DOI:
10.1039/D1NR06939J
(Review Article)
Nanoscale, 2022,
14, 3698-3719
Overcoming barriers in non-viral gene delivery for neurological applications
Received
20th October 2021
, Accepted 15th February 2022
First published on 20th February 2022
Abstract
Gene therapy for neurological disorders has attracted significant interest as a way to reverse or stop various disease pathologies. Typical gene therapies involving the central and peripheral nervous system make use of adeno-associated viral vectors whose questionable safety and limitations in manufacturing has given rise to extensive research into non-viral vectors. While early research studies have demonstrated limited efficacy with these non-viral vectors, investigation into various vector materials and functionalization methods has provided insight into ways to optimize these non-viral vectors to improve desired characteristics such as improved blood–brain barrier transcytosis, improved perfusion in brain region, enhanced cellular uptake and endosomal escape in neural cells, and nuclear transport of genetic material post- intracellular delivery. Using a combination of various strategies to enhance non-viral vectors, research groups have designed multi-functional vectors that have been successfully used in a variety of pre-clinical applications for the treatment of Parkinson's disease, brain cancers, and cellular reprogramming for neuron replacement. While more work is needed in the design of these multi-functional non-viral vectors for neural applications, much of the groundwork has been done and is reviewed here.
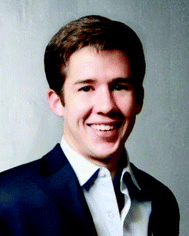 Aaron Tasset | Aaron Tasset received his B.Science degree in Biomedical Engineering with a focus on Cell and Tissue Engineering from the University of Miami (Coral Gables, FL) in 2015. He worked for 6 years at Biopact Ventures, an Austin based biotech start-up, as a R&D Associate and Scientist. Here he worked on the development of a carbon-nanotube based drug and gene delivery system. He is now a predoctoral candidate at the University of Texas working in Dr Evan (Huiliang) Wang's lab with an emphasis on the development of gene delivery systems for opsin protein expression in neuronal cells for optogenetic clinical applications. |
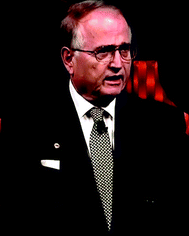 Nicholas Peppas | Nicholas Peppas, is a professor in Biomedical Engineering, Chemical Engineering, Pediatrics, Surgery and Pharmacy at the University of Texas at Austin, researching biomaterials, drug delivery, and bionanotechnology. AAPS Global Leader and AIChE, SFB, CRS, ASEE Founders Awards. Member NAE (Founders Award), (NAM Yarmolinsky Award), American Academy of Arts and Sciences, National Academy of Inventors, Academia Europaea, Canadian Academy of Engineering, Indian National Academy of Engineering, Chinese Academy of Engineering, Korean Academy of Science and Technology, National Academy of France, Royal Academy of Spain, Academy of Athens, Greece. 12 honorary doctorates and professorships. Published 1600 publications, 170 000 citations (H = 192). |
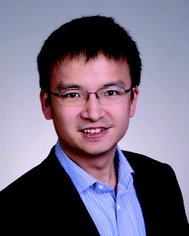 Huiliang Wang | Huiliang (Evan) Wang is an Assistant professor at University of Texas at Austin (UT Austin). Prior to joining UT Austin, he was a postdoc with Karl Deisseroth at Stanford Bioengineering, developing nanomaterial-based technologies for targeted neural modulation. Before then, he had his PhD degree from Stanford Materials Science and Engineering and worked on nanomaterials and polymers for flexible/wearable electronics with Zhenan Bao. He has received several awards and fellowships including the Materials Research Society (MRS) Gold Graduate Student Award, NIH F32 NRSA Postdoctoral Fellowship, NIH K01 Mentored Research Scientist Development Award and MIT Technology Review 35 under 35 (China). |
I. Introduction
1. Overview
Gene therapy is a rapidly growing market across the world with over 700 gene therapy clinical trials ongoing as of 2019, seeking to solve a variety of diseases and disorders, such as Parkinson's Disease,1,2 Cystic Fibrosis3,4 and various types of cancer5,6 at the genomic level. Current clinical applications in gene therapy make use of adeno-associated viral vectors (AAVs) due to their unmatched gene delivery efficiency but limitations in max gene size, disputed safety, high cost of production and lack of ease in scalability has drawn the desire for an alternative for gene delivery applications.7,8 Significant attention in research has been given to non-viral vectors with the hope of developing a system that matches the gene delivery ability without these constraints, but the majority have fallen short due to the numerous biological hurdles needed to overcome. Research in non-viral vectors for gene delivery covers a wide breadth of various nanoparticles including cationic polymers, cationic liposomes, carbon nanotubes (CNTs), and inorganics.8–10 Within each of these broad categories there is a never-ending list of materials, combinations, synthesis routes or functionalization methods that give rise to an infinite pool of non-viral vectors with their own distinct characteristics, specifically designed to overcome specific biological barriers needed for gene delivery in desired applications.
In non-viral vector design, there is a delicate balancing act of interconnected properties that govern the key areas of efficiency, cytotoxicity, cellular uptake, endosomal escape/avoidance, DNA loading, release of DNA, biodistribution and clearance, and ease of production/functionalization that has made the search for an optimal vector a difficult one. A nanoparticle might exhibit a high transfection rate but is limited by its cytotoxicity and a change that reduces cytotoxicity might inhibit its transfection efficiency or changes to promote improved blood-half life and biocompatibility might inhibit efficient loading of the genetic material. It is a delicate dance that has demonstrated the need for more research in order to accomplish the goal of an alternative to AAVs for neuron specific applications. In this review we identify the numerous barriers in non-viral gene delivery for nervous system applications and investigate the current methods researchers are investigating to overcome these hurdles. We will also look at preclinical application of multifunctional designed non-viral in a variety of models to highlight the advancement and potential of non-viral vectors to be used in gene therapy.
2. Short-comings of viral vectors
When it comes to viral vectors, their ability to deliver genes is unmatched by nonviral vectors but do suffer from numerous drawbacks that could limit their widespread adoption in gene delivery in the central and peripheral nervous system. While gene therapies utilizing AAVs have demonstrated safety and long-term efficacy in clinical trials for various applications, evidence has emerged that these vectors can lead to both an innate and humoral response that can lead to limited transgene expression and can lead to significant inflammation.11,12 More specifically, a number of groups have identified significant toxicity and limitations for repeat dosing with AAVs following intracranial injections intended for central nervous system gene delivery.11–14 One such group identified significant inflammation and expression of Class I multiple histocompatibility antigens with the persistent state of AAV vector remaining a potential target for destructive immune response that can lead to local demyelination of surrounding neurons.14 Another group identified chronic brain inflammation 3 months after treatment with cytotoxic gene therapy for the treatment of gliomas with significant presence of macrophages/microglia, astrocytes and T lymphocytes cells throughout the brain leading to widespread secondary demyelination.13 Lastly, one group demonstrated that dosing with recombinant adeno-associated virus vector (rAAV2) of rats that were pre-immunized with repeat dosing of rAAV2, demonstrated high levels of neutralizing antibodies that prevented viral transduction in the brain, resulting in limited gene expression in the central nervous system region, highlighting potential limitations in repeat dosing using similar viral serotypes.12
In order to efficiently deliver genes, viral particles must be able to reach target cells in the nervous system while also limiting off-target effects. A number of barriers exist for viral particles to reach target cells including the blood–brain barrier (BBB),15 diffusion through the extracellular matrix (ECM) of brain tissue,16 and availability of viral receptors on target cells.17 Additionally, the liver and spleen have been shown to effectively filter out systemically injected viral particles before reaching the brain and the heart and muscles have been shown to be non-target organs that can be transduced, leading to potential toxicity.18
Lastly, viral particles suffer from a number of manufacturing and scalability problems that they have sought to overcome in the past 20 years including limitations in the maximum size of genes able to be carried by AAVs to 4.7 kb for packing of foreign DNA,19 a high cost to manufacture drawing into question the economic scalability, and presence of cell impurities in all currently utilized vector production schemes.20
3. Current barriers for non-viral vectors
The delivery of genetic material to neurons using non-viral vectors requires a number of steps throughout the process to work effectively in order to achieve efficient gene delivery. Inhibition at early stages of the delivery process will have detrimental effects on the remaining down-stream events and limit the gene delivery ability. This means that while specific design strategies can be implemented to overcome individual biological hurdles (Fig. 1), special consideration must be made to ensure they do not negatively impact the non-viral vector's ability to overcome preceding hurdles and must take into consideration all the steps involved in the gene delivery process. In order to effectively develop and implement rationale-driven design strategies, the key biological hurdles experienced by non-viral vectors must first be identified and understood. Currently, the primary biological roadblocks identified consist of widespread delivery to the central and peripheral nervous system neurons, cellular uptake of the vector by the target cell, endosomal escape and nuclear entry following DNA release.10,21
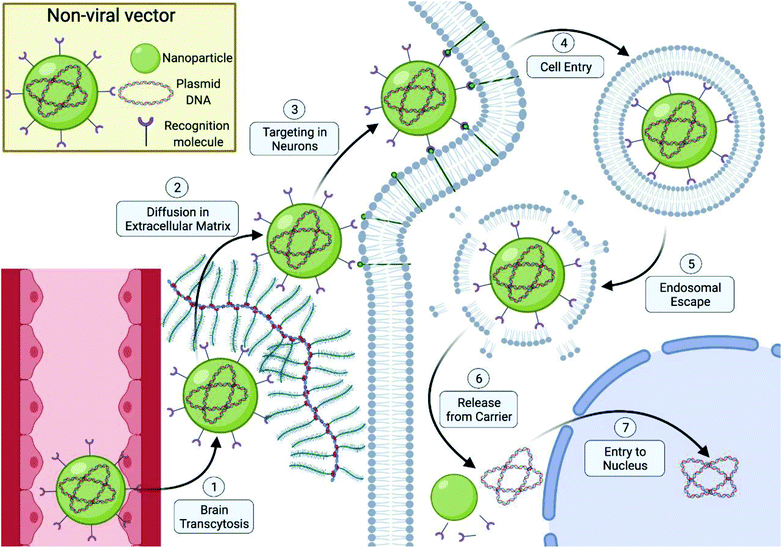 |
| Fig. 1 Extracellular and intracellular barriers for non-viral vectors in efficient DNA delivery for neurological applications, including, (1) crossing the blood–brain barrier, (2) diffusion through extracellular space and sequestration avoidance of non-viral vectors by the extra-cellular matrix components, (3) avoidance of uptake by immune cells, macrophages, and microglia and targeting of neuronal cell subtypes, (4) efficient cellular uptake by neuronal cells using active-transport mechanisms, (5) endosomal escape following endocytosis and avoidance of degradation by late-stage endosomes and lysosomes, (6) effective release of DNA from the carrier and (7) efficient transport and diffusion of DNA through cytoplasm and nuclear entry. Diagram created using Biorender.com. | |
In delivery to the central nervous system following systemic injections, the vectors have to be able to efficiently cross the blood–brain barrier whose purpose is to prevent unwanted molecules and materials from entering into the brain and spinal-cord space. While this barrier can be surpassed through the use of alternative invasive injection methodologies, an ideal non-viral vector would be injected systemically and possess the ability to transverse this biological barrier on its own.22 Once inside the brain region, the vector must be able to navigate the region of the brain to the desired targets while simultaneously avoiding uptake by surrounding glial cells and sequestration by the surrounding ECM. This surrounding ECM is rich in glycosaminoglycans, proteoglycans, glycoproteins, hyaluronan and chondroitin sulfate whose negative charges are capable of interacting with the cationic surface charges displayed on traditional cationic polyplexes and lipoplexes, resulting in sequestration of these particles before reaching the surface of the target cells.23–27 Not only do these functional structures seen in the ECM provide an electrostatic barrier, but also provide a steric barrier that must be overcome through the use of small, highly-condensed nanoparticles to overcome sequestration.27–29
Once the vector has reached the target cell of interest, it must be able to interact with the cellular surface or associated proteins to help facilitate cellular uptake either through basic electrostatic interactions or receptor-mediated interactions. A number of properties of the non-viral vector will ultimately dictate how it interacts with the cellular surface and by which process the vector is internalized.30 Once internalized by the cell, the nanoparticles are typically encapsulated inside an endosomal compartment, where efficient escape from these compartments into the cellular cytoplasm is crucial for high transgene expression. As the endosome matures, it becomes increasingly acidic and eventually fuses with vesicles containing hydrolytic enzymes to form lysosomes. Here vectors carrying genetic material that were unable to escape into the cytoplasm are enzymatically cleaved into smaller constituents for eventual expulsion from the cell as waste.30
Lastly, following endosomal escape, the vector carrying the genetic material or the genetic material itself must be able to diffuse or be actively transported through the cytoplasm of the cell to the nucleus and across the nuclear envelope in order to elicit a functional response. Research has shown that larger vector and genetic material constructs have slower diffusion properties and therefore must be aided by some form of active transport to reach the nucleus.31,32 Additionally, the pores of the nuclear envelope are less than 40 nm in diameter, thereby restricting the type and amount of genetic material that can enter the nucleus in non-dividing cells without the aid of nuclear transport proteins.31
II. Strategies investigated
1. Brain transcytosis
Expression of exogenous genes in the brain in vivo has been previously accomplished with both viral and non-viral vectors through the use of invasive intracranial administration of therapeutics. While this does allow circumvention of the highly selective brain capillary walls composing the blood–brain barrier, the possibility for repeat administration for therapeutic effects might limit the widespread utilization of these invasive routes of administration, highlighting the need for a vector capable of independent cranial entry through this barrier.
Arising from the highly selective permeability nature of the blood–brain barrier, substantial endogenous protein machinery exists that facilitate transport of needed molecules across this barrier into the brain. Researchers have sought to hijack the mechanisms of these transport systems to help facilitate transcytosis of non-viral vectors into the brain. One such group,22 identified the transferrin receptor (TfR) or insulin receptor as a viable candidate to target due to its previous success in shuttling of peptides or antisense agents across the BBB in vivo. Immunoliposomes were used to encapsulate either a pGL2 plasmid encoding for the firefly Photinus pyralis luciferase gene. Functionalization of the poly(ethylene glycol)-chains (PEG) of these immunoliposomes with an anti-rat transferrin receptor, OX26 monoclonal antibody (mAb), resulted in individual immunoliposomes with an average of 39 conjugated molecules of OX26 mAb. While an increase in off-target cellular uptake and faster blood clearance rate in rats was observed, a significant increase in brain uptake was also observed that resulted in uptake levels similar to those of neuroactive small molecules such as morphine. Transgene expression of firefly luciferase gene in the brain that peaked at 48 h was only observed with OX26 mAB functionalized immunoliposomes demonstrating the crucial role these targeting agents played in facilitating transcytosis and gene delivery for liposomal vector in the brain with i.v. administration. The effectiveness of this monoclonal antibody for promoting brain uptake of liposomal based nanoparticles was seen in a number of studies conducted by this group.33–35 Confirmation that this receptor can be exploited by a polymeric based system was observed by another group that functionalized polyamidoamine (PAMAM) with transferrin protein rather than a monoclonal antibody. They demonstrated similar effects with approximately 2-fold higher particle uptake and gene delivery in the brain over the naked vector.36 While the TfR facilitates central nervous system gene delivery following i.v. administration, substantial off-target transgene expression was observed in these studies with one study showing transgenic protein expression in liver hepatocytes that was 6-fold higher than the brain due to the abundance of TfR proteins on hepatocyte plasma membranes which may limit its adoption in non-viral vectors designed for clinical usage.22
Additional research into possible strategies to present in BBB endothelium cells that can facilitate transcytosis of large non-viral vectors has resulted in a number of other different possible targets. Due to its high degree of neurotropism in vivo and its ability to transverse the BBB, the rabies virus was investigated as a possible source of a targeting ligand.37 Phage display of the rabies viral glycoprotein (RVG) that functions to facilitate binding and entry of the rabies virus into neurons via nicotinic acetylcholine receptors, yielded a 29-amino acid peptide capable of selectively binding to the same receptors with high affinity. In vitro binding studies in multiple cell lines revealed selective peptide binding to Neuro2a and primary neurons demonstrating the potency of this targeting ligand to facilitate neuron specific delivery and transcytosis. Functionalization of this peptide with a nine D-arginine DNA binding moiety facilitated the binding of siRNA. In vitro assays looking at the silencing activity of green-fluorescent protein (GFP) of this novel vector carrying anti-GFP siRNA demonstrated the ability of this vector to enter Neuro2a cells, escape the endosome and release anti-GFP siRNA to elicit silencing activity that was comparable to Lipofectamine. Investigation in a functional in vivo test utilizing GFP-transgenic mice demonstrated the ability to silence GFP activity in the brain following i.v. administration. It is worth noting that unlike the use of transferrin ligands, there was no off-target silencing observed in the spleen and liver. Future work exploring the use of the RVG peptide demonstrated similar enhancement in BBB transcytosis effects in both polymeric and liposomal based systems. Functionalization of poly(mannitol-co-polyethyleneimine) vectors with this targeting ligand increased accumulation of vectors inside the brain by approximately 5.9-fold while also limiting systemic off-target effects.38 Liposomes functionalized with the same RVG moiety also demonstrated increased levels of vector delivery across the BBB over naked vectors with no off-target effects.39 The high cell-specificity of the RVG targeting ligand makes it an ideal candidate as it promotes transcytosis, enhanced cell-specific uptake and no off-target effects.
A number of other receptors and their ligand counterparts have been used successfully to improve polymeric, dendrimer and liposomal vector uptake across the blood–brain barrier. These include angiopep-2 (TFFYGGSRGKRNNFKTEEY) ligands to target low-density lipoprotein receptor-related proteins,40,41 leptin30 (30-amino-acid peptide derived from an endogenic hormone-leptin) to target leptin receptors on brain capillary endothelial cells,42 TGN peptide (TGNYKALHPHNG),43,44 and a peptide sequence isolated from the LIM Kinase 2 protein (LNP, KKRTLRKNDRKKRC).45
Alternatively, one nonviral method of gene therapy that has shown promise is the use of gold nanorods or gold nanoparticles (GNRs).46–48 These nanoparticles and nanorods have been garnering interest due to their biocompatibility, small size, and their surfaces that can be modified to incorporate cationic charges to facilitate a stable electrostatic complexation with the anionic genetic material for therapeutic purposes. In a Transwell BBB model, approximately 40% of the GNRs-siRNA had diffused through the BBB mimetic layer while native siRNAs was only capable of reaching 1% uptake in the opposite well highlighting the natural ability of GNRs to permeate through biological barriers without the need for specific ligands or functional groups.47 Further optimization and novel exploration of gold nanoparticles as a viable non-viral vector in central nervous system (CNS) applications yielded enhanced in vivo BBB and neuron targeting of gold nanoparticles through encapsulation by RVG-neuron targeted exosomes.48
While it may be difficult to design non-viral vectors intended for CNS application around i.v. administration due to the selective nature of the BBB barrier, other routes of administration that are less invasive relative to intracranial injections exist and have been utilized to deliver gene therapies to the brain. While utilizing these alternative routes allows circumvention of the BBB, they are not without their own biological barriers and design considerations. Intracerebroventricular injections that administer therapeutics directly into cerebral lateral ventricles allow for delivery of materials into the CNS through cerebrospinal fluid (CSF), bypassing the BBB. However, vectors must be able to be rapidly transported out the CSF across the ependymal cell border and capable of penetrating the brain parenchyma.49 PAMAM dendrimers functionalized with C-12 lipid chains and unfunctionalized amino PAMAM dendrimers were investigated as possible vectors that could be delivered through the CSF. While unmodified PAMAM dendrimers were capable of crossing the ependymal border and achieving widespread diffusion through the brain parenchyma, lipid functionalized dendrimers were found localized along the ependymal cell border with no presence in the brain parenchyma.50
While functionalization of non-viral vectors with various non-specific and specific BBB-targeting moetities has shown promising results in improving transcytosis, recent literature has demonstrated that adsorption of endogenous proteins (protein corona) can affect the effectiveness of these targeting moieties and the ability of non-viral vectors to undergo transcytosis.51–53 In two such studies, functionalization of non-viral vectors with PEG spaces and Transferrin ligands resulted in significantly higher cellular uptake in BBB related cells in the absence of serum but this effect was lost in the presence of serum. This demonstrated that the absorption of serum proteins and formation of a protein corona mitigated the ability for cellular receptors to recognize grafted targeting moieties in vitro. While loss of enhanced targeting was seen in vitro, similar experiments in vivo showed partial retention of targeting ability and improved brain transcytosis despite the protein corona.52,53 Another study further demonstrated the importance of the protein corona on BBB-transcytosis by tailoring the surface composition of their non-viral vector to promote adsorption and specific orientation of brain-targeting apolipoproteins (ApoE, ApoJ, ApoA1) for improved brain transcytosis.51 The importance and influence of protein absorption seen in both in vitro and in vivo work not only highlights the care needed when designing non-viral vectors but also in experimental design as results seen in vitro might not correlate to in vivo efficacy.
2. Diffusion in extracellular matrix
For many applications, non-viral vectors will require the ability to adequately diffuse throughout brain tissue following transcytosis or local injection to be considered an effective alternative to viral based methods. Research into vector diffusion throughout the brain parenchyma has revealed that size, surface charge and lipophilicity of the particle are influential characteristics as the ECM acts as both an adhesive and steric barrier.27,54
In one such experiment,55 the researchers attempted to synthesize a biodegradable polymer, poly(β-amino ester) (PBAE), that could penetrate the brain tissue (PBAE-BPN) through functionalization with PEG to limit ECM sequestration by neutralization of the cationic surface charge contributed by the PBAE polymer. PBAEs are a unique class of polymers utilized in gene delivery efforts because they possess multiple positively charged amine groups for complexation with genetic material, readily cleavable ester bonds within the backbone for enhanced degradation, pH buffering capacity, and minimal cytotoxicity.56 In a recent study, Green and associates57 explored the use of newly developed PBAEs with bio-reducible and non-reducible properties to deliver miRNA mimics to glioblastoma (GBM) tumors. The synthesized PBAE-BPN had a diameter roughly half that of non-PEGylated PBAE vectors and a near neutral zeta potential of +2.5 mV compared to the original of 35.3 mV. Ex vivo diffusion studies in rat brains demonstrated the effectiveness of designing smaller, neutrally charged vectors as the PEGylated PBAE vectors witnessed a 20-fold increase in diffusion rate and a 50-fold increase in average displacement of GFP transgene expression from the injection site. It should also be noted that even though the PEG barrier mitigated interaction with the ECM and membrane proteins, the barrier did not inhibit effective cellular internalization and gene delivery efficiency that has been observed in other works.58,59 While it is unclear whether the improvement in diffusion of these PBAE vectors in this study is attributed to the decrease in size or neutralization of surface charge by the dense PEG shield, other studies have illustrated the effectiveness of a dense PEG shield or anionic surface charge for non-viral vectors to promote diffusion in the brain.27,28,54 However, a similar experiment conducted using cationic PAMAM dendrimers demonstrated that the small size of the vector was sufficient in facilitating widespread diffusion despite the cationic nature of those vectors. When these dendrimers were functionalized with a hydrophobic C12 lipid, diffusion was completely stopped at the injection site as a result of stronger lipophilic interactions with the surrounding cell membranes and ECM.50
In addition to modification to non-viral vectors, other techniques like pressure-driven flow provided by convection enhanced delivery (CED) have been explored in conjunction with non-viral vectors to improve their diffusion in the brain.28,60–62 These methods may aid in more widespread and evenly distributed delivery across the brain, as well as improved permeation in glioblastomas During CED, fine intracranial catheters are implanted and deliver therapeutics along a pressure gradient from the catheter tip and extracellular space allowing for controlled and homogenous distribution throughout the injection area.28 Research in this area has highlighted the importance of specific vector properties, such as sub-100 nm size and neutral or anionic surface charge in order to adequately utilize CED as steric hindrance or electrostatic binding can't be overcome via CED. PEGylated PBAE vectors that previously demonstrated superior diffusion and widespread transgene expression in the brain without CED,55 demonstrated improvement in the total volume of vector diffusion and transgene expression distribution in the brain when delivered with CED.55 Anionic, non-PEGylated polymers also demonstrated the same benefit using CED over their cationic counterpart with widespread dispersion of particles and transgene expression.28 However, while the transgene expression level was more widespread from the injection site, there was no difference in the overall level of protein expression in the brain when compared to its cationic version. This phenomenon of increased distribution of gene expression but no increase in expression level in the brain was also seen in studies utilizing anionic and cationic liposomes of similar sizes for gene delivery in the brain with CED delivery. A 4-fold increase in distribution of anionic liposomes over the traditional cationic lipoplexes was witnessed but despite this increase in volume distribution by utilizing an anionic liposome vector, transgene expression throughout the brain was surprisingly lower compared to cationic liposomes.28 While improvements could be made in optimization in size and DNA condensing of anionic vectors, PEGylation of cationic particles for CED seem to have the added benefit of limited inhibition of cellular interaction and uptake that affords it both improved diffusion and transgene delivery.
3. Targeting in neurons
In the central and peripheral nervous system, a number of different cell types exist that can be divided into two broad categories: glial cells and neurons. Glial cells, which consist of astrocytes, oligodendrocytes and microglia cells, do not participate directly in electrical signaling but provide supportive functions that help define synaptic contacts and maintain the signaling abilities of neurons. Glial roles that are well-established include maintaining the ionic milieu of nerve cells, modulating the rate of nerve signal propagation, modulating synaptic action by controlling the uptake of neurotransmitters, providing a scaffold for some aspects of neural development, and aiding in (or preventing, in some instances) recovery from neural injury.63 Neuron cells, on the other hand, primary function is the conduction of electrical signals over long distances. These different cellular functions result in varied biophysical properties, like receptor protein expression, that researchers can seek to capitalize on for the goal of achieving cell-specific delivery to limit potential off-target effects. Strategies of specific targeting ligands to achieve cell-specific delivery will typically be accompanied by increased cellular uptake of vectors if the targeted receptor is involved in receptor-mediated transport functions.
Trisioaloganglioside (GT1b) receptors, expressed on the outer membrane, is one such receptor of interest for neural applications due to the fact that it exists almost exclusively in nerve cells so targeting of these proteins could improve cell-specific association/attachment of non-viral vectors that could promote cellular uptake of target cells while minimizing off-target effects. The effectiveness of targeting of this receptor is seen in the high neurotoxicity and potency of botulinum and tetanus clostridial neurotoxins that arises from their ability to selectively bind to peripheral neurons with a high affinity prior to cellular entry.64 From this knowledge a 12-amino acid peptide chain, Ten-eleven Translocation-1 (Tet1, HLNILSTLWKYR), was identified through phage display that demonstrated improved selective binding to several different neuronal cell lines including pheochromocytoma cells (PC12), primary motor neurons, and dorsal root ganglion cells (DRG), relative to control human embryonic kidney-293 (HEK293) cells.65 This amino acid sequence has been used to functionalize a number of different non-viral vectors to improve the specificity of the vectors to neuronal types.66–70 In one such in vitro study,70 Tet1 peptide was functionalized to 25k branched polyethyleneimine (PEI) and compared against unmodified PEI. In a cell-binding assay using neuron-like differentiated PC-12 cells, Tet1-PEI demonstrated superior interaction with the cell surface relative to unmodified PEI. The increase in cellular binding from the Tet1 was lost when assayed using non-neuronal 3T3 fibroblasts and the increase in cellular binding was successfully attributed to the receptor-mediated binding of the Tet1-PEI by a free-ligand competition assay. Arising from the interaction between the ligand and cell-surface receptor, transfection efficiency in PC-12 cells of the Tet1-PEI polyplexes carrying plasmids encoding for Firefly Luciferase was improved by 7-fold over unmodified PEI demonstrating the effectiveness of this strategy to improve gene delivery in neuron-like cells. Cellular uptake studies conducted in primary dorsal root ganglion (DRG) neurons co-cultured with primary astrocytes showed DRG neuron specific improvements in cellular binding with no cellular uptake seen in primary astrocytes, demonstrating the targeting and specificity of the Tet1 login to certain cells present in the nervous system.
In vivo work conducted using cationic 25k bPEI functionalized with PEG and the Tet1 neuron targeting agent demonstrated similar neuron targeting properties that were witnessed previously in vitro.44,67 These neuron-targeting polyplexes carrying plasmid encoding for a firefly luciferase reporter gene were injected into the lateral ventricle where they mediated increased luciferase expression levels in the surrounding brain tissue when compared with unmodified PEI-PEG polyplexes with 1.5 fold higher expression in the left hemisphere and a 2.5 fold increase in the right with transgene expression lasting at least 14 days. Immunofluorescent labeling of brain tissue slices revealed that Tet1-PEI-PEG polyplexes selectively transfected cells positive for nestin/sox2 and negative for glial fibrillary acidic protein (GFAP), indicative of neural progenitor cells (NPCs),71 while cells transfected with polyplexes without the Tet1 targeting ligand illustrated a diverse population consisting of NPCs, glial cells and immature astrocytes. Furthermore, the majority of cells transfected using the targeted polyplexes stained positive for the G1b receptor while minimal G1b expression was seen in cells transfected without the ligand highlighting the effectiveness of using this receptor to target NPCs through this receptor. While this work demonstrates the ability to target NPCs that have the ability to differentiate to mature neuron progeny, the lack of transfection witnessed in mature neurons demonstrates the need for synergistic methods to facilitate transgene expression in non-dividing cells.
In addition to the Trisioaloganglioside family of receptors, high affinity neurotensin (NT) receptors have been studied as a way to promote cell specific targeting to dopamine neurons of the nigrostriatal and mesolimbic system.28,72–74In vitro work consisting of poly-L-lysine (PLL) conjugated with neurotensin ligand demonstrated selective transfection only in NT receptors expressing cell lines. Expansion of this system to in vivo delivery of NT-PLL polyplexes to the substantia nigra region resulted in exclusive GFP expression in dopamine neurons for up to 15 days.75
Nerve growth factor (NGF) is a member of the neurotrophin family and uses Tropomyosin receptor kinase A (TrkA) and neurotrophin receptor P75 (P75NTR) as its receptors. The neurons that respond to NGF include sensory neurons in the dorsal root ganglia and trigeminal ganglion. NGF responsive neurons are those cholinergic neurons present in the basal forebrain, a potential location for gene therapy against Alzheimer's disease. Thus, the use of NGF receptors targeting gene vectors could enhance gene delivery for those neurons and help combat Alzheimer's disease. In this study,76 a recombinant polypeptide composed of NGF modified for DNA binding was synthesized and experimented in PC12 cells, a neuron-like cell line. Conjugation of the NGF targeting ligand (NL4) to low molecular PEI with a 10-lysine sequence resulted in a 6-fold increase in transfection rates in neuron-like PC12 cells when compared to the vector without the targeting moiety. The increase in efficiency was lost when tested with the NGF-receptor negative COS7 cell line illustrating the cell specific cellular targeting that can be achieved. The targeting effect of these receptors was seen in vivo when a P75NTR monoclonal antibody (MLR2) was conjugated to PEGylated polyethyleneimine (PEI-PEG12) to improve gene delivery to motor neurons using an intraperitoneal injection. Motor neurons located in the lumbar, thoracic and cervical spaces all demonstrated transgene expression which was not observed without the MLR2 targeting ligand highlighting the importance in gene delivery in neurons of the spinal cord.77
4. Cell entry
Cells have a number of mechanisms to move substances from the surrounding environment into their internal environment including simple diffusion, facilitated diffusion and active transport that depends on the physical and chemical properties of the molecule (Fig. 2).21,78 Due to the size of non-viral vectors, cells will use some form of endocytosis active transport that can be split into two groups: phagocytosis (macropinocytosis) and pinocytosis with the method of choice dependent on the size of the particle. Particles greater than 500 nm in size are typically phagocytosed by cells such as macrophages and dendritic cells while particles less than 500 nm will be endocytosed by cells using other various endocytic pathways.78 Pinocytosis can then be subdivided into 4 different mechanisms that depend on the molecular mechanism, with these four types of pinocytosis consisting of micropinocytosis, clathrin-dependent, caveolin-dependent and clathrin- and caveolin-independent endocytosis.78 Additionally, a non-endocytic pathway called transduction exists that involves the direct delivery of vectors into the cytoplasm of cells. While an attractive mechanism of cellular uptake due to the avoidance of transport vesicles, little is understood about the mechanisms relative to non-viral vectors. Different cellular uptake mechanisms mean different endocytic dynamics, intracellular trafficking, organelle association that will ultimately influence the fate and effectiveness of encapsulated vectors and genetic material cargo.21,79,80
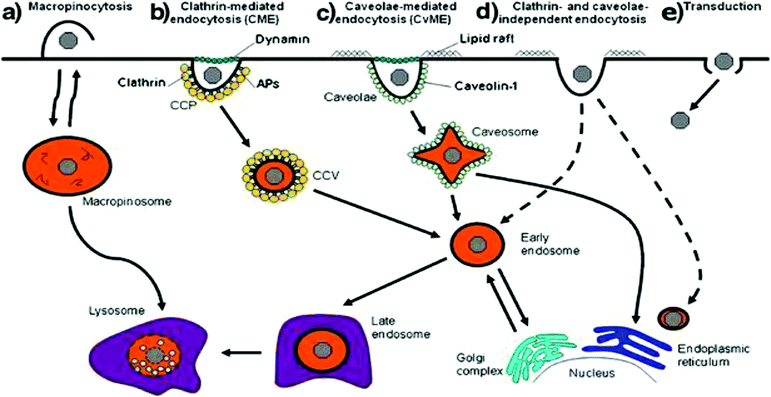 |
| Fig. 2 Scheme representing the different mechanisms of internalization, including (a) macropinocytosis, (b) clathrin-mediated endocytosis, (c) caveolae-mediated endocytosis, (d) clathrin and caveolae-independent endocytosis and (e) transduction. Dashed lines represent alternative pathways. Reproduced with permission from ref. 21. Copyright 2011 Springer. | |
The exact properties of non-viral vectors that govern the selection of endocytic pathway used is not well understood but research has shown that size, surface charge, presence of ligand receptors and choice of vector has some influence in addition to the cell type and cell membrane composition.46,78,81,82 Basic uptake studies utilizing various cationic and anionic surface coating on colloidal gold nanoparticles demonstrated improved cellular uptake in neural progenitor cells for cationic surface coating. The electrostatic interaction between cationic groups of vectors and the anionic moieties of cell-surface proteins and associated macromolecules results in non-specific cellular adhesion. This interaction can result in increased cellular uptake via clathrin-mediated endocytosis.46 A similar phenomenon was observed when studying the effect of chemical modification of PAMAM dendrimers (G4) on cellular internalization in hippocampal neurons shown in Fig. 3. Cationic PAMAM dendrimers that were unmodified were able to enter into neurons through classic clathrin-mediated endocytosis but when functionalized with PEG (PP50) or anionic acrylate groups (PAc), complete inhibition of cellular uptake was observed due to the neutralization of the cationic amino groups.58 While promotion of non-specific interaction between target cells and non-viral vectors by modifications of surface charge or non-specific ligands can improve cellular association, the subsequent cellular uptake in neural cells is lethargic due to the slow membrane recycling rate.83 Additionally, particles uptaken by the classical clathrin-mediated endocytic pathway will be subject to enzymatic degradation when the endosome fuses with enzymatic vesicles to form lysosomes. The slow uptake process of cationic vectors by neural cells and the fact that recent research has pointed to caveolin-dependent cellular uptake as a driving force in successful gene delivery due to the avoidance of lysosomes21,79 highlights the need for more targeted methods through the attachment of receptor ligands to the surface of non-viral vectors to improve cellular uptake and gene delivery in desired cells.
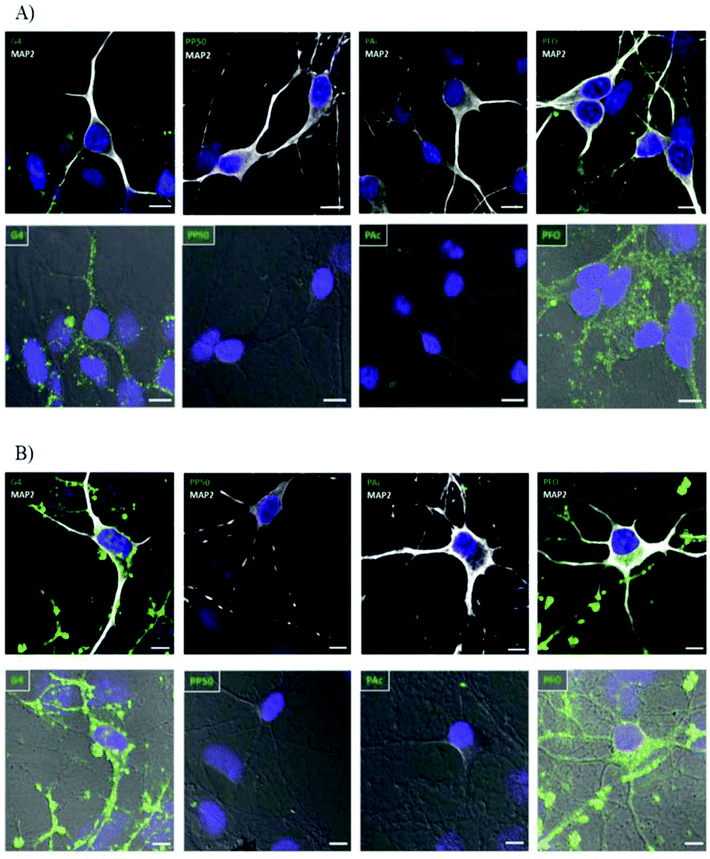 |
| Fig. 3 Internalization study of different dendrimers in hippocampal neurons over time demonstrating effect of surface charge on internalization of polyplexes. G4 dendrimers are unmodified cationic dendrimers, PP50 are dendrimers with 50% of functional groups replaced with polyethylene glycol, PAc are dendrimers modified with 2-carboxyethyl acrylate anionic groups and PFO are dendrimers modified with folate groups. Hippocampal neurons were incubated with different-conjugated dendrimers (green). MAP2white was used to mark neurons and DAPI (blue) for nucleus. (A) Neurons were incubated for 30 min with the indicated dendrimer (0.5 μM), washed, and processed for immunocytochemistry. Both normal confocal (top panel) and transmitted light (bottom panel) images are shown for a better visualization of the internalized molecules. (B) Neurons were incubated for a period of 6 h with the indicated dendrimers (0.5 μM) following the same protocol as in panel A. Scale bar = 10 μm. Reproduced with permission from ref. 58. Copyright 2016 American Chemistry Society. | |
Transferrin receptors are one such possible target for improving cellular uptake in target cells84 that was previously discussed in its role of promoting transcytosis across the BBB. In addition to being expressed in capillary endothelial cells in the BBB, this endocytosis-promoting receptor has also been identified in the plasma membranes of neuron cells in the brain and serves a similar function in the transport of iron-transferrin complexes into these cells.85–87 PEGylated immunoliposomes that were functionalized with transferrin mAb not only demonstrated improved transport of non-viral vectors across the BBB but also resulted in high levels of transgene expression of B-galactosidase in hippocampal neurons due to higher levels of receptor expression in these cells.22
While cell specific ligands can be used to promote cellular uptake in specific cell types, there are a number of targeting moieties or cell-penetrating peptides that lack specificity in targeting due to the presence of these receptors in multiple cells. Three such examples of non-specific targeting moieties that have been explored in their role in cellular uptake in neural gene delivery applications are TAT (GRKKRRQRRRPQ), RGD (GYGGRGDSP) and R8 (RRRRRRRR).75,88–90 Efficient internalization of some AAVs is mediated by the presence of five RGD motifs contained in the viral penton base that allow it to bind to integrins present on the surface of cells.88 while Tat targeting ligands derived from human immunodeficiency virus (HIV-1) vectors exhibit strong binding affinity to cell surface heparan sulfates and more specifically, glycosaminoglycan structures of the heparin sulfate found near cellular surfaces.91 By using these two ligands to functionalize PEI/DNA polyplexes, one group succeeded in improving the cellular uptake in differentiated neurons. Both TAT-PEI/DNA and RGD-PEI/DNA demonstrated enhanced cellular uptake and transfection efficiency relative to unmodified vectors with RGD functionalized polyplexes exhibiting slightly better uptake and transfection.92 Studies looking at the effect of the density of RGD moieties on a PEG based non-viral vector revealed that while increasing density of the ligand resulted in higher cellular association, it did not result in higher cellular uptake and transfection over the benefit seen in vectors modified with one ligand.93
While some of the aforementioned cellular uptake strategies are inherently non-specific, abnormal transcriptional or translational upregulation of targetable cell-surface receptors that occurs in many disease states can be exploited to promote preferential uptake in diseased cells. For example, while transferrin receptors are observed in multiple cell types in the brain, upregulation of TfR is observed in dopaminergic (DA) neurons in Parkinson's Disease, making them an attractive receptor to target.94 Non-viral vectors for gene therapies can be designed with this phenomena in mind to promote preferential uptake in diseased cells.95 One such study demonstrated this effect by designing a RGD-PEG-PEI vector to target the overexpression of a5b3 integrins observed in U87 glioblastoma cells. These RGD-PEG-PEI/DNA vectors demonstrated a 4-fold increase in tumor cell uptake relative to vectors without the RGD moiety.96
It is important to note that many vector design strategies that looked to utilize the aforementioned cellular uptake strategies and PEGylation to promote improved biocompatibility, blood circulation and prevent aggregation in ionic environments97 highlighted the importance of the positioning of the cell uptake moieties.59,98 Previous work in this dual-strategy that incorporated a PEG spacer to help protect bioactive molecules demonstrated lower cellular uptake and gene transfer due to the steric hindrance and covering of parts of the bioactive ligand arising from the PEG spacer.90 Further investigation of this work in optimizing positioning of the PEG shielding in relation to the targeting moiety, revealed that incorporation of an RGD ligand at the end of a PEG spacer rather than onto the base PEI polymer alongside PEG functional groups, enhanced cellular uptake in vitro. Using the same vector in vivo demonstrated no adverse effects to blood circulation time but had the added benefit of improved target uptake which led to improved gene transfer.96 Multi-functional design of nanoparticles intended for i.v. administration in vivo that will utilize PEG shielding to promote desired pharmacokinetics and targeting ligands for improved cell uptake must make note of the possible inhibitory interaction between the two.
5. Endosomal escape
Endosomal escape is one of the main barrier of non-viral gene delivery as failure to escape into the cytoplasm following cellular endocytosis results in the genetic material and vector being degraded in the acidic and enzymatic environment of maturing endosomes and lysosomes.21,79 Difficulty in overcoming this barrier is a multi-pronged problem stemming from the lack of mechanistic understanding of the different endocytic and endosomal pathways, how these endosomal pathways differ between cell types, how non-viral vectors and their properties influence these pathways, how different endosomal processes interact with each other and how different disease states alter endosomal pathways and mechanisms. The interconnected nature of these questions makes it impossible to generate a one-size-fits-all strategy to achieve efficient endosomal escape, highlighting the need for rationale-driven design built around the clinical application, cell targets and non-viral vector requirements. While a universal method might not exist, over the years there have been a few prevailing, but still debated,99 strategies that researchers have used during the design of the non-viral gene delivery vectors: proton-sponge effect to promote osmotic lysis and endosomolytic agents that disrupt the membrane or fusogenic agents that allow for vector fusion or pore formation (Fig. 4).30
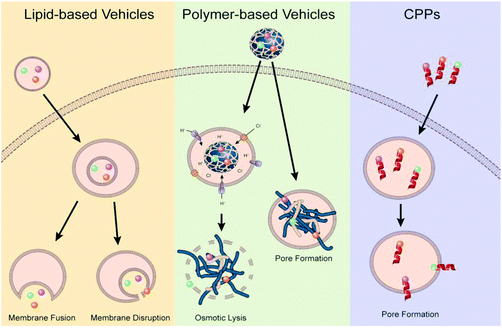 |
| Fig. 4 Illustration of proposed endosomal escape mechanisms based on vector type. Reproduced with permission from ref. 30. Copyright 2021 Elsevier. | |
Polycations, such as polyethyleneimine, have been hypothesized to escape from the endosomal and lysosomal pathways through a mechanism known as the proton-sponge effect. This hypothesis is based on the large buffering capacity at acidic pH values arising from the abundance of amino groups found in these polycations. Unprotonated amines of these polycations can absorb protons as they are pumped into maturing endosomes and lysosomes, resisting the drop in pH that normally occurs during this process. Resistance to the pH change associated with maturing endosome results in continued influx of protons into the endosome which will be accompanied by an increase in Cl-ions and water. Swelling of the polymer from repulsive forces coupled with osmotic swelling from the influence of water causes the membrane to rupture and release the vector.100,101 Not every polycation, like poly-L-lysine (PLL), is known to elucidate this effect due to lack of buffering capacity arising from lack of amino groups that can be protonated. However, the high effectiveness of other cationic gene delivery vehicles, such as poly(amido amine) dendrimers, lipo polyamines and other imidazole-containing polymers are believed to be attributed to the proton-sponge mechanisms.101 Many recent strategies looking to capitalize on improving this phenomenon have looked to functionalize existing polymers with polycationic peptides (cell penetrating peptides), imidazole terminal groups, designing new polymers such as poly(beta-amino esters) and acid-sensitive moieties.102,103 With the other strategy of using endosomolytic/fusogenic agents, researchers have tried to mimic and apply to non-viral vectors104–106 the perfectly evolved process by which endocytosed viruses undergo a conformational change triggered by endosomal microenvironments that allows for fusing of the virus with the endosomal membrane.101,107 With these strategies, researchers have utilized the acidification of the endosome to trigger phase transition in liposomes that allow them to fuse with the endosomal membranes or trigger the release an endosomolytic/fusogenic peptide or small molecule that can disrupt the cell membrane.108
These recent advances in strategies and modification of non-viral vectors for improved endosomal escape in various applications and cells has already been reviewed in detail.30 While many of those strategies utilized in other cell lines could be adopted with success in neural cells, it is important to investigate and review methods that have already been explored in neural cells and investigate possible future steps. One such study made use of R8, an arginine containing cell-penetrating peptide (CPP). Cationic guanidine moieties facilitate interaction with sulfates found on glycol-membrane proteins of cells while hydrophobicity of the CPP allows for insertion into the cell membrane facilitating endosomal escape. R8 functionalized PEI-600-Cyclodextrin demonstrated improved transfection efficiency in a C6 cell line which the author attributed to an increase in endosomal escape facilitated by the R8 functionality.109 Another study made use of a 22-amino acid long fusogenic peptide (GLFEAIAEFIEGGWEGLIEGCA) that was derived from the amino-terminus of influenza virus hemagglutinin HA2 that is capable of fusing into the lipid bilayer of endocytic vesicles to improve the gene delivery of the PLL based vector both in vitro and in vivo.74
6. Release from carrier
Research utilizing dStorm imaging for single particle tracking of DNA-PBAE polyplexes has been essential to better understand the intracellular fate of polyplexes following endosomal escape. Rierra and colleagues demonstrated that while cells might contain a high number of polyplexes inside the cell following endocytosis and endosomal escape, failure for DNA to de-complex from the carrier at the perinuclear or nuclear regions limited transfection efficiency. Furthermore, the extent of GFP expression in cells was directly correlated to the amount of free DNA observed in the nucleus of cells, highlighting the need for strategies that allow for efficient release of DNA from polymers once inside the cell.110 Studies conducted using lipoplexes demonstrated a similar phenomenon in which a liposomes’ ability to mix and fuse with the endosomal membrane did not correlate with DNA transfer efficiency as release of DNA from the lipoplex during this process was also required.111–115
While these studies promote the idea that release from the polyplex prior to nuclear entry results in high transcription efficiency, it is important to note that these authors and other researchers have observed highly variable nuclear localization and gene expression between cells with a correlation to cell cycle.116 So while nuclear transport and transcription of un-complexed DNA in the perinuclear regions might occur more readily in mitotic cells, the post-mitotic nature of neuronal cells might limit the transcription and gene expression of DNA released prior to nuclear entry. Additionally, release of DNA from vectors prior to nuclear transport will limit the efficacy of nuclear targeting strategies utilized. Other studies, looking at the de-complexion of DNA from non-viral vectors have noted the presence of partially de-complexed DNA and polyplexes inside the nucleus of cells demonstrating the nuclear transport of entire polyplexes can also facilitate gene transfer.116–118 One study demonstrated that only partial decomplexation of the DNA from the polyplex was necessary for gene transcription to occur. Additionally, this study noted that decomplexation of DNA from the polyplex inside the nucleus was a rate-limiting step while nuclear transport of lipoplexes was the rate-limiting step highlighting the need for different strategies dependent on non-viral vector composition.118
For polyplexes a number of strategies have arisen in order to promote release of DNA cargo following endosomal escape. One such strategy, coined “charge reversal”, has been investigated as it was speculated that a change from a cationic charge to an anionic charge would promote quick release of packed DNA. One such group designed a polymer, poly[(2-acryloyl)ethyl(p-boronic acid benzyl) diethylammonium bromide] (B-PDEAEA), that contained a reactive oxygen species (ROS)-labile group that caused the release of a quaternary ammonium group that produced a poly(acrylic acid) within two hours upon oxidation. This quick charge reversal facilitated efficient DNA release following endosomal escape that led to improved transfection efficiency.119 Similar polymers using this strategy have been designed to similar effects.120 Reduction of cationic surface-charge of polymeric based systems through functionalization with hydrophobic domains is another strategy being investigated to improve DNA release as high surface charge limits DNA release due to its strong affinity to negatively charged DNA.121–123 PAMAM dendrimers functionalized with heptafluorobutyric anhydride (fluorodendrimers) showed three-orders of magnitude higher transfection efficacy over unmodified dendrimers due to efficient DNA release following endosomal escape.121
For liposomal based carriers, the majority of strategies have been centered around alteration of lipid composition to lower DNA affinity or alter liposome morphology in order to facilitate release of DNA into the cytoplasm during the endosomal fusion process.124 A number of studies have utilized Dioleoylphosphatidylethanolamine (DOPE) in cationic liposome formulations to improve DNA release during membrane fusion by increasing lipophilicity of the liposome allowing for a hexagonal phase morphology.106,125–127 Inclusion of trimeric surfactants into DOPE containing liposomes has been utilized to improve the stability of these vectors without limiting endosomal escape and DNA release.128 One group demonstrated that utilization of a mixture of the cationic ester version of Dioleoylphosphatidylcholine (EDOPC) and Dilinoleoylphosphatidylcholine (EDLPC) enabled faster DNA release and superior transfection when compared to formulations utilizing only one of the lipids.129
7. Entry to nucleus
Following endosomal escape of non-viral vectors, the genetic material payload must transverse the cytoplasm to the nucleus to elicit a function response. A number of biological barriers, like the cytoskeleton, exist inside the cytosol that can result in the sequestration and limited efficacy of delivered genetic material. Once at the nucleus, larger DNA constructs must rely on an active process to enter the nucleus as neurons are postmitotic cells and therefore don't undergo mitosis where exposure of the nucleus occurs when the nuclear envelope breaks down. However, smaller genetic constructs are capable of simple diffusion through the 10 nm diameter nuclear pore aqueous channel.31 Due to the high possibility of sequestration of large genetic constructs during diffusion130,131 and limited cellular entry, strategies focused on improving nuclear transport are necessary to facilitate the exertion of biological effects of DNA constructs post-delivery. One such strategy is the use of peptide signals rich in basic amino acids, like arginine, that are present in protein transduction pathways or membrane translocation signals,132 like TAT-derived peptides.133,134 In one such study, PAMAM that was functionalized with L-arginine residues (PAMAM-Arg) demonstrated significant improvement in transfection efficiency in Neuro2 cells over unmodified PAMAM and 25k-branched PEI which was attributed to the high degree of arginine residues present promoting nuclear localization (Fig. 5).135 In addition to designing peptides that mimic the characteristics of nuclear localization signals (NLS), groups have investigated peptide sequences derived from viral vectors to enhance nuclear transport post endosomal escape.136,137 One such peptide is a 19-amino acid sequence (MAPTKRKGSCPGAAPNKPK) derived from the simian virus 40 (SV40) major capsid protein Vp1 that has potent nuclear transport activity.136 This peptide sequence was bound to plasmid DNA carried by a neurotensin functionalized PLL based vector and assessed for its ability to improve gene delivery through improved nuclear transport of internalized plasmids DNA. Vectors utilizing the NLS peptide demonstrated improved gene expression in vitro over vectors lacking the NLS peptide and this effect was maintained when vectors were delivered to dopamine neurons of the substantia nigra in vivo with gene expression lasting upwards of 2 months.74 Liposomes functionalized with M9 sequence from the non-classical nuclear localization signal that targets transportin-1 of the nuclear envelope demonstrated significant improvement in transfection efficiency in a variety of neuronal cell lines over liposomes functionalized with SV40-NLS sequence.138 While this group reported improvements in gene delivery efficacy, other groups have had limited success in replicating which leaves the effectiveness of this non-classical NLS sequence in question.32,139
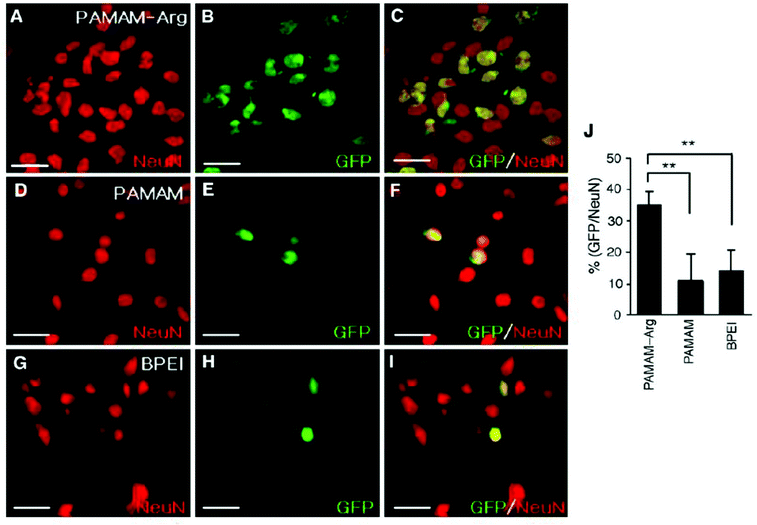 |
| Fig. 5 Transfection of primary cortical cultures with GFP-expressing plasmid using PAMAM-Arg, PAMAM, or PEI. Primary cortical cells were transfected with pEGFP-N1 (1 μg DNA per 4 × 105 cells per well) using PAMAM-Arg (A–C), PAMAM (D–F) or BPEI (G–I). At 48 h post-transfection, cells were washed, fixed in 2% paraformaldehyde, and permeabilized with 0.1% Triton. Images were obtained by immunostaining neurons with anti-Neu N antibody (A, D and G) and EGFP fluorescence (B, E and H) are presented. The right panels are overlays of the two preceding images. The numbers of Neu N-positive cells and GFP-positive cells counted in 20 randomly selected regions from two independent experiments are presented (J). Data represent averages ± SEM. * p < 0.05, ** p < 0.01. Scale bar presents 50 μm. Reproduced with permission from ref. 135. Copyright 2006 Elsevier. | |
III. Applications
While many of the strategies explored focused on demonstrating success in overcoming singular barriers of non-viral gene therapies, significant work has also been conducted in constructing more complex and multifunctional vehicles with the goal of overcoming numerous biological barriers to enable in vivo demonstration of functional gene delivery for therapeutic clinical application. It is evident that the design of these multifunctional vectors is largely rationale driven based on the intended cellular/tissue target, route of administration, and intended therapy. While non-viral vectors have limited exposure in clinical trials for neurological diseases and disorders, these promising preclinical studies demonstrate the potential for clinical presence in the future (Table 1).
Table 1 Various non-viral vectors and their gene delivery target used in specific in vivo models
Application |
Non-viral vector |
Gene delivered |
Cellular target |
Ref. |
Parkinson's disease |
PEGylated Immunoliposomes |
TH gene |
Nigral-striatial neurons |
142
|
Amine-modified ORMOSIL |
eGFP |
Nigral-striatial neurons |
157
|
Angiopep-modified PEGylated-PLL |
hGDNF gene |
DA neurons |
40
|
Neurotensin-modified PEGylated PLL |
hGDNF gene |
DA neurons |
146
|
Neurotensin-modified PEGylated PLL |
BDNF gene |
DA neurons |
148 and 149 |
PEGylated lysine 30-mer peptide |
hGDNF gene |
DA neurons |
147
|
|
Glioblastoma Cancer |
PEGylated PBAE |
HSV-TK |
F98 orthotopic GBM |
29
|
PEGylated PBAE |
Tumor suppressor p53 |
GS-9L orthotopic GBM |
29
|
T7-PEGylated-PLL |
pORF-hTRAIL + Doxorubicin |
U87 MG GBM |
152
|
Angiopep2-PEI-PLL |
HSV-TK |
U87 GBM |
164
|
siRNA-coated gold nanoparticles |
Bcl2Like12 |
GBM |
153
|
|
Cellular modulation |
ORMOSIL |
FGFR1 |
NPCs in SVZ |
162
|
PBAE |
Olig2 and Sox2 |
Astrocytes (in vitro) |
157
|
1. Parkinson's disease
Parkinson's Disease is a neurodegenerative disorder characterized by the degeneration of the nigrostriatal dopaminergic system. More specifically, the loss of dopaminergic neurons of the substantia nigra that project to the putamen results in depletion of critical striatal dopamine leading to clinical features of movement impairment consisting of bradykinesia, resting tremors and rigidity.140,141 Front-line treatment consist of delivery of Levadopa (L-DOPA), a precursor to dopamine precursor, to the brain to replace lost dopamine. However, tolerance to this therapeutic requires increased dosages over time that can eventually result in detrimental side effects, highlighting the potential for gene therapy to offer a more robust treatment plan.
One type of gene treatment for Parkinson's Disease (PD) is tyrosine hydroxylase (TH) replacement therapy. TH is the rate-limiting enzyme in the dopamine synthesis process and therefore significantly contributes to the loss of dopaminergic neurons. TH therapy aims to deliver the TH gene to the nigral-striatal neurons and therefore restore the dopaminergic neurotransmitter release in the striatum. Additionally, TH needs to be selectively expressed in this region of the brain without ectopic TH expression in the cortex or organs not in the brain; ectopic as TH expression can lead to unwanted high levels of dopaminergic activity in the peripheral organs. This study aimed to build on previous experiments35 which used pegylated immunoliposome (PIL) to deliver TH to a rat brain containing 6-hydroxydopamine (6-OHDA) and showed increased TH expression in the nigral-striatal tract and no change of expression in the cortex, as desired. However, this gene delivery led to ectopic expression in the rat liver due to cyclohydrolase I (GTPCH) being expressed in the liver while not in the cortex. The ectopic TH gene expression can, however, be reduced by using a combination of PIL and brain specific promoters. For the purposes of this study, Glial fibrillary acidic protein (GFAP) has shown to eliminate the unwanted expression caused by PIL alone and therefore a combination of TH-plasmid under the influence of a GFAP promoter was created to treat the 6-OHDA-lesioned rats. The PILs were targeted across both the BBB and the neuronal cell membrane with a mAb to the transferrin receptor. The SV40-TH gene therapy (control) showed no increase in TH activity in the cortex but caused a 10-fold increase in TH activity in the liver. On the other hand, there was no increase in liver TH enzyme activity from GFAP-TH encapsulated in the PIL. Both gene therapies additionally completely normalized the TH enzyme activity in the ipsilateral striatum of the 6-OHDA-lesioned rats.142,143
Another strategy for treating Parkinson's disease is treatment with human glial cell line-derived neurotrophic factor (hGDNF) but current treatments utilizing this therapeutic agent rely on chronic infusion which can potentially disrupt the BBB and therefore gene therapy models could be a viable, safer alternative. NT-polyplex has significantly improved transfection efficiency in previous studies while also retaining its biodegradability and other basic features of nonviral vectors.74,75,144,145 One group utilized this vector as their method to deliver genes for hGDNF to the nigral dopamine neurons in vivo as the endocytosis of neurotensin with its receptor NTS1 helps transfer the gene into dopamine neurons for treatment against dopamine neurodegeneration. A single dose of neurotensin polyplex containing the plasmid pEF-Bos-hGDNF into the substantia nigra of hemi-parkinsonian rats 1 week after 6-OHDA was injected into the ipsilateral striatum. RT-PCR showed that hGDNF was expressed in the substantia nigra just 24 h after transfection and remained stable until the end of the study, 3 weeks after treatment as seen in Fig. 6A. Behavioral test analyzing the drug-induced turning behavior after 6-OHDA induced lesions and after transfection with hGDNF demonstrated reduction in turning behavior over 1 and 3 weeks signaling restoration of dopamine neurons as shown in Fig. 6B. Western blot assays confirmed that hGDNF was expressed in the transfected substantia nigra of all animals as well as in the ipsilateral striatum showing its axonal transport from the gene expressing nigral neurons.146 Similar results were obtained when angiopep-2 functionalized poly-L-lysine (DGL) based vectors were used in place of neurotensin functionalized poly-L-lysine vectors mentioned above.40 Lastly, a rod-like nanoparticle composed of plasmid DNA compacted by polyethylene glycol substitute lysine 30-mer peptides was also able to successfully delivery genes for the overexpression of hGDNF in the striatum 1–3 weeks after local injection highlighting the effectiveness of different nanoparticles in the treatment of Parkinson's Disease.147
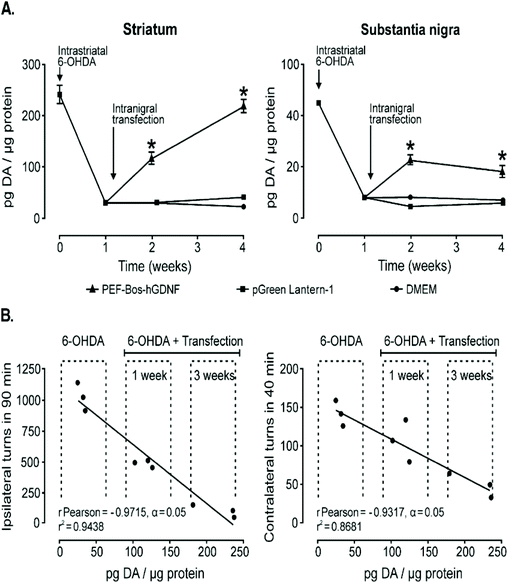 |
| Fig. 6 Dopamine content recovery and its correlation with the hemiparkinsonism remission following intranigral transfection of pEF-Bos-hGDNF. (A) The partial recovery of dopamine content. Each value represents the mean ± SEM of independent measurements in three rats. (B) Correlation analysis between the recovery of dopamine levels in the striatum and the reduction of drug-induced turning behavior in hemiparkinsonian rats with pEF-Bos-hGDNF transfection. Values from nine rats were included in the correlation analysis; three animals were control rats with lesions caused by 6-OHDA injection, three animals were pEF-Bos-hGDNF-transfected rats evaluated 1 week after transfection, and three animals were pEF-Bos-hGDNF-transfected rats evaluated 3 weeks after transfection. *Significantly different from DMEM and pGreen Lantern-1-injected control groups; P < 0.05, two-way ANOVA and Bonferroni posttest. Reproduced with permission from ref. 146 Copyright 2006 Cell Press. | |
In another in vivo Parkinson's disease model148,149 the same NT-polyplex146 was utilized to specifically deliver brain-derived neurotrophic factor (BDNF-flag) genes into dopamine neurons located in the substantia nigra as dopamine neurons have been shown to degenerate in the absence of BDNF. Successful gene transfer of the BDNF-flag was demonstrated in a number of ways but most notably through electromyography activity of the muscles of the hind limbs as loss of dopamine neurons produces muscle rigidity. Mice treated with the NT-polyplex demonstrated normalized electromyography activity for up to 2 months after treatment while saline controls were unable to elicit normalization. Confirmation of recovered number of TH+ nigrostriatal neurons in the treatment group confirmed analysis seen in the behavioral test. Overall, the neurotensin polyplex has shown to be a viable gene vector for gene therapies related to Parkinson's disease due to its ability to selectively deliver genes to dopamine neurons in the substantia nigra by using NT targeting ligands to induce high specificity and efficiency.
2. Glioblastoma cancer
Glioblastoma (GBM) is the most common and aggressive form of primary brain tumor that is defined by the presence of high-grade astrocytic neoplasm with either microvascular proliferation or tumor necrosis.150 Glioblastomas can be divided into subtypes dependent on molecular differences stemming from genetic and epigenetic differences. Despite the multimodal aggressive standard of care, extension in median survival time is only marginally improved stemming from difficulty in therapy distribution and these molecular differences in glioblastomas highlighting the need and potential for targeted gene therapy.35,150,151 Clinical trials using both viral and non-viral gene therapy methods have fallen short with limited therapeutic benefit attributed to poor distribution of therapeutic transgene expression throughout the brain.61 Work previously conducted in design of small PEGylation PBAE vectors, (DNA-BPN), demonstrated widespread GFP transgene expression in vivo when coupled with convection enhanced delivery.60 Based on this reason DNA-BPN non-viral vectors complexed with plasmids encoding for suicide herpes simplex virus type-1 thymidine kinase protein (HSV-tk) was investigated for its therapeutic potential in a F98 orthotopic tumor model in addition to DNA-BPN vectors complexed with plasmids encoding for tumor suppressor p53 protein evaluated in a GS-9L orthotopic model. Both models showed similar characteristics seen in previous studies where PEGylated PBAE polyplexes facilitated widespread transgene expression (Fig. 7A–C), which facilitated significant improvement in medium survival time when compared to non-PEGylated PBAE polyplexes and saline controls (Fig. 7D).29 In addition to this PEGylated PBAE vector, a vector was designed around the co-delivery of Doxorubicin, a small molecule chemotherapeutic drug, and pORF-hTRAIL gene agent for the combined therapy of glioma. The vector was composed of a PEGylated poly-L-lysine (DGL) that utilized a T7 peptide (HAIYPRH) targeting ligand that has specificity towards the transferring receptor that is overexpressed in both the BBB and brain glioma cells. The vector exhibited enhanced cellular uptake in tumor cells and enhanced transcytosis which culminated in a better therapeutic output using a co-delivery strategy with a median survival time of ∼60 days versus ∼35 days for mono-treatment.152
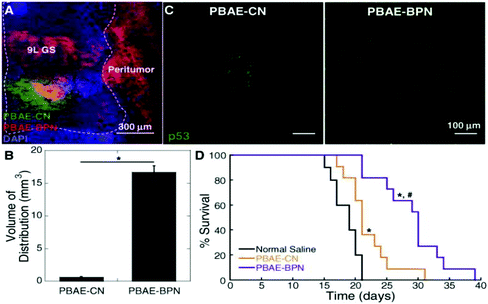 |
| Fig. 7
In vivo distribution, transgene expression and therapeutic effect in 9L GS-bearing rat brain tissues following CED of DNA-loaded nanocomplexes. (A) Representative image showing the distribution of PBAE-CN (Cy3; green) and PBAE-BPN (Cy5; red) in the 9L GS-bearing rat brain. DAPI (blue) staining indicates cell nuclei and co-localization of PBAE-CN and PBAE-BPN is depicted in yellow. Dashed line delineates the boundary between healthy and 9L GS tumor tissues. Scale bar = 300 μm. (B) Image-based quantification of volume of nanocomplex distribution in 9L GS-bearing rat brain tissues. Data represents the mean ± SEM (n = 3 per group). Difference is statistically significant (*p < 0.01). (C) Representative fluorescence images demonstrating p53 transgene expression mediated by CED of PBAE-CN (left) and PBAE-BPN (right). Scale bar = 100 μm. (D) Kaplan–Meier survival curve of an orthotopic 9L GS model following CED of nanocomplexes carrying plasmid DNA encoding p53 (i.e. pC53-SN3) or normal saline (n = 10–11 per group). Differences are statistically significant compared to groups treated with normal saline (*p < 0.01) and PBAE-CN (p < 0.00001). Reproduced with permission from ref. 29 Copyright 2016 American Chemistry Society. | |
More recently, RNA interference-based spherical nucleic acids have been developed for glioblastoma treatment. These vectors consist of a gold or lipid core that is surrounded by a oligonucleotide shell. In a phase 0 clinical trial in eight patients with recurrent glioblastoma, it was found that intravenous delivery of gold nanoparticle core spherical nucleic acids had no grade 4 or 5 treatment-related toxicities. Further, intravenous delivery resulted in intratumoral accumulation and Bcl2L12 protein expression reduction.153
3. Modulation of NPCs and CNS cell types
Many disease states of neurological disorders and disorders are characterized by the loss of neurons that can't be replaced due to the nervous system's limited potential to generate new neurons.154 However, stem-cell like cells do exist in the brain in the subventricular zone as neural progenitor cells155 that do have the potential to differentiate into neural cells if provided with appropriate cellular and environmental signals.156 One group designed an organically modified silica nanoparticle (ORMOSIL) that was modified with cationic amino groups to facilitate binding of a genes for fibroblast growth factor receptor 1 (FGFR1) to be delivered to NPCs via a subventrical injection in order to induce differentiation for therapeutic manipulation.157,158 In a different manner, astrocytes have been recently shown to be capable of cellular reprogramming into functional neurons through viral transduction of transcription factors such as Sox2159 and Neurogenin 2160 or into functional oligodendrocytes through viral overexpression of Olig2.161 One group designed a PBAE based polymer to explore the potential of non-viral vectors to mimic viral vectors capacity to deliver transcription factors to direct cellular reprogramming in astrocytes.162 PBAE based vectors were chosen due to the buffering capacity to promote endosomal escape via proton-sponge effect, biocompatibility arising from biodegradability, and high customization of terminal end-groups to promote cellular uptake via caveolae-mediated endocytosis and improved gene delivery.163 Use of these PBAE vectors to deliver genes for Sox2 expression in vitro showed that these particles were effectively uptaken by astrocytes and capable of converting them into Tuj1+ neurons. Likewise, when delivering genes for Olig2 expression, astrocytes were effectively converted into oligodendrocytes as seen in presence of oligodendrocyte specific markers in transfected cells. While in vivo work was not conducted using the PBAE based vectors to determine if astrocytes contained within the CNS can be differentiated into functional neurons or oligodendrocytes, it highlights the potential use in both in vitro and in vivo research.162
IV. Conclusion
Research into non-viral vectors has sought to overcome numerous biological barriers, such as the blood–brain barrier, cell-specific targeting and uptake, endosomal escape and nuclear transport, in order to advance the gene delivery efficacy to similar levels of that of current adeno-associated viral vectors. A number of strategies have been explored in vitro and in vivo that have culminated in a number of successful application-based studies that used multi-functional non-viral vectors to demonstrate efficacy in gene delivery.
Conflicts of interest
The authors declare no competing financial interest.
Acknowledgements
H. W. acknowledges funding support from NIH Mentored Research Scientist Career Development Award (National Institute of Mental Health 1K01MH117490-01) and Welsh Foundation Grant (F-2084-20210327). N.A.P. acknowledges support from the NIH (R01-EB022025).
References
- A. D. Van Laar,
et al., An Update on Gene Therapy Approaches for Parkinson’s Disease: Restoration of Dopaminergic Function, J. Parkinson’s Dis., 2021, 11(S2), 173–182 Search PubMed.
- E. M. Prasad and S.-Y. Hung, Current Therapies in Clinical Trials of Parkinson’s Disease: A 2021 Update, Pharmaceuticals, 2021, 14(8), 717 CrossRef CAS PubMed.
- K. M. Miah, S. C. Hyde and D. R. Gill, Emerging gene therapies for cystic fibrosis, Expert Rev. Respir. Med., 2019, 13, 709–725 CrossRef CAS PubMed.
- Z. Yan, P. B. McCray Jr. and J. F. Engelhardt, Advances in gene therapy for cystic fibrosis lung disease, Hum. Mol. Genet., 2019, 28, R88–R94 CrossRef CAS PubMed.
- D. Cross and J. K. Burmester, Gene therapy for cancer treatment: past, present and future, Clin. Med. Res., 2006, 4(3), 218–227 CrossRef CAS PubMed.
- M. H. Amer, Gene therapy for cancer: present status and future perspective, Mol. Cell. Ther., 2014, 2, 27 CrossRef PubMed.
- Y. K. Sung and S. W. Kim, Recent advances in the development of gene delivery systems, Biomater. Res., 2019, 23, 1–7 CrossRef PubMed.
- M. Ramamoorth and A. N. Non, Viral Vectors in Gene Therapy- An Overview, J. Clin. Diagn. Res., 2015, 9, GE01 Search PubMed.
- M. Morille, C. Passirani, A. Vonarbourg, A. Clavreul and J.-P. Benoit, Progress in developing cationic vectors for non-viral systemic gene therapy against cancer, Biomaterials, 2008, 29, 3477–3496 CrossRef CAS PubMed.
- C. A. Maguire, S. H. Ramirez, S. F. Merkel, M. Sena-Esteves and X. O. Breakefield, Gene therapy for the nervous system: challenges and new strategies, Neurotherapeutics, 2014, 11(4), 817–839 CrossRef CAS PubMed.
- H. C. Verdera, K. Kuranda and F. Mingozzi, AAV Vector Immunogenicity in Humans: A Long Journey to Successful Gene Transfer, Mol. Ther., 2020, 28, 723–746 CrossRef CAS PubMed.
- C. S. Peden, C. Burger, N. Muzyczka and R. J. Mandel, Circulating anti-wild-type adeno-associated virus type 2 (AAV2) antibodies inhibit recombinant AAV2 (rAAV2)-mediated, but not rAAV5-mediated, gene transfer in the brain, J. Virol., 2004, 78, 6344–6359 CrossRef CAS PubMed.
- R. A. Dewey,
et al., Chronic brain inflammation and persistent herpes simplex virus 1 thymidine kinase expression in survivors of syngeneic glioma treated by adenovirus-mediated gene therapy: implications for clinical trials, Nat. Med., 1999, 5, 1256–1263 CrossRef CAS PubMed.
- M. J. Wood, H. M. Charlton, K. Kajiwara and A. P. Byrnes, Immune responses to adenovirus vectors in the nervous system, Trends Neurosci., 1996, 19, 497–501 CrossRef CAS PubMed.
- Y. Persidsky, S. H. Ramirez, J. Haorah and G. D. Kanmogne, Blood-brain barrier: structural components and function under physiologic and pathologic conditions, J. Neuroimmune Pharmacol., 2006, 1, 223–236 CrossRef PubMed.
- N. Kuriyama, H. Kuriyama, C. M. Julin, K. Lamborn and M. A. Israel, Pretreatment with protease is a useful experimental strategy for enhancing adenovirus-mediated cancer gene therapy, Hum. Gene Ther., 2000, 11(16), 2219–2230 CrossRef CAS PubMed.
- P. Hadaczek, H. Mirek, J. Bringas, J. Cunningham and K. Bankiewicz, Basic fibroblast growth factor enhances transduction, distribution, and axonal transport of adeno-associated virus type 2 vector in rat brain, Hum. Gene Ther., 2004, 15, 469–479 CrossRef CAS PubMed.
- N. Tao,
et al., Sequestration of adenoviral vector by Kupffer cells leads to a nonlinear dose response of transduction in liver, Mol. Ther., 2001, 3, 28–35 CrossRef CAS PubMed.
- J. C. Grieger and R. J. Samulski, Packaging capacity of adeno-associated virus serotypes: impact of larger genomes on infectivity and postentry steps, J. Virol., 2005, 79, 9933–9944 CrossRef CAS PubMed.
- J. T. Bulcha, Y. Wang, H. Ma, P. W. L. Tai and G. Gao, Viral vector platforms within the gene therapy landscape, Signal Transduction Targeted Ther., 2021, 6, 1–24 CrossRef PubMed.
- F. C. Pérez-Martínez, J. Guerra, I. Posadas and V. Ceña, Barriers to non-viral vector-mediated gene delivery in the nervous system, Pharm. Res., 2011, 28, 1843–1858 CrossRef PubMed.
- N. Shi and W. M. Pardridge, Noninvasive gene targeting to the brain, Proc. Natl. Acad. Sci. U. S. A., 2000, 97, 7567–7572 CrossRef CAS PubMed.
- B. P. Mead,
et al., Targeted gene transfer to the brain via the delivery of brain-penetrating DNA nanoparticles with focused ultrasound, J. Controlled Release, 2016, 223, 109–117 CrossRef CAS PubMed.
- E. Syková and C. Nicholson, Diffusion in brain extracellular space, Physiol. Rev., 2008, 88(4), 1277–1340 CrossRef PubMed.
- D. R. Zimmermann and M. T. Dours-Zimmermann, Extracellular matrix of the central nervous system: from neglect to challenge, Histochem. Cell Biol., 2008, 130(4), 635–653 CrossRef CAS PubMed.
- C. S. Barros, S. J. Franco and U. Müller, Extracellular Matrix: Functions in the Nervous System, Cold Spring Harbor Perspect. Biol., 2011, 3(1), a005108 Search PubMed.
- E. A. Nance,
et al., A dense poly(ethylene glycol) coating improves penetration of large polymeric nanoparticles within brain tissue, Sci. Transl. Med., 2012, 4, 149ra119 Search PubMed.
- G. D. Kenny,
et al., Multifunctional receptor-targeted nanocomplexes for the delivery of therapeutic nucleic acids to the brain, Biomaterials, 2013, 34, 9190–9200 CrossRef CAS PubMed.
- P. Mastorakos,
et al., Biodegradable brain-penetrating DNA nanocomplexes and their use to treat malignant brain tumors, J. Controlled Release, 2017, 262, 37 CrossRef CAS PubMed.
- E. Xu, W. M. Saltzman and A. S. Piotrowski-Daspit, Escaping the endosome: assessing cellular trafficking mechanisms of non-viral vehicles, J. Controlled Release, 2021, 335, 465–480 CrossRef CAS PubMed.
- M. E. Dowty, P. Williams, G. Zhang, J. E. Hagstrom and J. A. Wolff, Plasmid DNA entry into postmitotic nuclei of primary rat myotubes, Proc. Natl. Acad. Sci. U. S. A., 1995, 92, 4572–4576 CrossRef CAS PubMed.
- E. V. B. van Gaal,
et al., DNA nuclear targeting sequences for non-viral gene delivery, Pharm. Res., 2011, 28, 1707–1722 CrossRef CAS PubMed.
- Y. Zhang, F. Schlachetzki and W. M. Pardridge, Global non-viral gene transfer to the primate brain following intravenous administration, Mol. Ther., 2003, 7, 11–18 CrossRef CAS PubMed.
- N. Shi, Y. Zhang, C. Zhu, R. J. Boado and W. M. Pardridge, Brain-specific expression of an exogenous gene after i.v. administration, Proc. Natl. Acad. Sci. U. S. A., 2001, 98, 12754–12759 CrossRef CAS PubMed.
- N. Shi, R. J. Boado and W. M. Pardridge, Receptor-mediated gene targeting to tissues in vivo following intravenous administration of pegylated immunoliposomes, Pharm. Res., 2001, 18, 1091–1095 CrossRef CAS PubMed.
- R.-Q. Huang,
et al., Efficient gene delivery targeted to the brain using a transferrin-conjugated polyethyleneglycol-modified polyamidoamine dendrimer, FASEB J., 2007, 21, 1117–1125 CrossRef CAS PubMed.
- P. Kumar,
et al., Transvascular delivery of small interfering RNA to the central nervous system, Nature, 2007, 448, 39–43 CrossRef CAS PubMed.
- T.-E. Park,
et al., Enhanced BBB permeability of osmotically active poly(mannitol-co-PEI) modified with rabies virus glycoprotein via selective stimulation of caveolar endocytosis for RNAi therapeutics in Alzheimer's disease, Biomaterials, 2015, 38, 61–71 CrossRef CAS PubMed.
- B. Pulford,
et al., Liposome-siRNA-peptide complexes cross the blood-brain barrier and significantly decrease PrP on neuronal cells and PrP in infected cell cultures, PLoS One, 2010, 5, e11085 CrossRef PubMed.
- R. Huang,
et al., Angiopep-conjugated nanoparticles for targeted long-term gene therapy of Parkinson’s disease, Pharm. Res., 2013, 30(10), 2549–2559 CrossRef CAS PubMed.
- W. Ke,
et al., Gene delivery targeted to the brain using an Angiopep-conjugated polyethyleneglycol-modified polyamidoamine dendrimer, Biomaterials, 2009, 30, 6976–6985 CrossRef CAS PubMed.
- Y. Liu, J. Li, K. Shao, R. Huang, L. Ye, J. Lou and C. Jiang, A leptin derived 30-amino-acid peptide modified pegylated poly-l-lysine dendrigraft for brain targeted gene delivery, Biomaterials, 2010, 31, 5246–5257 CrossRef CAS PubMed.
- Y. Qian,
et al., PEGylated poly(2-(dimethylamino) ethyl methacrylate)/DNA polyplex micelles decorated with phage-displayed TGN peptide for brain-targeted gene delivery, Biomaterials, 2013, 34, 2117–2129 CrossRef CAS PubMed.
- Q. Guo,
et al., A dual-ligand fusion peptide improves the brain-neuron targeting of nanocarriers in Alzheimer's disease mice, J. Controlled Release, 2020, 320, 347–362 CrossRef CAS PubMed.
- H. Yao, K. Wang, Y. Wang, S. Wang, J. Li, J. Lou, L. Ye, X. Yan, W. Lu and R. Huang, Enhanced blood–brain barrier penetration and glioma therapy mediated by a new peptide modified gene delivery system, Biomaterials, 2015, 37, 345–352 CrossRef CAS PubMed.
- D. Hühn,
et al., Polymer-coated nanoparticles interacting with proteins and cells: focusing on the sign of the net charge, ACS Nano, 2013, 7(4), 3253–3263 CrossRef PubMed.
- A. C. Bonoiu,
et al., Nanotechnology approach for drug addiction therapy: gene silencing using delivery of gold nanorod-siRNA nanoplex in dopaminergic neurons, Proc. Natl. Acad. Sci. U. S. A., 2009, 106, 5546–5550 CrossRef CAS PubMed.
- M. Khongkow,
et al., Surface modification of gold nanoparticles with neuron-targeted exosome for enhanced blood-brain barrier penetration, Sci. Rep., 2019, 9, 8278 CrossRef PubMed.
- W. M. Pardridge, Drug transport in brain via the cerebrospinal fluid, Fluids Barriers CNS, 2011, 8(1), 7 CrossRef PubMed.
- J. J. Glascock,
et al., Delivery of Therapeutic Agents Through Intracerebroventricular (ICV) and Intravenous (IV) Injection in Mice, J. Visualized Exp., 2011, 3(56), 2968 Search PubMed.
- Z. Zhang,
et al., Brain-targeted drug delivery by manipulating protein corona functions, Nat. Commun., 2019, 10, 3561 CrossRef PubMed.
- W. Xiao,
et al., The protein corona hampers the transcytosis of transferrin-modified nanoparticles through blood-brain barrier and attenuates their targeting ability to brain tumor, Biomaterials, 2021, 274, 120888 CrossRef CAS PubMed.
- A. Salvati,
et al., Transferrin-functionalized nanoparticles lose their targeting capabilities when a biomolecule corona adsorbs on the surface, Nat. Nanotechnol., 2013, 8, 137–143 CrossRef CAS PubMed.
- J. A. MacKay, D. F. Deen and F. C. Szoka Jr., Distribution in brain of liposomes after convection enhanced delivery; modulation by particle charge, particle diameter, and presence of steric coating, Brain Res., 2005, 1035, 139–153 CrossRef CAS PubMed.
- P. Mastorakos,
et al., Highly PEGylated DNA Nanoparticles Provide Uniform and Widespread Gene Transfer in the Brain, Adv. Healthcare Mater., 2015, 4, 1023–1033 CrossRef CAS PubMed.
- D. M. Ward, A. B. Shodeinde and N. A. Peppas, Innovations in Biomaterial Design toward Successful RNA Interference Therapy for Cancer Treatment, Adv. Healthcare Mater., 2021, 10, e2100350 CrossRef PubMed.
- H. Lopez-Bertoni,
et al., Bioreducible Polymeric Nanoparticles Containing Multiplexed Cancer Stem Cell Regulating miRNAs Inhibit Glioblastoma Growth and Prolong Survival, Nano Lett., 2018, 18(7), 4086–4094 CrossRef CAS PubMed.
- F. Vidal,
et al., Mechanism of PAMAM Dendrimers Internalization in Hippocampal Neurons, Mol. Pharm., 2016, 13, 3395–3403 CrossRef CAS PubMed.
- O. M. Merkel,
et al., Integrin alphaVbeta3 targeted gene delivery using RGD peptidomimetic conjugates with copolymers of PEGylated poly(ethylene imine), Bioconjugate Chem., 2009, 20, 1270–1280 CrossRef CAS PubMed.
- P. Mastorakos,
et al., Biodegradable DNA Nanoparticles that Provide Widespread Gene Delivery in the Brain, Small, 2016, 12, 678–685 CrossRef CAS PubMed.
- T. Wakabayashi,
et al., A phase I clinical trial of interferon-beta gene therapy for high-grade glioma: novel findings from gene expression profiling and autopsy, J. Gene Med., 2008, 10, 329–339 CrossRef CAS PubMed.
- J. Voges,
et al., Clinical protocol. Liposomal gene therapy with the herpes simplex thymidine kinase gene/ganciclovir system for the treatment of glioblastoma multiforme, Hum. Gene Ther., 2002, 13, 675–685 CrossRef CAS PubMed.
-
D. Purves, et al., Neuroscience, Neuroglial Cells, 2nd edn, 2001 Search PubMed.
- L. C. Williamson, K. E. Bateman, J. C. Clifford and E. A. Neale, Neuronal sensitivity to tetanus toxin requires gangliosides, J. Biol. Chem., 1999, 274, 25173–25180 CrossRef CAS PubMed.
- J. K. Liu,
et al., A novel peptide defined through phage display for therapeutic protein and vector neuronal targeting, Neurobiol. Dis., 2005, 19, 407–418 CrossRef CAS PubMed.
- A. Mathew,
et al., Curcumin loaded-PLGA nanoparticles conjugated with Tet-1 peptide for potential use in Alzheimer's disease, PLoS One, 2012, 7, e32616 CrossRef CAS PubMed.
- Y. Zhang,
et al., Targeted delivery of Tet1 peptide functionalized polymersomes to the rat cochlear nerve, Int. J. Nanomed., 2012, 7, 1015–1022 CrossRef CAS PubMed.
- E. J. Kwon,
et al., Targeted nonviral delivery vehicles to neural progenitor cells in the mouse subventricular zone, Biomaterials, 2010, 31, 2417–2424 CrossRef CAS PubMed.
- D. S. H. Chu, J. G. Schellinger, M. J. Bocek, R. N. Johnson and S. H. Pun, Optimization of Tet1 ligand density in HPMA-co-oligolysine copolymers for targeted neuronal gene delivery, Biomaterials, 2013, 34, 9632–9637 CrossRef CAS PubMed.
- I.-K. Park, J. Lasiene, S.-H. Chou, P. J. Horner and S. H. Pun, Neuron-specific delivery of nucleic acids mediated by Tet1-modified poly(ethylenimine), J. Gene Med., 2007, 9, 691–702 CrossRef CAS PubMed.
- A. K. Chojnacki, G. K. Mak and S. Weiss, Identity crisis for adult periventricular neural stem cells: subventricular zone astrocytes, ependymal cells or both?, Nat. Rev. Neurosci., 2009, 10, 153–163 CrossRef CAS PubMed.
- D. Martinez-Fong,
et al., Neurotensin-SPDP-poly-L-lysine conjugate: a nonviral vector for targeted gene delivery to neural cells, Brain Res. Mol. Brain Res. e.g. Molecular Brain Research., 1999, 69, 249–262 CrossRef CAS.
- D. Hernandez-Baltazar, D. Martinez-Fong and L.-E. Trudeau, Optimizing NTS-polyplex as a tool for gene transfer to cultured dopamine neurons, PLoS One, 2012, 7, e51341 CrossRef CAS PubMed.
- I. Navarro-Quiroga,
et al., Improved neurotensin-vector-mediated gene transfer by the coupling of hemagglutinin HA2 fusogenic peptide and Vp1 SV40 nuclear localization signal, Brain Res. Mol. Brain Res. e.g. Molecular brain research., 2002, 105, 86–97 CrossRef CAS.
- I. Alvarez-Maya, I. Navarro-Quiroga, M. A. Meraz-Ríos, J. Aceves and D. Martinez-Fong, In vivo gene transfer to dopamine neurons of rat substantia nigra via the high-affinity neurotensin receptor, Mol. Med., 2001, 7, 186–192 CAS.
- J. Zeng and S. Wang, Enhanced gene delivery to PC12 cells by a cationic polypeptide, Biomaterials, 2005, 26, 679–686 CrossRef CAS PubMed.
- M.-L. Rogers,
et al., Non-viral gene therapy that targets motor neurons in vivo, Front. Mol. Neurosci., 2014, 7, 80 Search PubMed.
- S. Kumari, S. Mg and S. Mayor, Endocytosis unplugged: multiple ways to enter the cell, Cell Res., 2010, 20, 256–275 CrossRef CAS PubMed.
- N. Gabrielson and D. Pack, Efficient polyethylenimine-mediated gene delivery proceeds via a caveolar pathway in HeLa cells, Nat. Preced., 2008 DOI:10.1038/npre.2008.2262.1.
- J. Rejman, A. Bragonzi and M. Conese, Role of clathrin- and caveolae-mediated endocytosis in gene transfer mediated by lipo- and polyplexes, Mol. Ther., 2005, 12, 468–474 CrossRef CAS PubMed.
- J. V. Georgieva,
et al., Surface Characteristics of Nanoparticles Determine Their Intracellular Fate in and Processing by Human Blood–Brain Barrier Endothelial Cells In Vitro, Mol. Ther., 2011, 19, 318–325 CrossRef CAS PubMed.
- I. A. Khalil, K. Kogure, H. Akita and H. Harashima, Uptake Pathways and Subsequent Intracellular Trafficking in Nonviral Gene Delivery, Pharmacol. Rev., 2006, 58, 32–45 CrossRef CAS PubMed.
- L. Albertazzi,
et al., In vivo distribution and toxicity of PAMAM dendrimers in the central nervous system depend on their surface chemistry, Mol. Pharm., 2013, 10, 249–260 CrossRef CAS PubMed.
- N. J. Kavimandan, E. Losi, J. J. Wilson, J. S. Brodbelt and N. A. Peppas, Synthesis and characterization of insulin-transferrin conjugates, Bioconjugate Chem., 2006, 17(6), 1376–1384 CrossRef CAS PubMed.
- S. Lakkadwala, B. Dos Santos Rodrigues, C. Sun and J. Singh, Biodistribution of TAT or QLPVM coupled to receptor targeted liposomes for delivery of anticancer therapeutics to brain in vitro and in vivo, Nanomedicine, 2020, 23, 102112 CrossRef CAS PubMed.
- D. C. Mash, J. Pablo, D. D. Flynn, S. M. Efange and W. J. Weiner, Characterization and distribution of transferrin receptors in the rat brain, J. Neurochem., 1990, 55, 1972–1979 CrossRef CAS PubMed.
- W. Zhao,
et al., Brain insulin receptors and spatial memory. Correlated changes in gene expression, tyrosine phosphorylation, and signaling molecules in the hippocampus of water maze trained rats, J. Biol. Chem., 1999, 274, 34893–34902 CrossRef CAS PubMed.
- J. Habault and J.-L. Poyet, Recent Advances in Cell Penetrating Peptide-Based Anticancer Therapies, Molecules, 2019, 24(5), 927 CrossRef PubMed.
- A. M. O'Mahony,
et al., In vitro investigations of the efficacy of cyclodextrin-siRNA complexes modified with lipid-PEG-octaarginine: towards a formulation strategy for effective neuronal siRNA delivery, Pharm. Res., 2012, 1–13 Search PubMed.
- K. Kunath, T. Merdan, O. Hegener, H. Häberlein and T. Kissel, Integrin targeting using RGD-PEI conjugates for in vitro gene transfer, J. Gene Med., 2003, 5(7), 588–599 CrossRef CAS PubMed.
- A. Ziegler and J. Seelig, Interaction of the protein transduction domain of HIV-1 TAT with heparan sulfate: binding mechanism and thermodynamic parameters, Biophys. J., 2004, 86, 254–263 CrossRef CAS PubMed.
- J. S. Suk,
et al., Gene delivery to differentiated neurotypic cells with RGD and HIV Tat peptide functionalized polymeric nanoparticles, Biomaterials, 2006, 27, 5143 CrossRef CAS PubMed.
- N. M. Moore and S. E. Sakiyama-Elbert, Analysis of cell binding and internalization of multivalent PEG-based gene delivery vehicles, IEEE Trans. Nanobiosci., 2012, 11, 54–61 Search PubMed.
- C. Dufès, M. Al Robaian and S. Somani, Transferrin and the transferrin receptor for the targeted delivery of therapeutic agents to the brain and cancer cells, Ther. Delivery, 2013, 4, 629–640 CrossRef PubMed.
- P. G. Mastroberardino,
et al., A novel transferrin/TfR2-mediated mitochondrial iron transport system is disrupted in Parkinson's disease, Neurobiol. Dis., 2009, 34, 417 CrossRef CAS PubMed.
- C. Zhan,
et al., Cyclic RGD-poly(ethylene glycol)-polyethyleneimine is more suitable for glioblastoma targeting gene transfer in vivo, J. Drug Targeting, 2011, 19, 573–581 CrossRef CAS PubMed.
- T. Merdan,
et al., PEGylation of poly(ethylene imine) affects stability of complexes with plasmid DNA under in vivo conditions in a dose-dependent manner after intravenous injection into mice, Bioconjugate Chem., 2005, 16(4), 785–792 CrossRef CAS PubMed.
- M. Lee and S. W. Kim, Polyethylene glycol-conjugated copolymers for plasmid DNA delivery, Pharm. Res., 2005, 22, 1–10 CrossRef CAS PubMed.
- S. Lai,
et al., Privileged delivery of polymer nanoparticles to the perinuclear region of live cells via a non-clathrin, non-degradative pathway, Biomaterials, 2007, 28, 2876–2884 CrossRef CAS PubMed.
- R. V. Benjaminsen, M. A. Mattebjerg, J. R. Henriksen, S. Moein Moghimi and T. L. Andresen, The Possible ‘Proton Sponge ‘ Effect of Polyethylenimine (PEI) Does Not Include Change in Lysosomal pH, Mol. Ther., 2013, 21, 149 CrossRef CAS PubMed.
- A. Akinc, M. Thomas, A. M. Klibanov and R. Langer, Exploring polyethylenimine-mediated DNA transfection and the proton sponge hypothesis, J. Gene Med., 2005, 7, 657–663 CrossRef CAS PubMed.
- T. Jiang,
et al., Dual-functional liposomes based on pH-responsive cell-penetrating peptide and hyaluronic acid for tumor-targeted anticancer drug delivery, Biomaterials, 2012, 33, 9246–9258 CrossRef CAS PubMed.
- P. Lönn,
et al., Enhancing Endosomal Escape for Intracellular Delivery of Macromolecular Biologic Therapeutics, Sci. Rep., 2016, 6, 32301 CrossRef PubMed.
- I. M. Hafez, N. Maurer and P. R. Cullis, On the mechanism whereby cationic lipids promote intracellular delivery of polynucleic acids, Gene Ther., 2001, 8, 1188–1196 CrossRef CAS PubMed.
- P. Midoux, E. LeCam, D. Coulaud, E. Delain and C. Pichon, Histidine containing peptides and polypeptides as nucleic acid vectors, Somatic Cell Mol. Genet., 2002, 27, 27–47 CrossRef CAS PubMed.
- S. Tassler,
et al., Lysine-based amino-functionalized lipids for gene transfection: 3D phase behaviour and transfection performance, Phys. Chem. Chem. Phys., 2018, 20, 17393–17405 RSC.
- C. Burkard,
et al., Coronavirus Cell Entry Occurs through the Endo-/Lysosomal Pathway in a Proteolysis-Dependent Manner, PLoS Pathog., 2014, 10, e1004502 CrossRef PubMed.
- E. K. Esbjörner, K. Oglecka, P. Lincoln, A. Gräslund and B. Nordén, Membrane binding of pH-sensitive influenza fusion peptides. positioning, configuration, and induced leakage in a lipid vesicle model, Biochemistry, 2007, 46(47), 13490–13504 CrossRef PubMed.
- Q.-Y. Jiang,
et al., Gene delivery to tumor cells by cationic polymeric nanovectors coupled to folic acid and the cell-penetrating peptide octaarginine, Biomaterials, 2011, 32, 7253–7262 CrossRef CAS PubMed.
- R. Riera,
et al., Tracking the DNA complexation state of pBAE polyplexes in cells with super resolution microscopy, Nanoscale, 2019, 11, 17869–17877 RSC.
- B. Mui, Q. F. Ahkong, L. Chow and M. J. Hope, Membrane perturbation and the mechanism of lipid-mediated transfer of DNA into cells, Biochim. Biophys. Acta, 2000, 1467(2), 281–292 CrossRef CAS.
- T. Stegmann and J. Y. Legendre, Gene transfer mediated by cationic lipids: lack of a correlation between lipid mixing and transfection, Biochim. Biophys. Acta, 1997, 1325, 71–79 CrossRef CAS.
- P. Harvie, F. M. Wong and M. B. Bally, Characterization of lipid DNA interactions. I. Destabilization of bound lipids and DNA dissociation, Biophys. J., 1998, 75, 1040–1051 CrossRef CAS PubMed.
- R. Tachibana,
et al., Quantitative analysis of correlation between number of nuclear plasmids and gene expression activity after transfection with cationic liposomes, Pharm. Res., 2002, 19, 377–381 CrossRef CAS PubMed.
- J. Zabner, A. J. Fasbender, T. Moninger, K. A. Poellinger and M. J. Welsh, Cellular and molecular barriers to gene transfer by a cationic lipid, J. Biol. Chem., 1995, 270(32), 18997–19007 CrossRef CAS PubMed.
- U. Lächelt and E. Wagner, Nucleic Acid Therapeutics Using Polyplexes: A Journey of 50 Years (and Beyond), Chem. Rev., 2015, 115, 11043–11078 CrossRef PubMed.
- B. Shi,
et al., Challenges in DNA Delivery and Recent Advances in Multifunctional Polymeric DNA Delivery Systems, Biomacromolecules, 2017, 18, 2231–2246 CrossRef CAS PubMed.
- Y. Matsumoto, K. Itaka, T. Yamasoba and K. Kataoka, Intranuclear fluorescence resonance energy transfer analysis of plasmid DNA decondensation from nonviral gene carriers, J. Gene Med., 2009, 11, 615–623 CrossRef CAS PubMed.
- X. Liu,
et al., Fusogenic Reactive Oxygen Species Triggered Charge-Reversal Vector for Effective Gene Delivery, Adv. Mater., 2016, 28, 1743–1752 CrossRef CAS PubMed.
- N. Qiu,
et al., Esterase-Activated Charge-Reversal Polymer for Fibroblast-Exempt Cancer Gene Therapy, Adv. Mater., 2016, 28, 10613–10622 CrossRef CAS PubMed.
- H. Wang,
et al., Self-Assembled Fluorodendrimers Combine the Features of Lipid and Polymeric Vectors in Gene Delivery, Angew. Chem., Int. Ed., 2015, 54, 11647–11651 CrossRef CAS PubMed.
- Z. Zhang,
et al., The fluorination effect of fluoroamphiphiles in cytosolic protein delivery, Nat. Commun., 2018, 9, 1377 CrossRef PubMed.
- Z. Liu, Z. Zhang, C. Zhou and Y. Jiao, Hydrophobic modifications of cationic polymers for gene delivery, Prog. Polym. Sci., 2010, 35, 1144–1162 CrossRef CAS.
- Y. Sun and E. Davis, Nanoplatforms for Targeted Stimuli-Responsive Drug Delivery: A Review of Platform Materials and Stimuli-Responsive Release and Targeting Mechanisms, Nanomaterials, 2021, 11(3), 746 CrossRef CAS PubMed.
- C. B. Garcia, D. Shi and T. J. Webster, Tat-functionalized liposomes for the treatment of meningitis: an in vitro study, Int. J. Nanomed., 2017, 12, 3009 CrossRef CAS PubMed.
- I. S. Zuhorn,
et al., Nonbilayer phase of lipoplex-membrane mixture determines endosomal escape of genetic cargo and transfection efficiency, Mol. Ther., 2005, 11, 801–810 CrossRef CAS PubMed.
- B. Ma, S. Zhang, H. Jiang, B. Zhao and H. Lv, Lipoplex morphologies and their influences on transfection efficiency in gene delivery, J. Controlled Release, 2007, 123, 184–194 CrossRef CAS PubMed.
- Ż Polańska,
et al., The Process of Binding and Releasing of Genetic Material from Lipoplexes Based on Trimeric Surfactants and Phospholipids, Int. J. Mol. Sci., 2021, 22(14), 7744 CrossRef PubMed.
- R. Koynova, Y. S. Tarahovsky, L. Wang and R. C. MacDonald, Lipoplex formulation of superior efficacy exhibits high surface activity and fusogenicity, and readily releases DNA, Biochim. Biophys. Acta, 2007, 1768, 375 CrossRef CAS PubMed.
- S. Grosse, Y. Aron, G. Thévenot, M. Monsigny and I. Fajac, Cytoskeletal involvement in the cellular trafficking of plasmid/PEI derivative complexes, J. Controlled Release, 2007, 122, 111–117 CrossRef CAS PubMed.
- R. Tachibana,
et al., An assessment of relative transcriptional availability from nonviral vectors, Int. J. Pharm., 2004, 270, 315–321 CrossRef CAS PubMed.
- D. Kalderon, B. L. Roberts, W. D. Richardson and A. E. Smith, A short amino acid sequence able to specify nuclear location, Cell, 1984, 39, 499–509 CrossRef CAS PubMed.
- S. R. Schwarze, In Vivo Protein Transduction: Delivery of a Biologically Active Protein into the Mouse, Science, 1999, 285, 1569–1572 CrossRef CAS PubMed.
- C.-H. Tung and R. Weissleder, Arginine containing peptides as delivery vectors, Adv. Drug Delivery Rev., 2003, 55, 281–294 CrossRef CAS PubMed.
- J.-B. Kim,
et al., Enhanced transfection of primary cortical cultures using arginine-grafted PAMAM dendrimer, PAMAM-Arg, J. Controlled Release, 2006, 114, 110–117 CrossRef CAS PubMed.
- E. E. Vaughan, J. V. DeGiulio and D. A. Dean, Intracellular Trafficking of Plasmids for Gene Therapy: Mechanisms of Cytoplasmic Movement and Nuclear Import, Curr. Gene Ther., 2006, 6, 671 CrossRef CAS PubMed.
- S. P. Loughran, C. M. McCrudden and H. O. McCarthy, Designer peptide delivery systems for gene therapy, Eur. J. Nanomed., 2015, 7, 85–96 CAS.
- H. Ma,
et al., Non-classical nuclear localization signal peptides for high efficiency lipofection of primary neurons and neuronal cell lines, Neuroscience, 2002, 112, 1–5 CrossRef CAS PubMed.
- J. M. Bergen, I.-K. Park, P. J. Horner and S. H. Pun, Nonviral Approaches for Neuronal Delivery of Nucleic Acids, Pharm. Res., 2008, 25, 983 CrossRef CAS PubMed.
- D. W. Dickson, Parkinson’s Disease and Parkinsonism: Neuropathology, Cold Spring Harbor Perspect. Med., 2012, 2(8), a009258 Search PubMed.
-
A. Kouli, K. M. Torsney and W.-L. Kuan, Parkinson's Disease: Etiology, Neuropathology, and Pathogenesis, in Parkinson's Disease: Pathogenesis and Clinical Aspects, Codon Publications, 2018 Search PubMed.
- Y. Zhang, F. Schlachetzki, Y.-F. Zhang, R. J. Boado and W. M. Pardridge, Normalization of Striatal Tyrosine Hydroxylase and Reversal of Motor Impairment in Experimental Parkinsonism with Intravenous Nonviral Gene Therapy and a Brain-Specific Promoter, Hum. Gene Ther., 2004, 15, 339–350 CrossRef CAS PubMed.
- R. J. Boado and W. M. Pardridge, The Trojan Horse Liposome Technology for Nonviral Gene Transfer across the Blood-Brain Barrier, J. Drug Delivery, 2011, 2011, 296151 Search PubMed.
- M. L. Arango-Rodriguez,
et al., Biophysical characteristics of neurotensin polyplex for in vitro and in vivo gene transfection, Biochim. Biophys. Acta, 2006, 1760, 1009–1020 CrossRef CAS PubMed.
- D. Martinez-Fong and I. Navarro-Quiroga, Synthesis of a non-viral vector for gene transfer via the high-affinity neurotensin receptor, Brain Res. Brain Res. Protoc. e.g. Brain research protocols, 2000, 6, 13–24 CrossRef CAS.
- J. A. Gonzalez-Barrios,
et al., Neurotensin polyplex as an efficient carrier for delivering the human GDNF gene into nigral dopamine neurons of hemiparkinsonian rats, Mol. Ther., 2006, 14, 857–865 CrossRef CAS PubMed.
- D. M. Yurek,
et al., Long-term transgene expression in the central nervous system using DNA nanoparticles, Mol. Ther., 2009, 17, 641–650 CrossRef CAS PubMed.
- L. F. Razgado-Hernandez,
et al., The Transfection of BDNF to Dopamine Neurons Potentiates the Effect of Dopamine D3 Receptor Agonist Recovering the Striatal Innervation, Dendritic Spines and Motor Behavior in an Aged Rat Model of Parkinson’s Disease, PLoS One, 2015, 10(2), e0117391 CrossRef PubMed.
- N. G. Hernandez-Chan,
et al., Neurotensin-polyplex-mediated brain-derived neurotrophic factor gene delivery into nigral dopamine neurons prevents nigrostriatal degeneration in a rat model of early Parkinson’s disease, J. Biomed. Sci., 2015, 22(1), 59 CrossRef PubMed.
- K. Aldape, G. Zadeh, S. Mansouri, G. Reifenberger and A. von Deimling, Glioblastoma: pathology, molecular mechanisms and markers, Acta Neuropathol., 2015, 129(6), 829–848 CrossRef CAS PubMed.
- A. Omuro, Glioblastoma and Other Malignant Gliomas, J. Am. Med. Assoc., 2013, 310, 1842 CrossRef CAS PubMed.
- S. Liu,
et al., Gene and doxorubicin co-delivery system for targeting therapy of glioma, Biomaterials, 2012, 33, 4907–4916 CrossRef CAS PubMed.
- P. Kumthekar,
et al., A first-in-human phase 0 clinical study of RNA interference–based spherical nucleic acids in patients with recurrent glioblastoma, Sci. Transl. Med., 2021, 13, eabb3945 CrossRef CAS PubMed.
- E. K. Stachowiak, X. Fang, J. Myers, S. Dunham and M. K. Stachowiak, cAMP-induced differentiation of human neuronal progenitor cells is mediated by nuclear fibroblast growth factor receptor-1 (FGFR1), J. Neurochem., 2003, 84, 1296–1312 CrossRef CAS PubMed.
- B. Lacar, S. Z. Young, J.-C. Platel and A. Bordey, Imaging and Recording Subventricular Zone Progenitor Cells in Live Tissue of Postnatal Mice, Front. Neurosci., 2010, 4, 43 Search PubMed.
- M. K. Stachowiak, P. A. Maher and E. K. Stachowiak, Integrative nuclear signaling in cell development–a role for FGF receptor-1, DNA Cell Biol., 2007, 26(12), 811–826 CrossRef CAS PubMed.
- D. J. Bharali,
et al., Organically modified silica nanoparticles: A nonviral vector for in vivo gene delivery and expression in the brain, Proc. Natl. Acad. Sci. U. S. A., 2005, 102, 11539–11544 CrossRef CAS PubMed.
- A. Wegener,
et al., Gain of Olig2 function in oligodendrocyte progenitors promotes remyelination, Brain, 2015, 138, 120–135 CrossRef PubMed.
- W. Niu,
et al., SOX2 reprograms resident astrocytes into neural progenitors in the adult brain, Stem Cell Rep., 2015, 4, 780–794 CrossRef CAS PubMed.
- C. Heinrich,
et al., Directing astroglia from the cerebral cortex into subtype specific functional neurons, PLoS Biol., 2010, 8, e1000373 CrossRef PubMed.
- C. L. Maire,
et al., Directing human neural stem/precursor cells into oligodendrocytes by overexpression of Olig2 transcription factor, J. Neurosci. Res., 2009, 87, 3438–3446 CrossRef CAS PubMed.
- X. Li,
et al., Nanoparticle-mediated conversion of primary human astrocytes into neurons and oligodendrocytes, Biomater. Sci., 2016, 4, 1100 RSC.
- J. C. Sunshine, M. I. Akanda, D. Li, K. L. Kozielski and J. J. Green, Effects of base polymer hydrophobicity and end-group modification on polymeric gene delivery, Biomacromolecules, 2011, 12, 3592–3600 CrossRef CAS PubMed.
- S. Gao,
et al., A non-viral suicide gene delivery system traversing the blood brain barrier for non-invasive glioma targeting treatment, J. Controlled Release, 2016, 243, 357–369 CrossRef CAS PubMed.
|
This journal is © The Royal Society of Chemistry 2022 |