DOI:
10.1039/D0RA08617G
(Review Article)
RSC Adv., 2020,
10, 41560-41576
Photodynamic diagnosis and photodynamic therapy of colorectal cancer in vitro and in vivo
Received
9th October 2020
, Accepted 8th November 2020
First published on 14th November 2020
Abstract
Colorectal cancer (CRC) is a global challenge to eradicate. Early diagnosis and treatment strategies with ideal advantages, such as high tumor selectivity and negligible adverse effects, are significant, since they can result in precise diagnosis and treatment to reduce the overall incidence of CRC. The photodynamic approach for the detection and therapeutic treatment of cancer is a promising novel strategy in comparison to conventional treatments. Photodynamic diagnosis (PDD) is a diagnostic modality that involves the emission of light-induced excitation fluorescence to enhance early detection, without tumor destruction, after photosensitizer exposure to blue light. Photodynamic therapy (PDT) is a photochemistry-based approach that is rapidly progressing to solve the limitations of standard CRC treatments. PDT involves the interaction of a photosensitizer, tissue oxygen, and red light, which forms reactive oxygen species and radicals to elicit localized cancer cell death. This review discusses conventional CRC diagnostic and treatment methods, with their limitations, in comparison to the newly evolving in vitro and in vivo photo-diagnostic and treatment regimes, which have been investigated over the last several years. It also gives an overview of the integration of PDT with PDD, and utilization of specific photosensitizers for the possible early diagnosis and treatment of CRC.
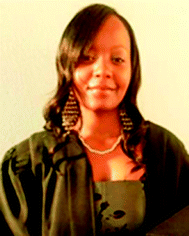 Nokuphila Winifred Nompumelelo Simelane | Nokuphila Winifred Nompumelelo Simelane (Hons.) obtained her honours from the University of Tswane in 2017. She is currently completing her master's studies at the Laser Research Centre, University of Johannesburg. Her research interests lie in the area of antibody nano mediated Photodynamic Therapy (PDT) and Photodiagnosis (PDD) for the early detection and treatment of colorectal cancer. |
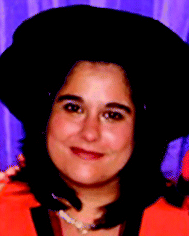 Cherie Ann Kruger | Cherie Ann Kruger (PhD) is a renowned young academic researcher at the University of Johannesburg (UJ), Laser Research Centre (LRC), Her research interest lies within the scientific field of Photodiagnosis (PDD) and Photodynamic Therapy (PDT), whereby she investigates the uses of various photosynthetic chemotherapeutic agents to target, detect, identify, as well as kill cancer tumour cells. She received UJ Vice Chancellor's Distinguished Award for Most Promising Researcher 2019. |
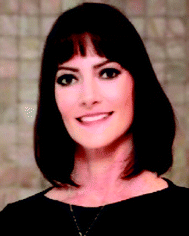 Heidi Abrahamse | Heidi Abrahamse (Prof, PhD) is the Director of the Laser Research Centre, UJ and DST/NRF SARChI Chair for Laser Applications in Health. Her research interests include, photobiology and photochemistry with specific reference to photodynamic cancer therapy, stem cell differentiation and wound healing. She was the recipient of the Faculty of Health Sciences highest research output for 2009 and the University of Johannesburg Vice-Chancellor's Distinguished Award for Outstanding Researcher of the Year, 2010 and 2020. |
1. Introduction
Colorectal cancer (CRC) frequently emerges from precancerous polyps that are initially confined in the innermost mucosal layers of the colon.1,2 This devastating disease is recognized amongst the most prevalent malignancies and can be considered as the second leading cause of tumor-associated deaths in women and the third most common in men worldwide.3 Recent projections have indicated that by 2030, the incidence and mortality rate of CRC will increase by 60% globally.3,4 Within current traditional diagnostic techniques and treatments patients often suffer lengthy invasive treatment regimens, with unwanted side effects in order to eliminate CRC and so earlier detection methods with limited treatment side effects require further research and investigation in order to improve overall diagnostic findings and therapeutic outcomes.5
Current CRC diagnostic methods are conducted to detect premalignant lesions and a positive diagnosis finding only then prompts an early application of an effective treatment modality.6 However, this asymptomatic clinical presentation in the early stages of CRC malignancies has led to a very late diagnosis and so by the time these premalignant lesions have been observed, the cancer is usually in a very late and progressed stage.7 Additionally, CRC carcinomas in situ can sometimes be deceiving in diagnosing owing to their anatomical presentation in the colon, due to their small size and so can be easily overlooked, when using standard screening and diagnostic modalities, hence they are only detected later as metastatic tumors, when a patient develops symptoms.8,9
Thus, early and accurate detection of CRC is of upmost importance, as well as improved localized treatments, in order to enhance patient survival rate and prevent secondary spread.8
2. Methods
This review followed a systematic methodical approach, when searching for comparable photo-diagnosis and photodynamic therapy CRC applied in vitro and in vivo studies. Databases such as PubMed, ScienceDirect and Springer were utilized to search for literature relevant articles. The inclusion criteria involved only utilizing articles that were published over the last seven years (i.e. 2013 to 2020), within cancer, cellular biology, photochemistry and nanomaterial-based journals. The key words of inclusion criteria included: cancer, colorectal cancer, in vitro, in vivo, photodynamic diagnosis, photodynamic therapy, photosensitizers, nanoparticles, targeting biomarkers and laser irradiation. Studies which included clinical trials or patents were excluded.
3. Colorectal cancer
The colon and rectum intestinal wall comprise of many layers made of different tissues that serve several key functions in the gastrointestinal tract.2,10 CRC arises from benign adenomatous polyps in the colon.1,2 When polyps become cancerous, they become full-blown tumors on the wall of the colon and later protrude outwards into the intestinal lumen.11,12 These outward protruding tumors can then also spread further into the cardiovascular or lymphatic system, as well as to other tissues or organs of the body, resulting in secondary cancer metastasis.13,14
3.1. Staging and survival of colorectal cancer
There are four distinct stages that are used to categorized, treat and determine an overall patient's survival rate when diagnosed with CRC. Stage 0; is the earliest stage of CRC, whereby abnormal cells arise from the mucosal membrane of the colon and if diagnosed at this stage, it can successfully be eliminated, and further progression stopped.6 Stage I; the primary polyps pass through the colorectal muscularis mucosa to the submucosa and can potentially protrude to the muscularis propria.6,7 A patient's survival rate at Stage I, is about 95%, when a surgical treatment modality is used, however generally only about 40% of cases are diagnosed at this stage.7 Stage II; is sub-divided into three sections. Stage IIA; the CRC has outstretched to the serosa (outermost layer) of the colon wall by filtering through the colon muscular layer.6 Stage IIB; the CRC penetrates the outermost membrane of the colon barricade but has not migrated to neighbouring organs.6 Stage IIC; the CRC has penetrated through the serosa of the colon barricade to the adjourning organs.6 Within, Stage II, surgical excision is advocated as treatment of choice with an overall patient survival rate of 85%, however less than 30% of cases are detected at this stage.7 Stage III; occurs when the entire walls of the colon has been penetrated by the CRC, as well as neighbouring lymph nodes.7 Surgical excision in combination with other treatment modalities, is the supported treatment of choice, with an overall patient survival rate between 30 to 60% and generally 80% of cases are usually only diagnosed at this stage.6,7 Stage IV; this is the metastatic phase of CRC, whereby the tumor has spread to other sites with a patient's body.7 The treatment of choice for Stage IV CRC is usually chemotherapy or targeted therapy in combination with surgery.14 However, patients usually only have a prognosis of about 3% survival and generally 80% to 90% of cases are usually only diagnosed at this very late stage.14 From these findings, it is thus imperative that in order to assure a patients CRC survival rate when applying an effective treatment, that early detection and diagnosis is of utmost importance.
4. Conventional diagnosis of colorectal cancer
Conventional diagnostic tests of CRC can be subdivided into two types; non-invasive tests that are stool-based and invasive tests which visually examine the colon.15
4.1. Stool-based tests
Stool-based tests involve the use of a two types fecal test assays known as the Fecal Immunochemical Test (FIT) or Fecal Occult Blood Test (FOBT), which generally assess any abnormal genetic material (i.e. blood or DNA) that could have been released into a stool by a CRC positive patient.15 Both stool-based tests detect invisible manifestations of blood and/or antibodies in a potential CRC patients feces.6 However, these tests are limited, since they lack sensitivity for early stage CRC diagnosis, are costly and simply cannot detect diminutive polyps.6
4.2. Visual examination tests
In relation to visual examination tests, white light colonoscopy is currently the most prominent and less costly techniques used by physicians to identify and detect CRC tumors or polyps, since it enables simultaneous visualization of the entire distal colon, as well as allows for biopsy sampling of any detected polyps.8,16 However, the current limitations of white light colonoscopy are that it lacks the sensitivity in identifying smaller CRC lesions, so many patients often go by undiagnosed.6,8,16 Furthermore, classical colonoscopy specificity and sensitivity limitations also include the fact that it may detect lesions other than CRC polyps and is unable to discriminate a benign adenoma from an invasive carcinoma.17–19
4.3. Blood and biopsy tests
Once conventional stool or visual based diagnostic tests of a colon have been performed and abnormalities have been detected then further confirmatory blood and biopsy tests on patients are usually conducted. Biopsies removed during a colonoscopy are usually morphologically and genetically evaluated.8 Genetic blood or biopsy patient specimen tests include the evaluation of elevated gene biomarkers.8 The biomarkers which are tested for, due to an overexpression in CRC patients, include three molecular pathway activation mechanisms, which associated with the progression of adenoma to carcinoma.8 These pathways include; chromosomal instability (CIN), microsatellite instability (MSI) and CpG island methylator phenotype (CIMP).20 However, the sensitivity of these assays is questionable; for example, manifestation mutations of specific biomarker oncogenes, such as KRAS proto-oncogene GTPase (KRAS) can be detected.20 KRAS is a transductor GTP protein/biomarker that is also expressed by non-tumorous cells and so is a highly non-specific bio molecular marker for CRC.12 The most used specific biomarkers for CRC detection are elevated serum biomarkers such as; carcinoembryonic antigen (CEA) and carbohydrate antigen, also known as cancer antigen (CA) 19-9.21 However, the reliability of these biomarkers for early CRC diagnosis is questionable, since they are usually only found overexpressed in progressive late stages of CRC.21
Undoubtedly, it can be observed from the above discussed CRC conventional diagnostic methods, that they lack sensitivity and most only able to confirm late stage CRC. Thus, in relation to CRC patient early diagnosis and detection with positive treatment outcomes, researchers urgently need to focus on the development of a simple, low-cost, non-invasive highly specific CRC tumor targeting diagnostic modalities.
5. Conventional treatment of colorectal cancer
The most routinely used treatment modalities for colorectal treatment available include surgery, chemotherapy, radiation and immunotherapy.11,22 The effectiveness of these treatment modalities is highly dependent on the stages, tumor size and advancement of cancer.2,23 Furthermore, these treatments can be used in conjunction with other treatment modalities based on the stage at which the patient was diagnosed.2 However, reports indicate that even though these treatment modalities are developed to circumvent CRC, they still yield harmful effects post-treatment.6
5.1. Surgery
Surgery is a the most widely used treatment modality for CRC.6 It is based on the excision of well-defined lesion as means to remove a CRC tumor and so prevent it growth and spread.6 However, the application of surgery in CRC patients is highly dependent on variables such as; tumor location, as well as the presence and degree of metastasis.11 A surgical procedure is generally effective within the early stages of CRC, especially if the tumor is small and localized in lesions that have not migrated to other organs.7 However, CRC cells can sometimes shed into the circulatory system during surgical procedures or parts of the lesion remain unresected and this can potentially trigger tumor recurrence or spread after excision.6 Furthermore, pain, tenderness and other uncomfortable complications have been associated with surgical CRC tumor removal.11,24
5.2. Radiation
Radiation utilizes physical radioactive agents and X-rays, which are aimed at tumor cells in order to eradicate their multiplication potential.25 The principle is based on suppressing malignant cell division and growth by introducing ionized radiation to eradicate tumor cells.26 This treatment is usually used to shrink a CRC tumors abnormal growth, in order to facilitate an easier excision during surgery.24 The most prominent disadvantage of radiation is that it causes DNA material destruction in adjacent healthy tissue cells.6 Moreover, other side effects such as bowel dysfunction, nausea, bladder dysfunction, fatigue and skin irritations have been reported from CRC patients receiving such treatments.2
5.3. Chemotherapy
Chemotherapeutic drugs are administered intravenously to CRC patient with the intent at stopping or destroying abnormal cells from proliferating uncontrollably.6 Standard chemotherapeutic agents used in CRC treatment include; the combination of leucovorin, 5-fluorouracil (5-FU), and either oxaliplatin (FOLFOX protocol) or irinotecan (FOLFIRI protocol), capecitabine (Xeloda) and oxaliplatin (CAPEOX or CAPOX protocol) and leucovorin, 5-FU, oxaliplatin and irinotecan (FOLFOXIRI protocol).2,16 These chemotherapeutic agents have mostly noted improved survival rates in patients, who have been diagnosed within early stages of CRC cancer and when used in combination with radiation, however, can sometimes have a poor outcome for those whom have been diagnosed with late CRC.2 Furthermore, chemotherapeutic drugs are frequently associated with a range of dose limiting adverse side effects such as hair loss, vomiting, diarrhea, and nausea.16 In addition, it must be noted that only a negligible dose of the actual chemotherapeutic drug reaches the affected CRC tumors, while the rest of the cytostatic drugs remain within the body, causing undesirable damage and unwanted side effects on healthy tissues.24
5.4. Immunotherapy
Immunotherapy is a type of treatment which harnesses the potential of the body's immune system to eradicate cancer cells by administering various cancer vaccines and monoclonal antibodies, etc.27 In cancer diseases the immune checkpoint inhibition prevents the inhibitory mechanisms of T-cell activation, allowing tumor-reactive T cells to detect tumor antigens and so promote antitumor immune responses.6 Keytruda (pembrolizumab) and Opdivo (nivolumab) have reported significant anticancer activity in advanced CRC with mismatch repair deficient (dMMR) and microsatellite instability high (MSI-H) abnormalities.6 However, most CRC immunotherapy drugs are novel and many of these treatments are still in clinical trials and so unavailable to patients at present.3 Furthermore, immunotherapy drugs are expensive and have been associated with post-therapeutic complications such as fatigue, nausea, disorientation and death if administered incorrectly.3,6
Overall, it can be stated from the above findings that conventional treatments for CRC such as surgery, chemo and radiotherapy are generally only mostly effective at eliminating CRC within its early stages of diagnosis, suggesting that patients with a late detection often have a poor prognosis. Immunotherapy is an up and coming treatment option, however, requires completion of clinical trials and FDA approval before it can be administered safely. Thus, the early diagnosis and detection of CRC is imperative to improve patient's survival rates and researchers need to start investigating more specific and unconventional treatment methods for CRC, in order to lessen the rates of patient fatality (Table 1).
Table 1 Conventional versus unconventional methods for the diagnosis and treatment of CRC
Types of CRC diagnosis and treatment options |
Ref. |
Conventional diagnosis |
CRC in situ is deceiving in diagnosing owing to their anatomical presentation and small size, hence they easily overlooked and usually only detected at later stages when a patient develops symptoms |
8 and 9 |
Stool-based tests |
• Non-invasive |
6 |
• However, lack sensitivity for early stage diagnosis and are costly |
Visual examination tests |
• Less costly |
6, 8, 16 and 17 |
• Allows simultaneous visualization of the entire distal colon, as well as allows for biopsy sampling |
• However, invasive, lacks the sensitivity in identifying smaller CRC lesions and unable to discriminate between a benign versus invasive carcinoma |
Blood and biopsy tests |
• More specific for detection |
21 |
• However, invasive and reliability of biomarkers for early CRC diagnosis is questionable, since they are usually only found overexpressed in progressive late stages of CRC |
Conventional treatment |
The effectiveness of treatment modalities is highly dependent on the stage and size of tumor. However, even though these treatment modalities are developed to circumvent CRC, they still yield harmful effects post-treatment |
6 and 23 |
Surgery |
• Success dependant on tumor location, as well as the presence and degree of metastasis |
6, 7, 11 and 24 |
• Generally effective within the early stages, especially if the tumor is small and localized in lesions that have not migrated to other organs |
• However, if cells are shed into the circulatory system during surgery or parts of the lesion remain unresected, this can potentially trigger tumor recurrence or spread after excision |
• Associated with pain and tenderness |
Radiation |
• Successfully, used to shrink tumors abnormal growth, in order to facilitate an easier excision during surgery |
2, 6 and 24 |
• However, causes DNA material destruction in healthy tissue cells and causes side effects, such as bowel dysfunction, nausea, bladder dysfunction, fatigue and skin irritations |
Chemotherapy |
• Mostly noted improved survival rates in early diagnosed patients and when used in combination with radiation |
2, 16 and 24 |
• However, can have a poor outcome in late diagnosed patients |
• Adverse side effects such as hair loss, vomiting, diarrhea, nausea and unwanted side effects on healthy tissues |
Immunotherapy |
• Keytruda (pembrolizumab) and Opdivo (nivolumab) have reported significant anticancer activity |
3 and 6 |
• However, most drugs are novel and many of these treatments are still in clinical trials and so unavailable to patients at present |
• Furthermore, drugs are expensive and have been associated with post-therapeutic complications such as fatigue, nausea, disorientation and death if administered incorrectly |
Photodynamic diagnosis and treatment |
Most prominent advantage is that via blue and red-light colonoscopy, can be specifically designed to be highly selective in identifying, as well as combatively targeting and treating cancer cells, without damaging adjourning tissues, as well as allow for multiple dosage administration with negligible side effects being observed. Treatment is localized with minimal side effects to surrounding tissues. However, this method is still in early stages of research and still requires clinical approval |
28, 29 and 33 |
6. Unconventional diagnosis and treatment of colorectal cancer
Several conventional diagnosis and treatment therapies are available for CRC however, as previously discussed most of these treatments have limitations which include; severe side effects, invasiveness and inability to effectively treat late stage CRC.7 Furthermore, current white light colonoscopy diagnostic optical imaging techniques lack sensitivity to detect early stage CRC.19 These unmet needs of conventional CRC diagnosis and treatment methods has caused researchers to investigate photodynamic diagnostic and therapeutic treatments.28 The most prominent advantage of these photodynamic approaches over conventional CRC diagnostic and treatment methods; is that via blue and red-light colonoscopy, they can be specifically designed to be highly selective in identifying, as well as combatively targeting and treating cancer cells, without damaging adjourning tissues, as well as allow for multiple dosage administration with negligible side effects being observed (Table 1).28
6.1. Photodynamic diagnosis and photodynamic therapy
The early detection of CRC is an important measure which can enhance patient survival rate and subsequently prevent metastasis.16 Thus, it is critical that a diagnostic tool be developed for CRC which is minimally invasive, is photodynamic diagnosis (PDD) is an innovative unconventional technique based on a photodynamic integrative method, whereby a photochemical molecule known as a photosensitizer (PS), is administered to a patient and selectively absorbed intracellularly by targeted tumor cells only.29 This selective absorption of a PS in a tumor cell, is due to the enhanced permeability retention (EPR) effect.14 Since all tumors have somewhat of a leaky vasculature, poor lymphatic drainage and increased vessel permeability, these factors allow macromolecular drugs (such as PSs) to accumulate more in tumor than in normal tissues.14
An absorbed PS can then become activated when a visible blue light source at an approximate wavelength of 330 to 400 nm is applied to it, causing it to become excited and so fluoresce.29 This causes the PS identified tumor cells to illuminate, without causing cellular or tissue harm and so allows for ease of identification of a pre-cancerous or cancerous lesion.29 Since, most PSs preferentially accumulate in abnormal tumor tissues and some have excitable characteristics within the lower visible blue region of wavelength, this PDD method can allow for easy visualization and detection of tumor cells, possibly allowing for early diagnosis and effective treatment outcomes.29,30
Photodynamic therapy (PDT), a promising unconventional cancer therapeutic strategy with achievable treatment outcomes that surpasses existing conventional strategies.22 PDT is currently a non-intrusive treatment modality used to treat many types of cancers.31 It integrates three essential components; (1) a photoactive chemical (PS) which selectively accumulates in discriminated cancerous cells/tissues and upon illumination from (2) a specific light source within the red/near infrared wavelength region of 600 to 800 nm, (3) which produces a photochemical reaction mechanism with surrounding tumor molecular oxygen to generate cytotoxic singlet molecular oxygen and other reactive oxygen species (ROS), which in turn destroy tumorous tissues.32 The success of this PDT photochemical mechanism of action is highly dependent on the concentration and specifically targeted subcellular accumulation of PSs in tumor tissues, in order to generate high enough cytotoxic singlet molecular oxygen and ROS to effectively induce apoptotic or necrotic cell death in tumors and so destroy them.31,32
Currently, researchers are trying to investigate and improve PS cancer cells subcellular uptake, accumulation and localization by developing PS photochemicals which can specifically identify and target cancer cells only, as to ensure selective identification and enhanced cancer treatment outcomes, as well as be used in combination when applying PDD and PDT methods in clinical or research fields for cancer management.29,33
6.2. Photodynamic diagnosis and photodynamic therapy mechanism of action
Both PDD and PDT incorporate the use of an administered PS photochemical, which can function as a molecular contrast agent when illuminated by blue light for diagnosis purposes, or function as a cytotoxic singlet molecular oxygen and ROS generators when activated with red light for localized tumor destruction.31 The administration of the PS is dependent on the location of a tumor; it can be orally ingested or intravenously delivered.34 Moreover, the PS may accumulate passively or actively within a cancerous cell or tissue depending on the drug delivery system involved.34
In PDD applications the PS can be effectively excited within the blue region of light for illumination diagnosis (Fig. 1), however in PDT applications, the PS is activated within the red to near infra-red light region in the presence of molecular oxygen contained within a tumor to cause apoptotic or necrotic cytotoxic cancer cell death (Fig. 2).29,35 Thus, depending on the type of wavelength used to active an intracellularly localized PSs, one of several types of mechanisms of action can occur, before it returns to its inactivated zero-point state.35
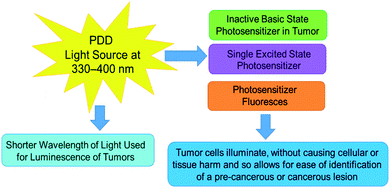 |
| Fig. 1 PDD mechanisms of action, after excitation/activation of a tumor localized PS at a short blue wavelength of light, it causes the PS to emit fluorescence to allow for tumor identification. | |
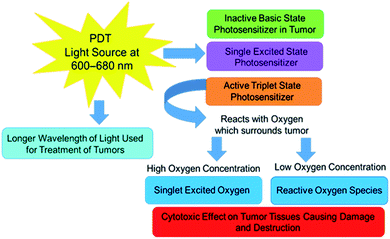 |
| Fig. 2 PDT mechanisms of action after excitation/activation of a tumor localized PS at a long red wavelengths of light, the PS reacts with tumor surrounding molecular oxygen and dependant of the concentration produces cytotoxic species which in turn cause tumour destruction. | |
In PDD, upon absorption of blue light (330 to 400 nm), PS electrons become excited into a singlet activated state, whereby they radiate this energy gained in the form of fluorescence.29,36 The PS in this singlet-excited state, cannot partake in any cellular mechanisms which induce cell signalling death pathways, since this activation is short-lived, with a lifespan that usually lasts anywhere between a few nanoseconds to a short picosecond and so only diagnostic illumination, with no cellular damage can be produced.35
Within a PDT mechanism of action, an excited PS electron can spontaneously interchange from a singlet energy level to an agitated triplet state.37 There are then two additional mechanisms of action which occur in a PSs agitated electron triplet state to move it down to a ground state.31 In a Type I reaction, the excited PS triplet state electrons directly react with adjacent cellular molecules, where there is a low surrounding oxygen level and so transfer this energy to produce ROS.38 Alternatively, in Type II reactions there is the direct transpose of excited PS triplet state electron energy to high surrounding subcellular molecular oxygen concentrations to form singlet state oxygen species.39 Both mechanisms of action in PDT usually react simultaneously to produce ROS and singlet state oxygen species, which are considered to be lethal within tumor cells, since they trigger a cascade of multifactorial cytotoxic pathways, which impose oxidative damage to surrounding proteins, lipids, and nucleic acids, inducing either apoptotic or necrotic forms of cell death in localized cancer cells.31 Apoptosis is a physiological cell death mechanism that transpires without eliciting inflammation or immunological reactions, whereas necrosis is an unregulated and aggressive form of cell death, frequently linked with inflammatory processes, either way within PDT mechanisms of action PS targeted tumor cells can adequately be destroyed.40
Additionally, besides PDT treatments being able to cause direct cytotoxic effects on laser irradiated cells, PDT can also induce the release of pro-inflammatory molecules and tumour vasculature shutdown.41 When PDT treatments cause damage to a tumour's vascular surroundings, this leads to a depletion of oxygen and nutrients to the tumour, indirectly killing the tumour. This distress in a tumour's microenvironment, post-PDT, provokes a strong acute inflammatory reaction, which causes an infiltration of host innate immune cells.41 Host innate immune cells, such as tumor-specific cytotoxic T-cells, carry out the removal of damaged cells, however are also capable of destroying distant untreated tumor cells and so this leads to the development of PDT anti-tumor memory immunity, which is another benefit of PDT treatments, since it potentially prevents the recurrence of cancer.41
6.3. Photosensitizers for PDD and PDT
One of the most important constituents involved within the process of PDD and PDT is a PS. A PS is a nontoxic photochemical compound, that when administered topically, orally or via intravenous injection, can accumulate either passively or actively within a cancer tumor and so be successfully activated with specific wavelengths of light to allow for effective PDD or PDT cancer treatment applications.42
Within PDT applications the ideal therapeutic wavelength of activation for a PS to yield high enough concentrations of cytotoxic species to trigger cancer cell death ranges from 600 to 800 nm,31 whereas within PDD PS diagnostic applications the excitation illumination wavelength ranges between 330–400 nm.29
In general, PSs are categorized into either first, second or third generations, depending on their overall complexity and abilities to generate successful application outcomes.38
First-generation PSs comprise of hematoporphyrins (HP) and photofrin.38 HP was initially developed as a fluorescent PDD dye; however, its undesirable characteristics of low intracellular localization hindered its clinical acceptance.43 Photofrin, is a haematoporphyrin derivative (HpD) and has been clinically approved for the PDT therapeutic treatments of different kinds of cancer such as; lung, esophageal, stomach and early stage cervical cancers.44,45 However, ongoing clinical studies have noted that photofrin has immanent drawbacks such as; prolonged cutaneous photosensitivity symptoms, due to its long-life span clearance from the body, as well as low light absorption and so this hinders optimal PDT treatment outcomes.46
Thus, second-generation PSs were developed to overcome the limitations of traditional first-generation PSs.47 Most second-generation PSs comprise of porphyrin and chlorin structures, which have their own individual distinctive properties.48 Both PSs showed improved PDT chemical and purity properties, when compared to first-generation PSs; they reported minimized adverse reactions and were able to be activated at longer wavelengths, allowing for deeper tissue light penetration and so enhanced diagnostic and treatment outcomes.48 Examples of second-generation PSs include; chlorins, protoporphyrin IX (PpIX), benzoporphyrins, hypericin, phthalocyanines and 5-aminolevulinic acid (ALA), which is a biological precursor of PpIX.49 However, within clinical studies several of these second-generation PSs noted limitations, such as being hydrophobic in nature and so this limited their passive absorption and sub-cellular localization in targeted tissues and so poor diagnostic and treatment outcomes in patients were reported.50
These outcomes persuaded researchers to investigate the development of third-generation PSs for PDT and PDD applications. Basically, third-generation PSs are second-generation PSs, which have been modified in some way to enhance PS uptake in target cells.30 In some cases, PSs are conjugated to carriers such as nanoparticles or liposomes, to promote passive absorption in target cells, as well as improve their hydrophilic nature by coating these nanoparticles with various layers, such as a poly-ethanol glycol (PEG) layer.30 Moreover, some studies go on further to improve third-generation PSs uptake, by conjugating either the PS itself or the nanoparticle carrier to various bio-targeting markers, such as; antibodies or ligands which can identify over expressed markers in tumor cells and so enhance specific active uptake of PSs, when these target cancer cells are recognized.34 Overall, researchers have focused on second-generation phthalocyanines, chlorins and benzoporphyrins PSs for manipulation and modification into third-generation PSs, since they idealistically can be excited within the low illumination wavelength ranges for PDD applications, as well as be activated within the high red PDT therapeutic wavelength ranges, for enhanced tissue penetration and high cytotoxic species yields, with uncompromising treatment outcomes.42
6.4. Photosensitizers for PDD and PDT colorectal cancer applications
For PSs to be considered for use in PDD applications, they need to possess the following properties; they need to have a the high affinity and preferential selective uptake in target tumor cells only, they must have a high fluorescence quantum yield within the 330–400 nm range, they must be rapidly cleared from the body and finally exhibit minimal levels of toxicity.38 Additionally, for a PS to ideally be considered for clinical PDT applications, it should preferentially accumulate in cancer tissues, with minimal phototoxic side-effects, as well as absorb light in the red to near infra-red wavelengths (600–800 nm) for maximum tissue light penetration in order to generate high enough levels of cytotoxic species to induce cancer cellular damage.48,51–53 The review below discusses the widely utilized photo diagnostic and treatment methods for CRC and their limitations, over the last seven years. In addition, it also gives an overview of the synergistic integration of the therapeutic characteristics of PDT with PDD, as well as the utilization of PSs at specific wavelengths for the early diagnostic and feasible treatment of CRC. Therefore, since PDT and PDD methods utilize specific PSs for targeting CRC tumors to allow for easy identification and treatment in the blue and red wavelengths of light, these method would be far more superior than conventional white light colonoscopy, which can only be used to detect CRC lesions and lacks sensitivity. Table 2 includes a summation of the various generations of PSs utilized for PDD and PDT treatment of CRC, noting their overall effectivity.
Table 2 Summation of the various generations of PSs and their overall effectivity for the PDD and PDT treatment of CRC
PS generation |
PS examples |
PDD and PDT effectivity for CRC |
Ref. |
1st (PS only, passive uptake) |
Hematoporphyrins (HP) and photofrin |
Ineffective |
• Low intracellular localization |
45 |
• Prolonged cutaneous photosensitivity |
• Long-life span clearance |
• Low light absorption and poor tissue penetration |
2nd (PS with structural modifications for passive uptake) |
Chlorins, protoporphyrin IX (PpIX), hypericin, phthalocyanines and 5-aminolevulinic acid (ALA) |
Moderately effective |
• Chemical and purity properties, minimized adverse reactions |
47 and 57 |
• Able to be activated at longer wavelengths, allowing for deeper tissue light penetration |
• Shorter-half life |
• Restrictions, such as hydrophobic nature limits their passive absorption and sub-cellular localization in targeted tissues |
3rd (PS functionalized for active uptake) |
2nd generation PS + nanoparticle + target biomolecule |
Effective |
• Improve PS tumor cell specific uptake and localization via active targeting, enhances outcomes |
14, 41, 76 and 77 |
Nanoparticles: gold, silver, quantum dots, up-conversion, polymer-based and silica-based materials |
• NPs improve PS solubility, stability and limit non-specific toxicity |
Target biomolecules: antibodies (e.g.: EGFR, FGFR, EpCAM, CA IX, PPARγ, COX-2, CD44+, CD133+, CD166+ and CD24+), ligands, peptides and carbohydrates (e.g.: glucose, glycol) or checkpoint blockade CRC immunotherapy |
• NPs mimic biological molecules and so protect PSs from immunological barriers improving their uptake in tumor cells |
|
• Rapid clearance from body |
|
• Able to be activated at longer wavelengths, allowing for deeper tissue light penetration |
|
• Most successful 2nd generation PSs for CRC was found to be: 5-ALA porphyrins, chlorins and phthalocyanines. Hypericin noted PDT resistance |
6.4.1. First-generation PSs for PDD and PDT colorectal cancer applications. In relation to CRC, the first PDD diagnostic tool and PDT applications was reported in the early 1900s using a first-generation PS known as photofrin (which is a HpD).43
Photofrin. Photofrin was considered for CRC photo diagnostic and treatment options, since it demonstrated the ability to passively accumulate in tumor cells, emit fluorescence, as well as generate cytotoxic species.43 Within, PDD and PDT post-clinical performed studies by Allison and Moghissi et al. (2013) and Kawczyk-Krupka et al. (2016) on solid CRC tumors, drawbacks, such as; insufficient tissue selectivity, photobleaching of the skin, lack of long wavelength absorption for activation and phototoxicity was reported in patients and so this hindered HpD PS use within these applications.44,54 Since then, several other clinical trials using HpDs for CRC PDD and PDT applications have been investigated, however noted poor outcomes due to unwanted side effects.55,56 Recently, even a study by Sun et al. (2016), was performed on 23 patients (observation group) with advanced CRC and they were subjected to photofrin 630 nm mediated PDT treatment and results showed that the CRC tumors were eliminated within an increased curative rate and improved clinical manifestation, however severe post-PDT skin photosensitivity was found.57
6.4.2. Second-generation PSs for PDD and PDT colorectal cancer applications. Thus, the need to overcome these limitations spurred researchers on to investigate second-generation PSs, for PDD and PDT CRC applications. Researchers have focused on second-generation PSs such as porphyrin like molecules (e.g. 5-aminolevulinicacid, chlorins), natural compounds like hypericin or structures with aromates fused to pyrrole rings (e.g. phthalocyanines), since they have longer absorption coefficients in the region of illumination and therapeutic transparency window, short half-life, as well as selective passive tumor tissue accumulation for successful CRC PDD and PDT outcomes.58
5-Aminolevulinicacid (5-ALA) and porphyrins. 5-Aminolevulinicacid (5-ALA), is a biosynthetic precursor of the PS protoporphyrin IX (PpIX), it has been utilized within PDD applications for various cancers, owing to its unique photo-properties, which allow for it to be activated at short and long wavelengths, thus allowing it to be used for both diagnostic and PDT cancer treatment applications.59,60 Various studies have elucidated that PpIX exhibits two particular emission of peaks; one at 405 nm emitting blue illumination for PDD diagnosis and one at 633 nm emitting red visible light for PDT treatment of malignant or premalignant tumors.29,61,62Within a study performed by Nakamura et al. (2015), ten patients with early-stage gastric/colorectal tumors were given 20 mg kg−1 of 5-ALA enteric-coated capsules orally.63 After examination with a blue light fluorescence endoscope, to induce PDD illumination the detection rate was found to be accurate in 53.8% of the patients, even though this diagnosis rate was lower than other PDD cancer studies, the potential to accurately diagnose CRC malignant tumors was evident.63 Within, another study performed by Filonenko et al. (2014) from 2008 to 2010, full PDD examinations were performed on 78 patients suffering from colon polyps using an alasens 5-ALA based PS.64 Using spectro-fluorescence colonoscopy PDD results were compared to patients histopathological results, noting a sensitivity of 94.9% and a specify of 62.5%, suggesting this method of diagnosis was specific at identifying areas of colon tumors transformation, as well as allowed for direct imaging and location of targeted polyps for biopsy.64 However, in further studies the utilization of alasens 5-ALA PDD in combination with CRC PDT treatment applications proved unsuccessful.64 Within, studies performed by Namikawa et al. (2015) intraoperative 5-ALA PDD was performed on 26 CRC lesions in 21 patients and 1 g of 5-ALA was administered orally, before surgery.65 After surgical removal polyps were examined using an endoscopic PDD system, and red illuminated-positive polyps were compared with pathological results.65 The overall sensitivity, specificity, and accuracy rate in detecting CRC cancer was between 57.7 to 66.7%, suggesting that 5-ALA PDD, could be a promising diagnostic tool for CRC, however still required further accuracy optimization.65
In relation to 5-ALA PDT research studies, outcomes have been noted to be far more efficient and sensitive than when compared to PDD results. A study by Hatakeyama et al. (2013) investigated the photodynamic activity of 250 mg kg−1 5-ALA on in vitro cultured human colon cancer HT-29 cells and in vivo mice inoculated with this cell line, noting an 88% tumor growth inhibition rate.66 Similarly, studies by Kawczyk-Krupka et al. (2016), reported that in vitro cultured SW480 and SW620 CRC cells treated with 5-ALA PDT reported a dose dependent decrease of 80 to 93% in cell viability, as well as an increase in cell membrane damage.54
Chlorins. Chlorins are second-generation PSs, that are a reduced form of porphyrins, to which groups like meta-tetra(hydroxyphenyl) chlorin (m-THPC) belongs.54,67 Chlorin PS-mediated PDT effects toward CRC cells has been extensively investigated, however PDD is not possible, due to its inability to illuminate at short wavelengths.54 Studies by Abdulrehman et al. (2018) reported that 11.6 μM of m-THPC PDT induced apoptosis in SW480 and SW620 CRC cell lines of 65% and 25%, respectfully, after 660 nm of irradiation was received.1 Studies by Kaizhen et al. (2018) noted similar chlorin e6 PDT results in SW620 CRC cell lines, however noted that autophagy form of cell death from which CRC cells can recover was also prominently present.68 Several other studies have also gone on to report significant cellular morphology, viability, proliferation and cytotoxicity changes in CRC cell lines and tumors, which received m-THPC mediated PDT, however also noted the induction of possible pro-survival autophagy cell death pathways and PDT resistance mechanisms.69–71 In order to overcome chlorin PS inabilities to be used in PDD applications, studies by Shimizu et al. (2018) developed talaporfin sodium (TS: mono-l-aspartyl chlorine e6, or NPe6), which a second-generation chlorin derivative PS, that has absorption peaks in the Soret band of 398 nm and Q bands of 502, 530, 620 and 654 nm.72 Thus, TS can emit illumination upon activation within its Soret band, as well as generate ROS and singlet oxygen when activated within its Q-band region.72 However, studies have only shown the successful use of TS for the diagnosis and PDT treatment of brain tumors, and investigations into its photodynamic activity for CRC diagnostic and treatment applications remains unknown.72
Hypericin. Hypericin is another natural second-generation photosensitizing compound, which can perform in both PDD and PDT applications, due to its dual absorption within the short blue and high red wavelengths of light.73 Hypericin has been clinically applied for the successful PDD and PDT treatment of bladder, head and neck cancers or gliomas.43,73 However, hypericin has showed little success in CRC PDD and PDT applications due to; its hydrophobicity and aggregation issues in cells, which interfered with its tissue distribution and so affected its ability to sensitivity diagnose CRC, as well as effectively generate enough ROS to destroy tumors.43,54,73,74 Furthermore, CRC has noted resistance to hypericin PDT, due to it inducing hypoxic conditions, which allows the survival of tumor resistant cells and risk of recurrence.54 Additionally, studies by Jendželovská et al. (2016), noted that CRC cells have multidrug resistant hypericin drug efflux transporters (i.e. ABC, MRP1 and BCRP) and so cellular absorption of this PS in CRC is so negligible, that significant results and outcomes for either PDD or PDT applications are implausible.73
Phthalocyanines. Phthalocyanine (Pc)-mediated PDT effects toward CRC cells has also been extensively investigated over the years, due to their abilities to produce high triplet ROS yields, however in relation to utilizing phthalocyanine PS for PDD application in CRC, little to no research has been done. Studies by Sekhejane et al. (2014), investigated the PDT effects of 20 μM sulfonated zinc phthalocyanine (ZnPcSmix) within DLD-1 and CaCo-2 in vitro cultured cell lines using 680 nm of red light. Both cell lines showed significant apoptotic cell death 24 h after PDT treatment.75 Furthermore, studies by Brozek-Pluska et al. (2020), utilized Raman imaging and spectroscopy to characterize and differentiate normal and cancerous colon tissues based on ZnPcS4 PS vibrational features.76 The study found that ZnPcS4 PS noted a higher affinity subcellular localization uptake in CRC cells and noted excitation wavelengths at 532 nm and 689 nm, suggesting that this PS could possibly be successfully used within PDT and PDD applications for CRC diagnosis and treatment, however required further research and investigation.76 However, this study did go on to note that the ZnPcS4 PS has poor solubility and this would affect its overall abilities to bring about sensitive and effective PDD and PDT applications and that this could possibly be overcome by introducing peripheral substitutes or nanocarriers into its frame work.77
6.4.3. Third-generation PSs for PDD and PDT colorectal cancer applications. Third-generation PSs are second-generation PSs, which have been modified with nanoparticle carriers and/or active targeting biomarkers.34 This advancement on second-generation PSs is done in order to improve PS tumor cell specific uptake and localization, allowing improved PDD PS photophysical and illumination properties, as well as concentrated tumor cytotoxic species generation for enhanced PDT treatment outcomes.77,78 To date, numerous studies have been conducted investigating third-generation PSs within CRC PDT treatment regimes, however there are very few that have extensively researched their application for CRC PDD applications.
Nanoparticles and active biomarkers. Nanoparticles have been utilized to improve passive PS uptake in CRC cells, since they enhance selective tumor uptake via the EPR effect, due to leaky vasculature, poor lymphatic drainage and increased vessel permeability.14 Additionally, nanoparticle carriers promote solubility, stability and limit non-specific toxicity of PSs.14 Examples of nanoparticles which have been used as improved passive carriers for third-generation CRC PS studies with great success include; gold nanoparticles, silver nanoparticles, quantum dots, polymer-based nanoparticles and silica-based materials.77 Furthermore, since nanoparticle carriers or PS alone can be functionalized with several biomolecules, researchers have considered investigating CRC PDD and PDT targeted and active PS uptake applications.79 This process involves a PS that is bound or remains unbound to a nanoparticle, that is directly delivered to a CRC tumor target site using specific ligands or antibodies to target overexpressed CRC cell receptors and so ensures specific and directed uptake.2 Common biotarget proteins for CRC include; epidermal growth factor receptor (EGFR), fibroblast growth factor receptor (FGFR), epithelial cell-adhesion molecule (EpCAM), carbonic anhydrase IX (CA IX), peroxisome proliferator-activated receptor γ (PPARγ), cyclooxygenase-2 (COX-2), as well as cluster of differentiation 44, 133, 166 and 24 (CD44+, CD133+, CD166+ and CD24+).14 There are several studies that have demonstrated improved delivery when a PS is actively delivered to specific CRC targeted cells using site specific moieties such as antibodies, peptides and carbohydrates.57
Photofrin. Within a study performed by Sun et al. (2016), 23 patients with advance CRC received 2 mg kg−1 of intravenously injected adjuvant photofrin in a 100 mls of 5% glucose and 630 nm of endoscopic optical fiber delivered laser radiation.57 The overall total effective rate of the observational patients was significantly higher than that of the control group which received conventional treatments, with improved photofrin localization due to glucose adjuvant uptake, however severe skin photosensitivity following laser irradiation was found.57 In relation to third-generation PDD studies utilizing photofrin, none could be found.
5-ALA and porphyrins. Studies by Chung et al. (2013), investigated the PDT effects of photoactivated 5-ALA conjugated to methoxy polyethylene glycol/chitosan (PEG–Chito) copolymer, within in vitro cultured CT26 CRC cells.80 Results noted that PEG–Chito–5-ALA nanoparticles induced far more significant apoptotic and necrotic forms of CRC tumor cell death than when compared to 5-ALA PDT administration alone.80 These findings suggest that the PEG–Chito nanoparticles promoted passive 5-ALA uptake and so increased phototoxicity and higher protoporphyrin IX accumulation in CRC tumor cells in comparison to 5-ALA alone and so this nano delivery vehicle should be considered to enhance CRC PDT treatment outcomes.80Furthermore, studies by Bretin et al. (2019), proved the added value of third-generation PS nanoparticle vectorization in CRC PDT tumor targeting efficacy using 5-(4-hydroxyphenyl)-10,15,20-triphenylporphyrin (TPPOH).81 Silica nanoparticles (SNPs) coated with xylan–TPPOH conjugate (TPPOH–X), showed more significant phototoxic effects within in vitro cultured HT-29, HCT116 and SW620 CRC cell lines and in vivo mouse models, post-PDT and stronger uptake in cells, when compared to free TPPOH.81 Additionally, this study demonstrated far more apoptotic cell death induced by TPPOH–X SNPs-PDT, with autophagy inhibition, when compared to free TPPOH, demonstrating the strong anticancer efficacy of this advanced nano-carrier system.81
Currently, the only fluorescence porphyrin PDD clinically approved technique that is available for cancer diagnosis, is hexaminolevulinate (Hexvix®) solution, which is used for the identification of bladder cancer.82 No studies utilizing any modified third-generation porphyrins for the PDD of CRC were found.83
Chlorins. A study by Gavrina et al. (2018), explored the PDT efficacy of Chlorin e6 (Ce6) conjugated to polyvinyl alcohol (PVA) nanoparticles in CRC CT26 xenograft models.84 Using in vivo fluorescence imaging it was found that Ce6–PVA nanoparticles resulted in a higher tumor-to-normal ratio and greater photobleaching when compared to the use of Ce6 alone.84 Upon tumor histological examination, CRC tumors revealed faster regression, with far more advanced necrosis following Ce6–PVA PDT, when compared to Ce6 PDT alone.84 Overall, this study revealed that the encapsulation of Ce6 in PVA represents a promising strategy for further increasing the selectivity and photo activity efficacy of PDT in CRC tumors.84 Furthermore, within a study by Sundaram et al. (2020) hyaluronic acid and Ce6 was successfully coated onto single walled carbon nanotubes (SWCNTs) and this PS conjugate was administered to in vitro cultured Caco-2 CRC cells.85 Post, 660 nm PDT enhanced apoptotic cell death was reported, suggesting that this nanobiocomposite system enhanced PS localization in CRC cells.85Interestingly, a study performed by Xu et al. (2017) reported positive outcomes in CRC mouse models, when using up-conversion nanoparticles loaded with Ce6 and imiquimod (R837), which is an immunotherapy toll-like-receptor antagonist.86 The multitasking UCNP–Ce6–R837 nanoparticles with NIR enabled enhanced PDT destruction of CRC tumors via the CTLA-4 checkpoint blockade and R837 promoted strong antitumor immune responses, to inhibit the growth of distant tumors left behind after treatment.86 Similarly, studies by Lu et al. (2016) described an effective PDT CRC treatment, which combined Ce6 with a nanoscale metal–organic framework (nMOF) and TBC–Hf, which is an immunotherapy agent that inhibits indoleamine 2,3-dioxygenase (IDO) and so induces systemic antitumor immunity.87 The synergistic PS combination achieved significantly effective PDT induced local and distant tumor rejection in CRC models, as well as enhanced checkpoint blockade immunotherapy.87
Within studies performed by Tanaka et al., (2011) they utilized the Warburg effect (which is a phenomenon whereby tumors consume higher glucose levels than normal cells) to enhance PS uptake in CRC cells by conjugating it to glucose.88 Within this study glycoconjugated chlorin (H2TFPC-SGlc), was compared with talaporfin, which is clinically used in Japan, to treat CRC. Both PSs were administered to gastric and colon cancer cell lines, as well as xenograft tumor mouse models, followed by PDT treatment. In vitro, H2TFPC-SGlc was 30 times more cytotoxic to cancer cells when compared to talaporfin.88 In vivo, H2TFPC-SGlc accumulation was higher in xenograft tumors and significantly suppressed tumor growth in comparison to talaporfin.88 This study suggested that glycoconjugated chlorin could be potentially useful for CRC PDT treatments.88 However, following on this no other studies to date have been conducted investigating the potential PDT treatment enhancement for CRC, utilizing glucose to enhance uptake and so should be further investigated.
Unfortunately, in relation to the utilization of Ce6 third-generation based PSs for the successful PDD of CRC no research studies could be found.
Hypericin. In relation to utilizing hypericin as a second-generation PS in CRC PDD and PDT applications, little success has been reported due to its hydrophobicity and aggregation issues, which interfere with its tissue distribution.43,73,74 Furthermore, CRC tumors have hypericin multi-drug resistant efflux transporters and so this affects its accumulation in cells, reducing its sensitivity in PDD applications and making it resistant to PDT.43,73,74 Studies by Mikešová et al. (2013) noted that the combination of glutathione level and NAD(P)H/FAD redox status when combined with hypericin in CRC cancer cells affects its resistance to PDT.89 Moreover, studies by Khot et al. (2018), treated spheroidal CRC cell models with hypericin PDT and noted significant resistance, with high expression levels of ABCG2 and suggested that possibly by inhibiting ABCG2 expression that this could potentially enhance the efficacy of hypericin-mediated PDT.90 Additionally, studies by Halaburková et al. (2017), reported enhanced CRC hypericin mediated PDT within in vitro cultured HT-29 cells using histone deacetylase inhibitors (HDACis) to sensitize CRC cells external stimuli to overcoming their resistance to hypericin by epigenetically reactivating the expression of CDKN1A.91Studies by Montanha et al. (2017) improved the water solubility of hypericin and its PDT effect within in vitro cultured Caco-2 and HT-29 CRC cells by encapsulating it in Pluronic P123 (P123).92 Results noted that the P123 nanocarrier improved permeation of Hyp into CRC cell membrane leading to significant cell death and showed itself to be a promising PS for PDT CRC treatment.92 Whereas, studies by Sardoiwala et al. (2020) synthesized a hypericin-loaded transferrin nanoformulation (HTfNPs) composite, to overcome the PSs hydrophobicity and poor bioavailability, as well as serve as a PDT epigenetic-based CRC therapy.93 This transferrin targeted PDT significantly induced BMI1 degradation assisted CRC in vitro cell retardation, as well as improved hypericin's availability at diseased sites.93 However, concerningly studies by Kaleta-Richter et al. (2020) noted that hypericin-mediated PDT, stimulates release of interleukin-8 and -10 in CRC, which is responsible for the progression of cancer and metastasis.74 Thus, PDT applications with third-generation hypericin PS, seems rather controversial and no studies noting its application in effective CRC PDD were found.
Phthalocyanines. Numerous successful studies were sourced utilizing phthalocyanines (Pcs) as third-generation PDT CRC PSs, however very few studies utilized this PS within PDD CRC applications.A study by Chiarante et al. (2017) reported the PDT effect of 2,9(10),16(17),23(24)tetrakis[(2-dimethylamino)ethylsulfanyl]phthalocyaninato zinc(II) – Pc9, when encapsulated into Tetronic® 1107 polymeric poloxamine micelles (T1107) on 2D and 3D cultures of CT26 CRC cells.94 The study showed that Pc9–T1107 was efficient in reducing cellular viability after PDT treatment, due to enhanced uptake in CRC lysosomal vesicles and the endoplasmic reticulum cellular regions, with improved apoptotic cell death.94 Furthermore, studies by Chiarante et al. (2020) examined the in vivo effect of PDT with a lipophilic phthalocyanine (Pc9) encapsulated into polymeric poloxamine micelles (T1107) in a murine colon carcinoma model.95 Pc9–T1107 delayed CRC tumor growth with apoptotic cell death being noted, due to a decrease in the expression levels of Bcl-XL, Bcl-2, procaspase 3, full length Bid and a significant increase in active caspase-3 and the detection of PARP-1 cleavage, as well as high levels of interferon-γ and tumor necrosis factor-α being found, in comparison to Pc9 delivery alone.95 Similarly, within a study performed by Obaid et al. (2015) zinc phthalocyanine (C11Pc) PS was bound to PEG gold nanoparticles and further functionalized with monoclonal antibodies specific for the human epidermal growth factor receptor-2 (HER-2).96 Subcellular localization studies within HT-29 CRC cells noted selective bioconjugate absorption in acidic organelles, which is typical of enhanced receptor-mediated endocytosis.96 Additionally, this biomolecule reported an 80 to 90% phototoxicity, in CRC cells, then when compared to (C11Pc) administration alone.96 These studies prove that the biofunctionalization of second-generation Pc PSs CRC via passive nanoparticle carriers or active targeting ligands most definitely allows for enhanced Pc in CRC tumors and so improves PDT treatment.
In a study performed by Sehgal et al. (2013), ZnPcS was conjugated to a monoclonal antibody directed against carcinoembryonic antigen (CEA) to enhance targeted PS uptake in CRC cells.97 This bioconjugate was administered to in vitro co-cultured HT-29 cells and normal colon epithelial cells, and the CRC cells noted a 37 times higher uptake, than when compared to unconjugated ZnPcS administration and normal colon epithelial cells reported no absorption.97 These findings suggest that CEA-mediated effective, as well as selective PS targeting in CRC cells only.97 Additionally, when light excitation at 550 nm was applied to the co-culture, which received this bioconjugate, intense illumination was noted selectively within CRC cells only, with no phototoxicity being reported.97 Thus, this study demonstrated CRC cancer selective targeting of ZnPc–anti-CEA conjugate, suggesting it could be used as a PDD imaging agent for the fluorescent surveillance of colon cancer foci-CEA conjugate as a lead imaging agent for fluorescent surveillance of CRC foci.97 Similarly, a recent study by Brozek-Pluska et al. (2020) reported that ZnPcS4 PS has excitation wavelengths at 532 nm and 689 nm, suggesting that this PS could possibly be successfully utilized within PDT and PDD applications for CRC diagnosis and treatment, however it requires nanocarrier platform investigates to enhance it subcellular uptake and specificity.76 Both these studies confirm the high potential third-generation Pc-antibody nanoconjugates can have in CRC PPD and PDT applications, since targeting Pc-bioconjugates enhanced this PSs uptake and so can allow it to serve as power tools for the photo-diagnosis and treatment of CRC.
7. Conclusions
The prognosis of CRC is generally very poor, since it is difficult to diagnose using conventional methods and so early stage symptoms go by unnoticed, due to their lack of sensitivity, hence patients are often only diagnosed within late stages of this disease.2 Thus, early detection of premalignant CRC tumors is the best chance for improving the survival outcomes of patients.16 Current conventional diagnosis modalities, such as white light colonoscopy is limited, since it lacks overall sensitivity, limiting its detection abilities and simply cannot be used for treatment applications.6
In relation to CRC conventional treatments, their overall effectiveness is highly dependent on the stages, tumor size and advancement of the disease and so a patient's survival rate is often associated with early detection.2,23 Furthermore, even though these conventional treatment modalities are developed to circumvent CRC cancer, they still yield harmful effects post-treatment, and so also affects optimal treatment outcomes.6
It can be seen from this review that PPD and PDT, which are unconventional diagnostic and treatment modalities, that can be administered to CRC patients via blue and red wavelength light regions colonoscopy seems very promising.2 Since both methods are inexpensive, minimally invasive, as well as allow for localized diagnosis and treatment with rapid elimination from the body and so cause negligible side effects.30 Even though both methods are impressive, the do have limitations such as; light penetration depth in tissues and effective PSs biodistribution in CRC tumors.2 These limitations tend to affect the sensitivity abilities of PDD for early fluorescent detection of CRC, as well as hinders the amount of cytotoxic species produced in PDT application to generate effective treatment outcomes.30
These limitations can be clearly observed in first-generation photofrin CRC PSs studies, where limited PDD could be applied and PDT treatments noted poor outcomes with severe skin photosensitivity.55,56 In order to overcome these limitations, several PDD and PDT CRC studies investigated the use of second-generation and third generation PSs, since they are purer compounds, with distinct chemical and optical properties, tend demonstrated minimal toxicity in dark conditions, have longer absorption coefficients in the region of illumination and therapeutic transparency window and are relatively safe.38,48
Within second-generation CRC PSs studies porphyrins such as 5-ALA and chlorins, natural hypericin compounds and phthalocyanines, have been investigated for successful PDD and PDT outcomes. In general, CRC PDD studies noted that these PSs are potentially promising diagnostic tools for CRC, however, still remain limited in relation to their overall sensitivity and specificity to detect early stage CRC. Most studies noted that this limitation was due to their inadequate concentrated absorption in CRC cells and so their targeted uptake required further investigation in order to optimize their use in PDD applications. Similarly, these same second-generation PSs showed improved CRC PDT treatment outcomes, however in general, it was found that due to their hydrophobic nature, their sub-cellular passive absorption in tumors was imperfect, hindering idealistic cytotoxic species generation and overall tumor cell death became rate limited.
The above limitations in relation to second-generation PSs for effective CRC early PDD and enhanced PDT treatment, seems to have been overcome in some cases, whereby researchers functionalized these PS with nanocarriers to enhance PS solubility and so promote passive uptake, as well as combining this strategy with specific CRC targeting biomolecules to enhance PS active absorption.
Within third-generation CRC studies 5-ALA porphyrins, chlorins, hypericin and phthalocyanine PS all noted significant phototoxicity PDT treatment outcomes, when bound to nanocarriers, in comparison to their administration alone. These studies support the suggestion that in order to attain idealistic CRC PDT treatment outcomes nanoparticles are essential components to promote passive PS uptake and ultimate cell death in tumors. Additionally, other studies utilizing third-generation CRC chlorin PSs, went even a step further and introduced checkpoint blockade CRC immunotherapy, which allowed PDT to achieve local tumor destruction, as well as inhibit the growth of distant tumors left behind after treatment, improving overall treatment outcomes. Furthermore, within hypericin third-generation CRC studies researchers attempted to overcome CRC PDT resistance by epigenetically modifying nanocarriers to promote cell death pathways. Within, Pc third-generation CRC PS studies significantly improved PDT treatment outcomes were noted, when these Pc PS nanocarriers were further functionalized with active bio-targeting monoclonal antibodies, since Pcs were actively targeted for concentrated uptake in tumors cells and so tumor cell death was enhanced. In relation to third-generation CRC PS PDD studies, it was found that the only PS to show potential for early diagnosis, as well as combined significant PDT treatment of CRC was targeting Pc-bioconjugates.
Overall, is can be concluded that third-generation PS for the effective photo-diagnosis and treatment of CRC seem promising within in vitro and in vivo studies, however much more research is required into these enhanced nano targeting PS carriers in order to bring them to the forefront of clinical applications.
Conflicts of interest
There are no conflicts to declare.
Acknowledgements
This work is based on the research supported by the South African Research Chairs Initiative of the Department of Science and Technology and National Research Foundation of South Africa (Grant No. 98337), National Research Foundation Thuthuka Fund (Grant No. TTK180409318735) and Cancer Association of South Africa (CANSA) Research Funding Grant. The authors sincerely thank the University of Johannesburg, the National Laser Centre and the African Laser Centre (ALC) for their financial grant support and student monetary support.
References
- G. Abdulrehman, K. Xv, Y. Li and L. Kang, Effects of meta-tetrahydroxyphenylchlorin photodynamic therapy on isogenic colorectal cancer SW480 and SW620 cells with different metastatic potentials, Lasers Med. Sci., 2018, 33, 1581–1590, DOI:10.1007/s10103-018-2524-7.
- C. A. Kruger and H. Abrahamse, Targeted Photodynamic Therapy as Potential Treatment Modality for the Eradication of Colon Cancer, Multidisciplinary Approach for Colorectal Cancer. Intech Open, 2019, DOI:10.5772/intechopen.84760.
- N. Huyghe, P. Baldin and M. Van den Eynde, Immunotherapy with immune checkpoint inhibitors in colorectal cancer: what is the future beyond deficient mismatch-repair tumors?, Gastroenterol. Rep., 2020, 8, 11–24, DOI:10.1093/gastro/goz061.
- M. Brand, P. Gaylard and J. Ramos, Colorectal cancer in South Africa: an assessment of disease presentation, treatment pathways and 5-year survival, S. Afr. Med. J., 2018, 108, 118–122, DOI:10.7196/SAMJ.2017.v108i2.12338.
- F. Bray, J. Ferlay, I. Soerjomataram, R. L. Siegel, L. A. Torre and A. Jemal, Global cancer statistics 2018: GLOBOCAN estimates of incidence and mortality worldwide for 36 cancers in 185 countries, Ca-Cancer J. Clin., 2018, 68, 394–424, DOI:10.3322/caac.21492.
- B. Viswanath, S. Kim and K. Lee, Recent insights into nanotechnology development for detection and treatment of colorectal cancer, Int. J. Nanomed., 2016, 11, 2491–2504, DOI:10.2147/IJN.S108715.
- J. Mishra, J. Drummond, S. H. Quazi, S. S. Karanki, J. J. Shaw, B. Chen and N. Kumar, Prospective of colon cancer treatments and scope for combinatorial approach to enhanced cancer cell apoptosis, Crit. Rev. Oncol. Hematol., 2013, 86, 232–250, DOI:10.1016/j.critrevonc.2012.09.014.
- K. Bibbins-Domingo, D. C. Grossman, S. J. Curry, K. W. Davidson, J. W. Epling Jr, F. A. R. García, M. W. Gillman, D. M. Harper, A. R. Kemper, A. H. Krist, A. E. Kurth, C. S. Landefeld, C. M. Mangione, D. K. Owens, W. R. Phillips, M. G. Phipps, M. P. Pignone and A. L. Siu, Screening for Colorectal Cancer: US Preventive Services Task Force Recommendation Statement, JAMA, 2016, 315, 2564–2575, DOI:10.1001/jama.2016.5989.
- T. Niedermaier, K. Weigl, M. Hoffmeister and H. Brenner, Flexible sigmoidoscopy in colorectal cancer screening: implications of different colonoscopy referral strategies, Eur. J. Epidemiol., 2018, 33, 473–484, DOI:10.1007/s10654-018-0404-x.
- L. L. Azzouz and S. Sharma, Physiology, Large Intestine, in StatPearls, StatPearls Publishing, Treasure Island (FL), 2020, https://www.ncbi.nlm.nih.gov/pubmed/29939634 Search PubMed.
- A. R. Marley and H. Nan, Epidemiology of colorectal cancer, Int. J. Mol. Epidemiol. Genet., 2016, 7, 105–114 Search PubMed , https://www.ncbi.nlm.nih.gov/pubmed/27766137.
- I. Mármol, C. Sánchez-de-Diego, A. Pradilla Dieste, E. Cerrada and M. J. Rodriguez Yoldi, Colorectal Carcinoma: A General Overview and Future Perspectives in Colorectal Cancer, Int. J. Mol. Sci., 2017, 18(1), 197, DOI:10.3390/ijms18010197.
- M. Palaghia, Metastatic Colorectal Cancer: Review of Diagnosis and Treatment Options, J. Surg., 2015, 10 DOI:10.7438/1584-9341-10-4-2.
- N. Hodgkinson, C. A. Kruger and H. Abrahamse, Targeted photodynamic therapy as potential treatment modality for the eradication of colon cancer and colon cancer stem cells, Tumor Biol., 2017, 39, 1010428317734691, DOI:10.1177/1010428317734691.
- I. A. Issa and M. Noureddine, Colorectal cancer screening: an updated review of the available options, World J. Gastroenterol., 2017, 23, 5086–5096, DOI:10.3748/wjg.v23.i28.5086.
- E. J. Kuipers, W. M. Grady, D. Lieberman, T. Seufferlein, J. J. Sung, P. G. Boelens, C. J. H. van de Velde and T. Watanabe, Colorectal cancer, Nat. Rev. Dis. Primers, 2015, 1, 15065, DOI:10.1038/nrdp.2015.65.
- A. Qudayr, A. M. Kabel, M. A. AbdElmaaboud, W. Y. Alghamdi and A. Alghorabi, Colorectal Cancer: New Perspectives, J. Cancer Res. Treat., 2018, 6(3), 80–83, DOI:10.12691/jcrt-6-3-4.
- U. Zaleska-Dorobisz, M. Lasecki, E. Nienartowicz, J. Pelak, J. Słonina, C. Olchowy, M. Scieżka and M. Sąsiadek, Value of Virtual Colonoscopy with 64 Row CT in Evaluation of Colorectal Cancer, Pol. J. Radiol., 2014, 79, 337–343, DOI:10.12659/PJR.890621.
- E. Van Cutsem, H. M. W. Verheul, P. Flamen, P. Rougier, R. Beets-Tan, R. Glynne-Jones and T. Seufferlein, Imaging in Colorectal Cancer: Progress and Challenges for the Clinicians, Cancers, 2016, 8 DOI:10.3390/cancers8090081.
- M. Porru, L. Pompili, C. Caruso, A. Biroccio and C. Leonetti, Targeting KRAS in metastatic colorectal cancer: current strategies and emerging opportunities, J. Exp. Clin. Cancer Res., 2018, 37(1), 57, DOI:10.1186/s13046-018-0719-1.
- M. A. Koc, S. U. Celik and C. Akyol, Colon Cancer, Current Trends in Cancer Management, 2019, DOI:10.5772/intechopen.81597.
- P. Agostinis, K. Berg, K. A. Cengel, T. H. Foster, A. W. Girotti, S. O. Gollnick, S. M. Hahn, M. R. Hamblin, A. Juzeniene, D. Kessel, M. Korbelik, J. Moan, P. Mroz, D. Nowis, J. Piette, B. C. Wilson and J. Golab, Photodynamic therapy of cancer: an update, Ca-Cancer J. Clin., 2011, 61, 250–281, DOI:10.3322/caac.20114.
- K. D. Miller, R. L. Siegel, C. C. Lin, A. B. Mariotto, J. L. Kramer, J. H. Rowland, K. D. Stein, R. Alteri and A. Jemal, Cancer treatment and survivorship statistics, Ca-Cancer J. Clin., 2016, 66, 271–289, DOI:10.3322/caac.21349.
- S. Tohme, R. L. Simmons and A. Tsung, Surgery for Cancer: A Trigger for Metastases, Cancer Res., 2017, 77, 1548–1552, DOI:10.1158/0008-5472.can-16-1536.
- S. Gianfaldoni, R. Gianfaldoni, U. Wollina, J. Lotti, G. Tchernev and T. Lotti, An Overview on Radiotherapy: From Its History to Its Current Applications in Dermatology, Maced. J. Med. Sci., 2017, 5, 521–525, DOI:10.3889/oamjms.2017.122.
- R. Baskar, J. Dai, N. Wenlong, R. Yeo and K.-W. Yeoh, Biological response of cancer cells to radiation treatment, Front. Mol. Biosci., 2014, 1, 24, DOI:10.3389/fmolb.2014.00024.
- P. Wrobel and S. Ahmed, Current status of immunotherapy in metastatic colorectal cancer, Int. J. Colorectal Dis., 2019, 34, 13–25, DOI:10.1007/s00384-018-3202-8.
- A. F. dos Santos, D. R. Q. de Almeida, L. F. Terra, M. S. Baptista and L. Labriola, Photodynamic therapy in cancer treatment – an update review, J. Cancer Metastasis Treat., 2019, 5, 25, DOI:10.20517/2394-4722.2018.83.
- J. Dobson, G. F. de Queiroz and J. P. Golding, Photodynamic therapy and diagnosis: principles and comparative aspects, Vet. J., 2018, 233, 8–18, DOI:10.1016/j.tvjl.2017.11.012.
- C. Naidoo, C. A. Kruger and H. Abrahamse, Simultaneous Photodiagnosis and Photodynamic Treatment of Metastatic Melanoma, Molecules, 2019, 24, 3153, DOI:10.3390/molecules24173153.
- S. Kwiatkowski, B. Knap, D. Przystupski, J. Saczko, E. Kędzierska, K. Knap-Czop, J. Kotlińska, O. Michel, K. Kotowski and J. Kulbacka, Photodynamic therapy – mechanisms, photosensitizers and combinations, Biomed. Pharmacother., 2018, 106, 1098–1107, DOI:10.1016/j.biopha.2018.07.049.
- I. M. Ndhundhuma and H. Abrahamse, A Review of the Photodynamic Application of 5-Aminolevulinic Acid, Hypericin and Phthalocyanines in Dermatology, Medical Technology SA, 2015, 29(2), 20–26 Search PubMed , https://journals.co.za/content/medtech/29/2/EJC187490?TRACK=RSS.
- T. Osaki, I. Yokoe, Y. Sunden, U. Ota, T. Ichikawa, H. Imazato, T. Ishii, K. Takahashi, M. Ishizuka, T. Tanaka, L. Li, M. Yamashita, Y. Murahata, T. Tsuka, K. Azuma, N. Ito, T. Imagawa and Y. Okamoto, Efficacy of 5-Aminolevulinic Acid in Photodynamic Detection and Photodynamic Therapy in Veterinary Medicine, Cancers, 2019, 11, 495, DOI:10.3390/cancers11040495.
- C. A. Kruger and H. Abrahamse, Utilisation of Targeted Nanoparticle Photosensitiser Drug Delivery Systems for the Enhancement of Photodynamic Therapy, Molecules, 2018, 23(10), 2628, DOI:10.3390/molecules23102628.
- I. O. L. Bacellar, T. M. Tsubone, C. Pavani and M. S. Baptista, Photodynamic Efficiency: From Molecular Photochemistry to Cell Death, Int. J. Mol. Sci., 2015, 16, 20523–20559, DOI:10.3390/ijms160920523.
- L. B. Josefsen and R. W. Boyle, Unique diagnostic and therapeutic roles of porphyrins and phthalocyanines in photodynamic therapy, imaging and theranostics, Theranostics, 2012, 2, 916–966, DOI:10.7150/thno.4571.
- H. Abrahamse and I. S. M. Tynga, Photodynamic Therapy, a Potential Therapy for Improve Cancer Management, Breast Cancer and Surgery, ed. N. Bulut, 1st edn, 2018, pp. 181–198, https://www.intechopen.com/books/breast-cancer-and-surgery/photodynamic-therapy-a-potential-therapy-for-improve-cancer-management Search PubMed.
- H. Abrahamse and M. R. Hamblin, New photosensitizers for photodynamic therapy, Biochem. J., 2016, 473, 347–364, DOI:10.1042/BJ20150942.
- E. J. Hong, D. G. Choi and M. S. Shim, Targeted and effective photodynamic therapy for cancer using functionalized nanomaterials, Acta Pharm. Sin. B, 2016, 6, 297–307, DOI:10.1016/j.apsb.2016.01.007.
- V. Rapozzi, E. Della Pietra and B. Bonavida, Dual roles of nitric oxide in the regulation of tumor cell response and resistance to photodynamic therapy, Redox Biol., 2015, 6, 311–317, DOI:10.1016/j.redox.2015.07.015.
- E. Reginato, P. Wolf and M. R. Hamblin, Immune response after photodynamic therapy increases anti-cancer and anti-bacterial effects, World J. Immunol., 2014, 4(1), 1–11, DOI:10.5411/wji.v4.i1.
- R. Baskaran, J. Lee and S. Yang, Clinical development of photodynamic agents and therapeutic applications, Biomater. Res., 2018, 22, 25, DOI:10.1186/s40824-018-0140-z.
- D. van Straten, V. Mashayekhi, H. S. de Bruijn, S. Oliveira and D. J. Robinson, Oncologic Photodynamic Therapy: Basic Principles, Current Clinical Status and Future Directions, Cancers, 2017, 9, 19, DOI:10.3390/cancers9020019.
- R. R. Allison and K. Moghissi, Photodynamic Therapy (PDT): PDT Mechanisms, Clin. Endosc., 2013, 46, 24–29, DOI:10.5946/ce.2013.46.1.24.
- U. Chilakamarthi and L. Giribabu, Photodynamic Therapy: Past, Present and Future, Chem. Rec., 2017, 17, 775–802, DOI:10.1002/tcr.201600121.
- J. Zhang, C. Jiang, J. P. Figueiró Longo, R. B. Azevedo, H. Zhang and L. A. Muehlmann, An updated overview on the development of new photosensitizers for anticancer photodynamic therapy, Acta Pharm. Sin. B, 2018, 8, 137–146, DOI:10.1016/j.apsb.2017.09.003.
- G. M. F. Calixto, J. Bernegossi, L. M. de Freitas, C. R. Fontana and M. Chorilli, Nanotechnology-Based Drug Delivery Systems for Photodynamic Therapy of Cancer: A Review, Molecules, 2016, 21, 342, DOI:10.3390/molecules21030342.
- I. Yoon, J. Z. Li and Y. K. Shim, Advance in photosensitizers and light delivery for photodynamic therapy, Clin. Endosc., 2013, 46, 7–23, DOI:10.5946/ce.2013.46.1.7.
- J. Kou, D. Dou and L. Yang, Porphyrin photosensitizers in photodynamic therapy and its applications, Oncotarget, 2017, 8, 81591–81603, DOI:10.18632/oncotarget.20189.
- H. Park, S.-H. Ko, J. M. Lee, J. H. Park and Y.-H. Choi, Troglitazone Enhances the Apoptotic Response of DLD-1 Colon Cancer Cells to Photodynamic Therapy, Yonsei Med. J., 2016, 57, 1494, DOI:10.3349/ymj.2016.57.6.1494.
- L. Benov, Photodynamic therapy: current status and future directions, Med. Princ. Pract., 2015, 24, 14–28, DOI:10.1159/000362416.
- M. Q. Mesquita, C. J. Dias, S. Gamelas, M. Fardilha, G. P. M. Maria and M. A. F. Faustino, An insight on the role of photosensitizer nanocarriers for Photodynamic Therapy, An. Acad. Bras. Cienc., 2018, 90, 1101–1130, DOI:10.1590/0001-3765201720170800.
- T. A. Debele, S. Peng and H.-C. Tsai, Drug Carrier for Photodynamic Cancer Therapy, Int. J. Mol. Sci., 2015, 16, 22094–22136, DOI:10.3390/ijms160922094.
- A. Kawczyk-Krupka, A. M. Bugaj, W. Latos, K. Zaremba, K. Wawrzyniec, M. Kucharzewski and A. Sieroń, Photodynamic therapy in colorectal cancer treatment—the state of the art in preclinical research, Photodiagn. Photodyn. Ther., 2016, 13, 158–174, DOI:10.1016/j.pdpdt.2015.07.175.
- M. Kohno, M. Takeda, Y. Niwano, R. Saito, N. Emoto, M. Tada, T. Kanazawa, N. Ohuchi and R. Yamada, Early diagnosis of cancer by detecting the chemiluminescence of hematoporphyrins in peripheral blood lymphocytes, Tohoku J. Exp. Med., 2008, 216, 47–52, DOI:10.1620/tjem.216.47.
- T. S. Zavadskaya, Photodynamic therapy in the treatment of glioma, Exp. Oncol., 2015, 37, 234–241 CrossRef , https://www.ncbi.nlm.nih.gov/pubmed/26710833.
- B. O. Sun, W. Li and N. Liu, Curative effect of the recent photofrin photodynamic adjuvant treatment on young patients with advanced colorectal cancer, Oncol. Lett., 2016, 11, 2071–2074, DOI:10.3892/ol.2016.4179.
- S. Singh, A. Aggarwal, N. V. S. D. K. Bhupathiraju, G. Arianna, K. Tiwari and C. M. Drain, Glycosylated Porphyrins, Phthalocyanines, and Other Porphyrinoids for Diagnostics and Therapeutics, Chem. Rev., 2015, 115, 10261–10306, DOI:10.1021/acs.chemrev.5b00244.
- M. Ishizuka, F. Abe, Y. Sano, K. Takahashi, K. Inoue, M. Nakajima, T. Kohda, N. Komatsu, S.-I. Ogura and T. Tanaka, Novel development of 5-aminolevurinic acid (ALA) in cancer diagnoses and therapy, Int. Immunopharmacol., 2011, 11, 358–365, DOI:10.1016/j.intimp.2010.11.029.
- K. M. Tewari and I. M. Eggleston, Chemical approaches for the enhancement of 5-aminolevulinic acid-based photodynamic therapy and photodiagnosis, Photochem. Photobiol. Sci., 2018, 17, 1553–1572, 10.1039/c8pp00362a.
- K. R. Rollakanti, S. C. Kanick, S. C. Davis, B. W. Pogue and E. V. Maytin, Techniques for fluorescence detection of protoporphyrin IX in skin cancers associated with photodynamic therapy, Photonics Lasers Med., 2013, 2, 287–303, DOI:10.1515/plm-2013-0030.
- K. Inoue, H. Fukuhara, T. Shimamoto, M. Kamada, T. Iiyama, M. Miyamura, A. Kurabayashi, M. Furihata, M. Tanimura, H. Watanabe and Others, Comparison between intravesical and oral administration of 5-aminolevulinic acid in the clinical benefit of photodynamic diagnosis for nonmuscle invasive bladder cancer, Cancer, 2012, 118, 1062–1074 CrossRef CAS , https://acsjournals.onlinelibrary.wiley.com/doi/abs/10.1002/cncr.26378.
- M. Nakamura, J. Nishikawa, K. Hamabe, A. Goto, J. Nishimura, H. Shibata, M. Nagao, S. Sasaki, S. Hashimoto, T. Okamoto and I. Sakaida, Preliminary study of photodynamic diagnosis using 5-aminolevulinic acid in gastric and colorectal tumors, World J. Gastroenterol., 2015, 21, 6706–6712, DOI:10.3748/wjg.v21.i21.6706.
- E. V. Filonenko, A. D. Kaprin, A. A. Raszhivina, A. N. Urlova and A. M. Nechipai, Fluorescence Diagnostics of Colon Malignant and Premalignant Lesions Using 5-Aminolevulinic Acid, Int. J. Photoenergy, 2014, 2014, 378673, DOI:10.1155/2014/378673.
- T. Namikawa, K. Inoue, S. Uemura, M. Shiga, H. Maeda, H. Kitagawa, H. Fukuhara, M. Kobayashi, T. Shuin and K. Hanazaki, Photodynamic diagnosis using 5-aminolevulinic acid during gastrectomy for gastric cancer, J. Surg. Oncol., 2014, 109, 213–217, DOI:10.1002/jso.23487.
- T. Hatakeyama, Y. Murayama, S. Komatsu, A. Shiozaki, Y. Kuriu, H. Ikoma, M. Nakanishi, D. Ichikawa, H. Fujiwara, K. Okamoto and T. Ochiai, Efficacy of 5-aminolevulinic acid-mediated photodynamic therapy using light-emitting diodes in human colon cancer cells, Oncol. Rep., 2013, 29(3), 911–916, DOI:10.3892/or.2013.2220.
- A. Kawczyk-Krupka, A. M. Bugaj, W. Latos, K. Zaremba, K. Wawrzyniec and A. Sieroń, Photodynamic therapy in colorectal cancer treatment: the state of the art in clinical trials, Photodiagn. Photodyn. Ther., 2015, 12, 545–553, DOI:10.1016/j.pdpdt.2015.04.004.
- Y. Kaizhen, N. Ting, L. Mengyu, T. Lihua and K. Ling, Enhanced cytotoxicity and apoptosis through inhibiting autophagy in metastatic potential colon cancer SW620 cells treated with Chlorin e6 photodynamic therapy, Photodiagn. Photodyn. Ther., 2018, 24, 332–334, DOI:10.1016/j.pdpdt.2018.10.012.
- E. Kukcinaviciute, A. Sasnauskiene, D. Dabkeviciene, V. Kirveliene and V. Jonusiene, Effect of mTHPC-mediated photodynamic therapy on 5-fluorouracil resistant human colorectal cancer cells, Photochem. Photobiol. Sci., 2017, 16(7), 1063–1070 RSC , https://pubmed.ncbi.nlm.nih.gov/28509917/.
- G. Ouyang, L. Xiong and Z. Liu, et al., Inhibition of autophagy potentiates the apoptosis-inducing effects of photodynamic therapy on human colon cancer cells, Photodiagn. Photodyn. Ther., 2018, 21, 396–403 CrossRef CAS , https://pubmed.ncbi.nlm.nih.gov/29355734/.
- C. Lange, C. Lehmann, M. Mahler and P. J. Bednarski, Comparison of Cellular Death Pathways after mTHPC-mediated Photodynamic Therapy (PDT) in Five Human Cancer Cell Lines, Cancers, 2019, 11(5), 702–710, DOI:10.3390/cancers11050702.
- K. Shimizu, M. Nitta, T. Komori, T. Maruyama, T. Yasuda, Y. Fujii, K. Masamune, T. Kawamata, T. Maehara and Y. Muragaki, Intraoperative Photodynamic Diagnosis Using Talaporfin Sodium Simultaneously Applied for Photodynamic Therapy against Malignant Glioma: A Prospective Clinical Study, Front. Neurol., 2018, 9, 24, DOI:10.3389/fneur.2018.00024.
- Z. Jendželovská, R. Jendželovský, B. Kuchárová and P. Fedoročko, Hypericin in the Light and in the Dark: Two Sides of the Same Coin, Front. Plant Sci., 2016, 7, 560, DOI:10.3389/fpls.2016.00560.
- M. Kaleta-Richter, D. Aebisher, D. Jaworska, Z. Czuba, G. Cieślar and A. Kawczyk-Krupka, The Influence of Hypericin-Mediated Photodynamic Therapy on Interleukin-8 and -10 Secretion in Colon Cancer Cells, Integr. Cancer Ther., 2020, 19, 1534735420918931, DOI:10.1177/1534735420918931.
- P. R. Sekhejane, N. N. Houreld and H. Abrahamse, Multiorganelle Localization of Metallated Phthalocyanine Photosensitizer in
Colorectal Cancer Cells (DLD-1 and CaCo-2) Enhances Efficacy of Photodynamic Therapy, Int. J. Photoenergy, 2014, 1–10, DOI:10.1155/2014/383027.
- B. Brozek-Pluska, A. Jarota, R. Kania and H. Abramczyk, Zinc Phthalocyanine Photochemistry by Raman Imaging, Fluorescence Spectroscopy and Femtosecond Spectroscopy in Normal and Cancerous Human Colon Tissues and Single Cells, Molecules, 2020, 25 DOI:10.3390/molecules25112688.
- N. Mehraban and H. S. Freeman, Developments in PDT Sensitizers for Increased Selectivity and Singlet Oxygen Production, Materials, 2015, 8, 4421–4456, DOI:10.3390/ma8074421.
- Y. Hu and K. Masamune, Flexible laser endoscope for minimally invasive photodynamic diagnosis (PDD) and therapy (PDT) toward efficient tumor removal, Opt. Express, 2017, 25, 16795–16812, DOI:10.1364/OE.25.016795.
- J. K. Patra, G. Das, L. F. Fraceto, E. V. R. Campos, M. del Pilar Rodriguez-Torres, L. S. Acosta-Torres, L. A. Diaz-Torres, R. Grillo, M. K. Swamy, S. Sharma, S. Habtemariam and H. S. Shin, Nano based drug delivery systems: recent developments and future prospects, J. Nanobiotechnol., 2018, 16, 71, DOI:10.1186/s12951-018-0392-8.
- C. W. Chung, K.-D. Chung, Y.-I. Jeong and D. H. Kang, 5-aminolevulinic acid-incorporated nanoparticles of methoxy poly(ethylene glycol)–chitosan copolymer for photodynamic therapy, Int. J. Nanomed., 2013, 8, 809–819, DOI:10.2147/IJN.S39615.
- L. Bretin, A. Pinon, S. Bouramtane, C. Ouk, L. Richard, M.-L. Perrin, A. Chaunavel, C. Carrion, F. Bregier, V. Sol, V. Chaleix, D. Y. Leger and B. Liagre, Photodynamic Therapy Activity of New Porphyrin–Xylan-Coated Silica Nanoparticles in Human Colorectal Cancer, Cancers, 2019, 11 DOI:10.3390/cancers11101474.
- D. Jocham, H. Stepp and R. Waidelich, Photodynamic diagnosis in urology: state-of-the-art, Eur. Urol., 2008, 53, 1138–1148, DOI:10.1016/j.eururo.2007.11.048.
- J. F. Georges, A. Valeri, H. Wang, A. Brooking, M. Kakareka, S. S. Cho, Z. Al-Atrache, M. Bamimore, H. Osman, J. Ifrach, S. Yu, C. Li, D. Appelt, J. Y. K. Lee, P. Nakaji, K. Brill and S. Yocom, Delta-Aminolevulinic Acid-Mediated Photodiagnoses in Surgical Oncology: A Historical Review of Clinical Trials, Front. Surg., 2019, 6, 45, DOI:10.3389/fsurg.2019.00045.
- A. I. Gavrina, M. V. Shirmanova, N. A. Aksenova, D. V. Yuzhakova, L. B. Snopova, A. B. Solovieva, P. S. Timashev, V. V. Dudenkova and E. V. Zagaynova, Photodynamic therapy of mouse tumor model using chlorin e6- polyvinyl alcohol complex, J. Photochem. Photobiol., B, 2018, 178, 614–622, DOI:10.1016/j.jphotobiol.2017.12.016.
- P. Sundaram and H. Abrahamse, Effective Photodynamic Therapy for Colon Cancer Cells Using Chlorin e6 Coated Hyaluronic Acid-Based Carbon Nanotubes, Int. J. Mol. Sci., 2020, 21, 4745, DOI:10.3390/ijms21134745.
- J. Xu, L. Xu, C. Wang, R. Yang, Q. Zhuang, X. Han, Z. Dong, W. Zhu, R. Peng and Z. Liu, Near-Infrared-Triggered Photodynamic Therapy with Multitasking Upconversion Nanoparticles in Combination with Checkpoint Blockade for Immunotherapy of Colorectal Cancer, ACS Nano, 2017, 11, 4463–4474, DOI:10.1021/acsnano.7b00715.
- K. Lu, C. He, N. Guo, C. Chan, K. Ni, R. R. Weichselbaum and W. Lin, Chlorin-Based Nanoscale Metal–Organic Framework Systemically Rejects Colorectal Cancers via Synergistic Photodynamic Therapy and Checkpoint Blockade Immunotherapy, J. Am. Chem. Soc., 2016, 138, 12502–12510, DOI:10.1021/jacs.6b06663.
- M. Tanaka, H. Kataoka, M. Mabuchi, S. Sakuma, S. Takahashi, R. Tujii, H. Akashi, H. Ohi, S. Yano, A. Morita and T. Joh, Anticancer effects of novel photodynamic therapy with glycoconjugated chlorin for gastric and colon cancer, Anticancer Res., 2011, 31(3), 763–769 CAS , PMID: 21498693, https://pubmed.ncbi.nlm.nih.gov/21498693/.
- L. Mikešová, J. Mikeš, J. Kovaľ and K. Gyurászová, Conjunction of glutathione level, NAD(P)H/FAD redox status and hypericin content as a potential factor affecting colon cancer cell resistance to photodynamic therapy, Photodiagn. Photodyn. Ther., 2013, 10(4), 470–483 CrossRef , https://www.sciencedirect.com/science/article/pii/S1572100013000513.
- M. I. Khot, S. L. Perry, T. Maisey, G. Armstrong, H. Andrew, T. A. Hughes, N. Kapur and D. G. Jayne, Inhibiting ABCG2 could potentially enhance the efficacy of hypericin-mediated photodynamic therapy in spheroidal cell models of colorectal cancer, Photodiagn. Photodyn. Ther., 2018, 23, 221–229, DOI:10.1016/j.pdpdt.2018.06.027.
- A. Halaburková, R. Jendželovský, J. Kovaľ, Z. Herceg, P. Fedoročko and A. Ghantous, Histone deacetylase inhibitors potentiate photodynamic therapy in colon cancer cells marked by chromatin-mediated epigenetic regulation of CDKN1A, Clin. Epigenet., 2017, 9, 62, DOI:10.1186/s13148-017-0359-x.
- M. C. Montanha, L. L. Silva, F. B. B. Pangoni, G. B. Cesar, R. S. Gonçalves, W. Caetano, N. Hioka, T. T. Tominaga, M. E. L. Consolaro, A. Diniz and E. Kimura, Response surface method optimization of a novel hypericin formulation in P123 micelles for colorectal cancer and antimicrobial photodynamic therapy, J. Photochem. Photobiol., B, 2017, 170, 247–255, DOI:10.1016/j.jphotobiol.2017.04.008.
- M. N. Sardoiwala, A. C. Kushwaha, A. Dev, N. Shrimali, P. Guchhait, S. Karmakar and S. Roy Choudhury, Hypericin-Loaded Transferrin Nanoparticles Induce PP2A-Regulated BMI1 Degradation in Colorectal Cancer-Specific Chemo-Photodynamic Therapy, ACS Biomater. Sci. Eng., 2020, 6, 3139–3153, DOI:10.1021/acsbiomaterials.9b01844.
- N. Chiarante, M. C. García Vior, J. Awruch, J. Marino and L. P. Roguin, Phototoxic action of a zinc(II) phthalocyanine encapsulated into poloxamine polymeric micelles in 2D and 3D colon carcinoma cell cultures, J. Photochem. Photobiol., B, 2017, 170, 140–151, DOI:10.1016/j.jphotobiol.2017.04.009.
- N. Chiarante, M. D. Vega, F. Valli, E. Zotta, H. Daghero, T. Basika, M. Bollati-Fogolin, M. C. García Vior, J. Marino and L. P. Roguin, In Vivo Photodynamic Therapy With a Lipophilic Zinc(II) Phthalocyanine Inhibits Colorectal Cancer and Induces a Th1/CD8 Antitumor Immune Response, Lasers Surg. Med., 2020 DOI:10.1002/lsm.23284.
- G. Obaid, I. Chambrier, M. J. Cook and D. A. Russell, Cancer targeting with biomolecules: a comparative study of photodynamic therapy efficacy using antibody or lectin conjugated phthalocyanine–PEG gold nanoparticles, Photochem. Photobiol. Sci., 2015, 14, 737–747, 10.1039/c4pp00312h.
- I. Sehgal, H. Li, B. Ongarora, D. Devillier and M. G. H. Vicente, Synthesis and biological investigations of a ZnPc–antiCEA bioconjugate for imaging of colorectal cancer, J. Porphyrins Phthalocyanines, 2013, 17, 150–156, DOI:10.1142/S108842461250143X.
|
This journal is © The Royal Society of Chemistry 2020 |
Click here to see how this site uses Cookies. View our privacy policy here.