DOI:
10.1039/C9RA09270F
(Paper)
RSC Adv., 2020,
10, 1757-1768
A direct Z-scheme Bi2WO6/NH2-UiO-66 nanocomposite as an efficient visible-light-driven photocatalyst for NO removal†
Received
8th November 2019
, Accepted 23rd December 2019
First published on 8th January 2020
Abstract
To explore an efficient photocatalyst for NO pollution, a direct Z-scheme photocatalytic system is successfully fabricated by coupling Bi2WO6 with NH2-UiO-66 via a simple hydrothermal synthesis technique. The Z-scheme system promotes the NO photocatalytic oxidation activity with an optimum NO removal rate of 79%, which is 2.7 and 1.2 times that obtained by using only pristine Bi2WO6 and NH2-UiO-66, respectively. Simultaneously, superior selectivity for converting NO to NO3−/NO2− is observed. The enhanced photocatalytic performance of the Bi2WO6/NH2-UiO-66 hybrids is attributed to the following two aspects: (i) large specific area of NH2-UiO-66, which exposes more active sites and is beneficial to the adsorption and activation of NO; (ii) outstanding Z-scheme structure constructed between BiWO6 and NH2-UiO-66, which can improve the efficiency of the separation of electron–hole pairs and preserves the strong oxidation ability of hybrids. ESR analysis shows that ·O2− and ·OH contribute to NO removal. A possible photocatalytic mechanism of NO oxidation on the direct Z-scheme photocatalyst (BWO/2NU) under visible light irradiation is proposed. This work displays the BWO/2NU hybrid's potential for treating low-concentration air pollutants, and the proposed Z-scheme photocatalyst design and promotion mechanism may inspire more rational synthesis of highly efficient photocatalysts for NO removal.
1. Introduction
Rapid industrialization and urbanization have led to a sharp increase in harmful gas emissions, such as volatile organic compounds (VOCs) and nitrogen oxides (NOx), which have become the most significant pollutants in prominent environmental issues.1,2 NO is a typical air pollutant and results in many hazards, such as tropospheric ozone depletion, global climate warming, acid rain, and human diseases.3 Different techniques including physical and chemical adsorption,4 heterogeneous catalytic reduction,5 and oxidation and so on6 are usually adopted to eliminate NO from gas emissions from combustion sources. However, these technologies are usually employed to treat NO at relatively high concentrations.7 For practical ppb-level NO, it widely exists in both indoor and outdoor atmospheric environments, which has severe impacts on ecology and human health. Photocatalytic oxidation appears to be an environment friendly and easy approach to removing NO at ppb levels in air.3,8,9
Among the various reported photocatalysts, it's difficult for a single-component material to achieve superior performance, including stability, effective separation of photogenerated charges, utilization efficiency of solar energy and strong redox ability.10 Therefore, a variety of strategies have been developed and applied to improve the performance of single-component materials, such as size and morphology control,11 surface modification,12 defect engineering13 and heterojunction construction.14 Among them, the constructed heterojunctions are promising due to high separation efficiency of electron–hole pairs, including type II heterojunctions and Z-scheme photocatalysts and so on. In comparison with type II heterojunctions, Z-scheme photocatalytic system can minimize unfavorable backward reaction at two different redox sites during the photocatalytic process15 and preserve the higher redox potentials of the photo-induced charges, showing excellent photocatalytic performance.14,16–18 Thus, direct Z-scheme photocatalysts have been studied and applied,15,16,19,20 ever since Yu et al. pioneered the direct Z-scheme photocatalyst g–C3N4–TiO2 and applied it to decompose formaldehyde in air.21
Bismuth tungstate (Bi2WO6), an environment-friendly material, has been widely studied and applied in photocatalysis owing to its unique properties, such as nontoxicity, a visible light response range and a regulable band gap of 2.75 eV.22 Moreover, its unique band structure makes the valence band largely dispersed and does benefits to the mobility of photo-induced carriers.23 However, a few drawbacks limit the photocatalytic performance of Bi2WO6, including high recombination of photoinduced electron–hole pairs and low utilization of visible light.24 Therefore, it is imperative to overcome these disadvantages. Coupling Bi2WO6 with other materials to construct Z-scheme heterojunction photocatalysts could help achieve this objective. For example, Hu et al.25 designed and synthesized a Z-scheme photocatalytic system (2D/2D BP/monolayer Bi2WO6). The Z-scheme photocatalyst showed superior performance in photocatalytic water splitting and photocatalytic NO removal. And the NO removal ratio was as high as 67%.
NH2-UiO-66, a typical metal–organic frameworks (MOFs) material, have been widely applied in different areas owing to their high specific surface areas, tailorable pore sizes, easy functional groups, stability and designed frame structures.26 Combing NH2-UiO-66 with Bi2WO6 can provide extra pathways for the charge migration and facilitate the efficient separation of photo-induced carriers. In addition, the incorporation may lead to the formation of a Z-scheme photocatalytic system and maintaining high redox potential, which are beneficial for good catalytic activity and selectivity.27
Here, we constructed a novel direct Z-scheme photocatalyst to promote NO removal and selectivity at a ppb level under visible light irradiation in a continuous gas flow. In our work, Bi2WO6 and NH2-UiO-66 were chosen to form an interactive interface between them via a simple hydrothermal method, and a novel direct Z-scheme system, Bi2WO6/xNH2-UiO-66 (BWO/xNU), was fabricated. On the one hand, bismuth tungstate (Bi2WO6), an environment-friendly material, is being extensively studied for photocatalysis owing to unique properties. NH2-UiO-66 displays superior features in owing to large surface areas, high stability, visible-light response, and semiconductor properties.27–31 One the other hand, the band position of NH2-UiO-66 matched well with that of Bi2WO6. Combining NH2-UiO-66 with Bi2WO6 can lead to forming a Z-scheme band structure, which ensures high separation efficiency of photogenerated electrons and holes. More importantly, the BWO/xNU hybrids can preserve the strong oxidation ability on the valence band (VB) of NH2-UiO-66 and high reduction ability on the conduction band (CB) of Bi2WO6 simultaneously. The as-prepared BWO/xNU hybrids exhibited both higher photocatalytic ability for NO oxidation and improved selectivity for NO3−/NO2− conversion. Hence, this work provides a basis for designing a MOFs-based direct Z-scheme photocatalytic system, simultaneously improving photocatalytic activity and selectivity for NO oxidation, and presents a mechanism of Z-scheme photocatalytic reaction for NO oxidation.
2. Experimental
2.1 Materials
Zirconium tetrachloride (ZrCl4), N,N-dimethyl formamide (DMF), glacial acetic acid (HAc), and methanol were obtained from Sinopharm Chemical Reagent Co., Ltd. Na2WO4·2H2O and Bi(NO3)3·5H2O were purchased from Aladdin Industrial Corporation. 2-NH2-terephthalic acid was procured from Sigma-Aldrich Chemistry. All the reagents were of analytical grade and were used without further purification.
2.2 Synthesis
2.2.1 Synthesis of NH2-UiO-66. NH2-UiO-66 was synthesized in accordance with a procedure reported in the literature.32 In a typical synthesis, ZrCl4 (0.136 g, 0.585 mmol) and 2-NH2-terephthalic acid (0.106 g, 0.585 mmol) were dissolved in DMF (130 mL) containing 15.6 mL HAc, and the solution was transferred to a 200 mL Teflon-lined stainless steel autoclave. The autoclave was sealed and heated in an oven at 120 °C for 24 h under autogenous pressure. Until it cooled naturally to room temperature, the solid particles were obtained via filtration with a mixture of methanol and DMF (v/v = 1
:
4) to remove residual DMF. The obtained pale yellow product was dried in vacuum at 90 °C for 12 h.
2.2.2 Synthesis of Bi2WO6/NH2-UiO-66. The Bi2WO6/NH2-UiO-66 composites were synthesized via a simple one-step solvothermal method.28 In detail, Na2WO4·2H2O (0.142 g, 0.430 mmol) was dissolved in deionized water (60 mL), vigorously stirring at room temperature. A series of different Bi2WO6/NH2-UiO-66 mass ratios of 1
:
0.5, 1
:
1, 1
:
2, 1
:
3, and 1
:
5 was obtained by adding 0.15 g, 0.30 g, 0.60 g, 0.90 g, and 1.20 g, respectively, of NH2-UiO-66 to the Na2WO4 solution, and the resultant mixtures were stirred for 0.5 h. Then, Bi(NO3)3·5H2O (0.417 mg, 0.860 mmol) was added to DMF (60 mL) to form a homogenous white solution. Subsequently, the reaction precursor was stirred for 1 h. And then, it was transferred to a 200 mL Teflon-lined stainless steel autoclave, where the reaction proceeded at 120 °C for 12 h. After naturally cooling, the final products were collected by centrifugation, washed with deionized water, and dried at 60 °C for 12 h. According to the Bi2WO6/NH2-UiO-66 mass ratios, the samples were denoted as BWO/xNU; the labels BWO/0.5NU, BWO/NU, BWO/2NU, BWO/3NU, and BWO/5NU, correspond to Bi2WO6/NH2-UiO-66 mass ratios of 1
:
0.5, 1
:
1, 1
:
2, 1
:
3, and 1
:
5, respectively.
2.3 Characterization
The crystal phases of the samples were observed via X-ray diffraction (XRD) with Cu Kα radiation (Shimadzu XRD-6000, Japan). The morphologies and structures of the catalysts were characterized using a scanning electron microscopy (SEM, SU-8010, Hitachi, Japan) and a transmission electron microscopy (TEM, H-9500, HITACHI, Japan). The N2 adsorption/desorption isotherms were collected on an N2 adsorption apparatus (JW-BK 132F, Beijing JWGB Sci & Tech Co., China) and used the Brunauer–Emmett–Teller (BET) method. The pore size distribution was acquired via the Barrett–Joyner–Halenda (BJH) desorption method. X-ray photoelectron spectroscopy (XPS) experiments were carried out on an XPS instrument (ESCALAB 250Xi, Thermo, USA) to investigate the surface chemical compositions. The binding energies were referenced to C 1s at 284.8 eV. To explore the light absorption properties, the ultraviolet-visible light diffuse reflection spectra (UV-vis DRS) were obtained using a UV-vis diffuse reflection spectrophotometer (Lambda 750 S, PerkinElmer, USA) with a spectral range 200–800 nm. The electron spin resonance (ESR) signals of spin-trapped radicals were detected using a JES FA200 spectrometer (JEOL Ltd., Japan). The photoluminescence spectra were obtained using a fluorescence spectrometer (FLS920, Edinburgh Instruments, England) and the excitation source used was a laser source emitting at 380 nm. The photocurrent, electrochemical AC impedance, and the parameters required for Mott–Schottky plots were measured using an electrochemical workstation (CHI 660E, China). And standard three-electrode system and 0.2 M Na2SO4 aqueous solution as the electrolyte are necessary for the electrochemical workstation.
2.4 Photocatalytic activity tests
The photocatalytic activity for NO removal was performed at room temperature in a continuous-flow stainless steel rectangular reactor. There are five channels in the reactor, and photocatalyst (50 mg) was dispersed uniformly on five glass slides, which were placed in the channels. The light source for photocatalytic reaction used a 500 W Xenon lamp (CEL-LAX500, Beijing AuLight Tech Co., Ltd, China), equipped with a 420 nm UV cut-off filter. The NO gas used in the tests was continuously obtained from a NO compressed gas cylinder (50 ppm, N2 balance). Air stream was used to dilute the concentration of NO to around 500 ppb. Simultaneously, air steam was delivered through a bubbler to adjust the relative humidity (RH) of the reaction gas to 55%. The volume flow rate of the reaction gas was about 0.8 L min−1, with corresponding residence time being 18.75 s. NO adsorption–desorption equilibrium should be achieved before switching on the Xe lamp with a current of 7 A. The concentrations of NO, NO2, and NOx were recorded using an online analyzer (NO–NO2–NOx Analyzer, Model 42i, Thermo Scientific). Based on the following formulae, NO and NOx removal efficiencies could be calculated.
NO removal = (NOin − NOout)/NOin, |
NO2 formation = NOx,out − NOout, |
where NOin/out represent the concentrations of NO at the inlet and outlet, respectively. And NOx,in/out has the similar meaning.
3. Results and discussion
3.1 Crystal phases and structures
To confirm the phases and crystal structures of pure NH2-UiO-66, Bi2WO6, and the BWO/xNU nanocomposites with different mass ratios of NH2-UiO-66, the catalysts were characterized via XRD. The XRD results are shown in Fig. S1† and 1a. The XRD patterns of pure NH2-UiO-66 are consistent with those reported previously,26,28,32,33 while pristine Bi2WO6 shows the characteristic peaks ascribed to russellite (JCPDS: 79-2020). After combining Bi2WO6 with NH2-UiO-66, similar XRD patterns at 2θ = 5–10° are observed in all of the as-prepared catalysts, which are ascribed to NH2-UiO-66.26,28,32,33 Compared with those of pure NH2-UiO-66, the characteristic peaks of BWO/xNU at low angles become weaker as the Bi2WO6 content increases. What is noteworthy in Fig. 1b is that the diffraction peaks shift to lower angles at 2θ = 7–9°. According to Bragg's law, the negative shift can be ascribed to the lattice expansion of NH2-UiO-66 in the hybrids.34 Moreover, no other impurity peaks can be seen, which indicates that the BWO–NU heterojunctions are successfully obtained and BWO/xNU is a two-phase composite.35
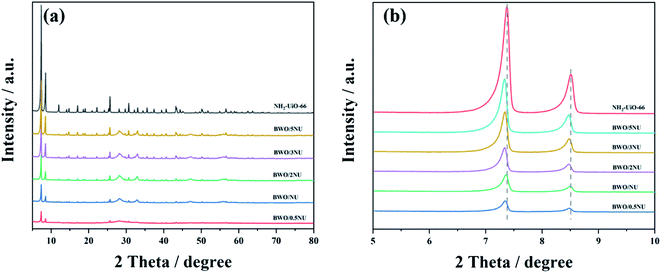 |
| Fig. 1 (a) XRD patterns of NH2-UiO-66 and BWO/xNU samples, and (b) diffraction peaks of pure NH2-UiO-66 and of BWO/xNU ascribed to NH2-UiO-66. | |
The samples's SEM images are shown in Fig. 2. As illustrated in Fig. 2a, pure NH2-UiO-66 displays a regular octahedral cubic morphology with clean and smooth surfaces and its size is approximately 200–500 nm. Pristine Bi2WO6 exhibits nanoparticle-like morphology (Fig. 2d). Interestingly, after the Bi2WO6 loading process, the NH2-UiO-66's surface from BWO/2NU hybrid becomes rough (Fig. 2b and c). In the SEM patterns of BWO/2NU (Fig. 2b and c), large number of nanoplates insert among the NH2-UiO-66 nanocubes. We believe that these nanoplates are Bi2WO6, introduced by the addition of NH2-UiO-66 during the growth of Bi2WO6. To further confirmation, TEM and energy dispersive spectroscopy (EDS) analyses were conducted. These Bi2WO6 nanoplates decorate and disperse on the surface of NH2-UiO-66.
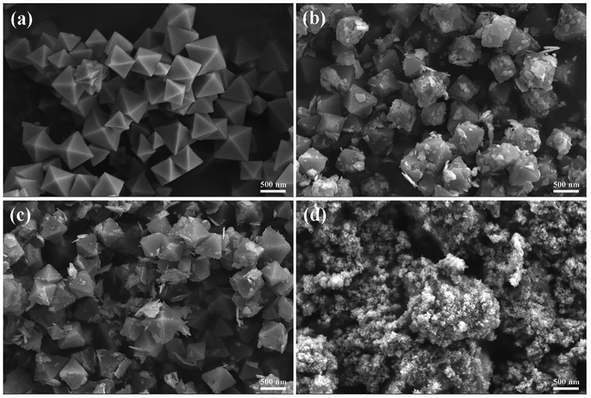 |
| Fig. 2 (a) SEM patterns of NH2-UiO-66 samples, (b and c) SEM patterns of BWO/2NU samples, and (d) SEM patterns of Bi2WO6 samples. | |
The morphology and structure of samples were additionally analyzed via TEM and high-resolution TEM (HRTEM). It is shown in Fig. 3a that pure NH2-UiO-66 has a cube-like morphology, of which size is approximately 200–500 nm. The results are in accordance with the SEM analysis. After hybridization with Bi2WO6 (Fig. 3b and c), the NH2-UiO-66 in the BWO/2NU hybrids can still be observed. Meanwhile, Bi2WO6 displays a nanoplate-like morphology. Further observations show that NH2-UiO-66 and Bi2WO6 are closely bonded, which is consistent with the XRD and SEM analysis. This structure does benefits to the rapid separation of photo-induced charges between NH2-UiO-66 and Bi2WO6 with improving its photocatalytic activity. Additionally, pristine Bi2WO6 displays the morphology of small nanoparticles (Fig. S2†), which differs from that of Bi2WO6 in the BWO/2NU hybrid. The HRTEM image (Fig. 3d) of as-prepared Bi2WO6 shows clear fringes. And lattice spacings of 0.248 nm and 0.255 nm are measured, which can be assigned to the (115) and (022) planes of russellite Bi2WO6, respectively. The EDS spectrum (Fig. 3e and f) confirms that the BWO/2NU hybrid contains elements including Zr, Bi, W, C, N, and O.
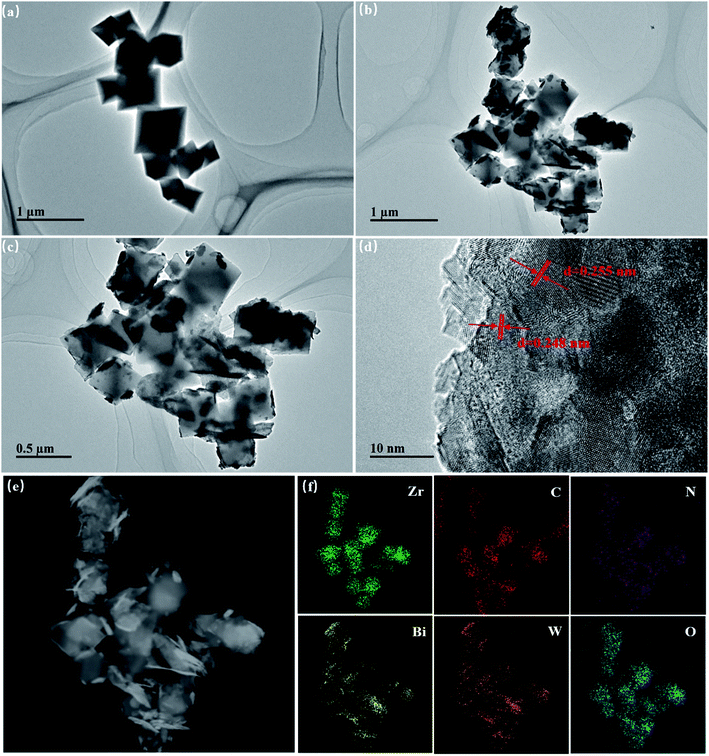 |
| Fig. 3 TEM image of (a) NH2-UiO-66 samples, (b and c) BWO/2NU samples, (d) HRTEM image of Bi2WO6 samples, and (e and f) EDS elemental mapping images of BWO/2NU samples. | |
The N2 adsorption–desorption measurements were performed to explore the BET surface areas and pore structures of the as-prepared photocatalysts. The results are shown in Fig. 4. NH2-UiO-66 has a large surface area (673.58 m2 g−1) and its isotherm is of type I, which indicates that the NH2-UiO-66 sample has a microporous structure.36–38 The BET surface area of pure Bi2WO6 is 52.67 m2 g−1 (Fig. 4), which is relatively low. Moreover, the isotherm of pure Bi2WO6 has a H3 type hysteresis loop, indicating its mesoporous structure. The BET surface areas of BWO/2NU and BWO/0.5NU are 685.41 m2 g−1 and 313.20 m2 g−1, respectively (Fig. 4). After the combination of Bi2WO6 and NH2-UiO-66, the specific surface areas of different samples increase with decrease in the content of Bi2WO6. It's worth noting that the N2 adsorption–desorption isotherms of all the hybrids present obvious hysteresis loops, revealing the formation of a mesoporous structure in NH2-UiO-66 after the hybridization of Bi2WO6. The combination of micropores and mesopores can be further confirmed using the pore size distributions (Fig. S3 and S4†). Photocatalysts with higher BET surface areas can be expected to produce more active sites for photocatalytic reaction and exhibit improved photocatalytic performance.
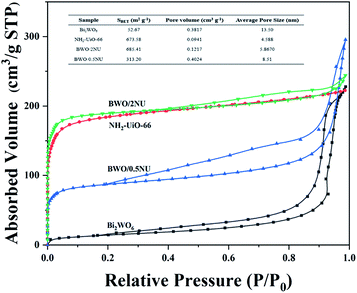 |
| Fig. 4 N2 adsorption–desorption isotherms and BET surface areas (inset table) of Bi2WO6, NH2-UiO-66, BWO/0.5NU, and BWO/2NU. | |
The XPS spectra of the as-prepared NH2-UiO-66, Bi2WO6, and BWO/2NU samples were obtained to further clarify the interfacial interaction between NH2-UiO-66 and Bi2WO6. The XPS survey spectrum (Fig. S5†) reveals that the BWO/2NU hybrid is composed of Zr, Bi, W, O, N, and C, which is consistent with the results of EDS. The characteristic peaks of the four elements with different valence states are ascribed to Zr 3d5/2 and Zr 3d3/2 (Fig. 5a), N 1s (Fig. 5b), Bi 4f7/2 and Bi 4f5/2 (Fig. 5c), and W 4f7/2 and W 4f5/2 (Fig. 5d). Compared with those of pure NH2-UiO-66, the high-resolution XPS spectra of Zr 3d5/2 and Zr 3d3/2 in BWO/2NU shift to lower binding energies by approximately 0.1 eV. It is noticeable that the shift in the binding energies of N 1s exhibits a similar result, indicating strong interfacial interaction between NH2-UiO-66 and Bi2WO6.15 Meanwhile, the characteristic peaks of Bi 4f7/2 and Bi 4f5/2, and of W 4f7/2 and W 4f5/2, attributed to the BWO/2NU hybrid, exhibit positive shifts of 0.2 eV and 0.3 eV, when compared with those observed for pure Bi2WO6. The shifts in the binding energy of a specific element suggest the electron migration from Bi2WO6 to NH2-UiO-66 with the formation of a built-in electric field at the interface in the direction from Bi2WO6 to NH2-UiO-66 and a strong interfacial interaction between them.
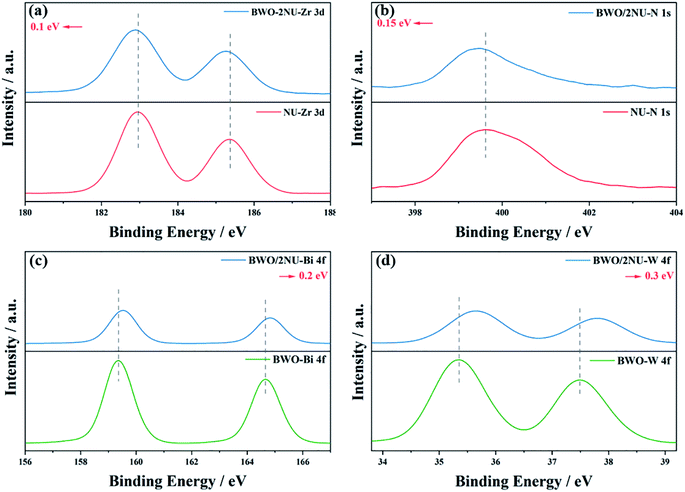 |
| Fig. 5 XPS spectra of (a) Zr 3d, (b) N 1s, (c) Bi 4f, and (d) W 4f. | |
3.2 Photocatalytic activities for NO removal
NO is chosen as the target pollutant to assess the photocatalytic performance of photocatalysts. An adsorption–desorption equilibrium between the test samples and NO was attained before the latter were irradiated using the Xe lamp. Fig. 6a shows that the blank test with no photocatalysts is not active for photocatalytic NO oxidation and the NO concentrations of the as-prepared samples decrease with increase in illumination time. The maximum NO removal ratio of pure NH2-UiO-66 is 63% but that of pristine Bi2WO6 is only 29%. Moreover, the time taken to achieve stable efficiency is longer in the case of NH2-UiO-66 and Bi2WO6 than in the case of the hybrids. As the NH2-UiO-66 content increases, the optimal activities of the as-prepared BWO/xNU (x = 0.5, 1, 2, 3, 5) hybrids assume an inverted V-shaped trend. When the NH2-UiO-66 content of BWO/xNU is 66.7%, the BWO/2NU exhibits an optimum NO removal efficiency of 79%, which is an increase of 272.41% and 118.44% over those achieved with pristine Bi2WO6 and NH2-UiO-66, respectively. It is interesting that the compounds, especially BWO/2NU, could achieve a state of balance rapidly, i.e., in only a few minutes. The photocatalytic NO oxidation of different catalysts displays small error variety (error bar < 5%) (Fig. S7†). And Table S1† shows other reported works in the field of photocatalytic NO removal. Among them, the BWO/2NU hybrid displays superior performance.
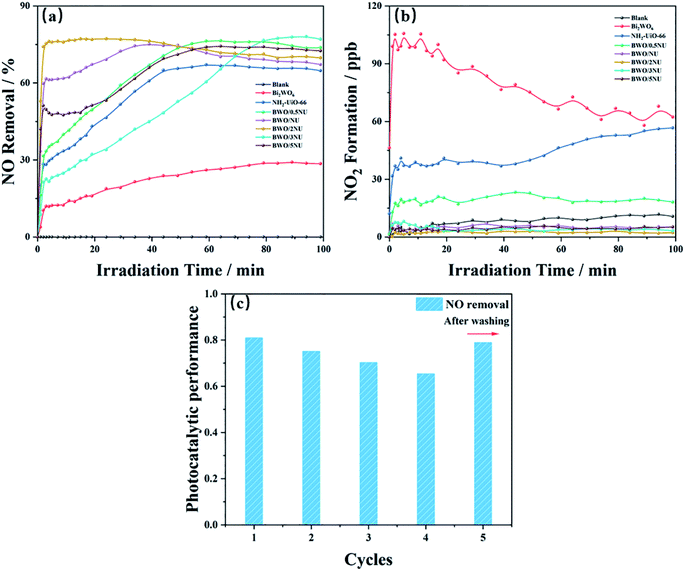 |
| Fig. 6 (a) NO removal efficiencies, and (b) quantities of NO2 formation using different as-prepared samples; and (c) consecutive cycles of photocatalytic reaction of NO on BWO/2NU. | |
NO3− is the desired product39 during the photocatalytic oxidation of NO, while NO2, as one of the reaction intermediates, is not desired. The formation of NO2 can be used to estimate the product selectivity and photooxidation ability of the materials.39 Therefore, the NO2 formation was detected online and the results are shown in Fig. 6b. It is noteworthy that the NO2 formation in the case of BWO/xNU hybrids decreases dramatically and is as low as 1.97 ppb when BWO/2NU is used. This quantity can reach 67.89 ppb and 56.22 ppb in the case of pristine Bi2WO6 and NH2-UiO-66, respectively, after stabilization. The above results indicate that the BWO/2NU hybrids are beneficial to the conversion of NO to NO3−/NO2− owing to their superior oxidation ability.
In addition, stability is usually used as an index to estimate the usefulness of photocatalysts in practical applications. Five-cycle experiments were performed to investigate the BWO/2NU's stability (Fig. 6c). After the first four runs, the efficiency of NO removal decreases slightly with increase in irradiation time. When the photocatalysts were washed with deionized water after four runs, their photocatalytic activity remained nearly unchanged. It is suggested in the literature40,41 that photocatalytic activity is weakened by the accumulation of HNO3/HNO2 on the surfaces of the catalysts, as the active sites of the as-prepared samples are likely covered. However, the powder XRD patterns (Fig. S6†) of the BWO/2NU hybrid used for photocatalysis shows no distinct differences in comparison with a fresh specimen, indicating no structural changes.
3.3 Enhanced mechanism of visible-light photocatalytic activities
To further investigate the enhanced photocatalytic activities, steady-state photoluminescence (PL) spectroscopy, time-resolved photoluminescence (TRPL) spectroscopy, photocurrent, and electrochemical impedance spectroscopy (EIS) analyses were performed. The PL emission can be utilized to investigate the recombination of photo-induced electron–hole pairs.42 The PL spectrum of pure NH2-UiO-66 (Fig. 7a) reveals an emission peak centered at 450 nm. And the PL emission peak of the BWO/2NU hybrid is significantly weaker than that of pure NH2-UiO-66, indicating efficient charge separation between Bi2WO6 and NH2-UiO-66. Fig. 7b presents the TRPL spectra of Bi2WO6, NH2-UiO-66, BWO/0.5NU, and BWO/2NU. Multiexponential decay is observed from the plot. The cubic exponential models were adopted to fit the decay spectra and obtain three radiative lifetimes with different percentages (inset of Fig. 7b). The three lifetimes (τ1, τ2, and τ3) of the BWO/2NU hybrid are all 0.452 ns, which is shorter than the corresponding lifetimes of pristine Bi2WO6 and NH2-UiO-66. Moreover, the BWO/2NU hybrid has a shorter average fluorescence lifetime (0.452 ns) than those of pure Bi2WO6 (0.962 ns) and NH2-UiO-66 (0.602 ns). The recombination of free excitons can lead to a longer lifetime. Therefore, the obvious quenching of all the lifetimes in the transients for BWO/2NU hybrid leads to a shorter decay lifetime, which indicates that interfacial electrons transfer faster at the BWO/2NU hybrid, otherwise charges will recombine and result in enhanced photoluminescence. The above result can be responsible for BWO/2NU's higher photocatalytic performance owing to the efficient repressed recombination of charges in Bi2WO6 and NH2-UiO-66. The spectra of the transient photocurrent response and electrochemical impedance spectroscopy were analyzed to investigate the separation efficiency of electron–hole pairs. Fig. 7c provides the transient photocurrent responses of Bi2WO6, NH2-UiO-66, BWO/0.5NU, and BWO/2NU under discontinuous visible light irradiation. When the light is switch on, the intensity of photocurrent response for pristine Bi2WO6 or NH2-UiO-66 is weak. After the incorporation of NH2-UiO-66 into Bi2WO6, both BWO/0.5NU and BWO/2NU hybrids show stronger photocurrent intensity than pristine Bi2WO6 or NH2-UiO-66. Generally, the transient photocurrent responses have positive correlations with the separation efficiency of electron–hole pairs, so the above result indicates more efficient separation of photo-induced charges and transfer over the BWO/2NU hybrid.43 As shown in Fig. 7d, BWO/2NU shows smaller diameter of the arc radius on the Nyquist plot compared with that of pristine Bi2WO6 or NH2-UiO-66, suggesting a considerably smaller charge transfer resistance between the interface of Bi2WO6 and NH2-UiO-66.44 That is to say the BWO/2NU hybrid has higher efficiency of photo-induced charge separation and migration.45 All the results reveal that the BWO/2NU hybrid has superior performance of the electron–hole separation and migration than the single component, leading to enhanced photocatalytic abilities.
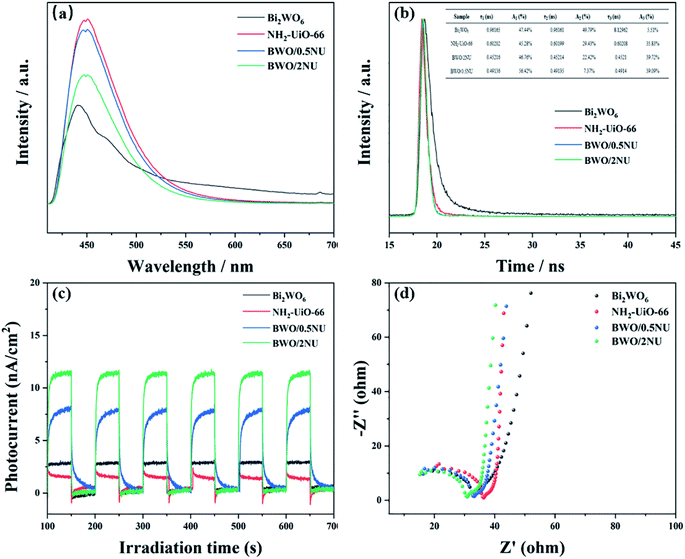 |
| Fig. 7 (a) Stable state PL, and (b) TRPL spectra, (c) photocurrent responses, and (d) EIS images of Bi2WO6, NH2-UiO-66, BWO/0.5NU and BWO/2NU. | |
Additional experiments were carried out to investigate the detailed band structures/positions of the photocatalysts. The UV-vis DRS analysis (Fig. 8) reveals that pristine Bi2WO6 holds an absorption edge of 440 nm, which is close to the reported values.46,47 NH2-UiO-66 exhibits strong absorption in the range 200–440 nm.26 When compared with those of pure Bi2WO6 and NH2-UiO-66, the absorption edges of the hybrids occur with a slight red shift and the BWO/2NU sample shows the strongest response in the visible region, indicating the production of more electron–hole pairs and thereby enhanced photocatalytic performance.26 The equation, αhν = A(hν − Eg)n/2,36,48 is used to calculate the band gaps of pristine Bi2WO6 and NH2-UiO-66, where α, h, ν, A, and Eg stand for the absorption coefficient, Planck's constant, optical frequency, a constant, and the band gap, i.e., highest occupied molecular orbital-lowest unoccupied molecular orbital (HOMO–LUMO) gap, respectively. N's values depend on the types of optical transitions in different semiconductors. Hence, the values obtained are n = 4 for Bi2WO6 (indirect semiconductor) and n = 1 for NH2-UiO-66 (direct semiconductor).36,49 From the plots of (αhν)2/n versus hν (Fig. S8†), the band gaps/HOMO–LUMO gaps of the as-prepared Bi2WO6 and NH2-UiO-66 samples are determined to 2.82 eV and 2.86 eV, respectively. Moreover, the HOMO–LUMO gap of the BWO/2NU hybrid is estimated to be 2.80 eV from the absorption edge in Fig. 8. The result suggests that the BWO/2NU hybrid is not a simple mixture of Bi2WO6 and NH2-UiO-66, but entails the formation of an interfacial interaction, which indicates the formation of a heterojunction structure.
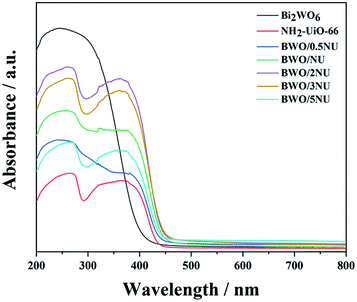 |
| Fig. 8 UV-vis DSR spectra of as-prepared samples. | |
It's demonstrated in Fig. 9 that the CBs of Bi2WO6, NH2-UiO-66, and BWO/2NU were determined using the Mott–Schottky method. The slopes of the Mott–Schottky curves are positive, indicating that both Bi2WO6 and NH2-UiO-66 are n-type semiconductors.26 The flat-band potentials of Bi2WO6 and NH2-UiO-66 are approximately −0.76 V vs. Ag/AgCl (i.e., −0.56 V vs. normal hydrogen electrode (NHE)) and −0.60 V vs. Ag/AgCl (i.e., −0.40 V vs. NHE), respectively. Generally, the flat band potential is about 0–0.2 V below the bottom of the CB in n-type semiconductors.50 Here, the voltage difference between the CB and flat band potential was set to 0.1 eV.50 Therefore, the CB positions of Bi2WO6 and NH2-UiO-66 can be calculated to be −0.66 V and −0.50 V (vs. NHE), respectively. By combining these results with the band gap values of the as-prepared photocatalysts derived from the UV-vis DRS analysis (Fig. 8), the VB positions of Bi2WO6 and NH2-UiO-66 are determined to be +2.16 V and +2.36 V (vs. NHE), respectively.
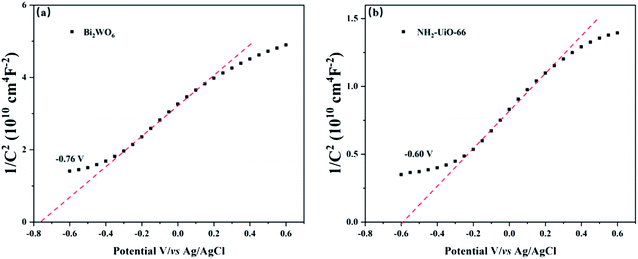 |
| Fig. 9 Mott–Schottky plots for (a) Bi2WO6, and (b) NH2-UiO-66. | |
To confirm and investigate the mechanism of the Z-scheme BWO/2NU system, the ESR spin-trap (with 5,5-dimethyl-1-pyrroline N-oxide (DMPO)) was employed. Fig. 10 shows that no characteristic peaks of ·OH or ·O2− are observed in pristine Bi2WO6, NH2-UiO-66, and the BWO/0.5NU, BWO/2NU, and BWO/5NU hybrids without irradiation. Under visible light irradiation (Fig. 10a), strong ESR signals corresponding to the DMPO-·O2− adduct are clearly observed for both pristine Bi2WO6 and the BWO/xNU hybrids, whereas no signal is observed in the case of pure NH2-UiO-66. Moreover, the signals of DMPO-·O2− attributed to the hybrids are generally much stronger than those in the case of pristine Bi2WO6. As depicted in Fig. 10b, the DMPO-·OH signals of the BWO/xNU hybrids are detected under visible light irradiation but not in the case of pure Bi2WO6 or NH2-UiO-66. The above results suggest that the BWO/xNU hybrids, with Bi2WO6 incorporated into the NH2-UiO-66 catalysts, can produce more active radicals, thereby enhancing photocatalytic activity.
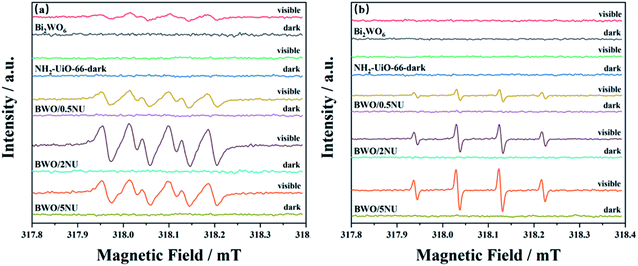 |
| Fig. 10 DMPO spin-trapping ESR spectra for (a) DMPO-·O2−, and (b) DMPO-·OH−. | |
Based on the UV-vis DRS analysis and Mott–Schottky plots, the VB/CB (HOMO/LUMO) positions of Bi2WO6 and NH2-UiO-66 are determined to be +2.16/−0.66 V and +2.36/−0.5 V (vs. NHE), respectively. The CB potential of Bi2WO6 (−0.66 V vs. NHE) is more negative than the standard potential of O2/·O2− (−0.33 V vs. NHE),51 and hence, the ·O2− generated by Bi2WO6 can be detected. In addition, the CB potential of NH2-UiO-66 (−0.5 V vs. NHE) is more negative than that of O2/·O2−, but no ·O2− signal is observed in the case of pure NH2-UiO-66, which is probably owing to the production of a few DMPO-·O2− adducts. Meanwhile, the HOMO energy of NH2-UiO-66 is higher than the potential energy of H2O/·OH (2.27 V vs. NHE),52 but ·OH was not generated for reasons similar to those mentioned above. Bi2WO6 has lower VB potential and hence, the production of ·OH is prohibited. Both ·O2− and ·OH signals are observed in the case of the BWO/xNU hybrids. Therefore, a direct Z-scheme photocatalytic system is proposed, which is displayed in Fig. 11.
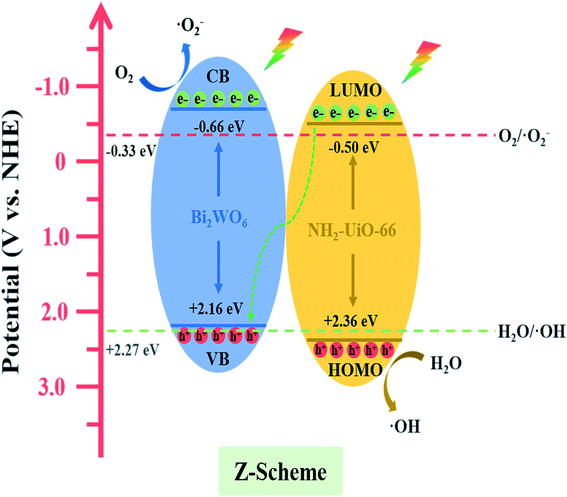 |
| Fig. 11 Schematic diagram of direct Z-scheme system for photocatalysis and charge migration pathway. | |
Moreover, the potential EF (Fermi level) of pristine Bi2WO6 is about −0.89 eV (vs. SCE),23 negative than that of pure NH2-UiO-66 about −0.58 eV (vs. SCE).53 When the Bi2WO6 and the NH2-UiO-66 get into contact, the electrons will migrate from the surface of Bi2WO6 to the surface of NH2-UiO-66 to achieve their Fermi level equilibrium.16 After Fermi level equilibrium is achieved, the region close to the interface of BWO/xNU is charged, which results in the formation of a built-in electric field in the direction from Bi2WO6 to NH2-UiO-66.
The photo-induced electrons from the CB of NH2-UiO-66 transfer to the interface and combine with the photo-induced holes on the VB of Bi2WO6. In addition, the formation of the built-in electric field pointing from Bi2WO6 to NH2-UiO-66 will accelerate the electron transfer from the LUMO of NH2-UiO-66 to the VB of Bi2WO6. This promotes the accumulation of electrons on the CB of Bi2WO6 and of holes on the VB of NH2-UiO-66, resulting in the preservation of the strong reducibility of electrons and strong oxidation ability of holes. The direct Z-scheme photocatalytic mechanism proposed is in excellent agreement with the results of ESR analysis.
4. Conclusion
In this study, a novel direct Z-scheme photocatalytic system composed of NH2-UiO-66 and Bi2WO6 for efficient NO oxidation under visible light irradiation was proposed. The incorporation of NH2-UiO-66 into Bi2WO6 can not only effectively improve its photocatalytic activity for NO oxidation, but also increase the selectivity for converting NO to NO3−/NO2−, truly diminishing the potential secondary pollution of NO2. The introduction of NH2-UiO-66 produces abundant active sites to promote the adsorption and photocatalytic reaction. Meanwhile, combining NH2-UiO-66 with Bi2WO6 can narrow the band gaps of the hybrids to some extent to enhance their light adsorption. The fabrication of a direct Z-scheme system improves the separation efficiency of electron–hole pairs. Simultaneously it preserves their strong redox abilities. This work provides a new idea for the construction of a direct Z-scheme photocatalytic system without cocatalysts for NO photocatalytic removal. We hope this work can provide some inspiration on the design and synthesis of more advanced photocatalysts for environmental protection.
Conflicts of interest
There are no conflicts to declare.
Acknowledgements
This work was supported by National Natural Science Foundation of China (NSFC-51578488), Zhejiang Provincial “151” Talents Program and the Program for Zhejiang Leading Team of S&T Innovation (Grant No. 2013TD07).
References
- Y. Feng, L. Ling, J. Nie, K. Han, X. Chen, Z. Bian, H. Li and Z. L. Wang, Self-Powered Electrostatic Filter with Enhanced Photocatalytic Degradation of Formaldehyde Based on Built-in Triboelectric Nanogenerators, ACS Nano, 2017, 11, 12411–12418 CrossRef CAS PubMed.
- F. Wang, Z. Xie, J. Liang, B. Fang, Y. Piao, M. Hao and Z. Wang, Tourmaline-Modified FeMnTiOx Catalysts for Improved Low-Temperature NH3-SCR Performance, Environ. Sci. Technol., 2019, 53, 6989–6996 CrossRef CAS PubMed.
- J. Angelo, L. Andrade, L. M. Madeira and A. Mendes, An overview of photocatalysis phenomena applied to NOx abatement, J. Environ. Manage., 2013, 129, 522–539 CrossRef CAS PubMed.
- F. Rezaei, A. A. Rownaghi, S. Monjezi, R. P. Lively and C. W. Jones, SOx/NOx Removal from Flue Gas Streams by Solid Adsorbents: A Review of Current Challenges and Future Directions, Energy Fuels, 2015, 29, 5467–5486 CrossRef CAS.
- S. Xiong, J. Weng, Y. Liao, B. Li, S. Zou, Y. Geng, X. Xiao, N. Huang and S. Yang, Alkali Metal Deactivation on the Low Temperature Selective Catalytic Reduction of NOx with NH3 over MnOx-CeO2: A Mechanism Study, J. Phys. Chem. C, 2016, 120, 15299–15309 CrossRef CAS.
- A. Wang, Y. Guo, F. Gao and C. H. F. Peden, Ambient-temperature NO oxidation over amorphous CrOx-ZrO2 mixed oxide catalysts: Significant promoting effect of ZrO2, Appl. Catal., B, 2017, 202, 706–714 CrossRef CAS.
- G. Jiang, X. Li, M. Lan, T. Shen, X. Lv, F. Dong and S. Zhang, Monodisperse bismuth nanoparticles decorated graphitic carbon nitride: Enhanced visible-light-response photocatalytic NO removal and reaction pathway, Appl. Catal., B, 2017, 205, 532–540 CrossRef CAS.
- Y. Huang, Y. Liang, Y. Rao, D. Zhu, J. J. Cao, Z. Shen, W. Ho and S. C. Lee, Environment-Friendly Carbon Quantum Dots/ZnFe2O4 Photocatalysts: Characterization, Biocompatibility, and Mechanisms for NO Removal, Environ. Sci. Technol., 2017, 51, 2924–2933 CrossRef CAS PubMed.
- J. Ma, H. He and F. Liu, Effect of Fe on the photocatalytic removal of NO over visible light responsive Fe/TiO2 catalysts, Appl. Catal., B, 2015, 179, 21–28 CrossRef CAS.
- Z.-F. Huang, J. Song, X. Wang, L. Pan, K. Li, X. Zhang, L. Wang and J.-J. Zou, Switching charge transfer of C3N4/W18O49 from type-II to Z-scheme by interfacial band bending for highly efficient photocatalytic hydrogen evolution, Nano Energy, 2017, 40, 308–316 CrossRef CAS.
- X. Chen, H. Zhang, D. Zhang, Y. Miao and G. Li, Controllable synthesis of mesoporous multi-shelled ZnO microspheres as efficient photocatalysts for NO oxidation, Appl. Surf. Sci., 2018, 435, 468–475 CrossRef CAS.
- Y. Luan, L. Jing, Y. Xie, X. Sun, Y. Feng and H. Fu, Exceptional Photocatalytic Activity of 001-Facet-Exposed TiO2 Mainly Depending on Enhanced Adsorbed Oxygen by Residual Hydrogen Fluoride, ACS Catal., 2013, 3, 1378–1385 CrossRef CAS.
- J. Cao, J. Zhang, X. a. Dong, H. Fu, X. Zhang, X. Lv, Y. Li and G. Jiang, Defective borate-decorated polymer carbon nitride: Enhanced photocatalytic NO removal, synergy effect and reaction pathway, Appl. Catal., B, 2019, 249, 266–274 CrossRef CAS.
- J. Zhang, Y. Hu, X. Jiang, S. Chen, S. Meng and X. Fu, Design of a direct Z-scheme photocatalyst: preparation and characterization of Bi2O3/g-C3N4 with high visible light activity, J. Hazard. Mater., 2014, 280, 713–722 CrossRef CAS PubMed.
- Z. Jiang, W. Wan, H. Li, S. Yuan, H. Zhao and P. K. Wong, A Hierarchical Z-Scheme alpha-Fe2O3/g-C3N4 Hybrid for Enhanced Photocatalytic CO2 Reduction, Adv. Mater., 2018, 30, 1706108 CrossRef PubMed.
- J. Low, B. Dai, T. Tong, C. Jiang and J. Yu, In Situ Irradiated X-Ray Photoelectron Spectroscopy Investigation on a Direct Z-Scheme TiO2/CdS Composite Film Photocatalyst, Adv. Mater., 2019, 31, 1802981 CrossRef PubMed.
- T. Di, B. Zhu, B. Cheng, J. Yu and J. Xu, A direct Z-scheme g-C3N4/SnS2 photocatalyst with superior visible-light CO2 reduction performance, J. Catal., 2017, 352, 532–541 CrossRef CAS.
- W. Yu, D. Xu and T. Peng, Enhanced photocatalytic activity of g-C3N4 for selective CO2 reduction to CH3OH via facile coupling of ZnO: a direct Z-scheme mechanism, J. Mater. Chem. A, 2015, 3, 19936–19947 RSC.
- J. Low, C. Jiang, B. Cheng, S. Wageh, A. A. Al-Ghamdi and J. Yu, A Review of Direct Z-Scheme Photocatalysts, Small Methods, 2017, 1, 1700080 CrossRef.
- M. Zhu, Z. Sun, M. Fujitsuka and T. Majima, Z-Scheme Photocatalytic Water Splitting on a 2D Heterostructure of Black Phosphorus/Bismuth Vanadate Using Visible Light, Angew. Chem., Int. Ed. Engl., 2018, 57, 2160–2164 CrossRef CAS PubMed.
- J. Yu, S. Wang, J. Low and W. Xiao, Enhanced photocatalytic performance of direct Z-scheme g-C3N4-TiO2 photocatalysts for the decomposition of formaldehyde in air, Phys. Chem. Chem. Phys., 2013, 15, 16883–16890 RSC.
- H. Zhou, Z. Wen, J. Liu, J. Ke, X. Duan and S. Wang, Z-scheme plasmonic Ag decorated WO3/Bi2WO6 hybrids for enhanced photocatalytic abatement of chlorinated-VOCs under solar light irradiation, Appl. Catal., B, 2019, 242, 76–84 CrossRef CAS.
- S. Luo, J. Ke, M. Yuan, Q. Zhang, P. Xie, L. Deng and S. Wang, CuInS2 quantum dots embedded in Bi2WO6 nanoflowers for enhanced visible light photocatalytic removal of contaminants, Appl. Catal., B, 2018, 221, 215–222 CrossRef CAS.
- J. Zhang, X. Yuan, L. Jiang, Z. Wu, X. Chen, H. Wang, H. Wang and G. Zeng, Highly efficient photocatalysis toward tetracycline of nitrogen doped carbon quantum dots sensitized bismuth tungstate based on interfacial charge transfer, J. Colloid Interface Sci., 2018, 511, 296–306 CrossRef CAS PubMed.
- J. Hu, D. Chen, Z. Mo, N. Li, Q. Xu, H. Li, J. He, H. Xu and J. Lu, Z-Scheme 2D/2D Heterojunction of Black Phosphorus/Monolayer Bi2WO6 Nanosheets with Enhanced Photocatalytic Activities, Angew. Chem., Int. Ed. Engl., 2019, 58, 2073–2077 CrossRef CAS PubMed.
- J. Meng, Q. Chen, J. Lu and H. Liu, Z-Scheme Photocatalytic CO2 Reduction on a Heterostructure of Oxygen-Defective ZnO/Reduced Graphene Oxide/UiO-66-NH2 under Visible Light, ACS Appl. Mater. Interfaces, 2019, 11, 550–562 CrossRef CAS PubMed.
- L. Shen, S. Liang, W. Wu, R. Liang and L. Wu, CdS-decorated UiO–66(NH2) nanocomposites fabricated by a facile photodeposition process: an efficient and stable visible-light-driven photocatalyst for selective oxidation of alcohols, J. Mater. Chem. A, 2013, 1, 11473 RSC.
- Z. Sha, J. Sun, H. S. On Chan, S. Jaenicke and J. Wu, Bismuth tungstate incorporated zirconium metal–organic framework composite with enhanced visible-light photocatalytic performance, RSC Adv., 2014, 4, 64977–64984 RSC.
- Z. Sha and J. Wu, Enhanced visible-light photocatalytic performance of BiOBr/UiO-66(Zr) composite for dye degradation with the assistance of UiO-66, RSC Adv., 2015, 5, 39592–39600 RSC.
- R. Wang, L. Gu, J. Zhou, X. Liu, F. Teng, C. Li, Y. Shen and Y. Yuan, Quasi-Polymeric Metal-Organic Framework UiO-66/g-C3N4 Heterojunctions for Enhanced Photocatalytic Hydrogen Evolution under Visible Light Irradiation, Adv. Mater. Interfaces, 2015, 2, 1500037 CrossRef.
- C. Gomes Silva, I. Luz, F. X. Llabres i Xamena, A. Corma and H. Garcia, Water stable Zr-benzenedicarboxylate metal-organic frameworks as photocatalysts for hydrogen generation, Chem.–Eur. J., 2010, 16, 11133–11138 CrossRef.
- X. Wang, W. Chen, L. Zhang, T. Yao, W. Liu, Y. Lin, H. Ju, J. Dong, L. Zheng, W. Yan, X. Zheng, Z. Li, X. Wang, J. Yang, D. He, Y. Wang, Z. Deng, Y. Wu and Y. Li, Uncoordinated Amine Groups of Metal-Organic Frameworks to Anchor Single Ru Sites as Chemoselective Catalysts toward the Hydrogenation of Quinoline, J. Am. Chem. Soc., 2017, 139, 9419–9422 CrossRef CAS.
- S.-T. Gao, W. Liu, C. Feng, N.-Z. Shang and C. Wang, A Ag–Pd alloy supported on an amine-functionalized UiO-66 as an efficient synergetic catalyst for the dehydrogenation of formic acid at room temperature, Catal. Sci. Technol., 2016, 6, 869–874 RSC.
- H. Huang, K. Liu, K. Chen, Y. Zhang, Y. Zhang and S. Wang, Ce and F Comodification on the Crystal Structure and Enhanced Photocatalytic Activity of Bi2WO6 Photocatalyst under Visible Light Irradiation, J. Phys. Chem. C, 2014, 118, 14379–14387 CrossRef CAS.
- H. Cheng, J. Hou, O. Takeda, X.-M. Guo and H. Zhu, A unique Z-scheme 2D/2D nanosheet heterojunction design to harness charge transfer for photocatalysis, J. Mater. Chem. A, 2015, 3, 11006–11013 RSC.
- Y. Su, Z. Zhang, H. Liu and Y. Wang, Cd0.2Zn0.8S@UiO-66-NH2 nanocomposites as efficient and stable visible-light-driven photocatalyst for H2 evolution and CO2 reduction, Appl. Catal., B, 2017, 200, 448–457 CrossRef CAS.
- Z. Hu, Y. Peng, Z. Kang, Y. Qian and D. Zhao, A Modulated Hydrothermal (MHT) Approach for the Facile Synthesis of UiO-66-Type MOFs, Inorg. Chem., 2015, 54, 4862–4868 CrossRef CAS PubMed.
- Y. Han, M. Liu, K. Li, Y. Zuo, Y. Wei, S. Xu, G. Zhang, C. Song, Z. Zhang and X. Guo, Facile synthesis of morphology and size-controlled zirconium metal–organic framework UiO-66: the role of hydrofluoric acid in crystallization, CrystEngComm, 2015, 17, 6434–6440 RSC.
- Y. Zhou, X. Zhang, Q. Zhang, F. Dong, F. Wang and Z. Xiong, Role of graphene on the band structure and interfacial interaction of Bi2WO6/graphene composites with enhanced photocatalytic oxidation of NO, J. Mater. Chem. A, 2014, 2, 16623–16631 RSC.
- G. Li, D. Zhang and J. Yu, A new visible-light photocatalyst: CdS quantum dots embedded mesoporous TiO2, Environ. Sci. Technol., 2009, 43, 7079–7085 CrossRef CAS PubMed.
- C. H. Ao and S. C. Lee, Enhancement effect of TiO2 immobilized on activated carbon filter for the photodegradation of pollutants at typical indoor air level, Appl. Catal., B, 2003, 44, 191–205 CrossRef CAS.
- W. Zhong, S. Shen, S. Feng, Z. Lin, Z. Wang and B. Fang, Facile fabrication of alveolate Cu2-xSe microsheets as a new visible-light photocatalyst for discoloration of Rhodamine B, CrystEngComm, 2018, 20, 7851–7856 RSC.
- W. Zhong, W. Tu, S. Feng and A. Xu, Photocatalytic H2 evolution on CdS nanoparticles by loading FeSe nanorods as co-catalyst under visible light irradiation, J. Alloys Compd., 2019, 772, 669–674 CrossRef CAS.
- W. Zhong, Z. Lin, S. Feng, D. Wang, S. Shen, Q. Zhang, L. Gu, Z. Wang and B. Fang, Improved oxygen evolution activity of IrO2 by in situ engineering of an ultra-small Ir sphere shell utilizing a pulsed laser, Nanoscale, 2019, 11, 4407–4413 RSC.
- W. Zhong, S. Shen, M. He, D. Wang, Z. Wang, Z. Lin, W. Tu and J. Yu, The pulsed laser-induced Schottky junction via in situ forming Cd clusters on CdS surfaces toward efficient visible light-driven photocatalytic hydrogen evolution, Appl. Catal., B, 2019, 258, 117967 CrossRef CAS.
- Z. Zhang, W. Wang, L. Wang and S. Sun, Enhancement of visible-light photocatalysis by coupling with narrow-band-gap semiconductor: a case study on Bi2S3/Bi2WO6, ACS Appl. Mater. Interfaces, 2012, 4, 593–597 CrossRef CAS PubMed.
- Y. Tian, B. Chang, J. Lu, J. Fu, F. Xi and X. Dong, Hydrothermal synthesis of graphitic carbon nitride-Bi2WO6 heterojunctions with enhanced visible light photocatalytic activities, ACS Appl. Mater. Interfaces, 2013, 5, 7079–7085 CrossRef CAS PubMed.
- L. Zhang, W. Wang, Z. Chen, L. Zhou, H. Xu and W. Zhu, Fabrication of flower-like Bi2WO6 superstructures as high performance visible-light driven photocatalysts, J. Mater. Chem., 2007, 17, 2526 RSC.
- Z. Wang, T. Hu, K. Dai, J. Zhang and C. Liang, Construction of Z-scheme Ag3PO4/Bi2WO6 composite with excellent visible-light photodegradation activity for removal of organic contaminants, Chin. J. Catal., 2017, 38, 2021–2029 CrossRef CAS.
- Y. Wang, Y. Zeng, X. Chen, Q. Wang, L. Guo, S. Zhang and Q. Zhong, One-step hydrothermal synthesis of a novel 3D BiFeWOx/Bi2WO6 composite with superior visible-light photocatalytic activity, Green Chem., 2018, 20, 3014–3023 RSC.
- W. He, Y. Sun, G. Jiang, H. Huang, X. Zhang and F. Dong, Activation of amorphous Bi2WO6 with synchronous Bi metal and Bi2O3 coupling: Photocatalysis mechanism and reaction pathway, Appl. Catal., B, 2018, 232, 340–347 CrossRef CAS.
- H. Huang, Y. He, X. Li, M. Li, C. Zeng, F. Dong, X. Du, T. Zhang and Y. Zhang, Bi2O2(OH)(NO3) as a desirable [Bi2O2]2+ layered photocatalyst: strong intrinsic polarity, rational band structure and {001} active facets co-beneficial for robust photooxidation capability, J. Mater. Chem. A, 2015, 3, 24547–24556 RSC.
- X. Wang, X. Zhao, D. Zhang, G. Li and H. Li, Microwave irradiation induced UIO-66-NH2 anchored on graphene with high activity for photocatalytic reduction of CO2, Appl. Catal., B, 2018, 228, 47–53 CrossRef CAS.
Footnote |
† Electronic supplementary information (ESI) available. See DOI: 10.1039/c9ra09270f |
|
This journal is © The Royal Society of Chemistry 2020 |