinyl azidesa

|
Entry |
Vinyl azide |
Solvent |
Adduct, yield |
Entry |
Vinyl azide |
Solvent |
Adduct, yield |
Time |
Time |
Unless otherwise noted, all reactions were carried out with 0.2 mmol GSH 2q (1.0 equiv.) and 0.4 mmol vinyl azide 1 (2.0 equiv.) at 40 °C, using 2.0 mL PBS (pH 7.4) as the solvent or cosolvent.
Isolated yields are reported.
LC-MS yields are reported.
|
1b |
|
PBS/THF (1/1, v/v) |
|
7c |
|
PBS |
|
4.0 h |
8.0 h |
2b |
|
PBS/THF (1/1, v/v) |
|
8c |
|
PBS |
|
24 h |
8.0 h |
3b |
|
PBS/THF (1/1, v/v) |
|
9c |
|
PBS |
|
24 h |
8.0 h |
4b |
|
PBS/THF (1/1, v/v) |
|
10c |
|
PBS |
|
24 h |
8.0 h |
5b |
|
PBS/THF (1/1, v/v) |
|
11c |
|
PBS |
|
24 h |
8.0 h |
6b |
|
PBS/THF (1/1, v/v) |
|
12c |
|
H2O (pH 7.0) |
|
8.0 h |
30 h |
To further investigate the applicability of the above system in protein chemical ligation, dansylated vinyl azide 1r was obtained and incubated with bovine serum albumin (BSA, 2r, 68 kDa) at 40 °C for 24 in air. In two solvent mixtures, i.e. 1/1 and 1/3 EtOH/PBS (v/v), two parallel experiments were conducted, respectively (Fig. 2a). SDS-PAGE (sodium dodecyl sulfate polyacrylamide gel electrophoresis) analysis of these reactions was carried out and the results are shown in Fig. 2b. For each reaction sample, a strong fluorescent-emission band appears around 70 kDa in the fluorescence image, which can also be visualized through Coomassie blue staining (lanes R1 and R2). As a comparison, no such band is observed in the fluorescence image but showed up upon staining for the control experiment for BSA (lane BSA). As expected, no protein band appears for the control with vinyl azide 1r (lane 1r). The fluorescent bands at the bottom correspond to the dansylated reagent 1r. These experimental outcomes clearly demonstrate that BSA has been efficiently labeled by the dansyl fluorescent vinyl azide. And we claim with confidence that the current methodology can serve as an effective chemical tool for selective protein modification.
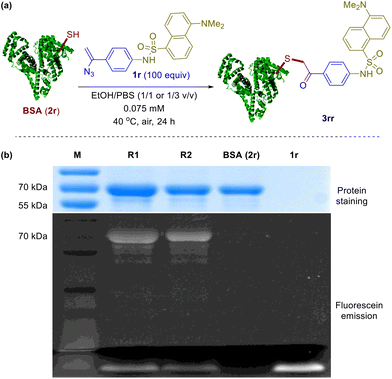 |
| Fig. 2 Labeling of BSA with a dansyl fluorescent probe: (a) coupling reaction of BSA with 1r; and (b) SDS-PAGE analysis (M: protein marker, R1: reaction in 1/1 EtOH/PBS (v/v), R2: reaction in 1/3 EtOH/PBS (v/v), BSA: control experiment without 1r, 1r: control experiment without BSA. | |
The validity of this biocompatible chemical tool in protein modification was further confirmed through the successful decoration of nickel NTA agarose beads (Ni-NTA) with a fluorescent protein (FP) carrying three fluorophores (Fig. 3a). Genetically encoded FP YPet-ECFP (2s),15 a 6×His tagged bioconjugate comprising a yellow fluorescent protein (YPet) and an enhanced cyan fluorescent protein (ECFP) linked via a peptide CPKESCNLFVLKD that bears two cysteine residues, was incubated with excess biotin-derived vinyl azide Biotin1s at 37 °C under physiological reaction conditions for 40 h. This reaction mixture, presumably containing the biotinylated FP 3ss, was then shaken with the Ni-NTA resin for 1 h and the biotin-grafted nickel particles Ni-3ss were obtained through subsequent centrifugation and washing. A 30 min treatment of these freshly made biotin-modified nickel beads with the dye Alexa Fluor® 568 streptavidin (STAV AF568) in PBS completed the installment of a third chromophore. After the removal of the unbound streptavidin dye molecules, the thus prepared nickel complex Ni-YPet-ECFP-AF568 was suspended in PBS and visualized through fluorescence microscopy (Fig. 3b). The bright annuli in both ECFP and YPet emission images suggest the successful attachment of YPet-ECFP (2s) onto the surface of the Ni-coated NTA resin beads; the identical but red annuli in the Alex Flor 568 emission microscopy spectrum indicate an efficient labeling of the Ni-NTA resin surface with the streptavidin dye STAV AF568 (Fig. 3b row I). These observations confirmed the successful installation of biotin segments on the nickel beads, which was accomplished via the coupling reaction of YPet-ECFP (2s) with biotin-conjugated vinyl azide Biotin1s. Following the same protocol but omitting the azide agent Biotin1s, a control sample was prepared and subjected to fluorescence imaging too (Fig. 3b row II). The green and yellow rings were present in its ECFP and YPet emissions, just like those in row I for Ni-YPet-ECFP-AF568. In contrast, in its Alex Fluor 568 emission spectrum, the otherwise red rings now become invisible but with even distribution of the red fluorescence in the beads. This sharp difference might be attributed to the staining of the whole Ni-NTA resin beads with the dye STAV AF568 simply via kinetic diffusion when no specific biotin–streptavidin conjugation took place.
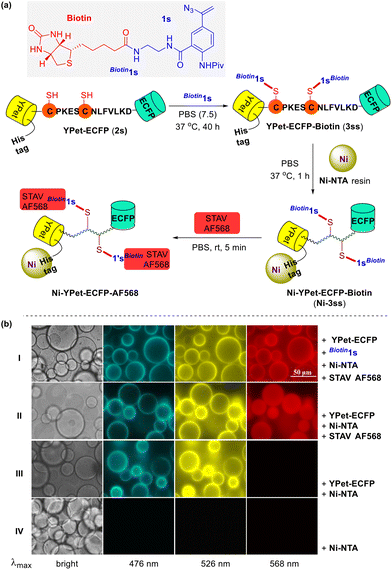 |
| Fig. 3 (a) The labeling of Ni-NTA resin with 6×His-tagged FP conjugate YPet-ECFP and streptavidin-Alex Flor 568 dye STAV AF568; and (b) fluorescence imaging of labeled, unlabeled Ni-NTA resin and control samples. | |
Conclusions
In summary, we have disclosed a coupling reaction of α-vinyl azides with cysteine-based alkyl mercaptans which gives stable β-ketothioethers. This reaction possesses several distinct merits that potentially make it a magnificent chemical platform for protein bioconjugation: (1) the intrinsic radical nature makes it completely feasible for use in aqueous solvent systems; (2) the non-electrophilic vinyl azide used prevents the attack from biological nucleophiles, especially other native amino acid side chains; (3) there is no need for extra reagents and the harmlessness of the by-products formed show its excellent biocompatibility; and moreover (4) the in situ formed stable β-ketothioether linkage might serve as a second ligating site specific for primary amine, hydrazine and alkoxylamine. These merits meet the characteristics of click reactions and we therefore would like to name this C–S bond forming process as vinyl azide–cysteine (VA–Cys) click coupling.16 Using this click reaction, the chemical installation of β-ketothioether on glutathione at cysteine has been realized with an array of α-vinyl azides under (quasi)physiological conditions. The effective fluorescent labelling of BSA with a dansylated α-vinyl azide shows its effectiveness in protein modification. Its avidity is confirmed unequivocally through the successful introduction of a biotin functionality to an engineered FP YPet-ECFP. Based on these solid experimental data, we can claim confidently that a useful chemical platform for selective and bioorthogonal protein modification at the cysteine residue has been established. Further studies aiming to extend its application scenarios are ongoing in our laboratory.
Conflicts of interest
There are no conflicts to declare.
Acknowledgements
The authors thank the National Natural Science Foundation of China (22177015), the Natural Science Foundation of Jiangsu Province (BK20211334) and the Postgraduate Research & Practice Innovation Program of Jiangsu Province (KYCX21-2860) for financial support. This work is also supported by the State Key Laboratory of Organic Electronics and Information Displays, Institute of Advanced Materials (IAM), Nanjing University of Posts & Telecommunications. We are grateful to Dr Aijun Cui and Dr Li Liu, Analysis and Testing Centre, NERC Biomass of Changzhou University, for their help with the NMR analysis.
Notes and references
-
(a)
Chemoselective and Bioorthogonal Ligation Reactions: Concepts and Applications, ed. W. Russ Algar, Philip E. Dawson and Igor L. Medintz, WILEY-VCH Verlag GmbH & Co. KGaA, 2017 Search PubMed;
(b) O. Boutureira and G. J. L. Bernardes, Advances in Chemical Protein Modification, Chem. Rev., 2015, 115, 2174 CrossRef CAS PubMed;
(c) T. Tamura and I. Hamachi, Chemistry for Covalent Modification of Endogenous/Native Proteins: From Test Tubes to Complex Biological Systems, J. Am. Chem. Soc., 2019, 141, 2782 CrossRef CAS PubMed;
(d) A. Chaikuad, P. Koch, S. A. Laufer and S. Knapp, The Cysteinome of Protein Kinases as a Target in Drug Development, Angew. Chem., Int. Ed., 2018, 57, 4372 CrossRef CAS PubMed;
(e) N. Krall, F. P. da Cruz, O. Boutureira and G. J. L. Bernardes, Site-selective protein-modification chemistry for basic biology and drug development, Nat. Chem., 2016, 8, 103 CrossRef CAS PubMed.
-
(a) E. A. Hoyt, P. M. S. D. Cal, B. L. Oliveira and G. J. L. Bernardes, Contemporary approaches to site-selective protein modification, Nat. Rev. Chem., 2019, 3, 147 CrossRef CAS;
(b) J. N. deGruyter, L. R. Malins and P. S. Baran, Residue-Specific Peptide Modification: A Chemist's Guide, Biochemistry, 2017, 56, 3863 CrossRef CAS PubMed;
(c) K. Yamada and Y. Ito, Recent Chemical Approaches for Site-Specific Conjugation of Native Antibodies: Technologies toward Next-Generation Antibody–Drug Conjugates, ChemBioChem, 2019, 20, 2729 CrossRef CAS PubMed;
(d) D. G. Rawale, K. Thakur, S. R. Adusumalli and V. Rai, Chemical Methods for Selective Labeling of Proteins, Eur. J. Org. Chem., 2019, 6749 CrossRef CAS;
(e) O. Koniev and A. Wagner, Developments and recent advancements in the field of endogenous amino acid selective bond forming reactions for bioconjugation, Chem. Soc. Rev., 2015, 44, 5495 RSC;
(f) C. S. McKay and M. G. Finn, Click Chemistry in Complex Mixtures: Bioorthogonal Bioconjugation, Chem. Biol., 2014, 21, 1075 CrossRef CAS PubMed;
(g) C. D. Spicer and B. G. Davis, Selective chemical protein modification, Nat. Commun., 2014, 5, 4740 CrossRef CAS PubMed;
(h) Y. Takaoka, A. Ojida and I. Hamachi, Protein Organic Chemistry and Applications for Labeling and Engineering in Live-Cell Systems, Angew. Chem., Int. Ed., 2013, 52, 4088 CrossRef CAS PubMed.
-
(a) P. Ochtrop and C. P. R. Hackenberger, Recent advances of thiol-selective bioconjugation reactions, Curr. Opin. Chem. Biol., 2020, 58, 28 CrossRef CAS PubMed;
(b) G. Hu, H. Jia, L. Zhao, D.-H. Cho and J. Fang, Small molecule fluorescent probes of protein vicinal dithiols, Chin. Chem. Lett., 2019, 30, 1704 CrossRef CAS;
(c) D. G. Hoch, D. Abegg and A. Adibekian, Cysteine-reactive probes and their use in chemical proteomics, Chem. Commun., 2018, 54, 4501 RSC;
(d) S. B. Gunnoo and A. Madder, Chemical Protein Modification through Cysteine, ChemBioChem, 2016, 17, 529 CrossRef CAS PubMed;
(e) J. M. Chalker, G. J. L. Bernardes, Y. A. Lin and B. G. Davis, Chemical Modification of Proteins at Cysteine: Opportunities in Chemistry and Biology, Chem. – Asian J., 2009, 4, 630 CrossRef CAS PubMed.
-
(a) D. Abegg, G. Gasparini, D. G. Hoch, A. Shuster, E. Bartolami, S. Matile and A. Adibekian, Strained Cyclic Disulfides Enable Cellular Uptake by Reacting with the Transferrin Receptor, J. Am. Chem. Soc., 2017, 139, 231 CrossRef CAS PubMed;
(b) S. I. van Kasteren, H. B. Kramer, H. H. Jensen, S. J. Campbell, J. Kirkpatrick, N. J. Oldham, D. C. Anthony and B. G. Davis, Expanding the diversity of chemical protein modification allows post-translational mimicry, Nature, 2007, 446, 1105 CrossRef CAS PubMed;
(c) D. P. Gamblin, P. Garnier, S. van Kasteren, N. J. Oldham, A. J. Fairbanks and B. G. Davis, Glyco-SeS: Selenenylsulfide-Mediated Protein Glycoconjugation—A New Strategy in Post-Translational
Modification, Angew. Chem., Int. Ed., 2004, 43, 828 CrossRef CAS PubMed;
(d) D. P. Gamblin, P. Garnier, S. J. Ward, N. J. Oldham, A. J. Fairbanks and B. G. Davis, Glycosyl phenylthiosulfonates (Glyco-PTS): novel reagents for glycoprotein synthesis, Org. Biomol. Chem., 2003, 1, 3642 RSC;
(e) B. G. Davis, M. A. T. Maughan, M. P. Green, A. Ullman and J. B. Jones, Glycomethanethiosulfonates: powerful reagents for protein glycosylation, Tetrahedron: Asymmetry, 2000, 11, 245 CrossRef CAS;
(f) P. Berglund, G. DeSantis, M. R. Stabile, X. Shang, M. Gold, R. R. Bott, T. P. Graycar, T. H. Lau, C. Mitchinson and J. B. Jones, Chemical Modification of Cysteine Mutants of Subtilisin Bacillus lentus Can Create Better Catalysts Than the Wild-Type Enzyme, J. Am. Chem. Soc., 1997, 119, 5265 CrossRef CAS.
-
(a) E. Calce and S. De Luca, The Cysteine S-Alkylation Reaction as a Synthetic Method to Covalently Modify Peptide Sequences, Chem. – Eur. J., 2017, 23, 224 CrossRef CAS PubMed;
(b) D. Wang, M. Yu, N. Liu, C. Lian, Z. Hou, R. Wang, R. Zhao, W. Li, Y. Jiang, X. Shi, S. Li, F. Yin and Z. Li, A sulfonium tethered peptide ligand rapidly and selectively modifies protein cysteine in vicinity, Chem. Sci., 2019, 10, 4966 RSC;
(c) C. Wang, D. Abegg, D. G. Hoch and A. Adibekian, Chemoproteomics-Enabled Discovery of a Potent and Selective Inhibitor of the DNA Repair Protein MGMT, Angew. Chem., Int. Ed., 2016, 55, 2911 CrossRef CAS PubMed;
(d) M. D. Simon, F. Chu, L. R. Racki, C. C. de la Cruz, A. L. Burlingame, B. Panning, G. J. Narlikar and K. M. Shokat, The site-specific installation of methyl-lysine analogs into recombinant histones, Cell, 2007, 128, 1003 CrossRef CAS PubMed.
-
(a) V. Laserna, D. Abegg, C. F. Afonso, E. M. Martin, A. Adibekian, P. Ravn, F. Corzana and G. J. L. Bernardes, Dichloro Butenediamides as Irreversible Site-Selective Protein Conjugation Reagent, Angew. Chem., Int. Ed., 2021, 60, 23750 CrossRef CAS PubMed;
(b) H. F. Motiwala, Y.-H. Kuo, B. L. Stinger, B. A. Palfey and B. R. Martin, Tunable Heteroaromatic Sulfones Enhance in-Cell Cysteine Profiling, J. Am. Chem. Soc., 2020, 142, 1801 CrossRef CAS PubMed;
(c) C. Zambaldo, E. V. Vinogradova, X. Qi, J. Iaconelli, R. M. Suciu, M. Koh, K. Senkane, S. R. Chadwick, B. B. Sanchez, J. S. Chen, A. K. Chatterjee, P. Liu, P. G. Schultz, B. F. Cravatt and M. J. Bollong, 2-Sulfonylpyridines as Tunable, Cysteine-Reactive Electrophiles, J. Am. Chem. Soc., 2020, 142, 8972 CrossRef CAS PubMed;
(d) C. Canovas, M. Moreau, C. Bernhard, A. Oudot, M. Guillemin, F. Denat and V. Goncalves, Site-Specific Dual Labeling of Proteins on Cysteine Residues with Chlorotetrazines, Angew. Chem., Int. Ed., 2018, 57, 10646 CrossRef CAS PubMed;
(e) A. M. Embaby, S. Schoffelen, C. Kofoed, M. Meldal and F. Diness, Rational Tuning of Fluorobenzene Probes for Cysteine-Selective Protein Modification, Angew. Chem., Int. Ed., 2018, 57, 8022 CrossRef CAS PubMed;
(f) P. Dai, C. Zhang, M. Welborn, J. J. Shepherd, T. Zhu, T. Van Voorhis and B. L. Pentelute, Salt Effect Accelerates Site-Selective Cysteine Bioconjugation, ACS Cent. Sci., 2016, 2, 637 CrossRef CAS PubMed;
(g) C. Zhang, M. Welborn, T. Zhu, N. J. Yang, M. S. Santos, T. Van Voorhis and B. L. Pentelute, π-Clamp-mediated cysteine conjugation, Nat. Chem., 2016, 8, 120 CrossRef CAS PubMed;
(h) S. P. Brown and A. B. Smith, Peptide/Protein Stapling and Unstapling: Introduction of s-Tetrazine, Photochemical Release, and Regeneration of the Peptide/Protein, J. Am. Chem. Soc., 2015, 137, 4034 CrossRef CAS PubMed;
(i) F. F. Schumacher, J. P. M. Nunes, A. Maruani, V. Chudasama, M. E. B. Smith, K. A. Chester, J. R. Baker and S. Caddick, Next generation maleimides enable the controlled assembly of antibody–drug conjugates via native disulfide bond bridging, Org. Biomol. Chem., 2014, 12, 7261 RSC;
(j) D. A. Shannon, R. Banerjee, E. R. Webster, D. W. Bak, C. Wang and E. Weerapana, Investigating the Proteome Reactivity and Selectivity of Aryl Halides, J. Am. Chem. Soc., 2014, 136, 3330 CrossRef CAS PubMed;
(k) N. Toda, S. Asano and C. F. Barbas Iii, Rapid, Stable, Chemoselective Labeling of Thiols with Julia–Kocieński-like Reagents: A Serum-Stable Alternative to Maleimide-Based Protein Conjugation, Angew. Chem., Int. Ed., 2013, 52, 12592 CrossRef CAS PubMed;
(l) C. Zhang, A. M. Spokoyny, Y. Zou, M. D. Simon and B. L. Pentelute, Enzymatic “Click” Ligation: Selective Cysteine Modification in Polypeptides Enabled by Promiscuous Glutathione S-Transferase, Angew. Chem., Int. Ed., 2013, 52, 14001 CrossRef CAS PubMed;
(m) A. M. Spokoyny, Y. Zou, J. J. Ling, H. Yu, Y.-S. Lin and B. L. Pentelute, A Perfluoroaryl-Cysteine SNAr Chemistry Approach to Unprotected Peptide Stapling, J. Am. Chem. Soc., 2013, 135, 5946 CrossRef CAS PubMed;
(n) D. Zhang, N. O. Devarie-Baez, Q. Li, J. R. Lancaster and M. Xian, Methylsulfonyl Benzothiazole (MSBT): A Selective Protein Thiol Blocking Reagent, Org. Lett., 2012, 14, 3396 CrossRef CAS PubMed;
(o) M. E. B. Smith, F. F. Schumacher, C. P. Ryan, L. M. Tedaldi, D. Papaioannou, G. Waksman, S. Caddick and J. R. Baker, Protein Modification, Bioconjugation, and Disulfide Bridging Using Bromomaleimides, J. Am. Chem. Soc., 2010, 132, 1960 CrossRef CAS PubMed.
-
(a) L. Xu, M. J. S. A. Silva, P. M. P. Gois, S. L. Kuan and T. Weil, Chemoselective cysteine or disulfide modification via single atom substitution in chloromethyl acryl reagents, Chem. Sci., 2021, 12, 13321 RSC;
(b) R. N. Reddi, A. Rogel, E. Resnick, R. Gabizon, P. K. Prasad, N. Gurwicz, H. Barr, Z. Shulman and N. London, Site-Specific Labeling of Endogenous Proteins Using CoLDR Chemistry, J. Am. Chem. Soc., 2021, 143, 20095 CrossRef CAS PubMed;
(c) C. E. Stieger, L. Franz, F. Körlin and C. P. R. Hackenberger, Diethynyl Phosphinates for Cysteine-Selective Protein Labeling and Disulfide Rebridging, Angew. Chem., Int. Ed., 2021, 60, 15359 CrossRef CAS PubMed;
(d) B. Bernardim, M. J. Matos, X. Ferhati, I. Compañón, A. Guerreiro, P. Akkapeddi, A. C. B. Burtoloso, G. Jiménez-Osés, F. Corzana and G. J. L. Bernardes, Efficient and irreversible antibody–cysteine bioconjugation using carbonylacrylic reagents, Nat. Protoc., 2019, 14, 86 CrossRef CAS PubMed;
(e) M. J. Matos, C. D. Navo, T. Hakala, X. Ferhati, A. Guerreiro, D. Hartmann, B. Bernardim, K. L. Saar, I. Compañón, F. Corzana, T. P. J. Knowles, G. Jiménez-Osés and G. J. L. Bernardes, Quaternization of Vinyl/Alkynyl Pyridine Enables Ultrafast Cysteine-Selective Protein Modification and Charge Modulation, Angew. Chem., Int. Ed., 2019, 58, 6640 CrossRef CAS PubMed;
(f) M.-A. Kasper, M. Glanz, A. Stengl, M. Penkert, S. Klenk, T. Sauer, D. Schumacher, J. Helma, E. Krause, M. C. Cardoso, H. Leonhardt and C. P. R. Hackenberger, Cysteine-Selective Phosphonamidate Electrophiles for Modular Protein Bioconjugations, Angew. Chem., Int. Ed., 2019, 58, 11625 CrossRef CAS PubMed;
(g) M.-A. Kasper, M. Glanz, A. Oder, P. Schmieder, J. P. von Kries and C. P. R. Hackenberger, Vinylphosphonites for Staudinger-induced chemoselective peptide cyclization and functionalization, Chem. Sci., 2019, 10, 6322 RSC;
(h) N. J. Smith, K. Rohlfing, L. A. Sawicki, P. M. Kharkar, S. J. Boyd, A. M. Kloxin and J. M. Fox, Fast, irreversible modification of cysteines through strain releasing conjugate additions of cyclopropenyl ketones, Org. Biomol. Chem., 2018, 16, 2164 RSC;
(i) R. Huang, Z. Li, Y. Sheng, J. Yu, Y. Wu, Y. Zhan, H. Chen and B. Jiang, N-Methyl-N-phenylvinylsulfonamides for Cysteine-Selective Conjugation, Org. Lett., 2018, 20, 6526 CrossRef CAS PubMed;
(j) B. Bernardim, P. M. S. D. Cal, M. J. Matos, B. L. Oliveira, N. Martínez-Sáez, I. S. Albuquerque, E. Perkins, F. Corzana, A. C. B. Burtoloso, G. Jiménez-Osés and G. J. L. Bernardes, Stoichiometric and irreversible cysteine-selective protein modification using carbonylacrylic reagents, Nat. Commun., 2016, 7, 13128 CrossRef CAS PubMed;
(k) D. Kalia, P. V. Malekar and M. Parthasarathy, Exocyclic Olefinic Maleimides: Synthesis and Application for Stable and Thiol-Selective Bioconjugation, Angew. Chem., Int. Ed., 2016, 55, 1432 CrossRef CAS PubMed;
(l) J. Sun, L. Zhang, X. Zhang, Y. Hu, C. Ge and J. Fang, An ultrafast turn-on thiol probe for protein labeling and bioimaging, Analyst, 2016, 141, 2009 RSC;
(m) P. Zhou, J. Yao, G. Hu and J. Fang, Naphthalimide Scaffold Provides Versatile Platform for Selective Thiol Sensing and Protein Labeling, ACS Chem. Biol., 2016, 11, 1098 CrossRef CAS PubMed.
-
(a) K. Kubota, P. Dai, B. L. Pentelute and S. L. Buchwald, Palladium Oxidative Addition Complexes for Peptide and Protein Cross-linking, J. Am. Chem. Soc., 2018, 140, 3128 CrossRef CAS PubMed;
(b) M. S. Messina, J. M. Stauber, M. A. Waddington, A. L. Rheingold, H. D. Maynard and A. M. Spokoyny, Organometallic Gold(III) Reagents for Cysteine Arylation, J. Am. Chem. Soc., 2018, 140, 7065 CrossRef CAS PubMed;
(c) A. J. Rojas, C. Zhang, E. V. Vinogradova, N. H. Buchwald, J. Reilly, B. L. Pentelute and S. L. Buchwald, Divergent unprotected peptide macrocyclisation by palladium-mediated cysteine arylation, Chem. Sci., 2017, 8, 4257 RSC;
(d) E. V. Vinogradova, C. Zhang, A. M. Spokoyny, B. L. Pentelute and S. L. Buchwald, Organometallic palladium reagents for cysteine bioconjugation, Nature, 2015, 526, 687 CrossRef CAS PubMed;
(e) K. K.-Y. Kung, H.-M. Ko, J.-F. Cui, H.-C. Chong, Y.-C. Leung and M.-K. Wong, Cyclometalated gold(III) complexes for chemoselective cysteine modification via ligand controlled C–S bond-forming reductive elimination, Chem. Commun., 2014, 50, 11899 RSC;
(f) A. On-Yee Chan, J. Lui-Lui Tsai, V. Kar-Yan Lo, G.-L. Li, M.-K. Wong and C.-M. Che, Gold-mediated selective cysteine modification of peptides using allenes, Chem. Commun., 2013, 49, 1428 RSC;
(g) T. Schlatzer, J. Kriegesmann, H. Schröder, M. Trobe, C. Lembacher-Fadum, S. Santner, A. V. Kravchuk, C. F. W. Becker and R. Breinbauer, Labeling and Natural Post-Translational Modification of Peptides and Proteins via Chemoselective Pd-Catalyzed Prenylation of Cysteine, J. Am. Chem. Soc., 2019, 141, 14931 CrossRef CAS PubMed;
(h) M. A. Waddington, X. Zheng, J. M. Stauber, E. Hakim Moully, H. R. Montgomery, L. M. A. Saleh, P. Král and A. M. Spokoyny, An Organometallic Strategy for Cysteine Borylation, J. Am. Chem. Soc., 2021, 143, 8661 CrossRef CAS PubMed.
-
(a) E. M. D. Allouche, E. Grinhagena and J. Waser, Hypervalent iodine-mediated late-stage peptide and protein functionalization, Angew. Chem., 2022, 61, e202112287 CAS;
(b) D. Abegg, M. Tomanik, N. Qiu, D. Pechalrieu, A. Shuster, B. Commare, A. Togni, S. B. Herzon and A. Adibekian, Chemoproteomic Profiling by Cysteine Fluoroalkylation Reveals Myrocin G as an Inhibitor of the Nonhomologous End Joining DNA Repair Pathway, J. Am. Chem. Soc., 2021, 143, 20332 CrossRef CAS PubMed;
(c) R. Tessier, J. Ceballos, N. Guidotti, R. Simonet-Davin, B. Fierz and J. Waser, “Doubly Orthogonal” Labeling of Peptides and Proteins, Chem, 2019, 5, 2243 CrossRef CAS;
(d) J. Václavík, R. Zschoche, I. Klimánková, V. Matoušek, P. Beier, D. Hilvert and A. Togni, Irreversible Cysteine-Selective Protein Labeling Employing Modular Electrophilic Tetrafluoroethylation Reagents, Chem. – Eur. J., 2017, 23, 6490 CrossRef PubMed;
(e) D. Abegg, R. Frei, L. Cerato, D. Prasad Hari, C. Wang, J. Waser and A. Adibekian, Proteome-Wide Profiling of Targets of Cysteine reactive Small Molecules by Using Ethynyl Benziodoxolone Reagents, Angew. Chem., Int. Ed., 2015, 54, 10852 CrossRef CAS PubMed;
(f) S. Capone, I. Kieltsch, O. Flögel, G. Lelais, A. Togni and D. Seebach, Electrophilic S-Trifluoromethylation of Cysteine Side Chains in α- and β-Peptides: Isolation of Trifluoro-methylated Sandostatin® (Octreotide) Derivatives, Helv. Chim. Acta, 2008, 91, 2035 CrossRef CAS;
(g) V. Laserna, A. Istrate, K. Kafuta, T. A. Hakala, T. P. J. Knowles, M. Alcarazo and G. J. L. Bernardes, Protein Conjugation by Electrophilic Alkynylation Using 5-(Alkynyl)dibenzothiophenium Triflates, Bioconjugate Chem., 2021, 32, 1570 CrossRef CAS PubMed.
-
(a) N. Griebenow, A. M. Dilmaç, S. Greven and S. Bräse, Site-Specific Conjugation of Peptides and Proteins via Rebridging of Disulfide Bonds Using the Thiol–Yne Coupling Reaction, Bioconjugate Chem., 2016, 27, 911 CrossRef CAS PubMed;
(b) Y. Wang and D. H.-C. Chou, A Thiol–Ene Coupling Approach to Native Peptide Stapling and Macrocyclization, Angew. Chem., Int. Ed., 2015, 54, 10931 CrossRef CAS PubMed;
(c) Y. Li, M. Pan, Y. Li, Y. Huang and Q. Guo, Thiol–yne radical reaction mediated site-specific protein labeling via genetic incorporation of an alkynyl-l-lysine analogue, Org. Biomol. Chem., 2013, 11, 2624 RSC;
(d) M. L. Conte, S. Staderini, A. Marra, M. Sanchez-Navarro, B. G. Davis and A. Dondoni, Multi-molecule reaction of serum albumin can occur through thiol-yne coupling, Chem. Commun., 2011, 47, 11086 RSC;
(e) A. Dondoni, A. Massi, P. Nanni and A. Roda, A New Ligation Strategy for Peptide and Protein Glycosylation: Photoinduced Thiol–Ene Coupling, Chem. – Eur. J., 2009, 15, 11444 CrossRef CAS PubMed;
(f) S. Wittrock, T. Becker and H. Kunz, Synthetic Vaccines of Tumor-Associated Glycopeptide Antigens by Immune-Compatible Thioether Linkage to Bovine Serum Albumin, Angew. Chem., Int. Ed., 2007, 46, 5226 CrossRef CAS PubMed;
(g) C. Zhang, P. Dai, A. A. Vinogradov, Z. P. Gates and B. L. Pentelute, Site-Selective Cysteine–Cyclooctyne Conjugation, Angew. Chem., Int. Ed., 2018, 57, 6459 CrossRef CAS PubMed;
(h) R. van Geel, G. J. M. Pruijn, F. L. van Delft and W. C. Boelens, Preventing Thiol-Yne Addition Improves the Specificity of Strain-Promoted Azide–Alkyne Cycloaddition, Bioconjugate Chem., 2012, 23, 392 CrossRef CAS PubMed;
(i) E. Mons, I. D. C. Jansen, J. Loboda, B. R. van Doodewaerd, J. Hermans, M. Verdoes, C. A. A. van Boeckel, P. A. van Veelen, B. Turk, D. Turk and H. Ovaa, The Alkyne Moiety as a Latent Electrophile in Irreversible Covalent Small Molecule Inhibitors of Cathepsin K, J. Am. Chem. Soc., 2019, 141, 3507 CrossRef CAS PubMed;
(j) S. Sommer, N. D. Weikart, U. Linne and H. D. Mootz, Covalent inhibition of SUMO and ubiquitin-specific cysteine proteases by an in situ thiol–alkyne addition, Bioorg. Med. Chem., 2013, 21, 2511 CrossRef CAS PubMed;
(k) R. Ekkebus, S. I. van Kasteren, Y. Kulathu, A. Scholten, I. Berlin, P. P. Geurink, A. de Jong, S. Goerdayal, J. Neefjes, A. J. R. Heck, D. Komander and H. Ovaa, On Terminal Alkynes That Can React with Active-Site Cysteine Nucleophiles in Proteases, J. Am. Chem. Soc., 2013, 135, 2867 CrossRef CAS PubMed.
-
(a) X. Zheng, Z. Li, W. Gao, X. Meng, X. Li, L. Y. P. Luk, Y. Zhao, Y.-H. Tsai and C. Wu, Condensation of 2-((Alkylthio)(aryl)methylene)malononitrile with 1,2-Aminothiol as a Novel Bioorthogonal Reaction for Site-Specific Protein Modification and Peptide Cyclization, J. Am. Chem. Soc., 2020, 142, 5097 CrossRef CAS PubMed;
(b) S. J. Mountford, B. M. Anderson, B. Xu, E. S. V. Tay, M. Szabo, M.-L. Hoang, J. Diao, L. Aurelio, R. I. Campden, E. Lindström, E. K. Sloan, R. M. Yates, N. W. Bunnett, P. E. Thompson and L. E. Edgington-Mitchell, Application of a Sulfoxonium Ylide Electrophile to Generate Cathepsin X-Selective Activity-Based Probes, ACS Chem. Biol., 2020, 15, 718 CrossRef CAS PubMed;
(c) J.-R. Deng, S.-F. Chung, A. S.-L. Leung, W.-M. Yip, B. Yang, M.-C. Choi, J.-F. Cui, K. K.-Y. Kung, Z. Zhang, K.-W. Lo, Y.-C. Leung and M.-K. Wong, Chemoselective and photocleavable cysteine modification of peptides and proteins using isoxazoliniums, Commun. Chem., 2019, 2, 93 CrossRef;
(d) J. K. Eaton, R. A. Ruberto, A. Kramm, V. S. Viswanathan and S. L. Schreiber, Diacylfuroxans Are Masked Nitrile Oxides That Inhibit GPX4 Covalently, J. Am. Chem. Soc., 2019, 141, 20407 CrossRef CAS PubMed;
(e) D. Crich, V. Krishnamurthy, F. Brebion, M. Karatholuvhu, V. Subramanian and T. K. Hutton, Dechalcogenative Allylic Selenosulfide and Disulfide Rearrangements: Complementary Methods for the Formation of Allylic Sulfides in the Absence of Electrophiles. Scope, Limitations, and Application to the Functionalization of Unprotected Peptides in Aqueous Media, J. Am. Chem. Soc., 2007, 129, 10282 CrossRef CAS PubMed;
(f) D. Crich, V. Krishnamurthy and T. K. Hutton, Allylic Selenosulfide Rearrangement: A Method for Chemical Ligation to Cysteine and Other Thiols, J. Am. Chem. Soc., 2006, 128, 2544 CrossRef CAS PubMed;
(g) D. Crich, F. Brebion and V. Krishnamurthy, Allylic Disulfide Rearrangement and Desulfurization: Mild, Electrophile-Free Thioether Formation from Thiols, Org. Lett., 2006, 8, 3593 CrossRef CAS PubMed.
- P. C. Montevecchi, M. L. Navacchia and P. Spagnolo, Generation of Iminyl Radicals through Sulfanyl Radical Addition to Vinyl Azides, J. Org. Chem., 1997, 62, 5846 CrossRef CAS.
- Y. Wang, Y.-J. Wang, X.-C. Liang, M.-H. Shen, H.-D. Xu and D. Xu, An aryl thiol-vinyl azide coupling reaction and a thiol-vinyl azide coupling/cyclization cascade: efficient synthesis of β-ketosulfides and arene-fused 5-methylene-2-pyrrolidinone derivatives, Org. Biomol. Chem., 2021, 19, 5169 RSC.
- S. Ariyasu, H. Hayashi, B. Xing and S. Chiba, Site-Specific Dual Functionalization of Cysteine Residue in Peptides and Proteins with 2-Azidoacrylates, Bioconjugate Chem., 2017, 28, 897 CrossRef CAS PubMed.
-
(a) M. Ouyang, S. Lu and Y. Wang, Genetically Encoded Fluorescent Biosensors for Live-Cell Imaging of MT1-MMP Protease Activity, Methods Mol. Biol., 2014, , 1071, 163 CrossRef PubMed;
(b) M. Ouyang, S. Lu, X.-Y. Li, J. Xu, J. Seong, B. N. G. Giepmans, J. Y. J. Shyy, S. J. Weiss and Y. Wang, Visualization of Polarized Membrane Type 1 Matrix Metalloproteinase Activity in Live Cells by Fluorescence Resonance Energy Transfer Imaging, J. Biol. Chem., 2008, 283, 17740 CrossRef CAS PubMed.
- C. E. Hoyle and C. N. Bowman, Thiol–Ene Click Chemistry, Angew. Chem., Int. Ed., 2010, 49, 1540 CrossRef CAS PubMed.
Footnote |
† Electronic supplementary information (ESI) available: Experiment details, characterization of compounds, copies of compound NMR spectra. See DOI: https://doi.org/10.1039/d2qo00736c |
|
This journal is © the Partner Organisations 2022 |