DOI:
10.1039/D0QM01006E
(Research Article)
Mater. Chem. Front., 2021,
5, 3388-3395
A dentin hypersensitivity treatment using highly stable photothermal conversion nanoparticles†
Received
3rd December 2020
, Accepted 18th February 2021
First published on 22nd February 2021
Abstract
Most of the currently available commercial photothermal agents suffer from issues in terms of photothermal instability and low photothermal conversion efficiency (PCE), which indeed impair their practical applications in disease treatment. Herein, a type of biocompatible organic nanoparticles (namely NDTB NPs) for an effective photothermal treatment with a high PCE of 40.6% was developed, which showed far higher photothermal stability compared with that of the clinically used indocyanine green. In addition, NDTB NPs combined with laser are significantly more effective than only laser irradiation in phototheranostic dental hypersensitivity treatments. This study thus provides an insight into the development of advanced photothermal NPs for practical phototheranostic applications.
Introduction
Dentin hypersensitivity (DH) is a growing oral disease, which affects the health of people worldwide.1 It is a painful symptom characterized by short, sharp pain arising from exposed dentin in response to various stimuli. Several strategies have been introduced to eliminate the discomforts of patients, such as chemical or physical occlusion, nerve desensitization, or photo-biomodulation (low-level light therapy).2 However, none of the treatments are effective and definitive enough. More recently, laser irradiation for DH treatment has attracted extensive attention and has been widely investigated due to its advantages such as pain-free, safe, vibration free and convenient.3,4 However, the major concern of the DH laser irradiation treatment is the high potential of the whole tissue overheating, which may cause different levels of damage to the dental pulp.5 Furthermore, the dentinal tubules cannot be occluded completely using laser only, resulting in inefficiently reduced DH.6,7 In order to further improve the desensitization effects, the combination of other desensitizing agents with laser has become a research hotspot.8,9
Recent years have witnessed considerable efforts in the development of photothermal agents.10,11 Photothermal agents are widely used in optional biochemical treatments, particularly in the field of phototherapy in vitro and in vivo, due to their unique properties.12,13 Among the existing photothermal materials, although organic small molecules/polymers take advantages of potential biodegradability, environmental friendliness, facile modification and good processability, there are two intractable issues that remain to be resolved.14–16 One serious issue is the low efficiency of photothermal conversion, and the other is the awkward instability problem. For instance, indocyanine green (ICG), which is widely used as the intermediate for light-mediated biomedical applications in clinics, suffers from the untrusted theranostic outcome caused by its poor stability.17 Despite the development of a series of small molecules/polymer photothermal agents with high photothermal conversion efficiency by numerous research groups including ours,18–22 the development of highly stable photothermal agents in aqueous solutions for effective photothermal therapy applications is still desired because most of the small molecule/polymer photothermal agents are hydrophobic or unstable in aqueous systems.23,24 To overcome such barriers, some exogenously amphipathic nano-carriers, such as PEG-b-PPG-b-PEG (F127) and 1,2-distearoyl-sn-glycero-3-phosphoethanolamine-N-[methoxy-(polyethylene glycol)-2000] (MPEG2000-DSPE), were used as the doping matrix to prepare biocompatible nanoparticles (NPs) for improving stabilities in terms of aqueous dispersion stability and photothermal stability.25,26 Amphiphilic polymer co-assembled nanoparticles, which can simply combine the advantages of small molecules/polymers and amphiphilic polymer matrix, have attracted extensive interest and been widely investigated due to their excellent advantages of having superb biocompatibility, low toxicity, high reproducibility, and so forth.27,28
Herein, photothermal agents were introduced to heat the dentin surface locally rather than the whole dentin. We developed a photothermal agent with a high photothermal conversion efficiency named NDTB based on benzo[1,2-c:4,5-c′]bis([1,2,5]thiadiazole) (BBTD), which is a low-band-gap electron acceptor. In addition, naphthalene and methoxy substituted triphenylamine as well as the 2-ethylhexyl substituted thiophene were introduced as the electron donors, which not only provided a larger steric hinderance to prevent the molecular packing too strongly but also strengthened the capacity of electronic donation. In addition, the copolymer MPEG2000-DSPE was selected as the doping matrix to form NDTB nanoparticles (NDTB NPs) for improving the stabilities and extending the molecules to aqueous media. The in vitro effects of NDTB NPs on the DH treatment were studied with the dentin disc model (Scheme 1). To the best of our knowledge, the significantly synergistic effects on the dentin tubule occlusion by the combination of organic photothermal nanoparticles with diode laser was confirmed for the first time, which may provide a potential approach to develop a suitable application of photothermal agents.
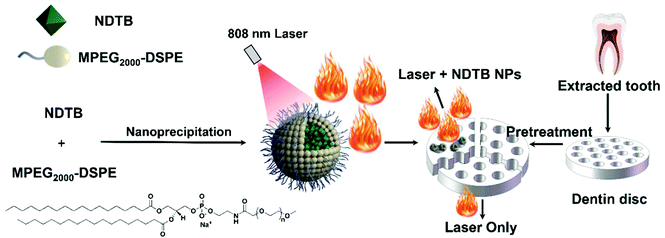 |
| Scheme 1 Schematic of the preparation and application of NDTB NPs via a nanoprecipitation method. | |
Results and discussion
To obtain the target product, the synthetic route of compound NDTB is shown in Scheme 2. We synthesized by a few steps of classical reactions such as Suzuki–Miyaura coupling and Stille coupling. Compound NDTB was obtained in high yield and characterized via1H NMR, 13C NMR and matrix-assisted laser desorption time of flight mass spectrometry (MALDI-TOF) (Fig. S1–S7, ESI†).
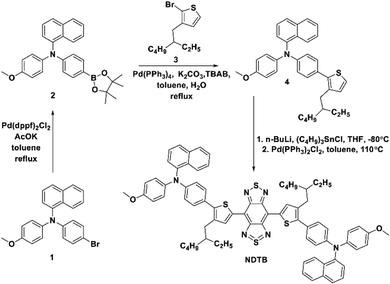 |
| Scheme 2 Synthetic routes to NDTB. | |
To explore more structural information, first-principles density functional theory calculations were performed. The results were obtained using the Gaussian 09W program at the B3LYP/6-31G(d) level (Fig. 1a and b). The optimized geometric structure of NDTB on the S0 energy level reveals that the planar structure is found around the core, while a more distorted geometry is found between the naphthalene/methoxy substituted triphenylamine and the molecular backbone. In addition, the lowest unoccupied molecular orbital (LUMO) is almost localized on the strong electronic acceptor BBTD, which possesses low band gap and large electron delocalization. The highest occupied molecular orbital (HOMO) is delocalized along the conjugated structure. Theoretical investigations demonstrated that the spatial separation of HOMO and LUMO could promote the formation of the twisted intramolecular charge transfer (TICT) state in the long conjugated donor–π bridge–acceptor–π bridge–donor (D–π–A) system.29,30 In addition, the formation of the dark TICT state could favourably promote the heat generation.31–33 The HOMO/LUMO energy levels of NDTB are −4.44 eV/−3.21 eV, and the HOMO and LUMO energy gap is 1.23 eV, which allows for the strong absorption band appearing in the red-shift wavelength region. Furthermore, the photophysical properties of NDTB was preliminarily evaluated (Fig. 1c). A long wavelength absorption was observed from 600 nm to 1000 nm in THF, which can be assigned to the low-energy charge transfer (CT) band. Furthermore, an emission peak of about 1100 nm was found in the second NIR range, which was attributed to the low band gap and the intensive electron deficient structure. We further measured the photoluminescence intensity in different THF/water ratios. As for NDTB, tetrahydrofuran (THF) is a good solvent and water is a poor solvent. The photoluminescence intensity of NDTB gradually decreases with the increase in the water fraction (fw) (Fig. 1d). It is noteworthy to mention that the photoluminescence signal of NDTB is hardly detected in the fw = 90% mixture solution, which suggests the aggregation of NDTB could probably favor numerous nonradiative quenching processes. Thus, NDTB in the aggregate state, particularly in NPs is expected to enable better photothermal properties.
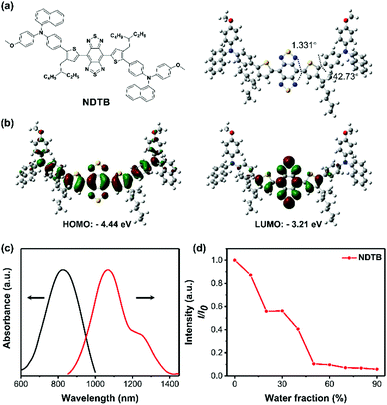 |
| Fig. 1 (a) Chemical structure (left) and optimized ground state geometries (right) of NDTB. (b) Calculated HOMO (left) and LUMO (right) of NDTB. (c) Normalized absorption and photoluminescence intensity spectra of NDTB in tetrahydrofuran (THF). (d) Variation in the PL intensity (I/I0) with water fraction in THF/water mixtures (I0 = the maximal PL intensity of different ratios of the THF/water-mixed solutions). | |
In order to maximize the photothermal property and apply it to the biomedical aqueous system, NDTB was transformed into NPs using copolymer MPEG2000-DSPE as the doping matrix by the classical nanoprecipitation method (Scheme 1). A subglobose micromorphology was observed via transmission electron microscopy (TEM), and subsequently, dynamic light scattering (DLS) reveals that NDTB NPs were successfully prepared with a mean diameter of about 110 nm (Fig. 2a and b), which revealed that the nanoparticles were dispersed well in the aqueous system. Moreover, NDTB NPs could be stored at 4 °C for more than 15 days without any obvious change in their diameters (Fig. S8, ESI†). We further monitored the heating behaviours of NDTB NPs at different concentrations as a function of irradiation time, which demonstrate that the photothermal effect is positively related to the concentration of NDTB NPs (Fig. 2c). The temperature of the NDTB NP solution reaches a plateau after 2 min irradiative activation, while it increases rapidly at the beginning. Notably, the aqueous solution displays a 65 °C temperature increase (ΔT) with only 40 μM NDTB NPs (based on the dye molecule), which reaches a maximum at 93.1 °C after 808 nm laser irradiation (660 mW cm−2) for 3 min. Indocyanine green (ICG) is a classical near-infrared dye used in clinical applications, which was approved by the Food and Drug Administration (FDA) of America. ICG was considered as an ideal theranostic platform for clinical study due to its good properties of photothermal therapy, photodynamic therapy and so forth. However, the photothermal stabilities of the photothermal agents are essential for clinical treatments in living systems as poor therapeutic efficacy and even harmful side effects would exist if the chemical structure is destroyed.34,35 The photothermal stabilities of ICG and NDTB NPs were evaluated under continuous 808 nm laser irradiation (660 mW cm−2) by detecting the absorbance spectra after irradiating for different time periods. As shown in Fig. 2d and e, the colours and the absorbance of NDTB NPs remained almost unchanged during 20 min irradiation, whereas the dark-green colour of ICG solutions turn to dark yellow-green colour gradually and the maximal absorbance of ICG dropped to nearly 50%. The results indicate that NDTB NPs possess excellent anti-photobleaching performance compared to ICG, which is widely used in clinical research. For quantitative application, the standard curve of absorbance at 808 nm with a correlation coefficient of 0.99877 was determined, suggesting that the absorption of NDTB NPs possesses a good linear relationship with its concentration (Fig. S9, ESI†). In addition, the correlation of the ICG concentration with absorbance was measured as well (Fig. S10, ESI†).
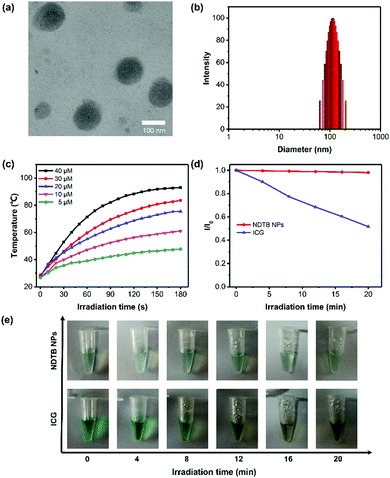 |
| Fig. 2 (a) Transmission electron microscope images of NDTB NPs (scale bar = 100 nm). (b) Dynamic light scattering profile of NDTB NPs. (c) Photothermal conversion behaviour of NDTB NPs at different concentrations (5–40 μM) under 808 nm light (660 mW cm−2) irradiation. (d) Plot of I/I0versus various irradiation time. I and I0 are the maximal NIR absorption intensity of NDTB NPs and ICG in PBS solutions after and before laser irradiation, respectively. (e) Photographs of NDTB NPs and ICG in PBS solutions after 808 nm light irradiation for different time. | |
To intuitively evaluate the photothermal conversion property, ICG was selected as the control and its temperature increase was compared with NDTB NPs upon the 808 nm laser irradiation as well. As depicted in Fig. 3a, NDTB NPs display a superior photothermal performance because of the higher plateau temperature and rapid temperature increase rate. The photothermal conversion efficiency (PCE) at 808 nm of NDTB NPs is calculated to be 40.6%, while the PCE of ICG is 33.1%, according to the method reported in the literature.36–38 Photothermal agents used in numerous biomedical applications often require heating and cooling for several cycles. Thus, we evaluated the anti-photobleaching properties of NDTB NPs and ICG for 5 irradiative cycles (the samples were irradiated by 808 nm laser for 3 min, and subsequently cooled them down to environmental temperature in 8 min), respectively. Notably, the photothermal behaviour of NDTB NPs was scarcely influenced. The temperature increase in NDTB NPs can be easily achieve over 60 °C after five irradiation cycles (Fig. 3b), while the temperature increase in ICG significantly dropped to near 30% (ΔT = ∼20 °C) of the initial value (ΔT = ∼60 °C) after only two irradiation cycles. In addition, the infrared (IR) thermal images intuitively reveal that the ICG lost the photothermal conversion ability gradually, while NDTB NPs showed superior stable photothermal conversion behaviour after five irradiation cycles (Fig. 3c). These findings demonstrate that NDTB NPs possess a great potential for serving as a light-mediated thermal candidate.
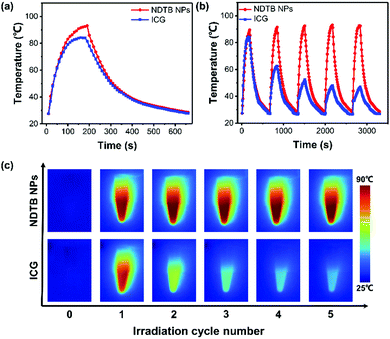 |
| Fig. 3 (a) Comparison of the photothermal conversion behaviors of NDTB NPs and ICG in the PBS solution at the same concentration (40 μM). (b) Anti-photobleaching property of NDTB NPs and ICG (40 μM) during five cycles of heating–cooling processes. (c) IR thermal images of NDTB NPs and ICG in PBS solutions (40 μM) under 808 nm laser irradiation for different cycle numbers. | |
Encouraged by the excellent photothermal conversion efficiency, photothermal stabilities and photobleaching stabilities of NDTB NPs, we further investigated the performance of the DH treatment. Fifteen impacted third molars of mature human with no caries were collected with ethical approval and informed consent. Fifteen disc-shaped samples were fabricated by the horizontal sectioning of the middle crown area of collected teeth using a low-speed saw. After that, the dentin discs were treated with 35% phosphoric acid to clear the dentin surfaces. The dentin tubular micrographs were captured via scanning electron microscopy (SEM) (Fig. 4a and b). The SEM images of the cross section and vertical section reveal that the dentin surfaces were smooth and all dentinal tubules were distributed well. The dentin tubules were open and empty without the smear layer before the treatment, suggesting the successful preparation of standard dentin hypersensitivity models. After that, the dentin discs were randomly assigned into two groups as follows: 808 nm diode laser irradiation (laser) and 808 nm diode laser irradiation combined with NDTB NPs (NDTB NPs + laser). Then, the NDTB NPs (100 μM) was evenly applied to the dentin discs, and we subsequently evaluated the treating effects of the “NDTB NPs + Laser” group and “Laser Only” group by alternative heating and cooling processes (the dentinal tubules were irradiated by 808 nm diode laser for 5 s, and then the laser was removed to cool down the samples for another 5 s. And the process was repeated for 9 times). The SEM images of the “NDTB NPs + Laser” group indicate that almost all the dentin tubular orifices were significantly closed or irregularly narrowed to the tubular centre and the occlusion in dentin tubules were uniformly dense. Moreover, the “Laser Only” group shows that few dentin tubular orifices were closed with a thin layer of sealed dentine and fissures appear between the occluded areas and tubules. The results confirmed the excellent synergistic effects of laser combined with NDTB NPs and the efficiency of the DH treatment.
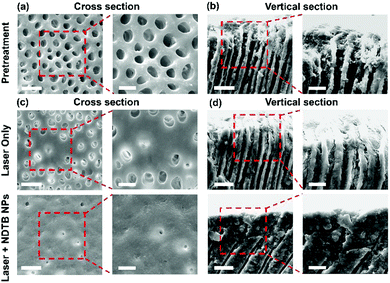 |
| Fig. 4 (a) Cross section SEM images of dentin discs after pretreatment (scale bar = 10 μm). (b) Vertical section SEM images of dentin discs after pretreatment (scale bar = 10 μm). (c) Cross section SEM images of dentin discs after treatment for the “Laser Only” group (top) and “Laser + NDTB NPs” group (down) (scale bar = 10 μm). (d) Vertical section SEM images of dentin discs after treatment for the “Laser Only” group (top) and “Laser + NDTB NPs” group (down) (scale bar = 10 μm). | |
The occlusion rate (%) and occlusion depth (μM) of dentin tubules were extracted by Image-pro plus 6.0 and subsequently analyzed by statistic software SPSS 21.0 (Fig. 5a and b). Collectively, the occlusion rate of the “Laser + NDTB NPs” group was obviously raised with a mean rate at about 86%, while the mean rate of the “Laser Only” group was about 57%. Furthermore, the occlusion depth of the “Laser + NDTB NPs” group was significantly increased with a mean depth of about 10.74 μm, while the mean depth of the “Laser Only” group was about 7.48 μm. It is noteworthy to mention that the damage of dental pulp is the major concern of the laser irradiation DH treatment resulted from the tissue overheating. Thus, we monitored the temperature of the irradiated dentin surface and the opposite side. The results display that the irradiated side temperature of the “Laser + NDTB NPs” group reaches a maximum at about 60 °C, while the irradiated side temperature of the “Laser Only” group is below 40 °C (Fig. 5c and d). Furthermore, both the “Laser + NDTB NPs” group and “Laser Only” group show a low temperature increase (ΔT < 3 °C), which is an acceptable temperature for sensitive dental pulp. Further considering the excellent photothermal conversion efficiency of NDTB NPs (Fig. 3a), and the good therapeutic effects of the DH treatment (Fig. 4c and d), it is reasonable to conclude that NDTB NPs is a type of highly suitable phototheranostic nanoagent with noticeable synergistic effects on the DH treatment. Further, to evaluate the biocompatibility of NDTB NPs, we first conducted the 3-(4,5-dimethylthiazol-2-yl)-2,5-diphenyltetrazolium bromide (MTT) cytotoxicity assay, which shows negligible toxicity (Fig. S11, ESI†). The cytotoxicity result towards HPDLF (human periodontal ligament fibroblasts) cells in vitro, as shown in the supporting information. NDTB NPs at a series of concentrations from 12.5 to 200 μM had negligible cytotoxicity towards HPDLF cells with cell viability of higher than 85%, which indicates that NDTB NPs possess good biocompatibility.
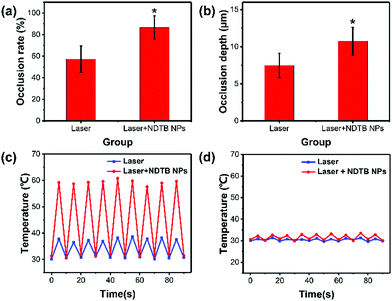 |
| Fig. 5 (a) Quantitative analyse of the occlusion rate of dentin tubules between the “Laser Only” group and “Laser + NDTB NPs” group (n = 15, *P < 0.05). (b) Quantitative analyses of the occlusion depth of dentin tubules between the “Laser Only” group and “Laser + NDTB NPs” group (n = 15, *P < 0.05). (c) The irradiated side temperature increase in the “Laser Only” group and “Laser + NDTB NPs” group. (d) The opposite side temperature increase in the “Laser Only” group and “Laser + NDTB NPs” group. | |
Conclusions
In summary, we have developed a NDTB NPs for effective photothermal combination DH therapy using copolymer MPEG2000-DSPE as the doping matrix. Notably, the MPEG2000-DSPE doped NDTB nanoparticles exhibit a high photothermal conversion efficiency (40.6%) upon 808 nm laser irradiation due to the intensive absorption of NDTB NPs as well as the excellent photothermal stabilities and anti-photobleaching stabilities, which are far better than the most extensively applied ICG in clinics. The NDTB NPs also display excellent synergistic effects on the DH treatment. The DH treatment with the dentin disc model reveals that the “Laser + NDTB NPs” gives impressive performance in dental occlusion accompanied with the increased occlusion rate (86%) and occlusion depth (10.74 μm). This study will inspire more exciting research on the development of photothermal agents for various disease treatment.
Experimental section
Preparation of dentin specimen
In total, fifteen impacted mature human third molars with no caries were collected with ethical approval and informed consent. According to the literature,39,40 the teeth were immersed in a 0.5% chloramine T solution for one week for disinfection. Disc-shaped samples were fabricated by the horizontal sectioning of the middle crown area of collected teeth using a low-speed saw. All discs were prepared with a uniform thickness of 1.5 mm, and unqualified discs were excluded. Then, the dentin surfaces were polished with a 1000-mesh diamond silicon carbide sandpaper. After that, the dentin discs were ultrasonically washed in deionized water for 2 min, followed by cleaning with huge amount of deionized water. After that, the dentin discs were treated with 35% phosphoric acid for 2 min. A large amount of deionized water was used to wash dentin slices and phosphoric acid was removed. Then, the dentin discs were stored in deionized water at 4 °C for later use.
Preparation and experimental design of dentin slice samples
Each dentin disc was divided into three segments with high-speed diamond burs under water coolant. The three segments were randomly assigned into three groups as follows: pre-treatment group: no intervention. “Laser Only” group: 808 nm diode laser irradiation. “Laser + NDTB NPs” group: 808 nm diode laser irradiation combined with NDTB nanoparticles. 100 μM was used as the suitable concentration of the NDTB nanoparticles. The distance between the 808 nm diode laser fiber and dentin slices is 2 mm. The laser energy density value is 247.5 J cm−2. A near-infrared thermal imager was used to record the temperature changes on the surface of dentin slices and on the opposite side. “Laser Only” group: the dentin slices were irradiated by the 808 nm diode laser (10 Hz, 660 mW) for 5 s and repeated for 9 times. “Laser + NDTB NPs” group: the surfaces of the dentin slices were treated with NDTB nanoparticle material for 2 min.41 Then, the dentin slices were processed in the same way with the “Laser Only” group. After 2 min of ultrasonic washing, the samples were placed at natural room temperature until dried and prepared for analysis by SEM.
Scanning electron microscopic (SEM) observation
The discs were mounted onto aluminium stubs and subsequently coated with a thin layer of gold in a sputter coater. Photographs of the samples were obtained from the camera, which was fixed to SEM. The closure of the dentin tubule of the samples were scanned and examined using SEM (FEI QUANTA 200, FEI, Holland). Three visual fields close to tooth enamel in the cross-sectional sample of dentin tubule were selected to obtain forty-five visual fields in each group. In addition, five visual fields of the vertical section samples of dentin tubules in each group was randomly selected.
Cell culture
Human periodontal ligament fibroblast was cultured in the Dulbecco's Modified Eagle's Medium (DMEM) containing 10% fetal bovine serum (FBS) and 1% penicillin–streptomycin at 37 °C in a humidified environment containing 5% CO2.
Cytotoxicity study
3-(4,5-Dimethylthiazol-2-yl)-2,5-diphenyltetrazolium bromide (MTT) assays were employed to estimate the biocompatibility of the NDTB NPs against HPDLF cells. In short, the cells were exposed to a series of doses of NDTB NPs at 37 °C. After 12 h of incubation, the sample wells were washed twice with PBS buffer and 100 μL of freshly prepared MTT solution (0.5 mg mL−1) in the culture medium was added into each sample well. The MTT medium solution was carefully removed after 3 h incubation in the incubator for the sample wells. DMSO (100 μL) was then added into each well and the plate was shaken for 5 min at room temperature to dissolve all the precipitates formed. The absorbance of individual wells at 570 nm was then monitored using a microplate reader (Genios Tecan). The biocompatibility was expressed by the ratio of the absorbance of MTT in the sample wells to that of the cells incubated with the culture medium only.
Conflicts of interest
There are no conflicts to declare.
Acknowledgements
This work was financially supported by the National Natural Science Foundation of China (51961160730 and 51873092), the National Key R&D Program of China (Intergovernmental cooperation project, 2017YFE0132200), Tianjin Science Fund for Distinguished Young Scholars (19JCJQJC61200), the Natural Science Foundation of Tianjin City (18JCYBJC27000), State Key Laboratory of Medicinal Chemical Biology (2018012), and the Fundamental Research Funds for the Central Universities, Nankai University.
Notes and references
- L. F. Zeola, P. V. Soares and J. Cunha-Cruz, Prevalence of dentin hypersensitivity: Systematic review and meta-analysis, J. Dent., 2019, 81, 1–6 CrossRef PubMed.
- V. Moraschini, L. S. da Costa and G. O. Dos Santos, Effectiveness for dentin hypersensitivity treatment of non-carious cervical lesions: a meta-analysis, Clin. Oral Investig., 2018, 22, 617–631 CrossRef PubMed.
- G. F. Moura, L. F. Zeola, M. B. Silva, S. C. Sousa, F. R. Guedes and P. V. Soares, Four-session protocol effectiveness in reducing cervical dentin hypersensitivity: A 24-week randomized clinical trial, Photobiomodulation, Photomed., Laser Surg., 2019, 37, 117–123 CrossRef PubMed.
- R. Praveen, S. Thakur, M. Kirthiga and M. Narmatha, Comparative evaluation of a low-level laser and topical desensitizing agent for treating dentinal hypersensitivity: A randomized controlled trial, J. Conservative Dent., 2018, 21, 495–499 CrossRef CAS PubMed.
- J. Lakhani, V. Agrawal, R. Mahant, S. Kapoor, D. Vaghamshi and A. Shah, Pulpal temperature rise: Evaluation after light activation of newer pulp-capping materials and resin composite, Contemp. Clin. Dent., 2018, 9, 644–648 CrossRef PubMed.
- F. Sgolastra, A. Petrucci, M. Severino, R. Gatto and A. Monaco, Lasers for the treatment of dentin hypersensitivity: a meta-analysis, J. Dent. Res., 2013, 92, 492–499 CrossRef CAS PubMed.
- S. K. Tunc, N. Z. Yayli, A. C. Talmac, E. Feslihan and D. Akbal, Clinical comparison of the use of ER, CR: YSGG and diode lasers in second stage implants surgery, Saudi. Med. J., 2019, 40, 490–498 CrossRef PubMed.
- Y. Kong, Y. Lei, S. Li, Y. Zhang, J. Han and M. Hu, Network meta-analysis of the desensitizing effects of lasers in patients with dentine hypersensitivity, Clin. Oral Investig., 2020, 24, 1917–1928 CrossRef PubMed.
- M. L. Hu, G. Zheng, J. M. Han, M. Yang, Y. D. Zhang and H. Lin, Effect of Lasers on Dentine Hypersensitivity: Evidence From a Meta-analysis, J. Evid.-Based Dent. Pract., 2019, 19, 115–130 CrossRef PubMed.
-
(a) W. Wu and Z. Li, Nanoprobes with aggregation-induced emission for theranostics, Mater. Chem. Front., 2021, 5, 603–626 RSC;
(b) M. Li, Z. Luo and Y. Zhao, Recent advancements in 2D nanomaterials for cancer therapy, Sci. China: Chem., 2018, 61, 1214–1226 CrossRef CAS.
- Z. Liu, Q. Xu, Y. Li and W. Chen, Fluorescent C-dot nano-composites as efficient photothermal agents and multi-modal imaging tracers, Mater. Chem. Front., 2017, 1, 538–541 RSC.
- K. K. Ng and G. Zheng, Molecular interactions in organic nanoparticles for photo-theranostic applications, Chem. Rev., 2015, 115, 11012–11042 CrossRef CAS PubMed.
- L. Cheng, C. Wang, L. Feng, K. Yang and Z. Liu, Functional nanomaterials for phototherapies of cancer, Chem. Rev., 2014, 114, 10869–10939 CrossRef CAS PubMed.
- K. Pu, J. Mei, J. V. Jokerst, G. Hong, A. L. Antaris, N. Chattopadhyay, A. J. Shuhendler, T. Kurosawa, Y. Zhou, S. S. Gambhir, Z. N. Bao and J. H. Rao, Adv. Mater., 2015, 27, 5184–5190 CrossRef CAS PubMed.
- D. Zhang, G. B. Qi, Y. X. Zhao, S. L. Qiao, C. Yang and H. Wang, In Situ Formation of Nanofibers from Purpurin18-Peptide Conjugates and the Assembly Induced Retention Effect in Tumor Sites, Adv. Mater., 2015, 27, 6125–6130 CrossRef CAS PubMed.
- U. S. Dinish, Z. Song, C. J. H. Ho, G. Balasundaram, A. B. E. Attia, X. Lu, B. Z. Tang, B. Liu and M. Olivo, Single Molecule with Dual Function on Nanogold: Biofunctionalized Construct for In Vivo Photoacoustic Imaging and SERS Biosensing, Adv. Funct. Mater., 2015, 25, 2316–2325 CrossRef CAS.
- Z. H. Sheng, D. H. Hu, M. B. Zheng, P. F. Zhao, H. L. Liu, D. Y. Gao, P. Gong, G. H. Gao, P. F. Zhang, Y. F. Ma and L. T. Cai, Smart Human Serum Albumin-Indocyanine Green Nanoparticles Generated by Programmed Assembly for Dual-Modal Imaging-Guided Cancer Synergistic Phototherapy, ACS Nano, 2014, 8, 12310–12322 CrossRef CAS PubMed.
- J. Li and K. Pu, Development of organic semiconducting materials for deep-tissue optical imaging, phototherapy and photoactivation, Chem. Soc. Rev., 2019, 48, 38–71 RSC.
- J. Qi, Y. Fang, R. T. K. Kwok, X. Y. Zhang, X. L. Hu, J. W. Y. Lam, D. Ding and B. Z. Tang, Highly Stable Organic Small Molecular Nanoparticles as an Advanced and Biocompatible Phototheranostic Agent of Tumor in Living Mice, ACS Nano, 2017, 11, 7177–7188 CrossRef CAS PubMed.
- Q. Wang, B. Xia, J. Xu, X. Niu, J. Cai, Q. Shen, W. Wang, W. Huang and Q. Fan, Biocompatible small organic molecule phototheranostics for NIR-II fluorescence/photoacoustic imaging and simultaneous photodynamic/photothermal combination therapy, Mater. Chem. Front., 2019, 3, 650–655 RSC.
- X. Cai, J. Liu, W. H. Liew, Y. Duan, J. Geng, N. Thakor, K. Yao, L.-D. Liao and B. Liu, Organic molecules with propeller structures for efficient photoacoustic imaging and photothermal ablation of cancer cells, Mater. Chem. Front., 2017, 1, 1556–1562 RSC.
- K. Wang, K. Amin, Z. An, Z. Cai, H. Chen, H. Chen, Y. Dong, X. Feng, W. Fu, J. Gu, Y. Han, D. Hu, R. Hu, D. Huang, F. Huang, F. Huang, Y. Huang, J. Jin, X. Jin, Q. Li, T. Li, Z. Li, Z. Li, J. Liu, J. Liu, S. Liu, H. Peng, A. Qin, X. Qing, Y. Shen, J. Shi, X. Sun, B. Tong, B. Wang, H. Wang, L. Wang, S. Wang, Z. Wei, T. Xie, C. Xu, H. Xu, Z. Xu, B. Yang, Y. Yu, X. Zeng, X. Zhan, G. Zhang, J. Zhang, M. Q. Zhang, X. Zhang, X. Zhang, Y. Zhang, Y. Zhang, C. Zhao, W. Zhao, Y. Zhou, Z. Zhou, J. Zhu, X. Zhu and B. Z. Tang, Advanced functional polymer materials, Mater. Chem. Front., 2020, 4, 1803–1915 RSC.
- A. Zerda, S. Bodapati, R. Teed, S. Y. May, S. M. Tabakman, Z. Liu, B. T. Khuri-Yakub, X. Chen, H. Dai and S. S. Gambhir, Family of enhanced photoacoustic imaging agents for high-sensitivity and multiplexing studies in living mice, ACS Nano, 2012, 6, 4694–4701 CrossRef PubMed.
- A. P. Gorka, R. R. Nani, J. Zhu, S. Mackem and M. J. A. Schnermann, A Near-IR Uncaging Strategy Based on Cyanine Photochemistry, J. Am. Chem. Soc., 2014, 136, 14153–14159 CrossRef CAS PubMed.
- J. Li and K. Pu, Semiconducting Polymer Nanomaterials as Near-Infrared Photoactivatable Protherapeutics for Cancer, Acc. Chem. Res., 2020, 53, 752–762 CrossRef CAS PubMed.
- J. Li, D. Cui, Y. Jiang, J. Huang, P. Cheng and K. Pu, Near-Infrared Photoactivatable Semiconducting Polymer Nanoblockaders for Metastasis-Inhibited Combination Cancer Therapy, Adv. Mater., 2019, 31, 1905091 CrossRef CAS PubMed.
- Y. Cai, P. Liang, Q. Tang, X. Yang, W. Si, W. Huang, Q. Zhang and X. Dong, Diketopyrrolopyrrole-Triphenylamine Organic Nanoparticles as Multifunctional Reagents for Photoacoustic Imaging-Guided Photodynamic/Photothermal Synergistic Tumor Therapy, ACS Nano, 2017, 11, 1054–1063 CrossRef CAS PubMed.
- S. Zhang, W. Guo, J. Wei, C. Li, X. J. Liang and M. Yin, Terrylenediimide-Based Intrinsic Theranostic Nanomedicines with High Photothermal Conversion Efficiency for Photoacoustic Imaging-Guided Cancer Therapy, ACS Nano, 2017, 11, 3797–3805 CrossRef CAS PubMed.
- S. Sasaki, G. P. C. Drummen and G. I. Konishi, Recent advances in twisted intramolecular charge transfer (TICT) fluorescence and related phenomena in materials chemistry, J. Mater. Chem. C, 2016, 4, 2731–2743 RSC.
- Z. R. Grabowski, K. Rotkiewicz and W. Rettig, Structural Changes Accompanying Intramolecular Electron Transfer: Focus on Twisted Intramolecular Charge-Transfer States and Structures, Chem. Rev., 2003, 103, 3899–4032 CrossRef PubMed.
- S. J. Liu, X. Zhou, H. K. Zhang, H. L. Ou, J. W. Y. Lam, Y. Liu, L. Q. Shi, D. Ding and B. Z. Tang, Molecular Motion in Aggregates: Manipulating TICT for Boosting Photothermal Theranostics, J. Am. Chem. Soc., 2019, 141, 5359–5368 CrossRef CAS PubMed.
- W. H. Zhu, A new strategy enabling intramolecular motion to obtain advanced photothermal materials, Sci. China: Chem., 2019, 62, 659–661 CrossRef CAS.
- H. Ou, S. Dai, R. Liu and D. Ding, Manipulating the intramolecular motion of AIEgens for boosted biomedical applications, Sci. China: Chem., 2019, 62, 929–932 CrossRef CAS.
- E. Engel, R. Schraml, T. Maisch, K. Kobuch, B. Konig, R. M. Szeimies, J. Hillenkamp, W. Baumler and R. Vasold, Light-Induced Decomposition of Indocyanine Green, Invest. Ophthalmol. Visual Sci., 2008, 49, 1777–1783 CrossRef PubMed.
- J. Weber, P. C. Beard and S. E. Bohndiek, Contrast agents for molecular photoacoustic imaging, Nat. Methods, 2016, 13, 639–651 CrossRef CAS PubMed.
- Y. Lyu, C. Xie, S. A. Chechetka and E. Miyako, Semiconducting Polymer Nanobioconjugates for Targeted Photothermal Activation of Neurons, K. Pu, J. Am. Chem. Soc., 2016, 138, 9049–9052 CrossRef CAS PubMed.
- Y. Liu, K. Ai, J. Liu, M. Deng, Y. He and L. Lu, Dopamine-Melanin Colloidal Nanospheres: An Efficient Near-Infrared Photothermal Therapeutic Agent for In Vivo Cancer Therapy, Adv. Mater., 2013, 25, 1353–1359 CrossRef CAS PubMed.
- Y. Lyu, J. Zeng, Y. Jiang, X. Zhen, T. Wang, S. Qiu, X. Lou, M. Gao and K. Pu, Enhancing both biodegradability and efficacy of semiconducting polymer nanoparticles for photoacoustic imaging and photothermal therapy, ACS Nano, 2018, 12, 1801–1810 CrossRef CAS PubMed.
- C. Goya, R. Yamazaki, Y. Tomita, Y. Kimura and K. Matsumoto, Effects of pulsed Nd:Y AG laser irradiation on smear layer at the apical stop and apical leakage after obturation, Int. Endod. J., 2000, 33, 266–271 CrossRef CAS PubMed.
- H. He, J. Yu, Y. Song, S. Lu, H. Liu and L. Liu, Thermal and Morphological Effects of the Pulsed Nd:YAG Laser on Root Canal Surfaces, Photomed. Laser Surg., 2009, 27, 235–240 CrossRef PubMed.
- A. O. Lopes, C. de P. Eduardo and A. C. C. Aranha, Clinical evaluation of low-power laser and a desensitizing agent on dentin hypersensitivity, Lasers Med. Sci., 2015, 30, 823–829 CrossRef PubMed.
Footnotes |
† Electronic supplementary information (ESI) available. See DOI: 10.1039/d0qm01006e |
‡ These authors contributed equally to this work. |
|
This journal is © the Partner Organisations 2021 |