DOI:
10.1039/D0MA00184H
(Paper)
Mater. Adv., 2020,
1, 2737-2744
Hydrophilic surfaces from simple dip-coating method: amphiphilic block copolymers with zwitterionic group form antifouling coatings under atmospheric conditions†
Received
9th April 2020
, Accepted 13th September 2020
First published on 15th September 2020
Abstract
Hydrophilic surfaces with antifouling properties are in demand for medical and environmental applications, especially for ready-to-use purposes. In the present study, a simple and effective surface modification of ceramic surfaces via amphiphilic block polymers with zwitterionic group was developed. Block copolymers with polymer chains containing hydrophilic segments of poly(2-methacryloyloxyethyl phosphorylcholine) (MPC) and random copolymer chains of hydrophobic segments of poly(3-(methacryloyloxy)propyl-tris(tri(methylsilyloxy))silane) (MPTSSi) and silane coupling segments of poly(3-methacryloxypropyl trimethoxysilane) (MPTMSi) were synthesized and successfully applied uniformly on glass substrates. For the block polymer chains with longer hydrophilic segments, the surfaces exhibited high hydrophilicity under dry conditions and excellent antifouling properties without hydration pretreatment. The zwitterionic block copolymer-modified surface showed good durability and protein inhibition properties for pH of 2–9. The hydrophilic surface coating based on the developed block copolymers is suitable for medical, healthcare, sanitary, and environmental applications because it requires no pre-hydration.
1. Introduction
Ceramic materials have long been used in the healthcare industry for eyeglasses, diagnostic instruments, chemical wares, thermometers, tissue culture flasks, and fiber optics for endoscopic procedures.1 They are known as bioceramics and further applied in bioreactors, separation refining, separation analysis, water purification systems, and bathroom sanitation. In use, bioceramics are exposed to potential biofouling or biological contamination by proteins, bacteria, sebum, and mold.2,3 While ceramics consisting of mainly oxide are hydrophilic and negatively charged, contaminants can still be adsorbed on such surfaces.4,5 Therefore, additional enhancements to the antifouling properties are necessary for the surfaces of ceramics exposed to particular surroundings.
Various antifouling methods are used for ceramic surfaces, mainly implemented by modifying their hydrophobicity or hydrophilicity. On highly hydrophobic (superhydrophobic) surfaces, water is repelled from the surface; droplets roll off the surface, carrying water-soluble contaminant particles. However, oil-based contaminants cannot be removed by water droplets.6 Zheng et al. created a polyurethane/Pluronic® surface with a lotus leaf-inspired topography. The water contact angle was enhanced by the lotus-leaf modification of the material, which increased its roughness. Protein adsorption was significantly impeded on these lotus leaf-inspired surfaces.7 Similarly, Chen et al. found that micro-texturing reduced protein adsorption as well as platelet and cell adhesion on polydimethylsiloxane (PDMS) substrates.8 On highly hydrophilic surfaces, water droplets pass beneath both water-soluble and oil-based contaminants and wash them away. Water on such surfaces spreads quickly to form a thin film.6 There are reports that hydrophilic surfaces are preferred to hydrophobic surfaces for antifouling purposes.9,10 In particular, zwitterionic polymers, such as poly(2-methacryloyloxyethyl phosphorylcholine) (pMPC), poly(carboxybetaine methacrylate) (pCBMA), and poly(sulfobetaine methacrylate) (pSBMA), have shown antifouling properties with high hydrophilicity, lubricity, electrical neutrality, and favorable biocompatibility.11–14 Among them, pMPC is suitable because of large capacity for hydration of phosphorylcholine (PC) group and it is essential in providing antifouling and blood-compatible properties.9,15
To obtain the excellent biofouling of pMPC, brush structure with high graft density prepared by surface-initiated atom-transfer radical polymerization (SI-ATRP) is candidate,16–19 however it is difficult to obtain targeted properties with SI-ATRP because it requires precise polymerization control. Moreover, the method is disadvantageous in terms of cost. For that reason, the “grafting to” method using anchoring groups, or a dip-coating is an effective approach to prepare hydrophilic polymer coatings onto various substrates.20 In practical medical uses, MPC random copolymers are typically conjugated with hydrophobic components.21,22 The PC group is highly hydrated moiety, it is rarely located at the outermost surface of the MPC random copolymer with hydrophobic components under dry condition. Generally, such medical devices with surface modification of antifouling and biocompatible polymer are pretreated in water to achieve suitable water wetting property. In fact, the MPC random copolymer requires a long wetting time to achieve equilibrium hydration by the reorientation of the PC group.23,24 Ueda et al. discussed the mobility of the PC group in a copolymer with hydrophobic components, poly(MPC-r-n-butyl methacrylate (BMA)) (PMB), by evaluating the dynamic contact angle (DCA) with water.25 They showed that under dry conditions, the PC groups of PMB were overlaid by the hydrophobic components in order to decrease the interfacial free energy; this tendency was only reversed by pre-hydration. However, in medical devices and sanitary wares, it is better to eliminate a hydration pretreatment before use. This is because the hydrophobic moiety is located at the outermost surface of the polymer coating in air conditions, which prevent to achieve the target hydrophilic property. For example, considering the applications as an in situ diagnosis device, the sample solution should be inserted to the device without the pretreatment. For obtaining antifouling property at atmosphere condition, stable hydrophilic surface which does not need the hydration pretreatment is necessary.
The aim of this study is the fabrication of an antifouling and hydrophilic surface on ceramics without pre-hydration by surface modification utilizing a designed zwitterionic block copolymer. In this work, we selected pMPC as a zwitterionic polymer and a simple dip-coating of block copolymers as surface modification method. The concept for the block copolymer is having a zwitterionic hydrophilic segment and a segment for bonding to the ceramic surface, and the surface should exhibit hydrophilicity in both atmospheric and water condition (Fig. 1). To achieve durability of the coating copolymer film, we copolymerized a monomer with a silane coupling agent to the hydrophobic segment, which mediated chemical bonding via intermolecular silane coupling reactions.26,27 The effect of the design of the anchoring segment on the uniformity of the resulting polymer coating were investigated by changing the component of the segment. Moreover, we evaluated pH durability test for the polymer coating and the effect on protein adsorption, considering the potential applications in sanitary wares.
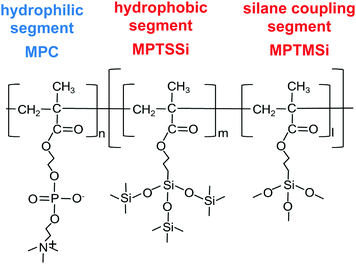 |
| Fig. 1 Chemical structure of block copolymer which is composed with hydrophilic block segment bind with random copolymer of hydrophobic and silane coupling segments. | |
2. Experimental section
2.1 Materials and methods
MPC was purchased from NOF Co. (Tokyo, Japan). 3-(Methacryloyloxy)propyl-tris(tri(methylsilyloxy)silane) (MPTSSi) and 3-methacryloxypropyl trimethoxysilane (MPTMSi) were purchased from Shin-Etsu Chemical Co. (Tokyo, Japan). Rhodamine 6G, hexane, ethanol, 1-propanol, acetone, diethyl ether, acetic acid, 1,1,1,3,3,3-hexafluoroisopropanol (HFIP), sodium dodecyl sulfate (SDS), and lysozyme from Egg White (Lot LKJ3000) were purchased from Wako Pure Chemical Industries Ltd (Osaka, Japan). These chemicals were used without purification. Dulbecco's phosphate-buffered saline (PBS, without calcium chloride or magnesium chloride) was purchased from Invitrogen Co. (Carlsbad, CA, USA). α,α′-Azobisisobutyronitrile (AIBN), ethanol-d6 as a solvent for nuclear magnetic resonance (NMR), deuterium oxide (D2O) for NMR, and bovine serum albumin (BSA) were purchased from Sigma-Aldrich (St. Louis, MO, USA). A μ-bicinchoninic acid (BCA) protein assay kit was purchased from Thermo Fisher Scientific Inc. (IL, USA). 4-Cyano-4-(phenylcarbonothioylthio)pentanoic acid (CPD) was purchased from Strem Chemicals, Inc. (Newburyport, MA, USA). Soda-lime glass (10 × 10 mm, 10 × 40 mm) was purchased from Matsunami Glass Inc., Ltd (Osaka, Japan) as standard ceramics. SiO2 of 10 nm thickness on a mirror-polished Si wafer was purchased from Furuuchi Chemical Co. (Tokyo, Japan) and used for the evaluation of the thicknesses of the coated copolymers.
The monomer contents in the obtained copolymers were determined by 1H NMR measurement (JEOL ECS-400, JOEL, Tokyo, Japan). D2O and ethanol-d6 were used for PMPC and for the other copolymers, respectively. The weight-averaged molecular weight (Mw) and number-averaged molecular weight (Mn) of the polymers were determined by gel permeation chromatography (GPC; JASCO RI-1530 detector, Tosoh Co, Tokyo, Japan, Column; TSK-GEL Super HM-M, solvent: HFIP, flow rate: 0.2 mL min−1). Poly(methyl methacrylate) was used as a standard.
2.2 Modification method of copolymer on substrates
The Si/SiO2 and glass substrates were washed by ultrasonic cleaning in hexane, ethanol, and acetone, followed by oxygen plasma treatment at 600 mTorr (PDC-001; Harrick Plasma, Ithaca, NY, USA). An aqueous solution containing 0.1 M acetic acid was added to the polymer solution (0.2 wt% in ethanol) to prepare the coating solution. The ratio of the polymer solution to acetic acid aqueous solution was 9
:
1 (w/w). The substrates were immersed in the copolymer solutions for 2 h, dried in solvent vapor atmosphere at room temperature for 2 h, and heated at 70 °C for 3 h. The copolymer-coated substrates were then immersed in distilled water to remove the remaining unreacted chemicals.
2.3 Modification method of copolymer on substrates
Uniformity of copolymer films.
An aqueous solution containing rhodamine 6G (200 ppm), which stains the PC group,29 was used to evaluate the uniformity of the copolymer film. After the copolymer-coated substrates were immersed in the rhodamine 6G solution, they were washed with distilled water and dried. Fluorescence images were obtained by a fluorescence microscope (Axioscope 2 Plus, Zeiss AG, Oberkochen, Germany, ×10 objective lens, exposure time: 1/30 s), equipped with a charge-coupled device (CCD) camera (VB-7010, Keyence Co., Osaka, Japan).
Characterization of copolymer films.
The thickness of the copolymer film on the substrate was measured by a spectroscopic ellipsometer (DVA-36L3, Mizojiri Opti-cal, Tokyo, Japan) using a He–Ne laser (632.8 nm) at the incident angle of 70°. X-ray photoelectron spectroscopy (XPS) was performed using a magnesium Kα source (JPS-9010MC, JEOL, Tokyo, Japan). The density of the copolymer film on the substrate was measured using X-ray reflectivity (XRR) (SmartLab (9 kW), Rigaku Co., Japan). The static water contact angle was measured in air to evaluate the wettability by a CA-W automatic contact angle meter (Kyowa Interface Science, Saitama, Japan). The water dynamic contact angles (DCAs) for the copolymer-coated surfaces were measured by the Wilhelmy plate method in order to evaluate the states of the hydrophilic units in both air and water. All copolymer-coated substrates were used without pre-hydration.
2.4 Protein adsorption test
The amounts of protein adsorbed on the copolymer-coated surfaces were measured by micro-BCA protein assay. Each sample was immersed in BSA solution (4.5 mg mL−1) or lysozyme (1.0 mg mL−1) at 37 °C for 30 min without pre-hydration. Then, the samples were rinsed with PBS and sonicated in 1 wt% aqueous sodium dodecyl sulfate (SDS) for 5 min to completely collect the adsorbed protein from the surface. Then, the μ-BCA protein assay was performed to determine the protein concentration in the SDS solution and thereby calculate the amount of protein adsorbed on each surface.
2.5 pH durability test
A pH solution was provided (pH = 1–12, HCl and NaOH (25 mM) in PBS). The copolymer-coated substrates (b-120 and b-160) were immersed in each pH solution for 1 h, washed with aqueous HCl, aqueous NaOH, and pure water, and then dried. To evaluate the existence of MPC copolymer, the rhodamine 6G staining test and surface element analysis by XPS were performed. Moreover, to determine the wettability retention, the static water contact angle was measured after pH treatment. To confirm the retention of antifouling properties, the amount of lysozyme (2.0 mg mL−1) adsorption was measured by the μ-BCA protein assay after pH treatment.
3. Results and discussion
3.1 Synthesis and characterization of the amphiphilic copolymers
The target block copolymer, poly(MPC-b-(MPTSSi-r-MPTMSi)) (Fig. 1) was synthesized by the two-step reversible addition–fragmentation chain transfer (RAFT) polymerization process. In the first phase, poly(MPC) (PMPC) was synthesized. The MPC monomer (0.5 M), AIBN as a radical initiator, and CPD as a RAFT agent were dissolved in 1-propanol, and polymerization was performed at 65 °C for 24 h. Without reprecipitation, the second step of copolymerization was performed. A 1-propanol solution containing MPTSSi and MPTMSi monomers were added to the reaction solution containing PMPC generated in the first polymerization. The second polymerization was performed at 65 °C for 24 h, and the resulting block copolymer was then collected by reprecipitation in the mixed solvent of diethyl ether and hexane (7/3, v/v). The block copolymers were dissolved in ethanol immediately after the reprecipitation. A random copolymer composed of MPC, MPTSSi, and MPTMSi, or poly(MPC-r-MPTSSi-r-MPTMSi) was also synthesized by using one-step RAFT polymerization.
Table 1 summarizes the characterizations of the obtained copolymers. All samples were named using the structure of the copolymer and the volume units of MPC in feed. In the abbreviations, “r” and “b” represent random copolymer and block copolymer, respectively. In the three types of poly(MPC-b-(MPTSSi-r-MPTMSi)), b-100, b-120, and b-160 indicate the use of 100, 120, and 160 monomer units of MPC, respectively. Note that they have the same total monomer units in feed, and different segment ratios. For comparison with the block copolymers, a random copolymer (poly(MPC-r-MPTSSi-r-MPTMSi); r-120, with 120 volume units MPC) was prepared under the same feed monomer contents as b-120. The monomer contents in these copolymers were determined by 1H NMR measurements. The weight-averaged molecular weight (Mw) and the polydispersity index (Mw/Mn) were estimated by using GPC. The monomer contents in the obtained copolymers were almost the same as those in feed. The molecular weights of these copolymers were similar. Thus, we successfully prepared the target amphiphilic block and random copolymers.
Table 1 Characterizations of the obtained copolymers
Abbr.a |
In feed (monomer contents) |
In copolymerb (monomer contents) |
M
w
|
M
w/Mnc |
MPC/MPTSSi/MPTMSi |
MPC/MPTSSi/MPTMSi |
All samples were named using the structure of the copolymer and the volume units of MPC in feed. In the abbreviations, “r” and “b” represent random copolymer and block copolymer, respectively.
Determined by 1H NMR measurement.
Determined by GPC measurement using polymethyl methacrylate (PMMA) as a standard.
|
r-120 |
120/40/40 |
112/36/34 |
9.5 × 104 |
1.6 |
b-100 |
100/50/50 |
98/44/42 |
9.3 × 104 |
1.5 |
b-120 |
120/40/40 |
114/36/31 |
8.4 × 104 |
1.5 |
b-160 |
160/20/20 |
158/18/16 |
8.3 × 104 |
1.5 |
3.2 Evaluation of the copolymer-coated surfaces
The block copolymers were coated onto the surface of Si/SiO2 or glass substrates by a dip coating method. We evaluated the modification of the copolymer and the uniformity of the resulting films by using rhodamine 6G, which can stain the PC unit in copolymer.28 In the fluorescence-dye staining method, the intensity corresponds to the amount of the ionic unit per area,29,30 which can be converted into the thickness by using the specific weight. Murata et al. reported that the density of the cationic group, which was estimated by fluorescein staining, increased as the thickness of the polymer brush increased.29 Cen et al. also applied the fluorescence-dye staining method to characterize the cationic polymer-grafted surfaces with various surface coverages.30 Firstly, we confirmed the applicability of the rhodamine 6G staining method to this copolymer coating. Fig. 2(a) shows the relationship between fluorescence intensity and film thickness of r-120. The thickness of the random copolymer, r-120 coating can be controlled as a function of the concentration of polymer in the coating solution. No significant staining was observed in the bare glass, and the relative fluorescence intensity increases with the film thickness of r-120 in the range up to 6 nm. When the film was thicker than 6 nm, the intensity does not correlate the film thickness. The results indicate that the fluorescence intensity can be used as an indicator of film thickness under 6 nm of film thickness. On the basis of this investigation, in-plane uniformity can was evaluated by the difference in brightness. As shown in Fig. 2(b), all samples including block-copolymer coated surfaces exhibited uniform fluorescence intensity. This result indicates that all of the synthesized copolymers were uniformly coated on the glass substrates.
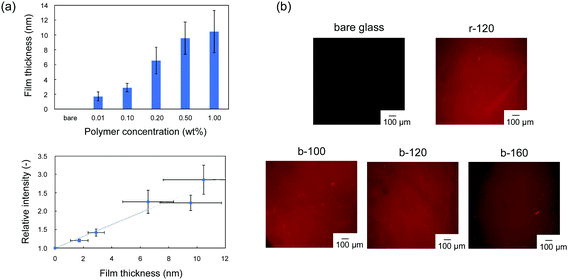 |
| Fig. 2 Film characterization of coated copolymer. (a) Upper figure shows the thickness of copolymer r-120 with treated of different concentrations of the copolymer in solution, under figure shows the relative intensity as a function of copolymer thickness of r-120. (b) Fluorescence images of bare glass, r-120, b-100, b-120, and b-160. All samples were stained by rhodamine 6G. | |
For evaluating the role of the hydrophobic random copolymer segment in poly(MPC-b-(MPTSSi-r-MPTMSi)), two kinds of diblock copolymers, poly(MPC-b-MPTSSi); p-120 without silane coupling segment, poly(MPC-b-MPTMSi); c-120 without hydrophobic segment, were synthesized. The characterizations of p-120 and c-120 are summarized in Table S1 and Fig. S1 (ESI†). The thicknesses of the p-120 and c-120 coatings were 12.1 ± 3.6 nm and approximately 8.4 ± 2.7 nm, respectively. The uniformity of the fluorescence intensity of b-120 is the best compared to those of p-120 and c-120; the c-120 coating contains brighter regions indicative of aggregates, probably caused by intramolecular condensation between c-120 chains.31 Thus, it is difficult to obtain uniform hydrophilic surface by a simple dip coating method using block copolymer composed of PMPC segment and homopolymer of silane coupling moiety. Furthermore, the uniformity of b-120 was the best compared with p-120 and c-120, suggesting that the strong intermolecular interaction between random copolymer segments of poly(MPTSSi-r-MPTMSi) facilitated structural formation.
Subsequently, we further evaluated the physicochemical properties of coted film to understand the functions of biocompatibility, such as anti-protein adsorption. Table 2 shows the characteristics of the copolymer films. All the synthesized block copolymer films are uniformly thin at <5 nm. Surface element analysis was performed using XPS and the nitrogen/carbon ratio (N/C) was calculated; the existence of the hydrophilic MPC polymer on the surface was evaluated because only the MPC unit contains nitrogen atoms. As the ratio of MPC unit increased in b-100, b-120, and b-160, the N/C value increased (Table 2), suggesting that each copolymer is successfully coated on the substrates. We measured the density of the copolymer films because it affects the antifouling properties of the MPC random copolymer.27 Among the block polymers, the density increased as the ratio of poly(MPTSSi-r-MPTMSi) segments increased. The MPTSSi segments connect to the ceramic surface via chemically, however, the density of r-120 is higher than that of b-120. This may be because the random copolymer has polymer–polymer crosslinking units in every part of the polymer, while the block copolymer only has them on one side of the copolymer. Furthermore, the static water contact angle values in air are also shown in Table 2. In the block copolymers, the static contact angles (SCAs) are decreased to around 20° with increased in the ratio of MPC units. On the r-120-coated surface, the static contact angle was approximately 90°. This result indicates that the surface prepared by a sufficiently long hydrophilic segment in a block copolymer, b-160, shows high hydrophilicity in air without pre-hydration.
Table 2 Results of polymer-coated surface analysis
Sample |
Film thickness in air (nm)a |
N/Cb (−) |
Densityc (g cm−3) |
SCA in air (deg) |
Determined by spectroscopic ellipsometry.
Determined by XPS.
Determined by XRR.
|
r-120 |
4.7 ± 0.5 |
0.05 ± 0.01 |
1.00 ± 0.10 |
94 ± 3 |
b-100 |
5.0 ± 0.5 |
0.04 ± 0.01 |
1.17 ± 0.01 |
67 ± 4 |
b-120 |
2.8 ± 0.3 |
0.05 ± 0.01 |
0.85 ± 0.04 |
25 ± 4 |
b-160 |
3.6 ± 0.6 |
0.06 ± 0.01 |
0.78 ± 0.04 |
20 ± 4 |
The synthesized copolymers contained both hydrophilic and hydrophobic components. The component of the copolymer is assumed to minimize surface free energy according to the surrounding environment would migrate to the surface. The important issue of this study is hydrophilic property with antifouling is appeared in air environment, and thus, further evaluation of the states of hydrophilic unit in air or in water was conducted by the use of dynamic contact angle (DCA) method. The hysteresis of contact angle from air to water, which is an indicator of hydrophilicity in air condition, was calculated as the difference between the advancing and receding contact angles. From Fig. 3(a), hysteresis of DCA on the block copolymer-coated surfaces is smaller than that on the random copolymer r-120-coated surface. With the block copolymers, as the ratio of MPC units increased to b-160, the hysteresis decreased. While the hydrophilic unit of the random copolymer has different states in air and water, those of the block copolymers with sufficient hydrophilic units suggest preserving the structure under air and water conditions as shown in Fig. 3(b). The possible nanostructure of the block copolymers composed of hydrophilic MPC polymer and hydrophobic polymer was considered based on our previous studies.32,33
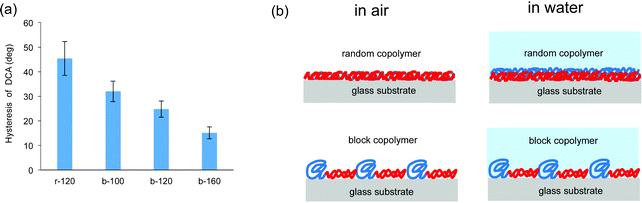 |
| Fig. 3 (a) Hysteresis of DCA for each copolymer and (b) illustration of possible polymer structures of coated films on the glass substrate under air and water conditions. | |
3.3 Protein adsorption test
Fig. 4 shows the amounts of BSA and lysozyme adsorbed on the substrates without pre-hydration. An albumin and lysozyme are the proteins containing in the egg white and having an opposite charge. Considering the contamination of environmental protein, they are the acceptable proteins to investigate the adsorption behaviour. In addition, as reported in a previous study, the size of protein affects the adsorption behaviour on the block copolymer-coated surfaces with phase-separated structure.34 To avoid this effect of protein size on the adsorption behaviour, BSA and lysozyme were selected as they have the same molecular weight order. Compared with r-120 and b-100, b-120 and b-160 exhibited decreased BSA and lysozyme adsorptions. The amounts of BSA adsorbed on b-120 and b-160 are not significantly different from those on bare glass, probably because both the BSA (isoelectric point (pI) = 4.7) and the glass surface are negatively charged, and it causes repulsive force.9 The amounts of BSA adsorbed on r-120 and b-100 were relatively higher compared with b-120, b-160, and glass. This would be because the contents of the hydrophobic segments were higher. However, b-120 and b-160 exhibited decreased adsorptions of lysozyme, which is positively charged. The hydrophilic surfaces from MPC block copolymer resist protein adsorption regardless of the electrical charge of proteins. The dependence on the electrostatic interactions between polymeric materials and proteins is in good agreement with our previous report.9 To avoid biofouling or cellular adhesion induced by adsorbed BSA, the amount of adsorbed BSA should be decreased to around 300 ng cm−2.5 These results suggest that b-120 and b-160 exhibited acceptable protein inhibition performances. In addition, the amounts of adsorbed BSA on of b-120 and b-160 were comparable to those of the random copolymer-based coatings.5,27 The films of both b-120 and b-160 have small DCA values. To reduce protein adsorption without pre-hydration, this suggests the hydrophilic surface produced by zwitterionic block copolymer with longer hydrophilic polymer chains must be maintained as illustrated in Fig. 3(b). To further enhance the protein inhibition performance, the densely-packed polymer brush structure prepared by the use of “grafting from” methods is desirable.35
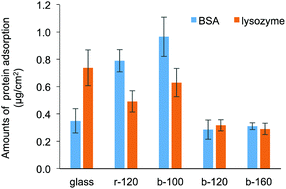 |
| Fig. 4 Amounts of BSA and lysozyme adsorbed on bare glass, r-120-, b-100-, b-120-, and b-160-coated substrates without pre-hydration. | |
3.4 pH durability test
Both medical devices and sanitary wares require durability; in the human body, the pH varies. In the applications as sanitary wares, the surface can be exposed to not only neutral but also acidic and basic detergent solution. Therefore, the pH resistances of the polymer coated ceramics were evaluated. The intensity of rhodamine 6G fluorescence after pH treatment is shown in Fig. 5(a) and (b). Fluorescence images after pH treatment are shown in Fig. S2 (ESI†). On both the b-120- and b-160-coated surfaces, the fluorescence intensity remains unchanged after treatment at pH = 2–8 compared to that without pH treatment. We also confirmed that the block copolymer-coated surface was stable at pH = 6–8 for 7 days (data for b-120 shown as Fig. S3 in ESI†). Meanwhile, the intensity dramatically decreased after treatment at pH = 1 and 10–12. After treatment at pH 9, the fluorescence intensity decreased slightly. As indicated from XPS measurements shown in Fig. 5(c) and (d), the N/C ratio changes in accordance with the results of the fluorescence intensity of rhodamine 6G staining. These results suggest that the films formed by b-120 and b-160 on glass substrate becomes detached from the glass substrate upon high pH treatment.
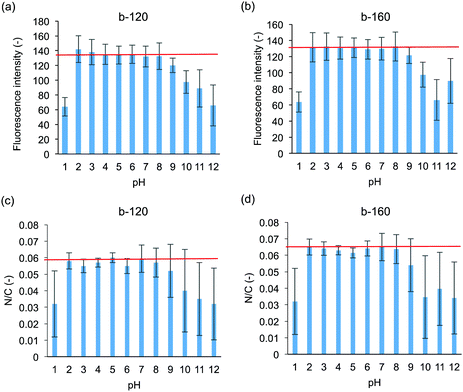 |
| Fig. 5 Results of fluorescence intensity of rhodamine 6G on (a) b-120 and (b) b-160 after pH treatment. Red line shows fluorescence without pH treatment. Results of N/C calculation from XPS measurement on (c) b-120 and (d) b-160 surfaces after pH treatment. Red line shows N/C without pH treatment. | |
We hypothesized that the cleavage of the coated polymer after pH treatment affects the antifouling property of the surface. Fig. 6 shows the amounts of lysozyme adsorption after treatment at pH 1, 5, 9, and 12. After treatment at pH 5, no significant copolymer detachment occurs, and both the b-120 and b-160 surfaces resist lysozyme adsorption at the same level as the untreated surfaces. However, after treatment at pH 1 and 12, much copolymer detachment occurs, and neither b-120 nor b-160 show lysozyme adsorption resistance. With treatment at pH 9, slight copolymer detachment occurs: the amount of lysozyme adsorption increases on the b-120 surface, but remains unchanged on b-160. One of the reasons for the detachment of the block copolymer with treating of pH 1 and 12 considers the corrosion of glass.36 This suggests that b-160 maintains its antifouling properties after some of the block copolymer is removed by pH treatment because it retains sufficient MPC units to provide antifouling.
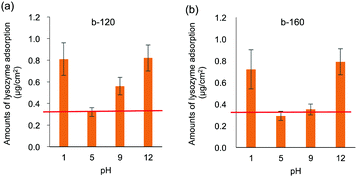 |
| Fig. 6 Lysozyme adsorption results on (a) b-120 and (b) b-160 after treatment at pH 1, 5, 9, and 12. Red line shows adsorption without pH treatment. | |
4. Conclusions
A hydrophilic surface requiring no pre-hydration was designed by the polymeric engineering of zwitterionic block polymers containing hydrophobic silane coupling segments on the glass. A series of poly(MPC-b-(MPTSSi-r-MPTMSi)) block copolymers synthesized by using RAFT polymerization was successfully applied to uniform polymer coating on glass substrates. Note that the uniform polymer coating was obtained owing to designing the hydrophobic segment as a random copolymer of hydrophobic MPTSSi and reactive MPTMSi. For block copolymers with longer hydrophilic segments, such as b-120 and b-160, the surfaces exhibited high hydrophilicity under atmospheric conditions. On such surfaces, protein adsorption decreased regardless of the protein species. The zwitterionic block copolymers of b-120 and b-160 showed excellent protein resistances, even though the density of b-120 was lower than that of the random copolymer r-120 synthesized as a control. Furthermore, after treatment at pH 2–8 of the b-120 and b-160 surfaces, the block copolymers were not significantly detached and protein adsorption resistance was maintained. After pH 9 treatment, some of the b-160 coated film was detached, but the coated substrate retained its antifouling properties. This demonstrates the pH durability of the b-160 copolymer in the range pH = 2–9. The hydrophilic surface with the zwitterionic block copolymer coating requires no pretreatment; this suggests applicability for medical, health care, and environmental devices such as catheters, biosensors, sanitary wares, and water treatment.
Conflicts of interest
There are no conflicts to declare.
Acknowledgements
This work was partially supported by a Grant-in-Aid for Scientific Research (A) from the Ministry of Education, Culture, Sports, Science and Technology of Japan. We greatly thank Dr Keiichiro Kushiro for helpful discussions on surface characterization.
Notes and references
- L. L. Hench, J. Am. Ceram. Soc., 1991, 74, 1487 Search PubMed.
- D. L. Taylor, T. M. Kahawita, S. Cairncross and J. H. H. Ensink, PLoS One, 2015, 10, DOI:10.1371/journal.pone.0135676.
- J. E. T. Grimes, G. Tadesse, I. A. Gardiner, E. Yard, Y. Wuletaw, M. R. Templeton, W. E. Harrison and L. J. Drake, PLoS Neglected Trop. Dis., 2017, 11, e0005948 Search PubMed.
- S. Vallar, D. Houviet, J. El Fallah, D. Kervadec and J.-M. Haussonne, J. Eur. Ceram. Soc., 1999, 19, 1017 Search PubMed.
- Y. Xu, M. Takai and K. Ishihara, Biomacromolecules, 2009, 10, 267 Search PubMed.
- S. Nishimoto and B. Bhushan, RSC Adv., 2013, 3, 671 Search PubMed.
- J. Zheng, W. Song, H. Huang and H. Chen, Colloids Surf., B, 2010, 77, 234 Search PubMed.
- H. Chen, W. Song, F. Zhou, Z. Wu, H. Huang, J. Zhang, Q. Lin and B. Yang, Colloids Surf., B, 2009, 71, 275 Search PubMed.
- D. Nagasawa, T. Azuma, H. Noguchi, K. Uosaki and M. Takai, J. Phys. Chem. C, 2015, 119, 17193 Search PubMed.
- T. Tamada and Y. Ikeda, J. Colloid Interface Sci., 1993, 155, 344 Search PubMed.
- L. Mi and S. Y. Jiang, Angew. Chem., Int. Ed., 2014, 53, 1746 Search PubMed.
- S. Y. Jiang and Z. Q. Cao, Adv. Mater., 2010, 22, 920 Search PubMed.
- J. B. Schlenoff, Langmuir, 2014, 30, 9625 Search PubMed.
- W. Yang, H. Xue, W. Li, J. Zhang and S. Jiang, Langmuir, 2009, 25, 11911 Search PubMed.
- T. Morisaku, J. Watanabe, T. Konno, M. Takai and K. Ishihara, Polymer, 2008, 49, 4652 Search PubMed.
- W. Feng, X. Gao, G. McClung, S. P. Zhu, K. Ishihara and J. L. Brash, Acta Biomater., 2011, 7, 3692 Search PubMed.
- M. Krishnamoorthy, S. Hakobyan, M. Ramstedt and J. E. Gautrot, Chem. Rev., 2014, 114, 10976 Search PubMed.
- T. Azuma, R. Ohmori, Y. Teramura, K. Ishihara and M. Takai, Colloids Surf., B, 2017, 159, 655 Search PubMed.
- C. Chu, Y. Higaki, C. H. Cheng, M. H. Cheng, C. W. Chang, J. T. Chen and A. Takahara, Polym. Chem., 2017, 8, 2309 Search PubMed.
- H. S. Sundaram, X. Han, A. K. Nowinski, J.-R. Ella-Menye, C. Wimbish, P. Marek, K. Senecal and S. Jiang, ACS Appl. Mater. Interfaces, 2014, 6, 6664 Search PubMed.
- T. A. Snyder, H. Tsukui, S. Kihara, T. Akimoto, K. N. Litwak, M. V. Kameneva, K. Yamazaki and W. R. Wagner, J. Biomed. Mater. Res., Part A, 2007, 81, 85 Search PubMed.
- J. L. Court, R. P. Redman, J. H. Wang, S. W. Leppard, V. J. Obyrne, S. A. Small, A. L. Lewis, S. A. Jones and P. W. Stratford, Biomaterials, 2001, 22, 3261 Search PubMed.
- A. Yamasaki, Y. Imamura, K. Kurita, Y. Iwasaki, N. Nakabayashi and K. Ishihara, Colloids Surf., B, 2003, 28, 53 Search PubMed.
- K. Futamura, R. Matsuno, T. Konno, M. Takai and K. Ishihara, Langmuir, 2008, 24, 10340 Search PubMed.
- T. Ueda, K. Ishihara and N. Nakabayashi, J. Biomed. Mater. Res., 1995, 29, 381 Search PubMed.
- B. Yuan, Q. Chen, W. Q. Ding, P. S. Liu, S. S. Wu, S. C. Lin, J. Shen and Y. Gai, ACS Appl. Mater. Interfaces, 2012, 4, 4031 Search PubMed.
- K. Nagahashi, Y. Teramura and M. Takai, Colloids Surf., B, 2015, 134, 384 Search PubMed.
- J. H. Wang, J. D. Bartlett, A. C. Dunn, S. Small, S. L. Willis, M. J. Driver and A. L. Lewis, J. Microsc., 2005, 217, 216 Search PubMed.
- H. Murata, R. R. Koepsel, K. Matyjaszewski and A. J. Russel, Biomaterials, 2008, 28, 4870 Search PubMed.
- L. Cen, K. G. Neoh and E. T. Kang, Langmuir, 2003, 19, 10295 Search PubMed.
- Y. Xie, C. A. S. Hill, Z. Xiao, H. Militz and C. Mai, Composites, Part A, 2010, 41, 806 Search PubMed.
- Y. Hiraguchi, K. Kushiro and M. Takai, Polymer, 2016, 99, 166 Search PubMed.
- K. Suthiwanich, Y. Hiraguchi, T. Nyu, E. A. Q. Mondarte, M. Takai and T. Hayashi, Chem. Lett., 2020, 49, 641 Search PubMed.
- T. Masuda, Y. Hiraguchi, K. Kushiro, Y. Araki, T. Wada and M. Takai, Eur. Polym. J., 2020, 135, 109885 Search PubMed.
- W. Feng, J. L. Bruah and S. Zhu, Biomaterials, 2006, 27, 847 Search PubMed.
- B. C. Bunker, J. Non-Cryst. Solids, 1994, 179, 300 Search PubMed.
Footnote |
† Electronic supplementary information (ESI) available. See DOI: 10.1039/d0ma00184h |
|
This journal is © The Royal Society of Chemistry 2020 |