DOI:
10.1039/C9QM00792J
(Research Article)
Mater. Chem. Front., 2020,
4, 1471-1482
AIE-active Schiff base compounds as fluorescent probes for the highly sensitive and selective detection of Fe3+ ions†
Received
2nd January 2020
, Accepted 1st March 2020
First published on 3rd March 2020
Introduction
Ferric ion (Fe3+) plays an important role at the cellular level in several biochemical processes; both its deficiency and its surplus can result in several diseases, while iron storage and transport regulate metabolic processes.1 A higher amount of Fe3+ in the body has been associated with increased tendencies of certain cancers, abnormal functioning of organs such as the heart, pancreas and liver, and diseases such as anemia, Alzheimer's disease, epilepsy and hemochromatosis.2 The use of iron in day-to-day life and its crucial role in biological processes demands the development of techniques for monitoring Fe3+ in the environmental and biological fields. Therefore, the development of a sensor for the detection of Fe3+ with high selectivity and sensitivity is vastly desirable.
Several efforts have been made to detect Fe3+ ions using various analytical techniques, including electrochemical methods, inductively coupled plasma atomic emission spectroscopy and atomic absorption spectrometry.3 These methods require sophisticated instrumentation, tedious sample preparation and trained manpower. Amongst these techniques, the colorimetric4 and fluorimetric5 methods are gaining increasing attention for the detection of significant metal ions, including Fe3+, due to their target ion-induced naked eye colour observations and changes in fluorescence. Fluorescence sensors offer inherent advantages in terms of response time, selectivity and sensitivity; thus, they are very attractive.6 Fluorescent molecular sensors are showing a wide range of development in various fields, including metal ion sensing, DNA sequencing and DNA fragmentation analysis, as well as in a variety of immunoassays.7
Based on the pioneering work on the aggregation-induced emission (AIE) effect,8 designing new molecules with AIE properties has recently garnered immense attention.9 AIE-active molecules exhibit either weak or no emission in solution, whereas their fluorescence intensity dramatically increases in the aggregated state.10 In the AIE state, the intramolecular rotations are restricted through a variety of molecular interactions, including hydrogen bonding, π–π interactions and steric effects;11 this results in the elimination of nonradiative transitions and the promotion of radiative transitions, affording strong emission. Owing to their interesting photophysical properties, these AIE-active molecules have found widespread applications in biosensors and optoelectronic devices as well as in the sensing of cations and anions.12 A few reports are available in the literature on the fluorescent sensing of metal ions, including Fe3+ ions.13 However, there is still a need to design AIE-active fluorescent probes for the competent detection of Fe3+ because of the enormous importance of these ions in the arenas of biology, chemistry and the environment.
Schiff base compounds are regarded as pertinent candidates for fluorescent sensing because they exhibit higher fluorescence in organic solutions and AIE medium than in the solid state.14 Among the different probes explored, salicylaldehyde azomethines have exhibited exclusive properties. They undergo rapid C
N isomerization in dilute solutions and are often non-fluorescent. This non-radiative process can be eliminated by the restriction of C
N isomerization through hydrogen bonding in AIE medium, resulting in enhanced emission.15 Taking into account this rationale, we have synthesized luminescent AIE-active Schiff base compounds, viz. 4-(tert-butyl)-2-((2-hydroxybenzylidene)amino)phenol (L1) and 4-(tert-butyl)-2-((2-hydroxy-5-methyl-benzylidene)amino)phenol (L2), and investigated their photophysical properties. The efficacy of L1 and L2 towards metal ion sensing has been tested in THF and THF–H2O media, and their fluorescence response has been examined through the photoinduced electron transfer (PET) mechanism. These ligands suffered from poor selectivity in THF, whereas both L1 and L2 demonstrated highly sensitive and selective response towards the colorimetric and fluorimetric detection of Fe3+ ion.
Experimental
Materials
Salicylaldehyde, 5-methylbenzaldehyde, 2-amino-4-tert-butylphenol and tetrabutylammonium perchlorate (TBAP) were purchased from Sigma-Aldrich, India, and the metal salts were obtained from Merck Chemicals, India. All the chemicals and solvents (AR grade) were utilized as received without any further purification. HPLC grade solvents and Milli-Q water were used for the preparation of stock solutions and dilutions during the photophysical investigations.
Instrumentation and methods
Nuclear magnetic resonance (NMR) spectra were recorded on a Bruker Avance 400 MHz spectrometer (using TMS as the internal reference). Mass spectra were obtained using PerkinElmer (Clarus 680 GC and Clarus 600 MS) spectrometers. Infrared spectra (IR) were recorded using a Shimadzu FT-IR spectrometer (IR Affinity-ICE with resolution IV) within the range of 4000–400 cm−1. UV-vis absorption spectral analysis was performed using a Jasco spectrophotometer (UV-VIS-NIR-V-670) within the range of 200–800 nm, and fluorescence emission spectra were recorded using a Hitachi (F-7000FL) fluorescence spectrophotometer within the range of 200–800 nm. Lifetime measurements were performed using time correlation single photon counting (TCSPC). Electrochemical measurements were carried out using an electrochemical workstation (CHI 760E; CH instruments, USA) using a conventional three-electrode setup. Glassy carbon electrode, Pt wire and Ag/AgCl (3 M KCl) were used as working, counter and reference electrodes, respectively. Stock solutions of L1 and L2 in THF with fixed concentrations of 1 × 10−3 M were prepared, and the solutions of metal salts were prepared in Milli-Q water. The test solutions for metal ion detection were prepared by diluting an appropriate aliquot of each metal ion and ligand stock solution. Solutions of L1 and L2 at 1 × 10−5 M concentrations in THF with different water fractions were prepared to analyze the AIE characteristics.
Synthesis of 4-(tert-butyl)-2-((2-hydroxybenzylidene)amino)phenol (L1).
The ligand L1 was synthesized using a procedure reported earlier16 with slight modification, as shown in Fig. 1. 2-Hydroxybenzaldehyde (0.24 g, 2 mmol) was dissolved in 5 mL of methanolic solution and stirred, to which 2-amino-4-tert-butylphenol (0.33 g, 2 mmol) in methanol was added dropwise at room temperature. The reaction mixture was further refluxed at 60 °C for 24 h. After cooling to room temperature, the excess solvent was removed and the ligand L1 was obtained as a dark orange solid. Yield: 85%. 1H NMR (400 MHz, DMSO-d6): δ 1.29 (s, 9H), 6.88–6.96 (p, 3H), 7.13–7.16 (q, 1H), 7.34–7.38 (t, 2H), 7.40–7.64 (t, 1H), 9.00 (s, 1H), 9.53 (s, 1H), 13.91 (s, 1H) ppm. 13C NMR (100 MHz, DMSO-d6): δ 31.9, 34.44, 114.47, 116.46, 116.92, 117.14, 119.11, 120.01, 125.21, 132.79, 133.17, 134.96, 142.90, 149.15, 161.29, 162.01 ppm. IR (ATR) (ν cm−1): 2955 (–OH), 1613 (C
N), 1476 (C
C), 1378 (C–N). GC-MS: m/z calculated for [M+] [C17H19NO2]+ as 269.2416; found: 269.2438.
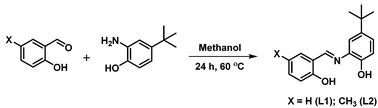 |
| Fig. 1 Synthesis of the AIE-active ligands L1 and L2. | |
Synthesis of 4-(tert-butyl)-2-((2-hydroxy-5-methylbenzylidene)amino)phenol (L2).
The synthesis of L2 was carried out using a similar procedure to that followed for L1 by replacing 2-hydroxybenzaldehyde with 2-hydroxy-5-methylbenzaldehyde (0.136 g, 1 mmol) (Fig. 1). The product was obtained (yield: 90%) as a red-brown solid. 1H NMR (400 MHz, DMSO-d6): δ 1.26 (s, 9H), 2.25 (s, 3H), 6.82 (d, 2H), 7.1 (d, 1H), 7.28 (d, 1H), 7.41 (d, 1H), 8.91 (s, 1H), 9.46 (s, 1H), 13.50 (s, 1H) ppm. 13C NMR (100 MHz, DMSO-d6): δ 20.41, 31.82, 34.42, 46.45, 119.69, 125.08, 127.63, 132.63, 133.91, 134.85, 142.50, 149.10, 158.97, 162.04 ppm. IR (ATR) (ν cm−1): 1600 (C
N), 1571 (C-C), 1486 (C
C), 1273 (C–N), 2927 (–OH). GC-MS: m/z calculated for [M+] [C18H21NO2]+ as 283.2572; found: 283.2228.
Results and discussion
The synthesized ligands, L1 and L2, were characterized using 1H NMR, 13C NMR, GC-MS and FT-IR spectra (Supplementary S1–S8, ESI†), and the spectral data were in accordance with their structures.
Photophysical properties and sensing behaviour in organic solvent
The photophysical properties and sensing behaviour of the ligands L1 and L2 were investigated using UV absorption and fluorescence spectroscopy. First, the UV-vis spectra of the ligands L1 and L2 (1 × 10−5 M) were recorded in THF, and the spectra are shown in Fig. S9 (ESI†). The molar absorptivity values were calculated for both the ligands, and the results are presented in Table S1 (ESI†). L1 exhibited two absorbance peaks at 254 and 355 nm with molar absorptivities of 3.36 × 104 M−1 cm−1 and 2.39 × 104 M−1 cm−1, corresponding to the π–π* and n–π* transitions, respectively. Similarly, L2 exhibited two peaks at 256 and 356 nm with molar absorptivities of 2.97 × 104 M−1 cm−1 and 1.99 × 104 M−1 cm−1. Also, the fluorescence emission spectra of L1 and L2 were measured in THF (1 × 10−5 M), and the emission peaks were observed at 317 and 320 nm for L1 and L2, respectively. Both the ligands exhibited excellent absorbance and emission characteristics; based on this, we decided to utilize these ligands towards colorimetric and fluorescent sensing. Because the ligands displayed better absorbance and fluorescence in THF medium (among the different solvents tested), THF was chosen as the solvent for further investigation.
The compounds L1 and L2 were designed with coordinating sites, such as imine nitrogen (C
N) and phenolic (–OH) groups, which can effectively bind with metal ions and thus can be employed for metal ion detection. Initially, the colorimetric response of the ligands L1 and L2 towards various metal ions was tested by adding 10 equivalents of metal ions to 1 × 10−5 M of the ligand in THF. The interactions of different metal ions, such as Al3+, Na+, Fe3+, Fe2+, Zn2+, Cu2+, Mn2+, Co2+, K+, Cd2+, Li+ and Hg2+, with the ligands were tested in THF by naked eye observation under a UV lamp (inset: Fig. 2A–D). Ligands L1 and L2 were responsive towards Fe3+, Cu2+ and Co2+ ions and slightly responsive to Fe2+ ions. The solutions turned yellow upon the addition of Fe3+ and pale yellow upon the addition of Fe2+. Meanwhile, the solutions changed from colorless to green in the presence of Cu2+ and Co2+. Thus, the ligands L1 and L2 have demonstrated their potential to be developed as naked eye sensors.
 |
| Fig. 2 Absorption spectra of 1 × 10−5 M (A) L1 and (B) L2 in THF in the presence of 10 equivalents of various metal ions. Insets: Photographs of L1 and L2 with different metal ions in THF under a UV lamp at 254 nm. Emission spectra of 1 × 10−5 M (C) L1 and (D) L2 in THF in the presence of different metal ions. Insets: Photographs of L1 and L2 with various metals under a UV lamp at 365 nm. | |
In the next step, the interactions between the ligands and metal ions were probed by observing the changes in the absorbance and fluorescence intensities. Among the different metals tested with L1 and L2 in THF, Co2+, Fe2+, Cu2+ and Fe3+ ions showed noticeable absorbance responses at 254, 250, 300 and 650 nm, respectively (Fig. 2A and B). In the fluorescence spectra (Fig. 2C and D), Fe3+ ions displayed prominent responses at 317 and 320 nm for L1 and L2, respectively, upon excitation at 255 nm, whereas Co2+, Fe2+ and Cu2+ also showed reasonable responses. This clearly demonstrates that the selectivities of the probes L1 and L2 towards the detection of Fe3+ in THF medium are unsatisfactory, and we further intended to enhance the selectivity by suitably tuning the ligand–solvent system.
Investigation of AIE characteristics
According to literature reports, interference from other closely related transition metals can be overcome by using an AIE medium.17
The ligands L1 and L2 are insoluble in water and highly soluble in organic solvents. The AIE behaviour of L1 and L2 were investigated in a solvent-non-solvent system (THF–H2O) using different water fractions (fw 0 to 99.5%) (Fig. 3A and B). The compounds L1 and L2 (1 × 10−5 M) showed weak fluorescence emissions in THF; meanwhile, the ligands were highly emissive in a THF–H2O mixture. The absorption spectra of ligands L1 and L2 were recorded in different water fractions from 10% to 99.5% (Fig. S10A and B, ESI†). The results revealed that the absorbance intensity gradually increased to a maximum at 90% and 60% water fractions for L1 and L2, respectively, in the UV region without any considerable change in its wavelength. Upon increasing fw from 10% to 99.5%, the fluorescence intensity (with an excitation wavelength of 255 nm) gradually increased and reached a maximum at 90% water fraction for L1 at 317 nm, and an emission maximum for L2 was attained with 60% at 320 nm. The lower fluorescence intensities of L1 and L2 in THF medium is due to intramolecular C
N isomerization. With increasing water content, the C
N isomerization is gradually arrested through intramolecular hydrogen bonding. The elimination of nonradiative decay of the fluorescence during the formation of these aggregates results in the enhanced emission. When the fw was increased beyond 90% for L1 and 60% for L2, the nanoaggregates of the salicylaldehyde azomethines underwent fast agglomeration and formed turbid solutions. The blue shift observed with this higher fw may be due to scattering by the agglomerated particles in the turbid solution. With further increase in fw, the agglomeration would increase and the aggregates would precipitate out, resulting in a drastic decrease in the fluorescence intensity. The quantum yields (Φ) were calculated for both L1 and L2 (1 × 10−5 M) in THF–water mixtures with different ratios using anthracene in methanol (Φ = 0.27) as a standard ref. 18:
where
Ir and
Is are the fluorescence intensities,
Ar and
As are the maximum absorbances, and
ns2 and
nr2 are the refractive indices of the sample and reference, respectively. As shown in Table S2 (ESI
†), the quantum yield values increased from 0.046 to 0.174 when the water fraction was increased from 0% to 90% for
L1 and decreased to 0.149 with a 99.5% water fraction. Similarly, the
Φ values for
L2 increased from 0.017 to 0.136 upon increasing the water fraction from 0% to 60% and decreased to 0.035 with a 99.5% water fraction. The variation in the quantum yield values with different ratios of the THF–water mixture followed the same trend as the fluorescence intensity, demonstrating the AIE behaviour of
L1 and
L2 with 10%
![[thin space (1/6-em)]](https://www.rsc.org/images/entities/char_2009.gif)
:
![[thin space (1/6-em)]](https://www.rsc.org/images/entities/char_2009.gif)
90% and 40%
![[thin space (1/6-em)]](https://www.rsc.org/images/entities/char_2009.gif)
:
![[thin space (1/6-em)]](https://www.rsc.org/images/entities/char_2009.gif)
60% THF–water mixtures. Furthermore, we examined the morphologies of these nanoaggregates by recording SEM images of
L1 and
L2 in AIE medium (THF–H
2O). As observed in
Fig. 3C and D, both ligands in THF–H
2O (10%
![[thin space (1/6-em)]](https://www.rsc.org/images/entities/char_2009.gif)
:
![[thin space (1/6-em)]](https://www.rsc.org/images/entities/char_2009.gif)
90% and 40%
![[thin space (1/6-em)]](https://www.rsc.org/images/entities/char_2009.gif)
:
![[thin space (1/6-em)]](https://www.rsc.org/images/entities/char_2009.gif)
60%) mixtures showed crystalline morphologies and formation of nanoaggregates, which resulted in the enhanced emissions.
19 Thus, it is clear that both
L1 and
L2 exhibit remarkable aggregation properties; therefore, they are suitable to be explored as AIE-active fluorescent probes for the selective detection of Fe
3+ ion.
 |
| Fig. 3 Fluorescence spectra of 1 × 10−5 M (A) L1 and (B) L2 in THF–H2O with various water fractions. SEM images of (C) L1 (10% : 90%, THF : H2O) and (D) L2 (40% : 60%, THF : H2O). | |
Selective binding of Fe3+ in AIE medium
Achieving good selectivity towards Fe3+ ions over dissimilar concomitant ions is essential to evaluate the competence of a sensor for practical applications. The abilities of the ligands L1 and L2 to selectively bind Fe3+ were evaluated by monitoring the variation in fluorescence intensity with continual addition of different metal ions. Experiments were performed at optimized conditions of THF–H2O mixtures of 10
:
90 and 40
:
60 volume % in the presence of 1 × 10−5 M concentrations of ligands L1 and L2, respectively. The absorbance (Fig. 4A and B) and fluorescence (Fig. 4C and D) spectral investigations indicate that L1 and L2 demonstrate very good selectivity towards Fe3+ ions among various metal ions, such as Al3+, Fe2+, Na+, Mn2+, Zn2+, Cd2+, Cu2+, Co2+, Hg2+, Li+, and K+, in THF–H2O. No significant change in the absorption and emission spectra was noted with either ligand, even upon addition of 10 equivalents of various metal ions, except Fe3+. Slight or negligible interference was observed with Fe2+ ions for both the ligands. The pictorial representation of the response of ligands L1 and L2 towards different metal ions under UV light (inset: Fig. 4A and B) illustrate the selectivity of the ligand towards Fe3+ ions. Further, the results shown in the inset to Fig. 4C and D indicate that both compounds can be utilized for the selective detection of Fe3+ ions with maximum turn-off fluorescence intensities.
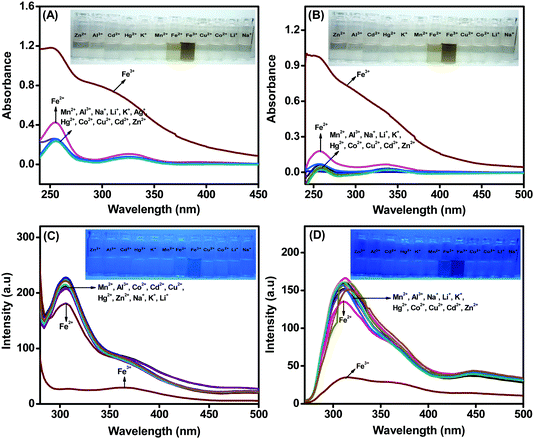 |
| Fig. 4 Absorption spectra of (A) L1 and (B) L2 in the presence of different metal ions in THF : H2O with 10 : 90% (L1) and 40 : 60% (L2) mixtures. Insets: Photographs of L1 and L2 in the presence of different metal ions in THF:H2O fractions under a UV lamp at 254 nm. Fluorescence spectra of (C) L1 and (D) L2 with different metal ions in THF:H2O. Insets: Photographs of L1 and L2 in the presence of various metals in AIE medium under a UV lamp at 365 nm. | |
Both the ligands exhibited fluorescence quenching towards four metal ions (Co2+, Fe2+, Cu2+ and Fe3+) in THF medium, whereas selective quenching towards Fe3+ ions was observed in the AIE medium. In pure THF medium, the acidic protons of Ar–OH in L1/L2 will be less labile; thus, the Ar–OH and C
N groups may provide soft base binding sites. In the presence of water along with THF in the AIE medium, the phenolic protons could be deprotonated to form phenoxide ion (Ar–O−), thus providing a hard base binding site.20 Metals such as Co2+, Fe2+ and Cu2+ ions are borderline acids and will have reasonable affinity with the ligands in THF medium (due to the soft base binding sites) and poor affinity with the ligands in the THF–water mixture (due to the hard base binding sites). On the other hand, Fe3+ ion, as a hard acid, will have higher affinity towards phenoxide ion in the THF–water mixture than the borderline acids; thus, the ligands L1 and L2 both demonstrate high selectivity towards Fe3+ ion in AIE medium.
The selectivity of the ligands in THF–H2O mixture was also tested by recording the fluorescence spectra in the presence of 1 × 10−5 M ligand containing 10 equivalents of Fe3+, to which equal concentrations of other metal ions were added (Fig. S11, ESI†). The black bars show that upon interaction with Fe3+, both L1 and L2 afforded significant changes in their fluorescence emission intensities. It could be observed that the tested metal ions present in equal amounts did not influence the fluorescence quenching caused by Fe3+. This confirms that remarkable selectivity towards Fe3+ ions (in the presence of different metal ions) can be attained with both the ligands in AIE medium.
Detection of Fe3+ using L1 and L2 in THF–H2O
To expand the scope of the AIE-active probes as suitable candidates for quantitative sensing of Fe3+ ions, the absorption and fluorescence spectra of L1 and L2 were examined in the presence of increasing concentrations of Fe3+ ions. The absorption spectra of L1 and L2 in the presence of different concentrations of Fe3+ ions are presented in Fig. S12(A) and (B) (ESI†). The absorption spectra of both L1 and L2 show increases in peak intensity upon the gradual addition of Fe3+ ions in THF–H2O (10%
:
90% and 40%
:
60%) at 255 and 355 nm, respectively. In addition, to investigate the efficiency of L1 and L2 towards Fe3+ recognition, we performed fluorescence titration in THF–H2O medium at an excitation wavelength of 255 nm. As shown in Fig. 5A and B, a gradual decrease in the fluorescence intensities of L1 at 317 and 355 nm as well as at 320 and 450 nm for L2 was observed upon successive addition of Fe3+ ion. The fluorescence quenching behaviour of L1 and L2 in the presence of Fe3+ confirms the formation of a ligand-to-metal charge transfer (LMCT) complex due to the photoinduced electron transfer (PET) process.
 |
| Fig. 5 Fluorescence titration spectra with 1 × 10−5 M (A) L1 and L2 (B) in THF–H2O (10% : 90% and 40% : 60%) upon successive addition of Fe3+ ions (0 to 5 equivalents) (λex = 255 nm). Insets: Ksv plots for L1 and L2. B–H plots of (C) L1 and (D) L2 using 1 : 1 stoichiometry for the associations of L1 and L2 with Fe3+ ions. Insets: Job's plot for the complexation of L1 and L2 with Fe3+ ions plotted using absorbance changes by the mole fraction method. Fluorescence lifetime decay plots of L1 (E) and L2 (F) in the absence and presence of Fe3+ ion. | |
In order to interpret the fluorescence quenching behaviour of the ligands in the presence of Fe3+ ions, the quenching efficiency was calculated using the Stern–Volmer equation I0/I = 1 + Ksv[Q]21 (where I0 is the initial fluorescence intensity of the ligand in the absence of Fe3+, I is the fluorescence intensity in the presence of Fe3+ and Q is the quenching constant). The plot of I0/I against the concentration of Fe3+ was found to be linear, and the Stern–Volmer constants (Ksv) were estimated to be 1.929 × 104 M−1 and 6.932 × 104 M−1 for L1 and L2, respectively. The S–V plots suggest that fluorescence involves a static quenching process, and the obtained Ksv values were found to be comparable to or better than those of previously reported Fe3+ sensors.22
Further, the binding affinities were estimated using the Benesi–Hildebrand (B–H) plot of 1/[I − I0] vs. 1/[Fe3+]. The B–H plots for the titration of the ligands with Fe3+ ions resulted in linear relationships, and the association constants (Ka) of the probes L1 and L2 (Fig. 5C and D) were calculated to be 1.317 × 105 M−1 and 2.930 × 105 M−1, respectively. The higher association constants (Table 2) confirm the strong binding affinities of both the ligands for complexation with Fe3+, resulting in sensitive detection of the same. In order to probe the reason for the selectivity of our ligands towards Fe3+ ion in AIE medium, we calculated Ka for Fe2+, Fe3+, Co2+ and Cu2+ metals in THF and AIE medium (Table S3, ESI†). All the metal ions showed very similar binding constants in THF medium. However, the Ka of Fe3+ with L1/L2 was very high (∼10 to 30 times) compared to those of Fe2+, Co2+ and Cu2+ in AIE medium. This observation demonstrates that the ligands L1/L2 exhibit higher binding affinity towards Fe3+ in AIE medium than the other metals investigated, which affords higher selectivity.
To determine the binding sites and ligand–metal stoichiometry, the continuous variation (Job's plot) methodology was used by plotting the changes in absorption maximum as a function of mole fraction at 255 and 256 nm for L1 and L2, respectively. During UV-visible absorption titrations, the plot exhibited maximum absorbance when the mole fraction of Fe3+ reached 0.5; thus, the stoichiometries of both L1 and L2 towards Fe3+ complex were established to be 1
:
1 (insets to Fig. 5C and D). From the fluorescence titrations, the detection limits of sensors L1 and L2 for Fe3+ ions were calculated to be 0.163 and 3.99 μM, respectively, using the equation 3σ/S, where σ denotes the standard deviation of blank measurements and S is the slope obtained for the plot of intensity vs. Fe3+ ion concentration. As shown in Table 2, sensors L1 and L2 both exhibit promising analytical characteristics in terms of high selectivity, high binding efficiency and low detection limit towards the quantitative detection of Fe3+ ion.
Quantum yield and lifetime measurements
The sensing behaviour of the probes L1 and L2 towards the detection of Fe3+ was also examined with quantum yield and lifetime measurements. The fluorescence decay behaviour of L1 and L2 in the presence and absence of Fe3+ ions was assessed using time-resolved spectrofluorometry, and the results are given in Fig. 5E and F. The average fluorescence lifetimes of L1 and L2 in THF–H2O were found to be 6.98 and 4.86 ns, respectively. However, shorter fluorescence lifetimes of 3.49 and 2.5 ns were observed for L1 and L2, respectively, in the presence of Fe3+ ions. We also estimated the radiative (kr) and nonradiative (knr) decay values of both L1 and L2 in the presence of Fe3+, and the values are summarized in Table 1. The calculated kr and Knr values of both L1 and L2 with Fe3+ show a decrease in kr decay and an increase in Knr decay. This reasonable reduction of radiative processes and enhancement of nonradiative processes results in fluorescence quenching of L1 and L2 in the presence of Fe3+ ions.
Table 1 Lifetime and quantum yield parameters of L1 and L2 in THF
:
H2O (10%
:
90% and 40%
:
60%) in the presence and absence of Fe3+
Sample |
τ
avg
|
Φ
|
K
r (ns−1) |
K
nr (ns−1) |
L1
|
6.98 |
0.174 |
0.024 |
0.118 |
L1 + Fe3+ |
3.49 |
0.013 |
0.0037 |
0.282 |
L2
|
4.86 |
0.136 |
0.027 |
0.177 |
L2 + Fe3+ |
2.5 |
0.01 |
0.004 |
0.396 |
Table 2 Comparison of L1 and L2 with recently reported fluorescent Fe3+ sensors
Fluorophore used |
Solvent medium (%) |
K
a (M−1) |
LOD (μM) |
Ref. |
Pyrrolo[3,4]pyridine |
DMSO : H2O (10 : 90) |
3.24 × 105 |
38.4 |
23
|
Novel BODIPY |
CH3CN : H2O (10 : 90) |
1.0 × 105 |
0.13 |
24
|
Acridine-based fluorophore |
DMSO : H2O (50 : 50) |
1.03 × 104 |
4.13 |
25
|
Quinoline derivative |
Tris–HCl |
5.1 × 104 |
0.12 |
26
|
1H-Imidazo-[4,5-b]phenazine |
DMSO |
3.91 × 105 |
4.8 |
27
|
Cell BODIPY |
H2O |
2.5 × 105 |
1.72 |
28
|
TPETHRB (rhodamine B) |
CH3CN : H2O (60 : 40) |
— |
3.2 |
29
|
Schiff base ligand L1 |
THF : H2O (90 : 10) |
1.31 × 105 |
0.63 |
This work |
Schiff base ligand L2 |
THF : H2O (60 : 40) |
2.93 × 105 |
3.99 |
This work |
Further, the quantum yield values were calculated in the presence and absence of Fe3+ in THF
:
H2O (10%
:
90% for L1 and 40%
:
60% for L2). The quantum yields of L1 and L2 in the absence of Fe3+ were found to be 0.174 and 0.136, which decreased to 0.013 and 0.01, respectively, in the presence of Fe3+ ions. The drastic drop in the quantum yield values during the ligand–Fe3+ complexation ensures that the interactions of L1 and L2 with Fe3+ in the excited state lead to fluorescence quenching.
Theoretical studies
To gain better insight into the binding mechanisms of L1 and L2 with Fe3+ ion, density functional theory (DFT) calculations were performed using the Gaussian 09W program in the gas phase. The spatial distributions as well as the electron distributions in the molecular orbitals of the ligands and ligand–Fe3+ complexes were also explored using DFT. The basis set at 6-31G** was used for the N, O, C, H, and Cl atoms of L1 and L2, whereas LANL2DZ was utilized for Fe3+ atom. The optimized geometries of L1, L2, L1-Fe3+ and L2-Fe3+ are shown in Fig. 6. The interaction energy (Eint = Ecomplex − EL) of L1-Fe3+ was found to be 1140.129, and 1140.177 kcal mol−1 was found for L2-Fe3+. The obtained bond lengths of N–Fe3+, O1–Fe3+ and O2–Fe3+ of the L1 and L2 complexes were 1.9116, 1.7938 and 1.8397 Å and 1.9971, 1.7628 and 1.8158 Å, respectively.
 |
| Fig. 6 DFT optimized structures of L1, L2, L1-Fe3+ and L2-Fe3+. | |
The electron distributions of the L1/L2-Fe3+ complexes were investigated on the basis of frontier molecular orbitals (FMO), and their structures are shown in Fig. 7A and B. In the cases of L1 and L2, the highest occupied molecular orbitals (HOMO) and lowest unoccupied molecular orbitals (LUMO) are fully located over the molecules, with band gaps of 3.93 and 3.91 eV, respectively; this facilitates π–π* electron transfer from the L1 and L2 molecules. In the case of ligands with Fe3+ complexation, the electron clouds of the ligands spread from the ligands to the metal, and the LMCT is due to the unpaired electrons present in the FMO. The unpaired electrons of the FMO further split into α-FMO and β-FMO. The α, β-HOMO and LUMO orbitals of L1-Fe3+ and L2-Fe3+ are located on the ligands as well as on the metal complexes, with band gaps of 1.83 and 2.23 eV for α-FMO and 1.95 and 2.22 eV for β-FMO, respectively. These results confirm the excited state π–π* transitions, which result in fluorescence emission quenching. The energy gaps of L1 and L2 increased and the energy band gaps of the ligand–Fe3+ complexes decreased for both the ligands. This band gap analysis reveals the stabilization of the complex due to the lowering of energy and the strong complexation occurring in L1-Fe3+ and L2-Fe3+. The formation of the 1
:
1 LMCT complex ratio confirms the 1
:
1 stoichiometry, which is supplemented well with the Job plot experimental results of the fluorophores L1 and L2; this further corroborates the PET process.
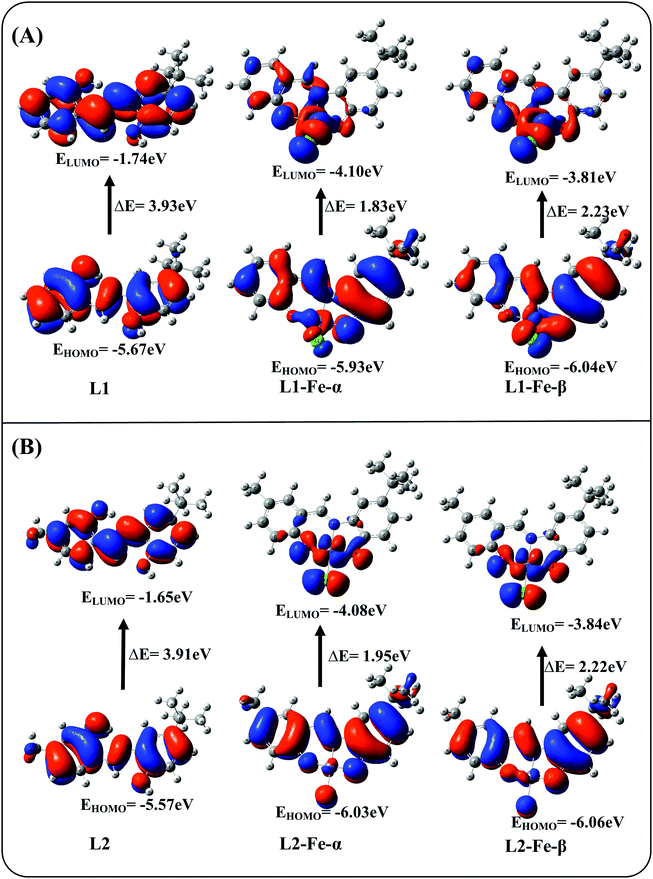 |
| Fig. 7 Frontier molecular orbitals of (A) L1 and (B) L2 and their Fe3+ complexes calculated using the DFT/B3LYP method. | |
Electrochemical investigation of the ligand–Fe3+ interactions
The interactions of ligands L1 and L2 with Fe3+ ion were further explored by differential pulse voltammetry (DPV). The DPVs of 1 mM L1 and L2 in the absence and presence of incremental concentrations of Fe3+ were recorded in CH3OH with 0.1 M TBAP as the supporting electrolyte at a scan rate of 50 mV s−1 (Fig. 8A and B). The voltammograms of L1 and L2 show two sets of well-defined oxidation peaks. The oxidation peaks for ligand L1 occurred at 0.78 and 1.01 V and those of L2 occurred at 0.79 and 1.15 V. The similar oxidation potentials of the first peaks for L1/L2 are due to the oxidation of tert-butyl-substituted phenolic hydroxyl groups to phenoxyl radical (PhO˙).30 The second peak at 1.01 V corresponds to the oxidation of the unsubstituted phenolic hydroxyl group of L1, and that at 1.15 V corresponds to the oxidation of the methyl-substituted phenolic hydroxyl group. Upon successive addition of 50 to 200 μM Fe3+ ions, the peak currents for both the oxidation peaks decreased gradually with slight positive shifts in their potentials, and a new signal appeared. The new peaks appeared at 0.89 and 1.23 V for L1 and at 0.91 and 1.28 V for L2. The positive shifts in the oxidation potentials in the presence of Fe3+ ions clearly indicate that both the hydroxyl groups of the ligands are coordinated to the metal ion. These observations further substantiate the binding mechanisms proposed in the previous sections and provide a new avenue for developing electrochemical sensors for the detection of Fe3+ using these newly developed Schiff base compounds.
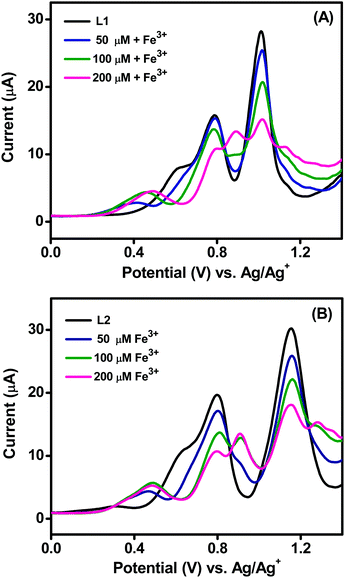 |
| Fig. 8 DPVs of 1 mM (A) L1 and (B) L2 with incremental addition of Fe3+ ion in methanol containing 0.1 M TBAP at a scan rate of 50 mV s−1. | |
Conclusions
In this work, we have synthesized simple Schiff base AIE-active ligands L1 and L2 and developed these ligands as efficient naked eye probes and fluorescent turn-off sensors for the selective detection of Fe3+ ions in THF–H2O medium. A noticeable colour change could be visually observed for L1 and L2 in the presence of Fe3+ ion. As fluorescent sensors, both the ligands were highly selective towards Fe3+ among other coexisting metal ions with a 1
:
1 binding ratio, and the detection limits were found to be 0.163 μM and 3.99 μM for L1 and L2, respectively. The fluorescence turn-off responses of L1 and L2 to Fe3+ can be attributed to a PET inhibition mechanism, as was demonstrated using DFT calculations and lifetime measurements. The association constants (Ka) of L1 and L2 in the presence of Fe3+ were calculated to be 1.317 × 105 M−1 and 2.930 × 105 M−1, respectively. The quantum yields of both ligands are higher than those of their Fe3+ complexes, which is indicative of fluorescence quenching by Fe3+ ion. The binding of ligands with Fe3+ was also ascertained by electrochemical investigations. Due to the low detection limits and the strong and selective binding attained with the newly developed AIE-active fluorophores, they are expedient for the detection of Fe3+ ions in complex environmental and biological samples.
Conflicts of interest
There are no conflicts to declare.
Acknowledgements
The authors thank the Vellore Institute of Technology (VIT) for providing a VIT SEED GRANT for carrying out this research work and are also thankful to DST-SIF, VIT for providing NMR, IR, GC-MS and other analytical facilities.
References
-
(a) J. L. Bricks, A. Kovalchuk, C. Trieflinger, M. Nofz, M. Buschel, A. L. Tolmachev, J. Daub and K. Rurack, On the development of sensor molecules that display FeIII-amplified fluorescence, J. Am. Chem. Soc., 2005, 127(39), 13522–13529 CrossRef CAS PubMed;
(b) S. Wang, X. Meng and M. Zhu, A naked-eye rhodamine-based fluorescent probe for Fe(III) and its application in living cells, Tetrahedron Lett., 2011, 52(22), 2840–2843 CrossRef CAS.
-
(a) D. Galaris, V. Skiada and A. Barbouti, Redox signaling and cancer: the role of “labile” iron, Cancer Lett., 2008, 266(1), 21–29 CrossRef CAS PubMed;
(b) H. Kozlowski, A. Janicka-Klos, J. Brasun, E. Gaggelli, D. Valensin and G. Valensin, Copper, iron, and zinc ions homeostasis and their role in neurodegenerative disorders (metal uptake, transport, distribution and regulation), Coord. Chem. Rev., 2009, 253(21–22), 2665–2685 CrossRef CAS;
(c) S. Sen, S. Sarkar, B. Chattopadhyay, A. Moirangthem, A. Basu, K. Dhara and P. Chattopadhyay, A ratiometric fluorescent chemosensor for iron: discrimination of Fe2+ and Fe3+ and living cell application, Analyst, 2012, 137(14), 3335–3342 RSC.
-
(a) M. E. Del Castillo Busto, M. Montes-Bayon, E. Blanco-Gonzalez, J. Meija and A. Sanz-Medel, Strategies to study human serum transferrin isoforms using integrated liquid chromatography ICPMS, MALDI-TOF and ESI-Q-TOF detection: application to chronic alcohol abuse, Anal. Chem., 2005, 77(17), 5615–5621 CrossRef CAS PubMed;
(b) S. R. Liu and S. P. Wu, New water-soluble highly selective fluorescent chemosensor for Fe(III) ions and its application to living cell imaging, Sens. Actuators, B, 2012, 171, 1110–1116 CrossRef;
(c) M. G. Van Den Berg, Chemical speciation of iron in seawater by cathodic stripping voltammetry with dihydroxynaphthalene, Anal. Chem., 2006, 78(1), 156–163 CrossRef PubMed;
(d) S. Lunvongsa, M. Oshima and S. Motomizu, Determination of total and dissolved amount of iron in water samples using catalytic spectrophotometric flow injection analysis, Talanta, 2006, 68(3), 969–973 CrossRef CAS PubMed.
- D. Wei, Y. Sun, J. Yin, G. Wei and Y. Du, Design and application of Fe3+ probe for “naked-eye” colorimetric detection in fully aqueous system, Sens. Actuators, B, 2011, 160(1), 1316–1321 CrossRef CAS.
-
(a) A. P. D. Silva, H. Q. N. Gunaratne, T. Gunnlaugsson, A. J. M. Huxley, C. P. McCoy, J. T. Rademacher and T. E. Rice, Signaling recognition events with fluorescent sensors and switches, Chem. Rev., 1997, 97(5), 1515–1566 CrossRef PubMed;
(b) K. Velmurugan, J. Prabhu, A. Raman, N. Duraipandy, M. S. Kiran, S. Easwaramoorthi, L. Tang and R. Nandhakumar, Dual functional fluorescent chemosensor for discriminative detection of Ni2+ and Al3+ ions and its imaging in living cells, ACS Sustainable Chem. Eng., 2018, 6(12), 16532–16543 CrossRef CAS;
(c) P. C. A. Swamy and P. Thilagar, Triarylborane-Appended new triad and tetrad: chromogenic and fluorogenic anion recognition, Inorg. Chem., 2014, 53(6), 2776–2786 CrossRef PubMed;
(d) N. Murugan and A. K. Sundramoorthy, Green synthesis of fluorescent carbon dots from Borassus flabellifer flowers for label-free highly selective and sensitive detection of Fe3+ ions, New J. Chem., 2018, 42(16), 13297–13307 RSC.
-
(a) G. G. Huang, Y. T. Chen and Y. R. Lin, Development of a gold nanoparticle based anti-aggregation method for rapid detection of mercury(II) in aqueous solutions, Anal. Methods, 2014, 6(15), 5690–5696 RSC;
(b) S. K. Sahoo, D. Sharma, R. K. Bera, G. Crisponi and J. F. Callan, Iron(III) selective molecular and supramolecular fluorescent probes, Chem. Soc. Rev., 2012, 41(21), 7195–7227 RSC;
(c) B. Valeur and I. Leray, Design principles of fluorescent molecular sensors for cation recognition, Coord. Chem. Rev., 2000, 205(1), 3–40 CrossRef CAS;
(d) M. Zulfajri, G. Gedda, C. J. Chang, Y. P. Chang and G. G. Huang, Cranberry beans derived carbon dots as a potential fluorescence sensor for selective detection of Fe3+ ions in aqueous solution, ACS Omega, 2019, 4(13), 15382–15392 CrossRef CAS PubMed.
-
(a) W. J. Ansorge, Next-generation DNA sequencing techniques, New Biotechnol., 2009, 25(4), 195–203 CrossRef CAS PubMed;
(b) K. P. Carter, A. M. Young and A. E. Palmer, Fluorescent sensors for measuring metal ions in living systems, Chem. Rev., 2014, 114(8), 4564–4601 CrossRef CAS PubMed.
-
(a) Y. Hong, J. W. Y. Lam and B. Z. Tang, Aggregation-induced emission, Chem. Soc. Rev., 2011, 40(11), 5361–5388 RSC;
(b) Z. Zhao, J. W. Y. Lam and B. Z. Tang, Aggregation-induced emission of tetraarylethene luminogens, Curr. Org. Chem., 2010, 14(18), 2109–2132 CrossRef CAS.
-
(a) W. L. Gong, M. P. Aldred, G. F. Zhang, C. Li and M. Q. Zhu, Aggregation-induced emission logic gates based on metal ion sensing of phenanthroline-tetraphenylethene conjugates, J. Mater. Chem. C, 2013, 1(45), 7519–7525 RSC;
(b) Q. Li, X. Wu, X. Huang, Y. Deng, N. Chen, D. Jiang, L. Zhao, Z. Lin and Y. Zhao, Tailoring the fluorescence of AIE active metal-organic frameworks for aqueous sensing of metal ions, ACS Appl. Mater. Interfaces, 2018, 10(4), 3801–3809 CrossRef CAS PubMed;
(c) M. Gao and B. Z. Tang, Fluorescent sensors based on aggregation-induced emission: recent advances and perspectives, ACS Sens., 2017, 2(10), 1382–1399 CrossRef CAS PubMed;
(d) J. Tavakoli, S. Pye, A. H. M. M. Reza, N. Xie, J. Qin, C. L. Raston, B. Z. Tang and Y. Tang, Tuning aggregation-induced emission nanoparticle properties under thin film formation, Mater. Chem. Front., 2020, 4, 537–545 RSC.
-
(a) Y. Yoon, S. Jo, S. J. Park, H. M. Kim, D. Kim and T. S. Lee, Unusual fluorescence of o-phenylazonaphthol derivatives with aggregation-induced emission and their use in two-photon cell imaging, Chem. Commun., 2019, 55(47), 6747–6750 RSC;
(b) S. Mukherjee and P. Thilagar, Insights into the AIEE of 1,8-Naphthalimides (NPIs): inverse effects of intermolecular interactions in solution and aggregates, Chem. – Eur. J., 2014, 20(26), 8012–8023 CrossRef CAS PubMed.
- J. Mei, N. L. C. Leung, R. T. K. Kwok, J. W. Y. Lam and B. Z. Tang, Aggregation-induced emission: together we shine, united we soar!, Chem. Soc. Rev., 2015, 115(21), 11718–11940 CrossRef CAS PubMed.
-
(a) Y. Chen, W. Zhang, Y. Cai, R. T. Kwok, Y. Hu, J. W. Y. Lam, X. Gu, Z. He, Z. Zhao, X. Zheng, B. Chen, C. Gui and B. Z. Tang, AIEgens for dark through-bond energy transfer: design, synthesis, theoretical study and application in ratiometric Hg2+ sensing, Chem. Sci., 2017, 8(3), 2047–2055 RSC;
(b) M. Shellaiah, Y. H. Wu, A. Singh, M. V. R. Raju and H. C. Lin, Novel pyrene- and anthracene-based Schiff base derivatives as Cu2+ and Fe3+ fluorescence turn-on sensors and for aggregation induced emissions, J. Mater. Chem. A, 2013, 1(4), 1310–1318 RSC;
(c) Z. Song, T. K. K. Ryan, D. Ding, H. Nie, J. W. Y. Lam, B. Liu and B. Z. Tang, An AIE-active fluorescence turn-on bioprobe mediated by hydrogen-bonding interaction for highly sensitive detection of hydrogen peroxide and glucose, Chem. Commun., 2016, 52(65), 10076–10079 RSC;
(d) R. T. Kwok, C. W. Leung, J. W. Y. Lam and B. Z. Tang, Biosensing by luminogens with aggregation induced emission characteristics, Chem. Soc. Rev., 2015, 44(13), 4228–4238 RSC.
-
(a) Y. He, Y. Li, H. Su, Y. Si, Y. Liu, Q. Peng, J. He, H. Hou and K. Li, An o-phthalimide-based multistimuli-responsive aggregation-induced emission (AIE) system, Mater. Chem. Front., 2019, 3(1), 50–56 RSC;
(b) S. K. Padhan, N. Murmu, S. Mahapatra, M. K. Dalai and S. N. Sahu, Ultrasensitive detection of aqueous Cu2+ ions by a coumarin-salicylidene based AIEgen, Mater. Chem. Front., 2019, 3(11), 2437–2447 RSC;
(c) J. Prabhu, K. Velmurugan, A. Raman, N. Duraipandy, M. S. Kiran, S. Easwaramoorthi and R. Nandhakumar, A simple chalcone based ratiometric chemosensor for sensitive and selective detection of nickel ion and its imaging in live cells, Sens. Actuators, B, 2017, 238, 306–317 CrossRef CAS;
(d) B. Fan, J. Wei, X. Ma, X. Bu, N. Xing, Y. Pan, L. Zheng and W. Guan, Synthesis of lanthanide-based room temperature ionic liquids with strong luminescence and selective sensing of Fe(III) over mixed metal ions, Ind. Eng. Chem. Res., 2016, 55(7), 2267–2271 CrossRef CAS.
-
(a) T. Han, Y. Hong, N. Xie, S. Chen, N. Zhao, E. Zhao, J. W. Y. Lam, H. H. Sung, Y. Dong, B. Tong and B. Z. Tang, Defect-sensitive crystals based on diaminomaleonitrile functionalized Schiff base with aggregation-enhanced emission, J. Mater. Chem. C, 2013, 1(44), 7314–7320 RSC;
(b) W. Tang, Y. Xiang and A. Tong, Salicylaldehyde azines as fluorophores of aggregation induced emission enhancement characteristics, J. Org. Chem., 2009, 74(5), 2163–2166 CrossRef CAS PubMed;
(c) M. Ziolek, M. Gil, J. A. Organero and A. Douhal, What is the difference between the dynamics of anion- and keto-type of photochromic salicylaldehyde azine?, Phys. Chem. Chem. Phys., 2010, 12(9), 2107–2115 RSC.
-
(a) N. Zhao, Y. H. Wu, J. Luo, L. X. Shi and Z. N. Chen, Aggregation-induced phosphorescence of iridium(III) complexes with 2,2′-bipyridine-acylhydrazone and their highly selective recognition to Cu2+, Analyst, 2013, 138(3), 894–900 RSC;
(b) Y. Q. Sun, P. Wang, J. Liu, J. Zhang and W. Guo, A fluorescent turn-on probe for bisulfite based on hydrogen bond-inhibited C
N isomerization mechanism, Analyst, 2012, 137(15), 3430–3433 RSC.
- W. G. Jia, H. Zhang, T. Zhang, D. Xie, S. Ling and E. H. Sheng, Half-Sandwich ruthenium complexes with Schiff-Base ligands: syntheses, characterization, and catalytic activities for the reduction of nitroarenes, Organometallics, 2016, 35(4), 503–512 CrossRef CAS.
-
(a) R. Kagit, M. Yildirim, O. Ozay, S. Yesilot and H. Ozay, Phosphazene based multicentered naked eye fluorescent sensor with high selectivity for Fe3+ ions, Inorg. Chem., 2014, 53(4), 2144–2151 CrossRef CAS PubMed;
(b) O. Sadak, A. K. Sundramoorthy and S. Gunasekaran, Highly selective colorimetric and electrochemical sensing of iron(III) using Nilered functionalized graphene film, Biosens. Bioelectron., 2017, 89, 430–436 CrossRef CAS PubMed.
- D. Roy, A. Chakraborty and R. Ghosh, Perimidine based selective colorimetric and fluorescent turn-off chemosensor of aqueous Cu2+: studies on its antioxidant property along with its interaction with calf thymus-DNA, RSC Adv., 2017, 7(64), 40563–40570 RSC.
- S. Densil, C. H. Chang, C. L. Chen, A. Mathavan, A. Ramdass, V. Sathish, P. Thanasekaran, W. S. Li and S. Rajagopal, Aggregation-induced emission enhancement of anthracene derived Schiff base compounds and their application as a sensor for bovine serum albumin and optical cell imaging, Luminescence, 2018, 33(4), 780–789 CrossRef CAS PubMed.
- K. Boonkitpatarakul, J. Wang, N. Niamnont, B. Liu, L. Mcdonald, Y. Pang and M. Sukwattanasinitt, Novel turn-on fluorescent sensors with mega Stokes shifts for dual detection of Al3+ and Zn2+, ACS Sens., 2016, 1(2), 144–150 CrossRef CAS.
- X. G. Li, Y. Liao, M. R. Huang, V. Strong and R. B. Kaner, Ultra-sensitive chemosensors for Fe(III) and explosives based on highly fluorescent oligofluoranthene, Chem. Sci., 2013, 4(5), 1970–1978 RSC.
- D. Yang, C. Dai, Y. Hu, S. Liu, L. Weng, Z. Luo, Y. Cheng and L. Wang, A new polymer-based fluorescent chemosensor incorporating propane-1,3-dione and 2,5-diethynylbenzene moieties for detection of copper(II) and iron(III), Polymers, 2017, 9(7), 267 CrossRef PubMed.
- P. Maity, B. Naskar, S. Goswami, C. Prodhan, T. Chaudhuri, K. Chaudhuri and C. Mukhopadhyay, Pyrrolo[3,4-c]pyridine-based fluorescent chemosensor for Fe3+/Fe2+ sensitivity and their application in living HepG2 cells, ACS Omega, 2018, 3(12), 18646–18655 CrossRef CAS.
- B. Sui, S. Tang, T. Liu, B. Kim and K. D. Belfield, Novel BODIPY based fluorescence turn-on sensor for Fe3+ and its bioimaging application in living cells, ACS Appl. Mater. Interfaces, 2014, 6(21), 18408–18412 CrossRef CAS PubMed.
- C. Wang, J. Fu, K. Yao, K. Xue, K. Xu and X. Pang, Acridine-based fluorescence chemosensors for selective sensing of Fe3+ and Ni2+ ions, Spectrochim. Acta, Part A, 2018, 199, 403–411 CrossRef CAS PubMed.
- B. Zhang, H. Liu, F. Wu, G. Hao, Y. Chen, C. Tan, Y. Tan and Y. Jiang, A dual-response quinoline-based fluorescent sensor for the detection of copper(II) and iron(III) ions in aqueous medium, Sens. Actuators, B, 2017, 243, 765–774 CrossRef CAS.
- G. Y. Gao, W. J. Qu, B. B. Shi, P. Zhang, Q. Lin, H. Yao, W. L. Yang, Y. M. Zhang and T. B. Wei, A highly selective fluorescent chemosensor for iron ion based on 1H-imidazo[4,5-b] phenazine derivative, Spectrochim. Acta, Part A, 2014, 121, 514–519 CrossRef CAS PubMed.
- M. Oguz, A. N. Kursunlu and M. Yilmaz, Low-cost and environmentally sensitive fluorescent cellulose paper for naked-eye detection of Fe(III) in aqueous media, Dyes Pigm., 2020, 173, 107974 CrossRef CAS.
- H. X. Yu, J. Zhi, Z. F. Chang, T. Shen, W. L. Ding, X. Zhang and J. L. Wang, Rational design of aggregation-induced emission sensor based on rhodamine B for turn-on sensing of trivalent metal cations, reversible data protection, and bioimaging, Mater. Chem. Front., 2019, 3(1), 151–160 RSC.
- D. Tomczyk, W. Bukowski and K. Bester, Redox processes in the solution of Ni(II) complex with salen type ligand and in the polymer films, Electrochim. Acta, 2018, 267, 181–194 CrossRef CAS.
Footnote |
† Electronic supplementary information (ESI) available: NMR, mass spectra, UV-visible and fluorescence spectral details of L1 and L2. See DOI: 10.1039/c9qm00792j |
|
This journal is © the Partner Organisations 2020 |