A critical review of resource recovery from municipal wastewater treatment plants – market supply potentials, technologies and bottlenecks
Received
10th October 2019
, Accepted 10th January 2020
First published on 13th January 2020
Abstract
In recent decades, academia has elaborated a wide range of technological solutions to recover water, energy, fertiliser and other products from municipal wastewater treatment plants. Drivers for this work range from low resource recovery potential and cost effectiveness, to the high energy demands and large environmental footprints of current treatment-plant designs. However, only a few technologies have been implemented and a shift from wastewater treatment plants towards water resource facilities still seems far away. This critical review aims to inform decision-makers in water management utilities about the vast technical possibilities and market supply potentials, as well as the bottlenecks, related to the design or redesign of a municipal wastewater treatment process from a resource recovery perspective. Information and data have been extracted from literature to provide a holistic overview of this growing research field. First, reviewed data is used to calculate the potential of 11 resources recoverable from municipal wastewater treatment plants to supply national resource consumption. Depending on the resource, the supply potential may vary greatly. Second, resource recovery technologies investigated in academia are reviewed comprehensively and critically. The third section of the review identifies nine non-technical bottlenecks mentioned in literature that have to be overcome to successfully implement these technologies into wastewater treatment process designs. The bottlenecks are related to economics and value chain development, environment and health, and society and policy issues. Considering market potentials, technological innovations, and addressing potential bottlenecks early in the planning and process design phase, may facilitate the design and integration of water resource facilities and contribute to more circular urban water management practices.
Water impact
Are we ready to perceive wastewater as a valuable resource instead of a dirty waste stream? Technologies to recover water, energy, fertilizers and other products from your waste develop rapidly. But water utilities still need to overcome technical, economic, environmental and societal bottlenecks to recover these valuable resources which may have a great potential to make our society more sustainable.
|
Introduction
Although wastewater resource recovery technologies have been extensively elaborated by the scientific community in recent decades, their large-scale implementation in municipal wastewater treatment plants (WWTPs) is still poor. This can primarily be explained by various non-technical reasons, as well as by technical reasons. Wastewater management plays a significant role in sustainable urban development.1 Traditionally, the goal of wastewater treatment was to protect downstream users from health risks. In more recent decades, protecting nature by preventing nutrient pollution in surface waters has become an extra goal. Consequently, nitrogen (N) and phosphorous (P) removal technologies have been implemented into WWTPs.2 The most widely used wastewater treatment technology is the conventional activated sludge (CAS) process, in which aerobic microorganisms metabolise the organic fraction present in the wastewater under constant oxygen supply.3 Although the CAS process succeeds in meeting legal effluent quality standards, it is considered unsustainable due to its low resource recovery potential and cost effectiveness on the one hand, and its high energy demand and large environmental footprint on the other.4
The urge for more sustainable development, including a more circular use of resources, and the resource inefficiency of current wastewater treatment practices have driven a paradigm shift within the scientific community with regard to wastewater solutions. It now proposes a transition from pollutant removal towards resource recovery, with wastewater recognised as a resource rather than a waste stream.5–7 By establishing more circular resource flows, the water sector can contribute to national and European sustainable development goals. As large-scale centralised WWTPs also represent centralised collection points for a variety of resources – namely water, energy, nutrients and other products – their redesign from treatment facilities into water resource factories (WRFs) provides possibilities to contribute to a more circular economy. Within academia, it seems clear that current wastewater treatment practices are based on outdated concepts established in the early 20th century. It seems inevitable that we will have to develop new practices if we are to cope with population growth and improving standards of living, which are pushing our use of natural resources towards limits beyond sustainability.8
Although the rationale and necessity to perceive wastewater as a resource has been emphasised, most water management utilities (WMUs) in Europe still focus on wastewater collection and treatment rather than resource recovery. Despite frequent scientific output over a long period on technological solutions to establish a more circular economy-based water sector, the implementation of full-scale resource recovery technologies in the wastewater sector is still very limited.9 The implementation of resource-oriented processes can be difficult because changing the current wastewater handling system incurs costs, creates operational distractions and consumes resources.8 Due to increasing numbers of available resource recovery technologies, WWTP process design is no longer a simple technical problem, but a complex issue that requires an integrated approach in order to make effective decisions.10 The question which of the growing range of available technical options we should focus on remains open. Uncertainty about which techniques are most useful and how to combine them is standing in the way of creating WRFs.11
In addition to technical uncertainties that are valid for many emerging resource recovery technologies, various non-technological bottlenecks could hinder the successful implementation of such technologies into wastewater treatment processes. In particular, the market potential of and competition against recovered resources introduce uncertainties.12 The water sector has hitherto been poorly equipped to address factors outside its traditional engineering-centred scope. Institutional compartmentalisation within the sector impedes integrated water-resource management and must be remedied in order to make progress in developing resource-oriented wastewater management strategies.5 Consequently, there is a need for WMUs to strategically plan the transition from wastewater treatment towards resource recovery. The transfer of scientific insights to decision-makers in WMUs is an important requirement for this planning process. Resource recovery technologies can only be implemented and potentials can only be exploited if decision-makers at WMUs have a clear understanding of available and emerging technologies.
Previous reviews looking at wastewater resource recovery provide very valuable insights into particular branches of this broad and complex research field. Outstanding examples include the reviews on biological recovery routes,13 energy and product recovery from sewage sludge,14 phosphorous recovery from domestic wastewater,15–17 platforms for energy and nutrient recovery from domestic wastewater,18 bioelectrochemical recovery systems19,20 and nutrient recovery with microalgae-based treatment systems.21 Despite these valuable contributions, as yet there is no review available that provides a holistic overview of the field.
This paper seeks to fill that gap by providing a holistic overview of resource recovery from municipal WWTPs. Data to calculate the potential of 11 resources recoverable in municipal WWTPs to supply markets in the Netherlands and Flanders (Belgium) was derived from a literature review. Resource recovery technologies investigated in academia were then comprehensively and critically reviewed. Finally, bottlenecks discussed in the reviewed literature that have to be overcome to successfully implement these technologies into WWTPs were categorised and analysed. By covering the market supply potential, the vast technical possibilities and the bottlenecks, this paper can inform innovators and decision-makers at WMUs holistically about wastewater resource recovery. Although the effective treatment of wastewater for safe and environmentally friendly discharge will remain the primary objective in WWTP design, it is time to improve these plants' sustainability performance by integrating innovative resource recovery technologies into treatment-process designs.22
Market supply potentials of recovered resources
The market supply potential of resources recovered from municipal wastewater is shown in Table 1. It indicates what role municipal WWTPs could potentially play in a circular economy if resource recovery routes (RRRs) were implemented nationwide. The supply potential for each resource is calculated on the one hand from the quantities that could be recovered from municipal wastewater under ideal circumstances and using the right technologies, and on other from the demand for those resources in the country. The calculations are based on the situation in the Netherlands. Data to calculate the supply potential was collected from scientific articles and from official institutional reports. For the calculation of the nutrient supply potential, data collected in Flanders (Belgium) was used. The reason for choosing this source23 is that it provides a very thorough, complete and up-to-date quantitative analysis of N and P flows within Flanders. No comparable analysis for the Netherlands is available. We assume, however, that N and P flows in Flanders are comparable with those in the Netherlands and so the calculated supply potentials for Flanders are also applicable there.
Table 1 Calculated market supply potentials of water, energy, fertilizer and other products recoverable from municipal WWTPs in the Netherlands or Flanders
Resource demand
|
Potential resource recovery from WWTPs
|
Market supply potential %
|
Water removed from any freshwater source in 2014, either permanently or temporarily; mine water and drainage water as well as water abstractions from precipitation are included.24
Influent into Dutch WWTPs per year = 1928 million m3;25 water content in wastewater = 99%.26
Water recovery efficiency: microfiltration–ultrafiltration unit = 85%.4
Water recovery efficiency: microfiltration–ultrafiltration unit = 85%, reverse osmosis unit = 75%.4
Natural gas gross consumption 2017.199
CH4 recoverable from wastewater per year in the Netherlands by anaerobic COD digestion under ideal conditions: all COD enters anaerobic digester and is recovered at a rate of 80%.27
Supply, transformation and consumption of electricity available for final consumption in 2016.28
CHP electricity conversion efficiency = 38%.4
Theoretical energy in sludge organic matter in NL = 4100 TJ a−1; energy required to evaporate the water content of the sludge = 2900 TJ a−1; actual potential energy of sludge incineration NL = 1200 TJ a−1;27 electrical efficiency of coal-fired power plant = 40%.29
Supply, transformation and consumption of heat energy available for final consumption and derived from gas, coal or biomass combustion in 2016.30
CHP heat conversion efficiency = 40%.4
Total recoverable heat energy from effluent by heat pumps in the Netherlands, assuming ΔT = 5 °C and operation time = 100%.25
Represents the total anthropogenic N fertiliser input in Flanders (organic waste, manure, processed manure, synthetic fertiliser) and excludes atmospheric N fixation from legumes.23
Calculated based on,23 N fluxes into WWTPs assuming that influent N could be fully recovered.
Assumed fraction of influent N ending up in sludge = 20%.31,32
N removal efficiency from sludge applying the biodrying concept = 70%.33
N produced with Haber–Bosch process.23
Calculated based on,23 N fluxes into WWTPs assuming that influent N could be fully recovered.
Assumed fraction of influent N ending up in sludge = 20%.31,32
N removal efficiency from sludge applying the biodrying concept = 70%.33
Represents the total anthropogenic P fertiliser input in Flanders (organic waste, manure, processed manure, synthetic fertiliser).23
Calculated based on,23 P fluxes into WWTPs assuming that influent P could be fully recovered.
Influent P recovery rate as struvite = 35%.34
Influent P ending up in activated sludge = 90%.34
Influent P ending up in activated sludge = 90%; P recoverable from sludge with wet chemical technologies = 90%.34
Ref. 23.
Calculated based on,23 P fluxes into WWTPs assuming that influent P could be fully recovered.
Influent P recovery rate as struvite = 35%.34
Influent P ending up in activated sludge = 90%.34
Influent P ending up in activated sludge = 90%; P recoverable from sludge with wet chemical technologies = 90%.34
Comprises the sum of graphic papers, sanitary and household papers, packaging materials and other paper and paperboard; excludes manufactured paper products such as boxes, cartons, books and magazines.35
Ref. 36; assuming the full influent cellulose fraction is sieved out.37
Total COD into Dutch WWTPs per year = 946 000 t;27 cellulose fraction in influent COD = 31%;38 biodegradability of cellulose in separated anaerobic digester = 100%;39 share of COD load anaerobically converted into biogas = 80%;40 CH4 content of biogas = 65%.27
CHP electricity conversion efficiency = 38%.4
Total cellulose entering Dutch WWTPs per year = 180 000 t;36 heating value of pellets = 13.8 MJ kg−1; combustion energy conversion efficiency to electricity = 29%.38
CHP heat conversion efficiency = 40%.4
Total cellulose entering Dutch WWTPs per year = 180 000 t;36 heating value of pellets = 13.8 MJ kg−1; combustion energy conversion efficiency to heat = 50%.38
Global VFA market sizes.41
Total COD in Dutch influent = 946 000 t;27 influent COD up-concentrated = 75% (bioflocculation HL-MBR); VFA yield per COD in optimised alkaline fermentation = 33%; acetate fraction in VFA fermentation broth = 60.5%; propionate fraction in VFA fermentation broth = 27.5%; butyrate fraction in VFA fermentation broth = 12.5%.42
Total COD in Dutch influent = 946 000 t;27 influent COD up-concentrated = 75% (bioflocculation HL-MBR); VFA yield per COD in optimised alkaline fermentation = 33%; acetate fraction in VFA fermentation broth = 60.5%; propionate fraction in VFA fermentation broth = 27.5%; butyrate fraction in VFA fermentation broth = 12.5%.42
Total COD in Dutch influent = 946 000 t;27 influent COD up-concentrated = 75% (bioflocculation HL-MBR); VFA yield per COD in optimised alkaline fermentation = 33%; acetate fraction in VFA fermentation broth = 60.5%; propionate fraction in VFA fermentation broth = 27.5%; butyrate fraction in VFA fermentation broth = 12.5%.42
Global conventional alginate production.43
EPS recovery: total COD into Dutch WWTPs per year = 946 000 t;27 sludge yield per COD = 40%;44 EPS content in granular sludge = 17.5%;45 assumed EPS downstream process yield = 100%.
Total N in fodder consumed in Flanders.23
Calculated based on,23 P fluxes into WWTPs assuming that influent N could be fully recovered.
Assumed fraction of influent N ending up in sludge (sludge N) = 20%;31,32 assumed fraction of sludge N that is solubilised in the liquor after anaerobic sludge digestion = 100%; assumed N conversion efficiency into protein = 100%.32
CO2 contained in biogas recovered in Dutch WWTPs in the year 2012.46
CO2 contained in biogas recovered in Dutch WWTPs in the year 2012.46
|
Water demand
|
Netherlands
|
Water recovery
|
Netherlands
|
Water
|
Water abstractiona |
9482m m3 a−1 |
Effluentsb |
1909m m3 a−1 |
20 |
Treated by MF-UFc |
1622m m3 a−1 |
17 |
Treated by MF-UF/ROd |
1217m m3 a−1 |
13 |
|
Energy demand
|
Netherlands
|
Energy recovery
|
Netherlands
|
Energy
|
Natural gase |
1227 PJ a−1 |
CH4 from COD (anaerobic)f |
9 PJ a−1 |
1 |
Electricityg |
379 PJ a−1 |
Electricity CH4 (CHP)h |
4 PJ a−1 |
1 |
Electricity sludge co-combustioni |
0.5 PJ a−1 |
0.1 |
Derived heatj |
88 PJ a−1 |
Heat CH4 (CHP)k |
4 PJ a−1 |
4 |
Heat (effluent)l |
40 PJ a−1 |
46 |
|
N demand
|
Flanders
|
N recovery
|
Flanders
|
N
|
N applied to cropsm |
169 kt N a−1 |
Influent Nn |
24 kt N a−1 |
14 |
N in activated sludgeo |
5 kt N a−1 |
2.9 |
Sludge N recoverable (biodrying)p |
3 kt N a−1 |
2 |
Industrial N fixationq |
574 kt N a−1 |
Influent Nr |
24 kt N a−1 |
4 |
N in activated sludges |
5 kt N a−1 |
0.8 |
Sludge N recoverable (biodrying)t |
3 kt N a−1 |
1 |
|
P demand
|
Flanders
|
P recovery
|
Flanders
|
P
|
P applied to cropsu |
24 kt P a−1 |
Influent Pv |
3.3 kt P a−1 |
14 |
P recovery as struvitew |
1.2 kt P a−1 |
5 |
P in activated sludgex |
3.0 kt P a−1 |
13 |
Sludge P recoverable (wet chemical technology)y |
2.7 kt P a−1 |
11 |
Imported mined Pz |
44 kt P a−1 |
Influent Paa |
3.3 kt P a−1 |
8 |
P recovery as struviteab |
1.2 kt P a−1 |
3 |
P in activated sludgeac |
3.0 kt P a−1 |
7 |
Sludge P recoverable (wet chemical technology)ad |
2.7 kt P a−1 |
6 |
|
Cellulose demand
|
Netherlands
|
Cellulose recovery
|
Netherlands
|
Cellulose
|
Paper (production)ae |
2671 kt a−1 |
Cellulose in influentaf |
180 kt a−1 |
7 |
|
Energy demand (see above) |
Netherlands
|
Cellulose to energy
|
Netherlands
|
|
CH4 from cellulose (anaerobic)ag |
1.9 PJ a−1 |
0.2 |
Electricity CH4 (CHP)ah |
0.7 PJ a−1 |
0.2 |
Electricity (cellulose combustion)ai |
0.7 PJ a−1 |
0.2 |
Heat CH4 (CHP)aj |
88 PJ a−1 |
1 |
Heat (cellulose combustion)ak |
1.2 PJ a−1 |
1 |
|
VFA demand
|
Global
|
VFA recovery
|
Netherlands
|
VFA
|
Acetateal |
16 000 kt a−1 |
Acetate recoveryam |
142 kt a−1 |
1 |
Propionateal |
380 kt a−1 |
Propionate recoveryan |
64 kt a−1 |
17 |
Butyrateal |
500 kt a−1 |
Butyrate recoveryao |
29 kt a−1 |
6 |
|
Alginate demand
|
Global
|
EPS recovery
|
Netherlands
|
EPS
|
Productionap |
30 kt a−1 |
Potential EPS productionaq |
76 kt a−1 |
252 |
|
Fodder demand
|
Flanders
|
SCP recovery
|
Flanders
|
SCP
|
Fodder N consumedar |
149 kt a−1 |
Influent Nas |
24 kt a−1 |
16 |
SCP from anaerobic digestateat |
4.8 kt a−1 |
3 |
|
CO2 demand
|
Netherlands
|
CO2 recovery
|
Netherlands
|
CO2
|
Industrial CO2au |
1239 kt a−1 |
CO2 from biogas in WWTPsav |
53 kt a−1 |
4 |
Water supply potential
Water reuse from municipal WWTPs can significantly reduce a city's freshwater demand.2 A well-studied success story of water reclamation and reuse is the city of Windhoek (Namibia), where 25% of the city's potable water supply stems from wastewater.4 Other urban examples include the city of Chennai (India), where the reuse of 40% of the generated wastewater satisfies 15% of the city's water demand.47 At Xi'an University in China, a decentralised treatment system produces water for various non-potable uses, such as toilet flushing, gardening and waterfront landscaping, and has cut freshwater consumption on the campus by 50%.48 In the water-scarce city of Monterey (California, USA), a large agricultural area is supplied with almost 80
000 m3 per day of nutrient-rich reclaimed municipal wastewater to irrigate and fertilise crops.40 At the state level, Israel and Singapore are two examples of countries with nationwide wastewater reuse schemes. In Israel, almost a quarter of the country's water demand is met by reclaimed wastewater,48 while Singapore achieves 40% with its NEWater reclamation plant.49
However, wastewater entering a municipal WWTP contains only water used domestically, fractions of industrial water and storm water. Water used in the agricultural sector, which is the second largest consumer of water in Western countries, after industry,50 does not reach these plants. Therefore, even if a large fraction of WWTP influent is reclaimed, it can only partly satisfy total regional demand for fresh water. As shown in the examples in Table 1, the effluents discharged by Dutch WWTPs equate to 20% of the total volume of fresh water abstracted in the Netherlands. Although the application of filtration technologies to these effluents implies water losses, advanced treatments could produce different water qualities suitable for various reuse purposes, depending on the process applied. Microfiltration and ultrafiltration could reduce Dutch freshwater abstraction by 17%, while reverse osmosis could reduce it by 13%. Only the latter technology could reclaim water of high enough quality to enter the potable supply, so the others would only be useful if the reclaimed water was intended to be used in a non-potable context.
Energy supply potential
A municipal WWTP can provide a significant share of the total energy consumption of its operating local authority.51 On the other hand, the potential chemical energy held in typical municipal wastewater has been measured as being five times higher than that needed for CAS process operations.44 As shown in Table 1, 94 petajoules (PJ) per year is the theoretical maximum energy that could be recovered from Dutch WWTPs as CH4, assuming that all the chemical oxygen demand (COD) in the influent were to enter an anaerobic digester to be converted into biogas at 80% efficiency. Currently, only about 25% of this maximum potential is exploited.27
Even under ideal conditions, however, CH4 recovered from wastewater would substitute less than 1% of Dutch annual natural gas consumption. If the recovered CH4 were converted into electricity and heat in a combined heat and power (CHP) unit of typical efficiency (ca. 40%), less than 1% of the Dutch electricity consumption and only 4% of the derived heat currently used in the Netherlands could be supplied. Assuming that all excess sludge were dewatered and then co-combusted in coal-fired power plants, the amount of electricity obtained would be a negligible 0.1% of overall consumption. The main reason for the low energy-recovery potential of sludge incineration is that a considerable amount of energy is required to evaporate its water content, as sludge is often 80% water even after mechanical dewatering.27
The total thermal energy contained in WWTP effluent by far exceeds the on-site demand for heat, indicating that these plants have huge potential to feed district heating networks or provide heat for industrial purposes.52 With a view to process optimisation, using this heat for sludge drying is also a promising possibility. The yearly average effluent temperature in Dutch WWTPs is 15 °C. Assuming that a heat-exchange or heat-pump system were installed to recover heat energy of 5 °C, 24 hours a day, 365 days a year, the total recoverable heat from municipal WWTP effluents in the Netherlands would be about 40 PJ.25 This equates with more than 40% of the total heat energy derived from gas, coal or biomass combustion processes. Moreover, heat recovered from Dutch WWTP effluents has an energy recovery potential approximately ten times higher than that of heat derived from recovered CH4 combustion in a CHP unit (see Table 1).
Fertilizer supply potential
Close to 100% of the phosphorous (P) eaten in food is excreted by the human body. On a global scale, about 17% of all mined mineral P ends up in human excreta. Cities are P ‘hotspots’ and urine is the largest single source of the P emerging from them.53Table 1 shows that in the Flanders region (Belgium), for example, the total P entering WWTPs is equal to 8% of Flemish industrial P ore imports and 14% of the total fertiliser orthophosphate P used in the region. Since P could be recovered from sludge incineration ash with efficiencies of about 90%,34 this recovery pathway would lead to a realistic supply potential of 11% of Flemish fertiliser demand or 6% of Flemish industrial P ore imports. By contrast, if soluble P is recovered as struvite, the influent P recovery percentage lies between 10 and 50% depending on the treatment process applied.34,54 The supply potential of the struvite recovery route is thus significantly lower (3%) than that of the sludge recovery route.
Thirty per cent of global N fertiliser demand could be met through wastewater N recovery practices. But in countries with intensive agriculture systems, like the Netherlands, this figure shrinks to just 18%, representing the fraction of fertiliser N that enters WWTPs.55 As shown in Table 1, much the same applies in Flanders, where 14% of total N fertiliser demand or 4% of that for industrially fixed N could theoretically be met from wastewater N recovery practices (assuming a 100% recovery rate of influent N concentrations). But since only 20% of influent N is retained in the sludge after the CAS process, recovery rates using the technologies currently available are significantly lower.31,32 The biodrying concept, for example, which converts sludge into an energetically favourable state and simultaneously recovers ammonium sulphate,33 could satisfy only 2% of total Flemish demand for N fertiliser or less than 1% of that for industrially fixed N.
Supply potential of other products
As exemplified for the Dutch case, in addition to fertilizers, multiple products – for example, cellulose, volatile fatty acids (VFAs), extracellular polymeric substances (EPS), single-cell protein (SCP) and CO2 – can be recovered from wastewater. In principle, more products can be recovered from wastewater, but data on such routes is still limited, which gives rise to uncertainties. The Dutch Foundation for Applied Water Research (STOWA), the Joint Scientific Centre of the Dutch water boards, is currently developing wastewater resource recovery strategies focusing on five of the products mentioned above, namely cellulose, EPS, VFA, PHA and CO2.56
Cellulose fibres may represent 50% of the total suspended solids and a significant fraction of the inert solid fraction in municipal WWTP influents. In the Netherlands, more than 80% of consumed toilet paper ends up in WWTPs and could be recovered by taking a real cradle-to-cradle approach – although it does remain questionable whether customers would accept recycled toilet paper.39 As shown in Table 1, if the cellulose fibres were used as raw material for the Dutch paper and paper board industry, they would have the potential to satisfy 7% of demand from this sector. In all, 180
000 t of toilet paper are flushed down Dutch toilets every year. As this represents approximately 180
000 trees,36 annual deforestation of 45 ha could be avoided by recycling toilet paper, assuming that the normal density of Dutch forests is 4000 trees per ha.57 Using sieved cellulose as feedstock for a separated anaerobic digestion unit, as tested by,39 would only produce quantities of CH4, electricity and heat equivalent to less than 1% of total societal demand. Not surprisingly, a similarly low energy-supply potential is expectable were the fibres to be dried, pressed into energy pellets and combusted for electricity and heat generation, as investigated by.38
VFAs produced in the Netherlands from up-concentrated COD combined with long sludge retention times could, depending on the VFA type, meet 1–17% of global market demand. But published figures on the global production volumes of the three main VFAs differ considerably,41,58–60 which makes this estimate uncertain. Country-specific market data about VFAs is not readily available for academic use, the only source being commercial market analysts selling reports for several thousand euros each.41 If COD-derived VFAs were converted into PHA, it is likely that a significant share of European PHA production could be supplied by the combined Dutch WWTPs. However, estimates of annual PHA market sizes vary greatly from almost 150
000 tonnes European market size61 to 100
000 tonnes global market size,62 which makes it difficult to estimate a reliable supply potential.
If Dutch influents were invariably treated using aerobic granular sludge processes, and assuming that EPS can be substituted for alginate due to their similar material properties, the potential supply of EPS recovered from Dutch municipal WWTPs would exceed global alginate production by a factor of around 2.5. If such a scenario were realised, it would certainly have a severe impact on the global alginate market, including prices.
Intensive livestock production relies on protein-rich fodder. If all Flemish influent N could be converted into protein fed to animals, 16% of the consumption of conventional fodder N stemming from protein-rich plants like soya beans could be avoided. The production of single-cell protein from wastewater as proposed by ref. 32 could be much more environmentally efficient than the production of conventional fodder. Its potential to satisfy Flemish demand for fodder, however, is rather limited: it could substitute only 3% of conventional fodder N because only the sludge N fraction is converted; most of the influent N remains in the water line as ammonium or is denitrified.
Upgrading recovered biogas by extracting a rather pure CO2 stream could contribute substantially towards achieving the greenhouse-gas emission-reduction target of the Dutch water boards. It could also satisfy some industrial CO2 consumption needs (4%) – although this should still be considered an important potential contribution, because the energy demand of CO2 from biogas is around 80% lower than that from conventional processes.46
Resource recovery technologies
By reusing resources contained in municipal wastewater, we could tackle water scarcity problems, lower fossil energy consumption and address global nutrient needs. In addition to water, energy and nutrient recovery, it should not be forgotten that a variety of other products can be recovered from wastewater.6 This section critically discusses RRRs for these four resource categories. We define an RRR as the route taken by a resource entering a WWTP, extracted from the flow and then refined before finally being used. While resource extraction happens on site at the WWTP, refining and usage can be undertaken elsewhere.
Water reclamation and reuse technologies
Around 99 wt% of the matter contained in wastewater is water,26 so reclaiming and reusing this could be a more sustainable option than, for example, desalination or long-distance fresh-water transfers.63 Furthermore, the main driver for the reclamation and reuse of domestic wastewater is water scarcity caused by generally uneven global fresh-water distribution and climate change-related water stress.48 Secondary wastewater treatment processes do not fully remove biological oxygen demand (BOD) and only eliminate 95% of total suspended solids (TSS) from effluents, which also contain residual concentrations of organic micropollutants, such as pharmaceuticals, polychlorinated biphenyls (PCPs) and pesticides. To meet the strict legal standards for microbe and micropollutant concentrations in reclaimed water, the effluent from secondary wastewater treatment processes needs to be further processed on advanced treatment lines.64 Advanced treatment technologies can be divided into filtration, disinfection and advanced oxidation processes (Fig. 1).
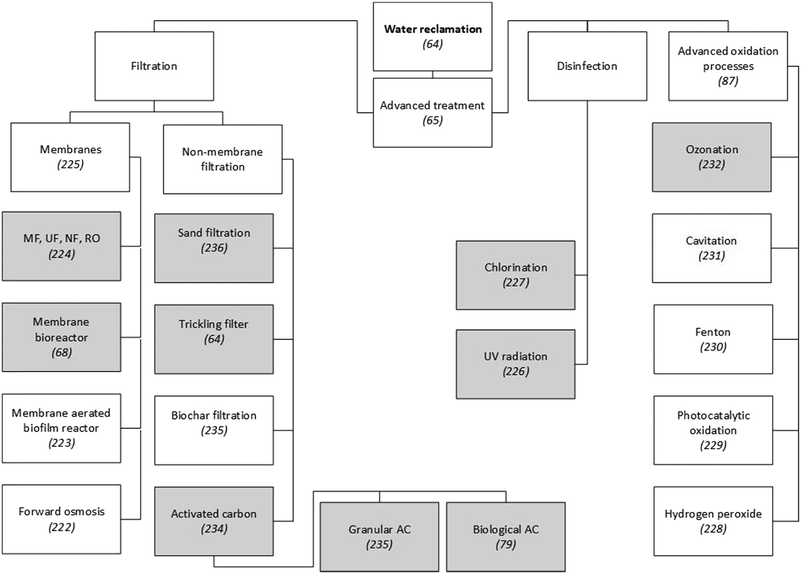 |
| Fig. 1 Examples of technologies to reclaim water from municipal WWTPs. Since a detailed presentation and discussion of these technologies is beyond the scope of this paper, scientific publications that explain or review them are referenced. Grey shading indicates techniques that have been applied on a large scale in municipal WWTPs. Unshaded boxes show technologies that are not widely applied.64,65,68,79,87,222–232,234–236 | |
Membrane filtration
Membrane processes allow reliable advanced treatment and are considered a key technology for advanced wastewater reclamation and reuse strategies. Their advantages include the need for less space, being a physical barrier against particle material, and efficiency at retaining microorganisms without causing resistance or by-product formation. Membranes are included in several prominent large-scale advanced treatment designs used worldwide for artificial groundwater recharge, indirect potable reuse or industrial process-water production. Ultrafiltration membranes (UF) remove colloids, proteins, polysaccharides, most bacteria and even some viruses, and produce high-quality treated effluents.65 Techniques using membranes with smaller pore sizes – namely nanofiltration (NF) and reverse osmosis (RO) – are useful to separate ions and dissolved solids from water.66 A successful example of the use of NF/RO membrane technology to recover water from wastewater for indirect potable reuse can be found in Singapore, as part of the NEWater project. The process consists of several treatment steps and generates significant amounts of reclaimed water to refill natural drinking-water reservoirs in the city state.67
Membrane bioreactors (MBRs) might be especially useful for wastewater reuse applications because they include an initial membrane filtration step. A pilot application within the NEWater project, using MBR/RO/UV after primary sedimentation, successfully recovered water of potable quality.67 MBRs combine the activated sludge process with microporous membranes for solid–liquid separation and have been frequently applied, on a large scale, for municipal wastewater treatment.68 Possible advantageous features of MBRs are the separate control of sludge and hydraulic retention times, and higher mixed liquor-suspended solids concentrations, which allow for smaller reactors. On the other hand, MBRs can also have several disadvantages compared with the CAS process; for example, greater process complexity, less readily dewaterable sludge and greater sensitivity to shock loads. In addition, MBRs are associated with higher equipment and operational costs, due mainly to membrane cleaning and, at high loading rates, higher aeration requirements.69
Although membrane technologies can provide very high quality effluent, useful for any type of water reuse, they are costly in operation. Membrane fouling in wastewater applications can be a significant problem, too, especially at high fluxes. Applying low fluxes reduces operational costs but increases capital costs, as more membrane units are necessary.70 To decrease potential fouling and clogging, effective operation requires extensive pre-treatment of secondary effluents.66 An additional cost factor for efficient large-scale membrane-technology application for wastewater reuse arises from disposing of the complex retentate.71 Moreover, high pressure is generally needed for membrane filtration. The energy requirements for MF/RO systems are approximately 3 kWh per m3 (ref. 18) and may far exceed the recoverable chemical energy in the wastewater.72 calculated a total lifecycle cost of about US$0.3 per m3 for water reclaimed by an UF/RO treatment.2 Estimated an overall cost of approximately €0.8 per m3 for the CAS process followed by UF/RO, including costs for retentate discharge and revenues from water valorisation. Reclaiming potable water for households and/or industries from wastewater was shown to be cost ineffective for the Amsterdam region due to high process costs by comparison with conventional options.12 Membrane-based filtration processes always require considerable electricity input,18 although lower water viscosity in warm climates may decrease these energy requirements. In our resource-constrained world, however, increasing the consumption of one resource in order to make another available has to be considered very carefully.73
Activated carbon filtration
Activated carbon (AC) filtration as an advanced treatment process can produce higher quality effluent that is useful for water reuse. AC units can be made from various raw materials, including coal, peat, petroleum coke and nutshells. These carbonaceous substances are activated by physical and/or chemical agents under high temperatures, endowing them with effective filtering capacity for COD, total organic carbon (TOC), chlorine and a wide range of hydrophobic organic pollutants like pharmaceuticals.74 Two major driving forces cause the adsorption of solubilised pollutants to the surface of AC filters: (i) the solubility of the dissolved pollutant and (ii) the affinity of the contaminant for the adsorbent. AC is applied as a powder (PAC) with a grain diameter of less than 0.07 mm or as granular activated carbon (GAC). PAC can be added directly to the activated sludge unit prior to advanced filtration steps, whereas GAC is used in a separate pressure- or gravity-driven filtration unit. While PAC needs to be disposed of after use together with the sludge, GAC can be regenerated cost effectively on site.75
Various studies have shown the effectiveness of combining AC filtration with other advanced treatment steps for the removal of water pollutants.76 showed that AC coupled with oxidation by ozone removes 90% of various types of pesticides during the production of drinking water. AC in combination with ozonation improves the removal/degradation of various emerging pollutants, since AC can function as a catalyst in the ozonation reaction while ozone increases the pore size and active surface area of AC.77–79 Furthermore, if AC is applied upstream of membrane filtration units, the filtration performance of the membrane systems is significantly improved.80–82 But, compared with other alternatives, the cost effectiveness of AC as a membrane pre-treatment step may be questionable. Possible shortcomings of AC filtration are that compounds of low molecular weight and high polarity – such as amines, nitrosamines, glycols and certain ethers – are not adsorbed.83 In addition, contaminants are transported from the water to the filter but are not degraded, so subsequent filter disposal or cleaning has to be considered as an additional cost.84
Advanced oxidation processes
The removal of emerging pollutants like pharmaceuticals is a growing concern in wastewater treatment50 and certainly needs to be considered in water-reclamation processes. Advanced oxidation processes (AOPs) form hydroxyl radicals (˙OH) as highly reactive oxidant agents for the destruction of a wide range of non-biodegradable organic contaminants like pharmaceuticals, dyes or pesticides, as well as bacteria, protozoa and viruses. AOPs are often run by external energy sources such as electric power or light. They are usually applied as the final polishing and disinfection step after biological treatment, but can also be used as a pre-treatment step that breaks down organic contaminants to enhance subsequent biological treatment measures.85 AOP systems can be configured according to the contaminant composition and concentration and the required effluent quality. Besides the sequential application of various AOPs to enhance the selectivity of several classes of different pollutants, the combined application of single AOPs can significantly enhance the oxidation rate of organics.86 Various publications provide a thorough overview of the vast range of possible combinations of AOPs to treat recalcitrant pollutants in industrial or municipal wastewater.84,85,87,88 But the application of AOPs may also have shortcomings, like high costs for reagents such as ozone and hydrogen peroxide or for the required energy source, such as ultraviolet light.89 The following paragraphs briefly describe ozone and ultraviolet irradiation, the most widely used AOP techniques. Unless membrane treatment in the form of RO is already applied, an additional disinfection unit may be needed for safe wastewater reuse.
Ozone (O3) is a commonly used oxidising agent, often produced on site from dry air or pure oxygen. It is useful for the elimination of bacteria, viruses and protozoa and therefore a suitable process for water reuse. While higher pressure, pH value and contact time enhance pollutant degradation efficiency, a higher temperature limits it. The main disadvantages of ozonation are its high energy demand and the short stability of ozone itself, which can make the process costly. For water that contains certain levels of bromide, there is a potential risk of its conversion to bromate during ozonation, which can lead to the formation of carcinogenic bromated organic compounds. This is especially relevant in seawater desalination and drinking-water treatment, and to a lesser extent in wastewater effluent polishing. After ozonation, activated carbon filtration is often applied to reduce the content of biodegradable compounds in the flow.74
Ultraviolet (UV) irradiation is considered a fast, efficient, safe and cost-effective process, and is thus one of the most prominent alternatives to chemical disinfection.90 UV light wavelengths hold enough energy to let pollutant molecules release electrons and therefore become unstable. In addition to this direct photolytic action on compounds dissolved in the water, UV technology may degrade other contaminants through the photochemically-assisted production of oxidants like hydroxyl radicals and through photochemically-assisted catalytic processes.91 Microorganisms have evolved mechanisms to repair their partially denatured DNA after UV light exposure, however, which can lead to DNA reactivation after the treatment. This potential risk is dependent on the UV dose applied, the stability of added disinfectants, contact time, pH, temperature and the number and type of microorganisms present in the wastewater. Moreover, the physiochemical parameters of the treated effluent, such as turbidity, hardness, suspended solids, iron, manganese and humic acids content, can be disruptive factors preventing UV light waves from reaching all microorganisms.90 After treating advanced municipal wastewater effluent with UV light,92 concluded that microbial communities change after the treatment in respect of the types of bacteria present, but that the total amounts of bacteria in the water can increase to the same level as in non-disinfected effluent within only five days. UV irradiation therefore requires careful adjustment of the factors just described in order to ensure sufficient contaminant removal from wastewater.92
To eliminate bacteria, viruses and protozoa for safe water reuse, chlorination is the most widely applied method. Chlorine is applied around the world for wastewater disinfection, as chlorine gas, hypochlorite solution or in solid form.74 Despite its effectiveness in destroying pathogens, chlorination is accompanied by potential risks. Harmless substances can react with the disinfectant and form harmful molecules, so-called chlorination by-products.93 In addition, research has shown that some viruses and bacteria are resistant to chlorination. It is therefore advisable to combine this technique with additional and advanced treatment methods for safe water reclamation.94 Typical chlorine doses are 5–20 mg l−1 for a contact time of 30–60 min. If residual chlorine concentrations in the reclaimed water are too high for its intended reuse type, a dechlorination step is required. This can increase the cost of chlorination by about 20–30%.95
Summary: water reclamation and reuse
Successful wastewater reclamation and reuse is hindered not only by technology-related bottlenecks but also by more general ones. Taken together, these indicate that such reuse might be a valid option only in water-constrained regions, like Singapore, or in delta zones where salt water is abundant but fresh water is not. One of the general bottlenecks is that potential users might be scattered across the city, requiring a dedicated distribution network. Since water reuse is rather a new concept in urban planning, current infrastructure seldom takes the distribution of reclaimed water into account. Consequently, there is little room to install a new separate pipeline network, whilst retrofitting is costly, impractical and inconvenient.96
Beyond that, water reuse including a new distribution network may have a greater lifecycle impact than surface-water treatment and distribution via the conventional pipeline system. But if non-potable water qualities are produced, new distribution lines – and hence increased costs – are inevitable.97 In Tokyo's Shinjuku district, a second pipeline system has been successfully installed to flush toilets with reclaimed wastewater. Due to the high density of high-rise buildings in this area, the pipes are mostly above ground in the buildings themselves. Compared with an underground network, this has kept costs relatively low.98 In cities that withdraw their water from aquifers or natural bodies of water, the recharge of those sources with reclaimed water (indirect reuse) might be the preferred option due to its much easier practicalities and lower costs, compared with building new distribution systems to reach end users. The Catalan Water Agency, for example, promotes aquifer recharge to prevent water scarcity during periods of drought but also to refill the aquifer as a hydraulic barrier against saltwater intrusion. A similar approach is implemented at the Torreele facility in Belgium.99 Ideally, potential large-scale water users like industries or farms should be located close to the WWTP so that they can be supplied through a single pipeline in order to keep distribution costs low.48 In practice, however, the topographical location of WWTPs is usually down-gradient so as to make use of gravity for wastewater flow. This can make the distribution of reclaimed water costlier, because it needs to be pumped uphill back to the city or other areas of usage.40 In addition, the temporal variability in the demand for and supply of reused wastewater is an important issue to consider in distribution planning.97
Another reported bottleneck in wastewater reclamation is health concerns, especially if the water produced is destined for direct or indirect potable reuse. When the water board in Amsterdam, the Netherlands, analysed and assessed potential alternative fresh-water sources, potable water reuse was evaluated as being too risky. Since enough fresh water is already available in Amsterdam anyway, other alternatives were chosen.100 However, the importance of social acceptance is illustrated by a case from San Diego, California, where 90% of the local water supply stems from sources several hundred kilometres away. A wastewater reclamation technology implemented there eventually had to be scrapped due to public safety concerns. Similar cases are reported from Toowoomba, Australia, and the Californian cities of San Ramon–Dublin and Los Angeles.5 When it comes to wastewater reclamation and reuse, it is widely agreed that without public acceptance, it is difficult for any water management utility (WMU) to finance, construct and operate adequate processes to prevent future supply shortages during periods of drought. Social acceptance therefore needs to be perceived as a potential problem at an early stage in water reuse project planning. Public participation is essential to meet people's needs, to collect local knowledge so as to help improve the design of the project and to build vital institutional trust.97 On the other hand, if citizens have experience of immediate and severe water shortages, their acceptance of such schemes increases even when these involve direct potable reuse. This has been the case, for example, with the system in place for almost 40 years now in Windhoek, Namibia.26 If shortages are not perceived as a threat, the willingness to pay for water services is low and that makes it difficult to implement reuse schemes that are cost effective.22
The use of reclaimed water for the irrigation of crops also entails risks, including the uptake by plants of sodium and other ions that can lead to yield losses, alter soil structures, change water infiltration rates and contaminate soils.101 Various cases have shown the significant contribution that reclaimed water can make to more sustainable agricultural production.98 describe a variety of successful reuse projects undertaken in cooperation with the agricultural sector. However, a lack of common legal standards and policies is a serious bottleneck obstructing the wider implementation of water reuse projects in Europe, because this lack increases planning and investment uncertainties.102 Government policies to make water reuse an attractive business venture for financial service providers and investors are also needed in other parts of the world, such as China.96 In this context, it is commendable that the European Commission established the European Innovation Partnership (EIP) for Water and identified wastewater reclamation and reuse as one of its top five priorities. In 2018, the Commission published an initial proposal for a regulation on minimum requirements for water reuse. Its general objective is to increase the uptake of this solution for agricultural irrigation wherever it is relevant and cost effective.63
Energy recovery technologies
Global energy demand is expected to grow by approximately 50% between 2010 and 2040, and fossil fuels will likely satisfy almost 80% of this. Consequently, fossil-related emissions are projected to increase by a similar amount.103 These projections drive the need to substantially decrease the energy intensity of WWTPs by designing treatment processes with a focus on energy efficiency and recovery. The treatment of municipal wastewater currently accounts for about 4% of the national electricity consumption in both the United States104 and the United Kingdom.3 As shown in Fig. 2, the recovery of fuels from wastewater is achievable through the application of different technologies. The chemical energy in typical municipal wastewater is 17.8 kJ g−1 COD.105 This is about five times the electrical energy needed to operate the conventional activated sludge (CAS) process,44 although in the latter process a significant fraction of the energy stored in the COD is lost as heat during microbial metabolism.27 Its current configuration hardly achieves energy self-sufficiency, which is usually in the range of 30–50%,44 depending on the country concerned.
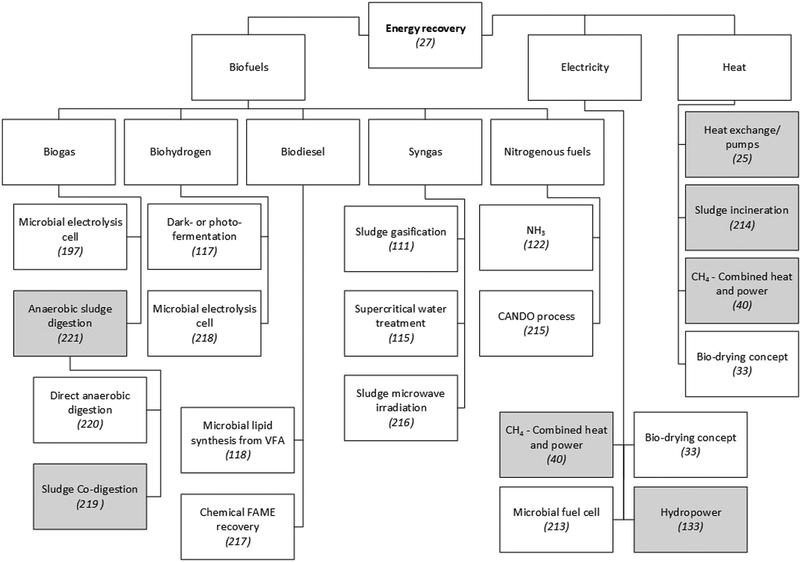 |
| Fig. 2 Examples of technologies to recover energy from municipal WWTPs. Since a detailed presentation and discussion of these technologies is beyond the scope of this paper, scientific publications that explain or review them are referenced. Grey shading indicates techniques that have been applied on a large scale in municipal WWTPs. Unshaded boxes show technologies that are not widely applied.25,27,33,40,111,115,117,118,122,197,213–221 | |
Methane
The production of biogas by anaerobic sludge digestion is currently the most widely used energy recovery method, and it is applied worldwide on different scales.106 About 80% of the biodegradable COD fraction in the sludge can be converted into harvestable biogas in completely mixed reactors.40 In advanced reactor configurations, biodegradation efficiency and the recovery of dissolved methane from the broth may be improved.107 If the recovered methane is not used on site, it needs to be pressurised and transported to customers. This can be too expensive in countries where CH4 is cheaply available and distributed using a comprehensive pipeline grid.108 One important cost factor of digesters is heating, since at moderate temperatures up to 40% of the produced methane is dissolved in the broth. This dissolved methane might ultimately contribute to climate change. Anaerobic wastewater treatment and sludge digestion therefore need to be properly controlled in order to minimise the risk of methane leakage.27
One promising concept to maximise the recovery of biogas is maximum COD capture at the entrance of the plant, followed by digestion of the primary sludge.27 Up-concentration of COD can be achieved by applying either chemically enhanced primary treatment or high-rate activated sludge as an A stage in a WWTP.44 On average, plants applying this energy-recovery route consume 40% less net energy.27 But using the generated biogas for combined heat and power recovery implies high energy conversion losses of about 60%. Converting 60% of influent COD with anaerobic digestion and CHP generates only approximately half of the energy required for total COD removal as part of a CAS process.44
It is also possible to treat wastewater directly, that is, anaerobically, for example in anaerobic membrane bioreactors (AnMBRs) or up-flow anaerobic sludge blanket (UASB) reactors. These processes may provide low-energy carbon removal, but they also require additional post-treatment steps due to insufficient pathogen removal.18 The organic carbon concentrations in municipal wastewater, however, are too low for direct anaerobic treatment. Consequently, anaerobic digesters are only used in large conventional plants for treatment of the sludge line, not the water line.109
Other biofuels
As well as methane, other fuels can also be recovered from municipal wastewater streams. In conventional biofuel production using sugar, 40–80% of the overall production costs are related to the feedstock alone. Converting wastewater COD into biofuels may therefore offer significant economic potential,110 although downstream processing and the high dilution of recoverable matter remain major challenges.13 However, syngas can be produced by the fast gasification of wet sewage sludge111 – a thermal conversion process that converts any carbonaceous material into, for the most part, carbon monoxide and hydrogen in a controlled oxygen environment, sometimes at high pressures of 15–150 bar.112 If sewage sludge-derived syngas is used as a fuel, it needs to be cleaned as it contains undesirable impurities that may damage fuel cells, engines or turbines.111
Syngas can also be obtained from municipal sewage sludge using supercritical water treatment processes. During supercritical water gasification or partial oxidation processes, the temperature and pressure are raised above the critical point of water (374 °C, 221 bar). In these conditions, biomass is converted into syngas at high rates and energetic efficiencies. In addition to syngas, a disposable clean-water stream and solids (metal oxides, salts) leave the process.113 The advantage over other sludge-handling technologies is that the sludge is converted into an energy carrier in much shorter residence times of only a few minutes. Moreover, excess sludge from WWTPs does not need to be dewatered before being fed to supercritical water reactors.114 Although existing thermodynamic equilibrium models can predict the major product compounds formed in reactors, not all parameters determining the final gas composition are yet clear. One operational challenge is corrosion of the reactors due to harsh operating conditions. Another is salt precipitation and clogging due to the rapid decrease in the solubility of salts in supercritical water conditions.114 Several commercial applications have partially demonstrated the economic feasibility of the process.115 Possible success and failure factors, COD destruction efficiencies and research needs in respect of commercial processes have been reported and reviewed elsewhere.115
Hydrogen can also be recovered from wastewater by biological means, namely in a two-step anaerobic sludge treatment process limited to hydrolysis and acidogenic fermentation by phototropic and/or lithotrophic microorganisms. Photofermentation is frequently employed together with dark fermentation because the latter converts only about one third of the COD into hydrogen and the rest into VFA, which can subsequently be used in photofermentation to enhance overall hydrogen production.116 However, the major bottleneck in fermentative H2 production is the quite low yields.117
Biodiesel is another fuel that can be derived from sludge. Lipids can represent a significant proportion of the organic fraction in municipal wastewater and specialised microorganisms can assimilate and accumulate these anaerobically. Harvesting this lipid-rich biomass by simply skimming the surface of wastewater treatment reactors could provide feedstock for high-yield biodiesel production.118 The use of phototrophic microalgae that treat the wastewater in high-rate ponds is a well-studied production route for biodiesel.119 One major bottleneck, however, is that the performance of phototrophic organisms depends on climatic conditions that are not available all year round in countries that have a winter season.120 In addition, land use for this type of biodiesel production is high,121 as are the costs of photo-bioreactors and algae harvesting.122
Nitrogenous fuels can also be recovered from wastewater. One route for this is the CANDO process, which involves three steps: (i) nitritation of NH4+ to NO2−, (ii) partial anoxic reduction of NO2− to N2O and (iii) chemical N2O conversion to N2 with energy recovery. Another route recovers NH3 directly from concentrated side streams, for example by stripping. NH3 can be burned to generate power or used as a transport fuel. It can even be converted, by nitritation and further abiotic or biological reduction, into N2O for co-combustion with methane recovered by sludge digestion. However, processes that recover ammonia for fuel generally consume more energy than they recover, which makes them economically unfeasible. Another major problem with these routes is the low N concentrations in municipal wastewater. Thus, recovering ammonia as fertiliser instead of as an energy carrier seems preferable.122
Sludge incineration
When sewage sludge is incinerated, complete oxidation of its organic content is achieved, thus forming CO2, water and inert material (ash), all of which have to be disposed of. The ash can be used, for instance, as aggregate for building materials.14 The combustion heat can be recovered as electricity. Raw sewage sludge has a 30–40% higher heating value than digested sludge, which makes it theoretically attractive as a combustion fuel to produce electricity. Whether sludge digestion or incineration is the energetically favourable route, however, depends on specific and local conditions like the treatment system, the methods used for sludge drying and the type of incineration.27 Various plant configurations for the large-scale combustion of biomass, including dried sewage sludge, are applied worldwide and recover energy from the organic matter. Typical electrical efficiencies of stand-alone biomass combustion plants are 25–30%. To be economically viable, such plants rely on low-cost fuels, carbon taxes or fixed tariffs for the electricity they generate. Fluidised bed technology in combustion plants can increase electrical efficiencies to 40%, at lower cost and with higher fuel flexibility. Co-combustion of sludge in coal-fired power plants is another method widely applied in the EU, and it achieves similar efficiencies.29
The major drawback of sludge incineration is the typically high water content of waste sludge. To achieve a positive energy balance from combustion, the water content needs to be reduced to below 30% – which usually requires energy and therefore creates costs.40 The actual energy recovery potential of sludge incineration is much lower than the energy content of the organic matter in the sludge, because a lot of energy is required to evaporate its water content.27 As a solution to this problem, significant heat energy can be recovered from WWTP effluent by heat-exchanger and heat-pump systems.123 To improve the heating value of waste sludge, this low-cost heat can be supplied to dewatering and drying systems in the plant.
Bioelectrochemical systems
In bioelectrochemical systems (BESs), COD is oxidised by microorganisms and the electrons generated during this process are then used to produce energy or other valuable compounds.20 Within these systems, microbial electrosynthetic processes can take place in which the electricity-driven reduction of CO2 and the reduction or oxidation of other organic feedstocks like wastewater occur. A BES consists of an anode compartment, a cathode compartment and a membrane separating the two. An oxidation process (e.g. wastewater or acetate oxidation) occurs on the anode side, and reductive reactions (e.g. O2 reduction or H2 evolution) on the cathode side.108 Since electrons are donated to or received from electrodes, redox balances can be achieved by microorganisms without the oxidation of substrates or the production of reduced by-products.13 Electrons can be transferred either directly between the cell and the electrode or via soluble molecules that are able to become reduced and oxidised and to receive electrons from cells to transport them to the electrode, and vice versa. The efficiency of a scaled-up BES depends strongly on those electron transfer rates, which current research efforts are seeking to maximise.109
A BES can be operated in three modes.
• As a microbial fuel cell (MFC) to deliver electricity directly.
• As a microbial electrolysis cell (MEC) in which the anode and the cathode are connected without a resistor.
• As an MEC into which power is invested to increase the reaction rate and/or to enable thermodynamically unfavourable reactions.108
In addition to electricity generation, in theory three product groups are particularly suited to wastewater resource recovery by means of a BES, in that this offers real advantages over conventional production techniques. These product groups are:
• Bulk chemicals, like biofuels, platform chemicals and plastics.
• High-value chemicals, like pharmaceutical precursors, antibiotics and pesticides.
• Inorganics like nutrients, which can serve as fertilisers and so on.13
Despite remarkable research progress, the major bottlenecks hindering large-scale BES-based wastewater resource recovery are high overall costs (especially for expensive metal catalysts and membranes) and the fact that most research is limited to lab-scale applications. Outside the laboratory, the performance of pilot plants remains unstable due to water leakage, low power output, influent fluctuations and unfavourable product formations. To become a viable alternative to conventional wastewater treatment, BESs need to be scaled up to at least cubic-metre proportions, with reactor configurations that allow easy integration into current plant designs and infrastructures.20 Due to these technical bottlenecks and the low value of electricity, energy recovery by BES is considered likely to remain, at best, a niche application in wastewater treatment.19 As for BES-based H2 production, limited rates of microbial metabolism and rather restricted physical and chemical operational conditions are severe limitations.124 Moreover, MECs cannot compete with methane production in conventional anaerobic digesters, even at moderate temperatures.125 Consequently, methane production via electromethanogenesis is most unlikely to replace anaerobic digestion for methane recovery from high-strength wastewaters.126,127 To sum up, bioelectrochemical routes are still far from being a practical solution for resource recovery in WWTPs.
Thermal energy
Municipal wastewater contains 2.5 times more thermal energy than the theoretical maximum chemical energy stored in the COD (assuming a 6 °C effluent temperature change).7 Thermal energy in WWTP effluent stems from household and industrial water heating and, marginally, from microbial reaction heat released during the treatment process.128 Since the temperature of the effluent shows relatively small seasonal variations by comparison with atmospheric temperatures, it can serve as a stable source of heat that is recoverable using heat pumps. It is recommended that the effluent be used as an intake source for heat pumps because the influent still contains many contaminants that can cause fouling problems in the equipment. In addition, the decrease in the influent temperature caused by heat exchangers may adversely affect biological reactions during treatment.129 Heat pumps use electricity to extract low-temperature thermal energy from the wastewater and usually provide 3–4 units of heat energy per unit of electrical energy consumed.130 In addition to heating or cooling buildings, a potentially interesting on-site use of recovered thermal energy is sludge drying.
As with water reuse, however, the potential mismatch between supply and demand in terms of time and location represents a potential bottleneck hindering thermal energy recovery. One possible solution to this problem is the use of thermal energy storage facilities, such as aquifers.12 Selling surplus heat to nearby consumers is recommendable, but especially in spring and autumn demand may be insufficient due to a reduced need for district heating or cooling.129 In 2008, it was reported that more than 500 heat pumps for wastewater, with capacities of 10–20 MW, were already operational.131 Large-scale district-heating systems using thermal energy derived from wastewater have been established in many parts of the world.130 Especially in Japan, it has been shown that heating and cooling systems using wastewater can reduce energy consumption substantially. In Osaka, for example, the city government achieved energy savings of 20–30% by introducing thermal energy recovery from effluents. In the city of Sapporo, effluents are used directly to melt large quantities of snow every winter.94
Hydropower
Applying hydropower technologies to effluents is a well-known means of recovering electricity by taking advantage of constant discharge from WWTPs and, depending on the location, a certain hydraulic head. Useful technologies range from the Archimedes screw to water wheels and turbines, all of which deliver reliable performance when applied to an effluent flow. However, if such technologies are applied to untreated wastewater, they must be made from stainless steel to prevent corrosion.132 The power output of a hydropower technology depends on the rate of flow and the hydraulic head. As with any other energy-recovery route, its economic viability is also influenced by non-technical factors such as electricity prices, taxes, financial incentives and the cost of connection to the power grid. If the recovered electricity is used on site, the system becomes economically more attractive when energy prices rise. Economic viability is therefore always site specific and depends not only on physical circumstances, such as the technology selected, but also on both present and future market conditions.133 Although individual large-scale applications in Australia, the UK and Ireland have proven the economic viability of hydropower technologies in WWTPs, most scientific case studies lack a detailed analysis of this factor. The most important parameter for the hydropower potential of a WWTP effluent stream is the rate of flow, which is subject to seasonal, economic, infrastructural and demographic variations. Installations are usually designed for a defined flow and pressure, and so these parameters should be kept as constant as possible in order achieve consistent performance.134
Summary: energy recovery
Although complete recovery of all the energy contained in wastewater may be unrealistic due to conversion losses, energy-neutral or even energy-positive WWTPs are increasingly becoming practicable.122 At least 12 plants in Europe and the USA have been reported as reaching more than 90% energy self-sufficiency.135 The European research project Powerstep is currently elaborating designs for energy-neutral and energy-positive WWTPs through six different case studies.136 The recovery of methane to generate electricity can usually offset 25–50% of a WWTP's energy needs, assuming that conventional treatment technology is used.40 If thermal energy recovery from effluent is applied along with chemical energy recovery, carbon neutrality or better can be achieved.137 However, the water industry's strong focus on energy sustainability has also been criticised as misleading because, it is argued, wastewater treatment should prioritise the optimisation of the hydrological cycle over energy and climate concerns.5 Moreover, materials – rather than energy – can be recovered from COD. This aspect is gaining increasing attention, as discussed below.
Fertiliser recovery technologies
WWTPs are linked to global nutrient cycles because a fraction of the N and P applied as fertiliser in agriculture ends up in the wastewater stream.8 One global estimate suggests that fertiliser production accounts for more than 1% of the world's emissions of anthropogenic greenhouse gas (GHG) and demand for energy. Over 90% of these emissions are related to the production of ammonium fertiliser.138 From a resource-efficiency perspective, it is a paradox to produce ammonia fertiliser by the Haber–Bosch process, with its high energy consumption, and then to destroy it again after use in WWTPs by biological nitrification and denitrification, which also consume large amounts of energy. Ammonia recovery therefore offers potential energy savings, as long as it can be achieved with lower energy consumption than industrial production.8
Compared with N, the recovery of P is much more urgent because it is a finite resource with projected scarcity.120 Mining P from rocks has a huge environmental impact because it generates by-products like gypsum, which are often contaminated with radioactive elements and heavy metals and are not disposed of in an environmentally friendly manner.2 P enters the wastewater stream in faecal matter, household detergents and industrial effluents,139 at a typical concentration of about 6 mg P per l.140 If influent P is not removed during the treatment process, it can reach bodies of surface water and cause their ecological destruction.53
Nutrient-recovery technologies have been widely studied and a variety of solutions have been developed (see Fig. 3). Since the efficiency of nutrient recovery typically decreases with lower concentrations in the wastewater stream, a sequential three-step framework has been recommended:141
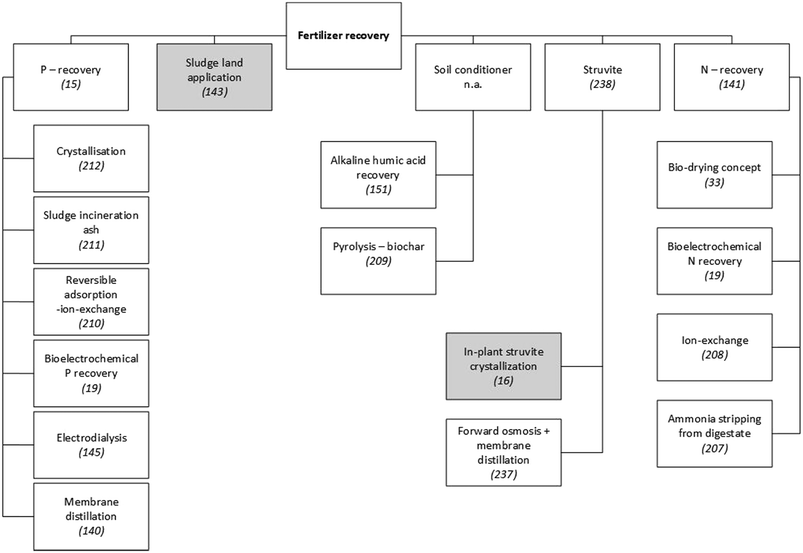 |
| Fig. 3 Examples of technologies to recover fertiliser from municipal WWTPs. Since a detailed presentation and discussion of these technologies is beyond the scope of this paper, scientific publications that explain or review them are referenced. Grey shading indicates techniques that have been applied on a large scale in municipal WWTPs. Unshaded boxes show technologies that are not widely applied.15,16,19,33,140,141,143,145,151,207–212,237,238 | |
1. Nutrient accumulation by biological, chemical or physical methods.
2. Release of nutrients by biological, chemical or thermal methods.
3. Nutrient extraction and recovery in the form of concentrated fertilizer, by chemical or physical methods.
Land application of sludge
Wastewater fertiliser recovery currently takes place either indirectly through struvite precipitation or directly by spreading sewage sludge onto agricultural land.142 About 40% of all sludge generated in the EU is recycled using the latter method.54 However, contamination can be a problem when sludge is applied to arable land. High contaminant loads have been found in bacterial biomass leaving WWTPs as secondary sludge.138 Moreover, sludge has a low nutrient content and is therefore a low-quality fertiliser compared with conventional fertiliser products. Nevertheless, it can still contribute towards the stabilisation of a soil's organic carbon content. The transport of dewatered sludge to the field can also be a bottleneck, since it is expensive due to the product's high water content (70–90%).143
Struvite
Struvite precipitation as a recovery route for ammonia and phosphate has gained a lot of interest among researchers in recent decades, and it is applicable on a large scale.16 Struvite is magnesium ammonium phosphate (MgNH4PO4·6H2O), a mineral commonly formed in WWTPs through spontaneous precipitation if Mg concentrations are high enough, although this is often not the case. The formation and growth of struvite crystals in WWTPs is affected by various parameters, such as pH, temperature, mixing energy and turbulences, and the presence of other ions like calcium or carbonates.144 Struvite precipitation is usually introduced to solve operational problems, in particular the clogging of equipment.145 The N and P fractions in struvite are slowly soluble, which makes struvite usable as a slow-release commercial fertiliser suitable for soils with a low pH value.138,140
It has been shown that effective struvite precipitation can only be achieved if P concentrations are above 100 mg l, and also depends on ammonium concentration and pH value. Lower P concentrations lead to significantly lower recovery rates and longer precipitation reaction times, and require higher pH values. Consequently, struvite precipitation is probably not feasible for wastewater with low P concentrations.140,145 Nutrient enrichment is usually required prior to struvite precipitation and recovery from side streams in WWTPs. Using an enhanced biological phosphorous removal (EBPR) process, like supernatant from anaerobic sludge digestion or sludge dewatering processes, is most feasible. In most cases, Mg salt has to be added to fully remove soluble P as struvite from these streams.146 The majority of WWTPs, however, have chemical P-removal systems that preclude struvite formation.54 Due to those wastewater P fractions that are fixed in biomass or bound to metals like Fe and are consequently unavailable for struvite formation, the efficiency of the recovery of influent P as struvite is usually only 10–40%.34 Even if favourable conditions for struvite precipitation, such as low total suspended solids (TSS) and high solubilised NH4+ and PO43− concentrations, are established by continuously removing biomass,138 the recoverable amounts are rather low and unlikely to exceed 1 kg of struvite per 100 m3 of wastewater.147
Ref. 16 reveal that the cost of recovering struvite after sludge digestion with the aid of chemical additives (e.g. magnesium salt), including manpower and maintenance, could be as high as €2 per m−3 of raw wastewater. This is economically unviable. The cost effectiveness of struvite recovery from the water line without prior P concentration by EBPR or chemical P removal (CPR) has not been calculated.120 However, since struvite recovery can significantly reduce volumes of sludge due to its subsequent enhanced dewaterability, this technique may decrease sludge handling and disposal costs.16 In addition, it prevents the clogging of pipes.145 These operational cost benefits should be included when assessing the cost effectiveness of struvite recovery. The market value of struvite, as a relatively new fertiliser, is uncertain and may be influenced by rates of production and regional demand.16 In addition, fractions of heavy metals and organic contaminants present in wastewater could end up in the product and thus limit its safe agricultural application,140,148 for example, have revealed that recovered struvite crystals can contain arsenic concentrations of up to 570 mg kg. Successful struvite recovery can also be hindered by a lack of legal regulation. It was first successfully recovered in the Netherlands in 2006, but it took about ten years before the legal framework was finally adjusted to allow the application of struvite in agriculture.12 Despite the change in the law, however, no breakthrough in the implementation of struvite recovery seems to have occurred. It must therefore be questionable how severely that legislative bottleneck actually impacted the use of the technique.
Sludge incineration ash
Technologies that recover P from sludge incineration ash are currently in focus because they promise high influent P recovery rates. In order to achieve high recovery efficiencies, however, they require special incinerators and these can be very costly.149 Moreover, this technique is still under development and not all its pros and cons are yet known. But one clear advantage over other P recovery routes is that it occurs at the very end of the process and so does not conflict with other measures taken in the WWTP.12 Like the use of sewage sludge in the environment, however, ash is associated with heavy metal contamination. Whilst chemical extraction can be used to obtain pure phosphates from it, post-treatment of the treated ash – at greater cost – may then be required for heavy metal removal. Alternatively, ashes can be used in the construction industry without any pre-treatment. But this does not involve P recovery.141
Soil conditioner
Used alongside mechanical and thermal methods, alkaline treatment is a simple and highly efficient chemical means of disintegrating sludge. In addition to reducing the volume of sludge even further after conventional dewatering processes have been applied, it also responds to the fact that the released water contains large amounts of dissolved organics like proteins, humic acids, lipids and polysaccharides, plus residual NaOH. Most of these can be degraded further by subsequent treatment processes, but that is more difficult in the case of humic acids due to their high recalcitrance to microbial degradation. Applied as a soil conditioner, humic acids contribute to the slow release of nutrients and high cation-exchange and pH-buffer capacity, as well as the retention of heavy metals and xenobiotics in soils.150 The extraction of humic acids from alkaline sludge treatment supernatant can be achieved by membrane filtration with a 45 μm mesh,151 but the cost effectiveness and detailed impact of humic acid recovery remain to be analysed.
Another soil conditioner recoverable from sewage sludge is biochar, which can also be used as a coal-like fuel. The production of biochar and its storage in soils is often suggested as a potential means to sequester atmospheric carbon.152 Biochar is obtained from sludge pyrolysis, which is the process of thermally cracking organic matter via an external heat source and without the supply of air.153 As well as carbon sequestration, biochar's potential addition to soils is associated with a wide range of other possible secondary benefits, such as the liming of acidic soils, reducing plant aluminium availability, increasing cation-exchange capacities, reducing nutrient leaching, remediating sites contaminated by heavy metals and chemicals, increasing agrochemical sorption and reducing net GHG emissions from soil.154 In general, though, our understanding of the impact of biochar on single or combined soil attributes remains poor. Because of this, the consequences of its application for crop yields and its related potential impact on global warming are both hard to predict and very site specific.155
Membrane-based nutrient recovery
Electrodialysis, membrane distillation and forward osmosis are emerging nutrient-recovery technologies that have been reviewed extensively by.140 The attractiveness of membrane-based technologies for wastewater nutrient recovery lies in the separated streams of concentrated nutrient ions and the abatement of chemicals for ion precipitation.156 But no detailed techno-economic analyses revealing demand for energy, CO2 footprint, system robustness, operating costs, product quality and market demands are available. These technologies therefore remain a fairly theoretical option, still a long way from practical application in large-scale wastewater treatment facilities.140
Summary: fertiliser recovery
One general bottleneck hindering energy- and cost-effective nutrient recovery from wastewater is the rather low quantities obtainable, certainly by comparison with industrial fertiliser production systems, which gives this route a competitive disadvantage.120 Numerous new P recovery technologies have been developed for various access points in WWTPs, and in recent years some of them have been implemented at full scale. A thorough assessment of these emerging routes is provided by.15 Since global demand for fertiliser is expected to increase by 4% a year due to population growth,157 it can be expected that P fertiliser recovery from wastewater will gain further importance in the future. Its cost, however, is likely to exceed that of P ore-derived fertiliser several times over, as shown by ref. 34 for German market conditions. As well as conventional fertiliser, manure from livestock production also competes with fertilizer recovered from wastewater.23 show that, in Flanders, the P entering WWTPs could fulfil 14% of total local fertiliser P demand, while the P contained in manure could easily satisfy this demand alone.23 It is therefore likely that wastewater-derived P fertiliser is redundant in livestock-intensive regions, as shown in Fig. 4.
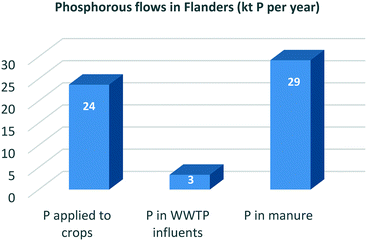 |
| Fig. 4 Phosphorous flows (kt per year) in the livestock-intensive region of Flanders (Belgium).23 | |
However, P can be recovered in a WWTP at different stages of the process. Although 30% of influent P is not solubilised as phosphate (PO43−) but bound to organics, much of the remainder will likely solubilise by hydrolysis in the primary clarifier at the start of the process.158 After primary treatment, therefore, P is predominantly present in the liquid phase. Following secondary treatment with either EBPR or CPR, or both, 90% of the influent P is contained in the sludge as either metal phosphates or polyphosphate in biomass. It might therefore be most efficient to apply a recovery step after the biological treatment process – for example, recovery from sludge incineration ash. This can achieve a recovery rate of up to 90%.34
The recovery of N from municipal wastewater could save fossil energy used to produce N fertilisers by the highly energy-intensive Haber–Bosch process.120 Usually, at least 75% of WWTP influent N is solubilised ammonium (NH4+).158 This fraction is highly diluted, which makes ammonium recovery an energy-intensive process and thus too costly.159 At typical municipal wastewater concentrations of 20–70 mg per N l, physico-chemical ammonia recovery technologies (e.g. stripping and thermal evaporation) would not be economical. During the CAS process, ammonia is converted biologically into nitrogen gas that is released into the atmosphere. The 25% organic influent N consists partly of urea and hydrolysed proteins, both of which are also present in a solubilised form. Consequently, the reported values of influent N fractions that end up as organic N in the sludge during the CAS treatment are only about 20%.31,32 Current N recovery technologies are usually limited to this minor N fraction. Because of this, in recent years greater attention has been paid to more energy- and carbon-efficient biological N removal technologies, such as the combined nitritation–anammox processes, rather than N recovery practices.120 However, an extensive overview of economic N recovery constraints has been produced and still appears to be valid.160
Other product recovery technologies
Besides fertilizers, various other products can be recovered from wastewater, as shown in Fig. 5. A number of publications point out the potential contribution towards sustainable development that is achievable by applying product recovery technologies in WWTPs.6,13,161 Although some of these routes are attracting increased interest in terms of upscaling their application, none is yet reported as being widely used.
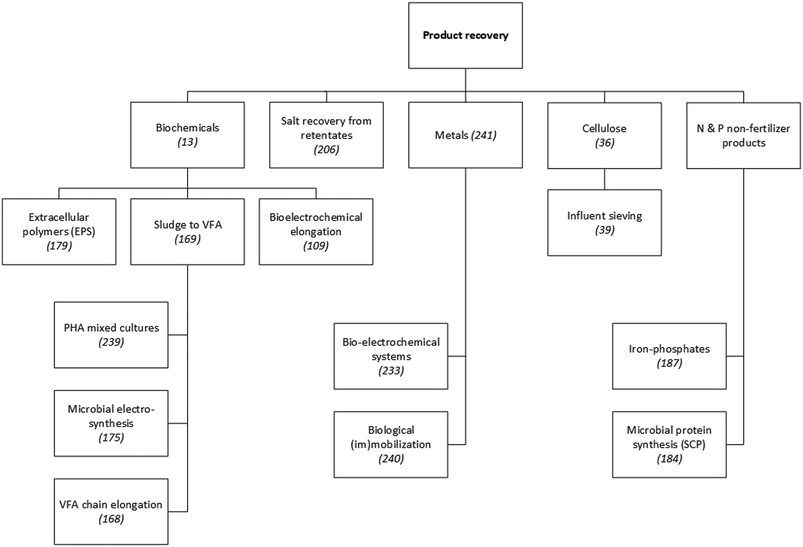 |
| Fig. 5 Examples of technologies to recover other products from municipal WWTPs. Since a detailed presentation and discussion of these technologies is beyond the scope of this paper, scientific publications that explain or review them are referenced.13,36,39,109,168,169,175,179,184,187,206,233,239–241 | |
Volatile fatty acids
One possible product recovery route is the integration of the carboxylate platform into wastewater treatment systems. Carboxylates are dissociated organic acids that can be produced by hydrolysing and fermenting primary sludge with undefined mixed microbial communities. To do so, it is necessary to inhibit methanogenic bacteria accumulation by applying a short sludge retention time (SRT) to wash slow growing methanogens out of the reactor, and/or by establishing a very high pH value during fermentation.162 Important products of these procedures include VFAs, which consist primarily of the short-chain fatty acids acetate, propionate, lactate and n-butyrate. These are valuable products when separated from the fermentation broth because they act as substrates for secondary fermentation and electrochemical or thermochemical refinements to higher-value chemicals like fuels or bioplastics.163 VFA recovery from primary sludge can be improved either by adding activated sludge to the fermentation broth,164 or by using a surfactant like sodium dodecylbenzene sulphonate and maintaining a high pH value during fermentation.120 The fermentation liquids from a VFA fermenter can be used for treatment process optimisation, as they contain easily biodegradable carbon sources that are useful for biological nitrogen and phosphorous removal.116,164,165 Another advantage of VFA fermentation is the reduction of excess sludge quantities and the associated disposal costs.166
Ref. 167 state that controlling the product spectrum in open-culture fermentation systems remains a major bottleneck in VFA recovery from waste streams, especially for products derived from carbohydrates. Another bottleneck is the solubility of VFAs, which leads to difficulties in efficient downstream processing.168 VFAs can be distilled off the fermentation broth under atmospheric pressure, but that requires too high an input of energy to be economical.110 The same applies to the concentration of VFAs through nanofiltration or liquid–liquid extraction, whereas anion exchange might well be a more feasible downstream solution. Another possibility is to convert VFAs directly after fermentation into an end product that is then separated from the liquid.167 However, very few studies have examined all pertinent parameters of VFA production routes from waste streams and most of the variables have yet to be examined satisfactorily. Such uncertainties contribute to the fact that most waste-based VFA production concepts are still confined to the laboratory.116
Although higher added-value products can be derived from VFAs, this does not imply that waste-based VFA production is economically preferable to methane generation. Only if calculations consider the costs of bioprocess operations and downstream processing, as well as potential subsidies for biogas production, can an economically substantiated decision be made.167 As an economically feasible recovery route with municipal wastewater,120 propose a COD up-concentration step with subsequent alkaline VFA fermentation. If COD is up-concentrated and fermented to VFAs, denitrification might underperform due to the lack of an easily degradable carbon source. Because of this, the development of N removal processes that perform sufficiently well at low COD concentrations is required.169
Polyhydroxyalkanoates (PHAs)
One possibility for the refining of VFAs is to convert them into PHAs, which are fully biodegradable biopolyesters that are able to substitute fossil-fuel derived polymers. Due to their comparable properties, PHAs are often referred to as bioplastics. PHAs act as carbon/energy storage polymers for more than 300 species of bacteria and archaea. These species can produce and store high concentrations of a PHA inside their cells.170 Mixed-culture PHA production from wastewater and other organic waste streams is currently achieved using a three-step procedure.
• COD is fermented in an acidogenic reactor to produce VFAs.
• PHA-producing biomass is established and maintained in a separated reactor.
• The biomass is fed with the VFAs in a third reactor until the PHA content of the selected community is maximised.171
An alternative procedure was trialled in 2015 by Dutch water utilities, which collaborated in a one-year pilot study on activated sludge in 15 WWTPs using biological nutrient removal (BNR). BNR plants with pre-denitrification exert an anoxic feast period on the biomass, and this promotes PHA-storing biomass. Up to 50% gPHA/gVFS have been harvested from these WWTPs without changing their infrastructure.62
However, the PHA yield on the substrate and the efficiency of the downstream processing lead to costs that are 20–80% higher than those for petrochemical polymers of a similar quality.172 Value creation from wastewater-derived PHA depends on the security of polymer supply in terms of both quantity and quality, but until recently no study had provided a clear answer to the question whether a mixed culture process could fulfil these criteria.62 Recovered bioplastics are not yet cost-competitive and therefore have limited market potential.12 The development of new PHA utilisation routes and marketable applications remains a challenge for the future.173
Carbon-chain elongation
One rather innovative route for refining wastewater-derived VFAs in a way that overcomes their inefficient downstream processing is elongation of the carbon chains to form medium-chain fatty acids that have a higher monetary value.174 Such elongation can be achieved along different microbial pathways in anaerobic open-culture fermentation processes when reduced compounds are present.175 The medium-chain fatty acids (MCFAs) thus obtained have much higher energy densities due to their lower oxygen to carbon ratio, and are therefore superior to VFAs as fuel-precursor chemicals.176 Their increased hydrophobicity results in lower solubility, and thus in more energy- and cost-efficient separation properties.168 However, questions about how best to shape the microbiome and, if such shaping is successful, how to construct a stable and resilient system suitable for industrial-scale application need further study. In addition, improved extraction technologies are needed, in particular to operate in line with the fermentation system.175 Moreover, the metagenomics of impactful microbial cultures need to be analysed in order to further verify and define them.174
Extracellular polymeric substances (EPSs)
In recent years, the aerobic granular sludge (AGS) process – which is also known as the NEREDA process – has been applied successfully in several full-scale wastewater treatment plants around the world. AGS can be described as self-immobilised bacterial communities.177 Its formation can be stimulated by discontinuous influent feeding.178 EPSs are responsible for the physical and chemical structure of the granules; they are bacteria-secreted sticky polymers consisting of proteins, polysaccharides, phospholipids, lipids and humic acids, which evoke cell adhesion and lead to the formation of aerobic granules. Extracting EPSs from AGS is a potential future product recovery route that can yield a high-value product. In the Netherlands, plans have been drawn up for two full-scale demonstration systems for commercially viable and sustainable EPS recovery.45
A method using sodium carbonate (Na2CO3) and calcium ions (Ca2+) extracts EPSs from sludge in the form of stable ionic gel granules that, amongst other properties, behave in a similar way to alginate,179 even though they have a very different chemical composition. Recently, ‘Kaumera’ has been registered as a product name for EPSs derived from AGS. However, alginate is conventionally produced from brown seaweed180 and can form hydrogels that are biocompatible, non-toxic, non-immunogenic and biodegradable.181 Established alginate utilisations include pharmaceutical, food and technical applications, such as in printing paste for the textile industry.182 It is likely that the alginate market is not the only potential niche for recovered EPSs. Because their wide range of interesting material properties are still not fully understood, and also due to their novelty, it has yet to be demonstrated which conventionally produced niche polymers could be substituted with these materials and their composites.183 Indicates that the range of possible applications for EPSs, both as a composite and as a raw material, is extensive. If alginate is to be substituted with wastewater-derived EPSs, however, they must be produced more cheaply than conventional alginate, not least because the current level of production – namely 30
000 tonnes annually – is estimated to be only 10% of the alginate-like material potentially obtainable from wastewater. This indicates a high unexploited potential for conventional production, which is especially valid if new chemical and biochemical techniques are developed to allow the creation of conventional but modified alginic-acid derivatives tailored for certain applications.43
Single-cell protein (SCP)
One well-documented product recovery technology is SCP synthesis. This process uses electrical energy from renewable energy surpluses to produce H2 by electrolysis, to function as an electron donor for H2 oxidising bacteria. In addition, ammonia stripped from sludge digestion liquids provides a third feedstock for the process. For the protein synthesis, minerals are added to promote optimum growth of the biomass. As a result, ammonia-to-protein efficiencies of close to 100% can be achieved.184 Used as feed for livestock, this protein could alleviate the pressure for land conversion since approximately 80% of agricultural land is used to grow fodder. If the protein obtained were to be used in food applications, though, consumer acceptance would be an issue.32 Nevertheless, we believe that the inherent fear related to the use of products recovered from faecal matter could be overcome by education as well as the application of safe and effective technologies. Currently, the use of SCP produced from municipal wastewater is forbidden anyway by EU legislation.169 If this technology were integrated into the CAS process, however, it would recover only the influent N that ends up in the sludge (approximately 20% of the total).31,32 To harvest the solubilised ammonia in municipal wastewater as well, up-concentration techniques would have to be applied.141 provide a detailed overview of emerging N recovery technologies, which can be used for a more in-depth analysis of the topic.
Iron phosphate
Significant iron (Fe) loads can enter a WWTP via Fe-rich industrial wastewater, groundwater infiltration and Fe dosing of the sewerage system to prevent the emission of hydrogen sulphide (H2S). Moreover, the addition of iron in the form of ferric (FeIII) or ferrous (FeII) salts is the most common chemical P removal (CPR) method used in WWTPs and can introduce significant iron phosphate precipitates into their sludge lines. When CPR is applied, 40–50% of the total influent P precipitate is in the form of vivianite (Fe32+(PO4)2·8H2O).185 This is therefore likely to be the most abundant form of phosphate in digested sludge, and hence of particular interest when it comes to P recovery. However, the extraction of pure vivianite in crystal form requires more knowledge about the factors that determine its formation.149 Varying reaction conditions in different reactors (aerobic or anaerobic), amorphous and crystalline iron-phosphate molecule structures, the presence of humic substances and sulphates, and varying oxidation–reduction potentials and pH values in a plant's different units, make microbial- and chemical-induced iron phosphate reactions exceptionally diverse. In order to develop P recovery pathways and possibly to control favoured iron phosphate formations during the treatment process, a better understanding of these mechanisms is needed.54 Nevertheless, an innovative pilot system (‘ViviMag’) using magnetic separation to recover vivianite from digested sewage sludge is under construction in the Netherlands.186,187
Cellulose
Cellulose recovery from wastewater treatment processes has recently gained attention in scientific literature.36 Cellulose fibres in municipal wastewater originate mainly from toilet paper, which is a considerable fraction of the influent COD, and they are hardly degradable during aerobic treatment, especially under cold-weather conditions, and only 50% are anaerobically digested.39 Although cellulose recovery decreases biogas production by more than 10%, cellulose extraction improves WWTP operations through lower aeration requirements and reduced excess sludge quantities, which may lead to an overall positive energy balance.161 High recovery rates can be achieved by applying fine mesh sieves (<0.5 mm) in the primary treatment line;38 these remove a significantly higher fraction of the cellulose fibres from the main line than do primary settling tanks.37 Potential applications for recovered cellulose include soil conditioner, fuel for biomass combustion plants, feedstock for the fermentation industry,39 aggregate for construction materials (e.g. asphalt) and raw material for the paper pulp industry.38 Another interesting emerging application of cellulose is its refinement into nanocellulose – a nanocomposite with unique properties.36 The production of new toilet paper is also possible, but it is questionable whether consumers would accept this true cradle-to-cradle approach.39
Summary: product recovery
Initial findings concerning some of the product recovery routes reviewed above show promising results in terms of quantities and market prices.6 Since most of these routes utilise the organic carbon in wastewater, methane recovery from COD by integrating anaerobic digestion into the CAS process has been criticised for its high energy losses, leading to an overall energy efficiency of only about 15%.27,120 The recovery of COD as organic materials rather than energy is seen as a promising alternative due to the much higher monetary value of organic chemicals.13 Since COD-derived product recovery routes may exclude each other or require trade-offs, the value of the recovered products can also be an important criterion when choosing a specific route. This is the case with, for example, the recovery of EPS and PHA.12 As mentioned, however, the consumer's association of wastewater-derived products with faecal matter is a major barrier to several innovative recovery routes. Developing value chains for these products therefore poses new challenges for water management utilities, as they are often in non-consumer niche markets.9 To ensure that they are marketable, their technological development must involve input from regulators, managers of wastewater facilities, engineers, researchers and the public.11 The financial and operational risk of upscaling innovative product recovery routes should be shared among these stakeholders to build confidence in pioneering applications.188
Bottlenecks in wastewater resource recovery
As discussed above and presented in Table 2, a variety of issues that may hinder the successful implementation of RRRs are mentioned in the scientific literature. These relate to nine bottlenecks that can be grouped into three categories (A–C).
Table 2 Detailed overview of bottlenecks mentioned in scientific literature that may hinder the successful implementation of RRRs in municipal WWTPs. Bottlenecks are categorized into (A) economics and value chain, (B) environment and health, or (C) society and policy
Category A. Economics and value chain |
Bottleneck |
Description |
Resource |
Issue |
Ref. |
Process costs |
A resource recovery process is not cost effective due to excessive operational or investment costs |
Water |
High energy demand of membrane technologies. Per m3 water reclaimed by secondary effluent treatment with ultrafiltration and reverse osmosis a cost of 0.46 € and a benefit of 0.25 € has been calculated |
2, 18 |
|
Fouling as an additional cost factor for membrane technologies. Costs vary greatly and depend on membrane characteristics, operating conditions, feedwater quality and applied cleaning techniques |
194
|
|
Disposal costs of membrane retentate depend on level of treatment, retentate characteristics and disposal method |
64
|
|
Advanced oxidation processes are energy intensive and require expensive reagents |
89
|
|
Energy |
Microbial fuel cells: expensive equipment and operation |
3, 195, 196 |
|
NH3 recovery for fuel is not cost effective because energy costs of removing NH3 often exceed the energy and value of recovered gas |
122
|
|
Fertilizer |
P recovery costs exceed conventional P ore costs. Assuming a load of 660 g P per capita per year, recovery costs would be 3.600–8.800 € per tonne recovered P under German market conditions |
34
|
|
Struvite recovery processes may not be cost effective which depends strongly on profits from struvite sales. Market prices vary greatly and have been estimated for e.g. Australia to lie between 180–330 € per tonne |
16
|
|
No cost-effective processes for recovering P from Fe–P have yet been developed |
54
|
|
P recovery from sludge incineration ash requires specialised and expensive incinerators |
149
|
|
Other products |
PHA recovery processes can be more costly than conventional production routes. Recovery costs depend greatly on applied downstream processes and may range between 1.4–1.95 € per kg |
172
|
|
CO2 recovery from biogas is economically feasible only if a biogas upgrading unit is already present. Payback times for recovery equipment may vary between 1–12 years |
46
|
|
Bioelectrochemical systems may require expensive electrodes (e.g. platinum cathodes) |
109, 197 |
|
Microbial electrolysis cells using CO2 for chemical production require extra energy input depending on the electron donor used. The potential of municipal wastewater as electron donor is not quantified yet |
108
|
|
Resource quantity |
Compared with conventional production systems, only small quantities of a resource can be recovered at a WWTP. This may be due to low process yields, low resource concentrations or low overall resource quantities in the wastewater stream |
Energy |
Combined heat and power units for recovered CH4 have high conversion losses of ca. 60% |
44
|
|
COD may be too diluted for effective direct anaerobic digestion of wastewater.750 mg COD per litre is a medium concentration for municipal WWTP influents |
27, 109 |
|
Dark fermentation of sludge shows very low H2 yields of ca. 17% |
117
|
|
Fertilizer |
Nutrient quantities recoverable from wastewater are low compared with industrial production rates. E.g. in Flanders (Belgium) yearly mined P imports amount of 44.100 tonnes while combined WWTP influent-P amounts only of 3.350 tonnes |
23, 167 |
|
Struvite: low P concentrations limit precipitation which requires at least 100 mg P per litre |
140, 145 |
|
Struvite: only soluble P fraction of side streams is recovered |
54
|
|
Low N concentrations of only 30 mg per litre NH4-N in average Dutch wastewater may make NH4 recovery uneconomical |
120, 159 |
|
Other products |
VFA concentration in wastewater and fermenter effluent may be too low for economical extraction |
108
|
|
Optimisation by economies of scale is limited due to low resource quantities in wastewater |
167
|
|
Resource quality |
The quality of a recovered resource is not high enough to market easily. This may be due to contaminants or impurities in the resource |
Fertilizer |
Field application of sewage sludge: high water content (70–90%) and low nutrient content (7 kg P per tonne) |
143
|
|
Possible contamination of struvite |
140, 198 |
|
Other products |
Recovered biochemicals often lack the purity demanded by chemical industries |
13
|
|
Controlling the product spectrum in open-culture VFA fermentation is a challenge and depends on pH, temperature, dilution rate, types of carbohydrates present and feeding patterns |
167
|
|
Uncertainty about whether mixed culture derived PHA from municipal wastewater can deliver reliable quality remains to be validated although pioneer pilot testing has been conducted with promising results |
62
|
|
Market value and competition |
Conventional production systems potentially outcompete the RRR. This may be due to various factors, including higher product quality and quantities or lower production costs |
Energy |
CH4 has a low market value (EU-28 average 2019: 0.046 € per kWh for household consumers) |
108, 167, 199 |
|
Electricity has a low market value (EU-28 average 2019: 0.22 € per kWh for household consumers) |
13, 200 |
|
Fertilizer |
Bulk nutrients from the fertiliser industry are available cheaply (phosphate rock: 110 US$ per tonne in 2014) |
13, 120, 201 |
|
In livestock intensive regions P-rich manure is often abundantly available as an alternative fertiliser (see Fig. 4) |
>23
|
|
The market value of struvite is hard to estimate due to a lack of knowledge and trust of farmers into its fertilizing potential |
16
|
|
Other products |
Petrol-based plastics may outcompete bioplastics and the latter are produced more economically from pure microbial cultures using sugar as feedstock instead from mixed microbial cultures applied to wastewater |
12, 173 |
|
Finding real advantages of recovered biochemicals over fuel- or sugar-based alternatives to justify higher price of biodegradable/bio based plastics compared to conventional plastics (2.5 US$ per kg compared to 1.5 US$ per kg in 2014) |
13, 173 |
|
Utilisation and applications |
The usefulness of recovered resources might be unknown. New market niches, applications and partners have to be found to make an RRR successful |
Other products |
Identifying niche markets (local or otherwise) and applications with unique selling propositions to increase competitiveness |
167
|
|
Developing public–private partnerships to market products can be a challenge |
9
|
|
New PHA product utilisation routes have to be found |
173
|
|
Logistics |
If recovered resources are not used on site, distribution and transport have to be organised. This may be challenging due to geographical and temporal discrepancies between supply and demand, lack of infrastructure, or cost |
Water |
Temporal and geographical discrepancies between supply of and demand for water must be considered |
97
|
|
Topographical location of WWTP might require uphill pumping of reclaimed water. A 100 m vertical lift is as costly as a 100 km horizontal transport (0.05–0.06 US$ per m3 in 2005) |
40, 202 |
|
Possible need for new pipeline infrastructure for reclaimed water |
48, 96 |
|
Energy |
Temporal and geographical discrepancies between supply of and demand for thermal energy need to be balanced out |
12, 129 |
|
Costs of pressurising and transporting CH4 if no connection to the natural-gas grid is present |
108
|
|
Fertilizer |
In-field sludge application: transport between WWTP and arable land might be too costly due to high water content |
143
|
|
Category B. Environment and health |
Bottleneck |
Description |
Resources |
Issue |
Ref. |
Emissions and health risks |
The use of recovered resources or the recovery process may entail risks to human health due to contaminants, or may cause emissions and environmental problems. This may be due to insufficient process control |
Water |
Potable water reuse has been evaluated as too great a health risk (by Amsterdam water board) |
12, 100 |
|
Incomplete removal of chemicals or pathogens during treatment may cause disease |
203
|
|
Chemical biocides used in tertiary treatment can generate harmful by-products |
68
|
|
Plant or soil contamination as consequence of wastewater reuse for irrigation |
101
|
|
Energy |
Unheated anaerobic digesters may promote emissions of solubilised CH4 |
27
|
|
Fertilizer |
Struvite may be contaminated with emerging pollutants and heavy metals |
140, 198 |
|
PAO biomass may accumulate contaminants if sludge is applied to agricultural land |
138
|
|
Category C. Society and policy |
Bottleneck |
Description |
Resources |
Issue |
Ref. |
Acceptance |
User acceptance of resources recovered from wastewater may be low due to fears or misconceptions about the risks they pose |
Water |
Water reuse projects can rarely be implemented without social acceptance |
22, 97 |
|
Direct potable water reuse raises psychological barriers |
4
|
|
Other products |
Toilet-paper production from recovered cellulose may not be accepted by consumers |
39
|
|
Single-cell protein: negative perception of faecal matter as source for feed/food production |
184
|
|
Policy |
To be successful, RRRs need adequate policy and legal frameworks. A lack of legislation, political will or economic incentives may hinder successful implementation |
Water |
Government incentives are needed to make water reuse financially attractive (in China) |
96
|
|
A lack of common regulations is a barrier to water reuse (in southern Europe) |
204
|
|
Lack of political will to implement legislation and policies for water reuse |
5
|
|
Energy |
Anaerobic digestion needs to be subsidised to become competitive with natural gas |
167
|
|
Fertilizer |
Lack of legislation on in-field struvite application |
12
|
|
Other products |
Legislation forbids the use of protein produced from faecal substrate (in Europe) |
169
|
|
Economics and value chain (A)
1. Process costs.
2. Resource quantity.
3. Resource quality.
4. Market value and competition.
5. Utilisation and application.
6. Distribution and transport.
Environment and health (B)
7. Emissions and health risks.
Society and policy (C)
8. Acceptance.
9. Policy.
Most of the bottlenecks are in the economics and value-chain development category. This reflects the findings of ref. 12, who state that particularly market potential and competition introduce uncertainties in respect of successful resource recovery from wastewater. However, some of the bottlenecks presented in Table 2 overlap other categories and so should be perceived as interlinked rather than absolute. Moreover, rather than interpreting bottlenecks as barriers to the implementation of resource recovery routes, they should be seen as starting points for WWTP process design and management strategies to overcome them. Their early consideration in the planning phase of resource-oriented wastewater treatment processes increases the chance of developing successful recovery routes.
However, a general policy related bottleneck for wastewater resource recovery implementation is the definition of waste. The end-of-waste concept has been manifested in the Waste Framework Directive 2000/98 to re-introduce recovered products from solid waste streams into consumption and change their definition by fulfilling certain end-of-waste criteria. The criteria shall promote product standardization and quality and safety assurance and improve harmonization and legal certainty in the recyclable material markets.189 Moreover, recovering materials from waste streams in accordance with generally accepted criteria gives a positive association with the materials as they are not any longer labelled as waste but as new and safe-to-use products. End-of-waste criteria need to be developed in accordance with the following four conditions: (i) the substance or object is commonly used for a specific purpose; (ii) a market or demand exists for such a substance or object; (iii) the substance or object fulfils the technical requirements for its specific purpose and meets the existing legislation and standards applicable to products; and (iiii) the use of the substance or object will not lead to overall adverse environmental or human health impacts.190 However, the end-of-waste concept has been considered so far only marginally in the field of wastewater resource recovery. It has been suggested mainly for the use of sewage sludge as feedstock or co-substrate for biogas production190 or as soil amendment product189,191 and for nutrient recovery strategies.192 The bottlenecks identified in this review hint clearly that the end-of-waste concept is yet insufficiently considered for most resources recoverable from municipal wastewater. Reasons are that active support from legislators and governance is lacking because recycling is mostly governed by fragmented decision-making in regional administrations. Active regulatory support such as recovery obligation or subsidies are yet missing in many countries. For example, to facilitate the marketing of recovered P the inclusion of such materials into existing fertiliser regulations has to be focused.193
The role of water management utilities
Water management utilities (WMUs) could possibly ease or even eliminate the listed bottlenecks to successful RRR implementation by proactively planning resource recovery routes. However, WMUs may not be sufficiently influential to tackle all bottlenecks to the same extent (Fig. 6). To reduce process costs, recover safe and environmentally benign products, or ensure that quality requirements for recovered resources are met, the right decisions need to be made at the process design level. Here, the WMU may have significant influence over the design of a process that meets all these requirements, because it traditionally possesses substantial expertise in process engineering and operations. To overcome bottlenecks related to the distribution and transport of recovered resources, as well as to find applications and utilisation possibilities, requires management decisions that are beyond the scope of technical process design. Similarly, the recovery of resources in competitive quantities can be managed actively. The volumes of recovered resources might be limited by factors related to the technical process, such as process yields, or by the fact that the wastewater stream contains only small quantities of a resource, but once this is recognised it may still be possible to increase the output of a resource by integrating other waste streams into the recovery process.116 If, for example, VFAs are recovered from COD, the integration of solid organic waste to obtain higher product volumes may strengthen the WMU's market power as a VFA supplier. Joining forces with other WMUs to recover and market a resource collectively is another possible management-driven strategy to increase output.
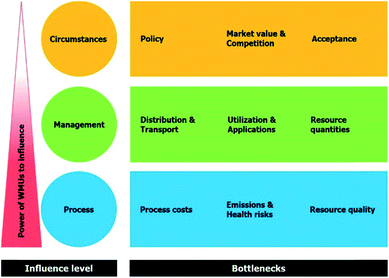 |
| Fig. 6 The power of water management utilities (WMUs) to influence identified bottlenecks for resource recovery technology implementation into WWTPs. | |
However, the successful implementation of RRRs also depends on factors that are more difficult for a WMU to influence. These are related to the broader circumstances in which an RRR operates. Examples include relevant policy and legislative frameworks, market values and the competitive situation, as well as user acceptance of a particular recovered resource. Though it is more difficult to leverage positive change at this level, a WMU can still develop strategies to convince policymakers or users about the necessity or harmlessness of an RRR. In general, greater competitiveness can be achieved by finding niche markets or by forming strategic partnerships with stakeholders within the value chain to develop a common approach, thus making the most of synergies.9 In addition, cooperation between WMUs – for example, joining forces to apply a common recovery strategy across multiple WWTPs and thus exploit economies of scale – could well enhance economic competitiveness.
WMUs may also need to find ways to gain support in scaling up innovative resource-recovery technologies. The implementation of new practices requires access to reliable data in order to build confidence that the innovation is compatible with the current process. There is currently little benefit for a WMU in being a pioneer in resource recovery, so these utilities should therefore seek support from value-chain actors or political institutions to share the risks of innovation implementation.188
Conclusion
Although domestic wastewater cannot fully satisfy the elemental or energy demands of industrialised societies, it does represent a substantial resource that should be fully utilised in the future. However, the data presented in Table 1 shows that not all resource recovery routes (RRRs) can meet substantial shares of overall resource demands. The market potentials of recovered water, energy, fertilizer and other products depend on the volumes demanded, the quantities contained in wastewater streams and the recovery yields obtainable. Before future treatment processes are designed from a circular-economy perspective, it is useful to be aware of the likely ability of the proposed RRRs to satisfy overall demand for relevant resources and, on that basis, to invest primarily in those with the potential to diminish conventional resource exploitation most substantially. We hypothesise that those RRRs, that contribute significantly to meeting overall societal resource needs are likely to attract more interest from public funding bodies or policy incentive schemes than those with lesser potential in this respect.
Although numerous technologies for the recovery of water, energy, fertilizer and other products from wastewater have been explored in the academic arena, few of these have ever been applied on large scale due to technical immaturity and/or non-technical bottlenecks. In all, we have identified nine such bottlenecks mentioned in scientific literature that may hinder the successful integration of RRRs into wastewater treatment plants (WWTPs) (Table 2). Six of these are related to economics and value-chain development (process costs, resource quantities, resource quality, market value, application and distribution), two to environmental (emissions) and health (contamination) risks, and two to social (acceptance) and policy issues. It is unlikely that water management utilities (WMUs) can influence the resolution of all these bottleneck to an equal extent. We hypothesise that those related to issues other than the technical process itself are currently difficult for WMUs to solve. This is due to their rather narrow management focus on wastewater treatment rather than resource recovery. Implementing RRRs successfully will require WMUs to extend their engineering expertise and to become market participants actively engaged with all aspects relevant to the creation of value chains for recovered resources, without losing sight of their primary focus on treating wastewater to meet legal effluent standards.
To implement future water resource recovery facilities (WRFs) that recover multiple resources, WMUs need to perceive themselves as market actors producing goods rather than as utilities managing a fixed budget for cost-effective treatment plant operations. The challenge is to abandon the paradigm of merely operating existing WWTPs and to start perceiving wastewater as a resource that requires management at different levels and investments in research and development in order to reintroduce resources successfully into markets for societal consumption. Value can be created if the interests of all stakeholders, including business partners, end users and policymakers, are integrated into the planning process, and applications with unique selling propositions are found. If a WMU plans the implementation of a technically feasible resource recovery technology, it is recommendable that it analyses in advance whether any of the non-technical bottlenecks presented in this review still need to be tackled. In the future, WMUs could develop a common recovery strategy for selected resources that coordinates efforts to exploit synergies and the advantages of economies of scale. If several WMUs recover the same resource, value-chain development could be facilitated by acting as one supplier, thus increasing their collective market power. This idea has already been put into practice in the Netherlands, where water boards have established the Energy and Raw Materials Factory (Energie en Grondstoffen Fabriek) to act as a collaborative network organisation coordinating the recovery efforts of several WMUs.
The most precious resource contained in municipal wastewater is the water itself. Unlike energy, which can be obtained from multiple sources, it has no alternative origin. Wastewater reuse can provide an important alternative source of fresh water in regions that expect lasting shortages in the future. It should preferably be promoted where it is less energy- and resource-demanding than conventional fresh-water treatment and distribution. It is possible that in the future, stricter effluent-quality regulations will require the elimination of emerging pollutants. Thus, advanced energy-intensive treatment steps could become necessary anyway.205 The resulting higher effluent quality would also increase water reuse opportunities. Anaerobic digestion as a bioenergy production system will only become economically viable if subsidies are made available to ensure its competitiveness with commercial natural-gas supplies.167 This counteracts the development of potentially more sustainable solutions, like the recovery of COD as biomaterials. In addition to the recovery of chemical energy stored in the COD, municipal WWTP effluents contain thermal energy that could recover ten times more energy than the CH4–CHP route and should therefore be considered more prominently in wastewater resource recovery planning to operate a plant carbon neutrally. Fertilizer recovery technologies should aim for the capture of most nutrients. For P recovery, that could mean that it is beneficial to place the recovery unit at the end of the treatment process, as is already the case with sludge incineration ash. In livestock-intensive regions, however, P recovery strategies should focus on manure before municipal wastewater due to the recoverable quantities, as shown in Fig. 4. Ammonium recovery is only recommendable if the process consumes less energy than conventional ammonium production.
However, the supply potentials and bottlenecks presented in this paper should be perceived as challenges rather than obstacles. We believe that successfully implementing wastewater resource recovery requires the proactive management of potential bottlenecks and the finding of partners along a value chain to share the risks associated with pioneering. To achieve the transition from WWTPs to WRFs, resource recovery needs to be considered a strategic goal from the earliest process design and planning stages of new processes. Designing and implementing a WRF requires decisions in fields that are far beyond the traditional responsibilities of WMUs. The scientific community should therefore elaborate the insights into process integration and the decision-support tools needed to help WMUs strategically plan and design WRFs to exploit their vast technological potential and to overcome non-technological bottlenecks.
Conflicts of interest
There are no conflicts to declare.
Acknowledgements
This study was funded by European Union's Horizon 2020 research and innovation programme, under Marie Skłodowska–Curie Grant Agreement no. 676070. It reflects the authors' views alone. The Research Executive Agency of the EU is not responsible for any use that may be made of the information it contains.
References
-
UNEP, Sick water? The central role of wastewater management in sustainable development: a rapid response assessment, ed. E. Corcoran, UNEP/GRID-Arendal, Arendal, Norway, 2010, p. 85 Search PubMed.
- W. Verstraete, P. Van de Caveye and V. Diamantis, Maximum use of resources present in domestic “used water”, Bioresour. Technol., 2009, 100(23), 5537–5545 CrossRef CAS PubMed.
- S. T. Oh, J. R. Kim, G. C. Premier, T. H. Lee, C. Kim and W. T. Sloan, Sustainable wastewater treatment: How might microbial fuel cells contribute, Biotechnol. Adv., 2010, 28(6), 871–881 CrossRef CAS PubMed.
- W. Verstraete and S. E. Vlaeminck, ZeroWasteWater: short-cycling of wastewater resources for sustainable cities of the future, Int. J. Sustainable Dev. World Ecol., 2011, 18(3), 253–264 CrossRef.
- J. S. Guest, S. J. Skerlos, J. L. Barnard, M. B. Beck, G. T. Daigger and H. Hilger,
et al. A New Planning and Design Paradigm to Achieve Sustainable Resource Recovery from Wastewater, Environ. Sci. Technol., 2009, 43(16), 6126–6130 CrossRef CAS PubMed.
- M. C. M. van Loosdrecht and D. Brdjanovic, Anticipating the next century of wastewater treatment, Science, 2014, 344(6191), 1452–1453 CrossRef CAS PubMed.
- J. Ma, Z. Wang, Y. Xu, Q. Wang, Z. Wu and A. Grasmick, Organic matter recovery from municipal wastewater by using dynamic membrane separation process, Chem. Eng. J., 2013, 219, 190–199 CrossRef CAS.
- G. T. Daigger, Evolving Urban Water and Residuals Management Paradigms: Water Reclamation and Reuse, Decentralization, and Resource Recovery, Water Environ. Res., 2009, 81(8), 809–823 CrossRef CAS PubMed.
-
P. Stanchev, V. Vasilaki, J. Dosta and E. Katsou, Measuring the circular economy of water sector three-fold linkage of water, energy and materials, 2017 Search PubMed.
-
H. Bozkurt, K. V. Gernaey and G. Sin, Superstructure-based optimization tool for plant design and retrofitting, in Innovative Wastewater Treatment & Resource Recovery Technologies. IWA Publishing, 2017, pp. 581–597 Search PubMed.
- W.-W. Li, H.-Q. Yu and B. E. Rittmann, Chemistry: Reuse water pollutants, Nature, 2015, 528(7580), 29–31 CrossRef CAS PubMed.
- J. P. van der Hoek, H. de Fooij and A. Struker, Wastewater as a resource: Strategies to recover resources from Amsterdam's wastewater, Resour., Conserv. Recycl., 2016, 113, 53–64 CrossRef.
- D. Puyol, D. J. Batstone, T. Hülsen, S. Astals, M. Peces and J. O. Krömer, Resource Recovery from Wastewater by Biological Technologies: Opportunities, Challenges, and Prospects, Front Microbiol, 2017, 7, 2106 Search PubMed.
-
V. K. Tyagi and S.-L. Lo Energy and Resource Recovery From Sludge, in: Environmental Materials and Waste, Elsevier, 2016, pp. 221–244, Available from: https://linkinghub.elsevier.com/retrieve/pii/B978012803837600010X Search PubMed.
- L. Egle, H. Rechberger, J. Krampe and M. Zessner, Phosphorus recovery from municipal wastewater: An integrated comparative technological, environmental and economic assessment of P recovery technologies, Sci. Total Environ., 2016, 571, 522–542 CrossRef CAS PubMed.
- K. S. Le Corre, E. Valsami-Jones, P. Hobbs and S. A. Parsons, Phosphorus Recovery from Wastewater by Struvite Crystallization: A Review, Crit. Rev. Environ. Sci. Technol., 2009, 39(6), 433–477 CrossRef CAS.
- B. E. Rittmann, B. Mayer, P. Westerhoff and M. Edwards, Capturing the lost phosphorus, Chemosphere, 2011, 84(6), 846–853 CrossRef CAS PubMed.
- D. J. Batstone, T. Hülsen, C. M. Mehta and J. Keller, Platforms for energy and nutrient recovery from domestic wastewater: A review, Chemosphere, 2015, 140, 2–11 CrossRef CAS PubMed.
- P. T. Kelly and Z. He, Nutrients removal and recovery in bioelectrochemical systems: A review, Bioresour. Technol., 2014, 153, 351–360 CrossRef CAS PubMed.
- H. Wang and Z. J. Ren, A comprehensive review of microbial
electrochemical systems as a platform technology, Biotechnol. Adv., 2013, 31(8), 1796–1807 CrossRef CAS PubMed.
- T. Cai, S. Y. Park and Y. Li, Nutrient recovery from wastewater streams by microalgae: Status and prospects, Renewable Sustainable Energy Rev., 2013, 19, 360–369 CrossRef CAS.
- A. N. Bdour, M. R. Hamdi and Z. Tarawneh, Perspectives on sustainable wastewater treatment technologies and reuse options in the urban areas of the Mediterranean region, Desalination, 2009, 237(1–3), 162–174 CrossRef CAS.
- J. Coppens, E. Meers, N. Boon, J. Buysse and S. E. Vlaeminck, Follow the N and P road: High-resolution nutrient flow analysis of the Flanders region as precursor for sustainable resource management, Resour., Conserv. Recycl., 2016, 115, 9–21 CrossRef.
-
Eurostat, Annual freshwater abstraction by source and sector. 2018, Available from: http://appsso.eurostat.ec.europa.eu/nui/show.do?dataset=env_wat_abs&lang=en.
- K. Roest, J. Hofman and M. van Loosdrecht, De Nederlandse watercyclus kan energie opleveren, H2O, 2010, 25/26, 47–50 Search PubMed.
-
WWAP UNWWAP, The United Nations World Water Development Report 2017 - Wastewater: The untapped resource, UNESCO, Paris, 2017 Search PubMed.
- J. Frijns, J. Hofman and M. Nederlof, The potential of (waste)water as energy carrier, Energy Convers. Manage., 2013, 65, 357–363 CrossRef CAS.
-
Eurostat, Supply, transformation and consumption of electricity - annual data, 2018, Available from: http://appsso.eurostat.ec.europa.eu/nui/submitViewTableAction.do.
- A. P. C. Faaij, Bio-energy in Europe: changing technology choices, Energy Policy., 2006, 34(3), 322–342 CrossRef.
-
Eurostat, Supply, transformation and consumption of heat - annual data, 2018, Available from: http://appsso.eurostat.ec.europa.eu/nui/show.do?dataset=nrg_106a&lang=en.
- H. Siegrist, D. Salzgeber, J. Eugster and A. Joss, Anammox brings WWTP closer to energy autarky due to increased biogas production and reduced aeration energy for N-removal, Water Sci. Technol., 2008, 57(3), 383–388 CrossRef CAS PubMed.
- S. Matassa, D. J. Batstone, T. Hülsen, J. Schnoor and W. Verstraete, Can Direct Conversion of Used Nitrogen to New Feed and Protein Help Feed the World?, Environ. Sci. Technol., 2015, 49(9), 5247–5254 CrossRef CAS PubMed.
- M.-K. H. Winkler, M. H. Bennenbroek, F. H. Horstink, M. C. M. van Loosdrecht and G.-J. van de Pol, The biodrying concept: An innovative technology creating energy from sewage sludge, Bioresour. Technol., 2013, 147, 124–129 CrossRef CAS PubMed.
- P. Cornel and C. Schaum, Phosphorus recovery from wastewater: needs, technologies and costs, Water Sci. Technol., 2009, 59(6), 1069–1076 CrossRef CAS PubMed.
-
Eurostat, Total paper and paperboard production, 2018, Available from: https://ec.europa.eu/eurostat/web/products-datasets/-/tag00074.
- S. I. Mussatto and M. van Loosdrecht, Cellulose: a key polymer for a greener, healthier, and bio-based future, Biofuel Res. J., 2016, 3(4), 482–482 CrossRef.
-
C. Ruiken, Influent fijnzeven in RWZI'S, Amersfoort, STOWA, 2010 Search PubMed.
-
C. Visser, I. Odegard, N. Naber, G. C. Bergsma, A. F. van Nieuwenhuijzen and M. H. A. Sanders, Levenscyclusanalyse van grondstoffen uit rioolwater, 2016 Search PubMed.
- C. J. Ruiken, G. Breuer, E. Klaversma, T. Santiago and M. C. M. van Loosdrecht, Sieving wastewater – Cellulose recovery, economic and energy evaluation, Water Res., 2013, 47(1), 43–48 CrossRef CAS PubMed.
- P. L. McCarty, J. Bae and J. Kim, Domestic Wastewater Treatment as a Net Energy Producer–Can This be Achieved?, Environ. Sci. Technol., 2011, 45(17), 7100–7106 CrossRef CAS PubMed.
- I. Baumann and P. Westermann, Microbial Production of Short Chain Fatty Acids from Lignocellulosic Biomass: Current Processes and Market, BioMed Res. Int., 2016, 2016, 1–15 CrossRef PubMed.
- R. Khiewwijit, K. J. Keesman, H. Rijnaarts and H. Temmink, Volatile fatty acids production from sewage organic matter by combined bioflocculation and anaerobic fermentation, Bioresour. Technol., 2015, 193, 150–155 CrossRef CAS PubMed.
- S. N. Pawar and K. J. Edgar, Alginate derivatization: A review of chemistry, properties and applications, Biomaterials, 2012, 33(11), 3279–3305 CrossRef CAS PubMed.
- J. Wan, J. Gu, Q. Zhao and Y. Liu, COD capture: a feasible option towards energy self-sufficient domestic wastewater treatment, Sci. Rep., 2016, 6(1), 1–9 CrossRef PubMed.
-
H. van der Roest, M. van Loosdrecht and E. J. Langkamp Recovery and reuse of alginate from granular Nereda sludge, Water21 Magazine. 2015, 2015 Search PubMed.
-
A. Hogendoorn, J. Hulzebos, W. van Betuw and A. Meindertsma, Stichting Toegepast Onderzoek Waterbeheer, CO2-winning op RWZI's, 2014 Search PubMed.
-
IWA, Wastewater Report 2018: The reuse opportunity, The International Water Association, London, 2018 Search PubMed.
-
X. C. Wang, C. Zhang, X. Ma and L. Luo, Water cycle management: a new paradigm of wastewater reuse and safety control, Springer briefs in water science and technology, Springer, Heidelberg, 2015, p. 98 Search PubMed.
-
PUB, Our Water, Our Future, Singapore's National Water Agency (PUB), 2016 Search PubMed.
-
V. V. Ranade and V. M. Bhandari, Industrial Wastewater Treatment, Recycling, and Reuse: An Overview, in Industrial wastewater treatment, recycling, and reuse. 2014 Search PubMed.
- K. Schopf, J. Judex, B. Schmid and T. Kienberger, Modelling the bioenergy potential of municipal wastewater treatment plants, Water Sci. Technol., 2018, 77(11), 2613–2623 CrossRef CAS PubMed.
- F. Kretschmer, G. Neugebauer, R. Kollmann, M. Eder, F. Zach and A. Zottl,
et al. Resource recovery from wastewater in Austria: wastewater treatment plants as regional energy cells, J. Water Reuse Desalin., 2016, 6(3), 421–429 CrossRef.
- D. Cordell, J.-O. Drangert and S. White, The story of phosphorus: Global food security and food for thought, Glob. Environ. Change, 2009, 19(2), 292–305 CrossRef.
- P. Wilfert, P. S. Kumar, L. Korving, G.-J. Witkamp and M. C. M. van Loosdrecht, The Relevance of Phosphorus and Iron Chemistry to the Recovery of Phosphorus from Wastewater: A Review, Environ. Sci. Technol., 2015, 49(16), 9400–9414 CrossRef CAS PubMed.
- A. Mulder, The quest for sustainable nitrogen removal technologies, Water Sci. Technol., 2003, 48(1), 67–75 CrossRef CAS PubMed.
- Efgf.nl. De Energie- & Grondstoffenfabriek. Energie en Grondstoffen Fabriek. 2019 [cited 2019 May 1], Available from: https://www.efgf.nl/producten.
- M. J. Schelhaas, The wind stability of different silvicultural systems for Douglas-fir in the Netherlands: a model-based approach, Forestry, 2008, 81(3), 399–414 CrossRef.
- S. K. Bhatia and Y.-H. Yang, Microbial production of volatile fatty acids: current status and future perspectives, Rev. Environ. Sci. Bio/Technol., 2017, 16(2), 327–345 CrossRef CAS.
- M.-P. Zacharof and R. W. Lovitt, Complex Effluent Streams as a Potential Source of Volatile Fatty Acids, Waste Biomass Valoriz., 2013, 4(3), 557–581 CrossRef CAS.
- A. Zhang and S.-T. Yang, Engineering Propionibacterium acidipropionici for enhanced propionic acid tolerance and fermentation, Biotechnol. Bioeng., 2009, 104, 4 Search PubMed.
- E. de Jong, A. Higson, P. Walsh and M. Wellisch, Product developments in the bio-based chemicals arena, Biofuels, Bioprod. Biorefin., 2012, 6(6), 606–624 CrossRef CAS.
-
S. Pratt, L.-J. Vandi, D. Gapes, A. Werker, A. Oehmen and B. Laycock, Polyhydroxyalkanoate (PHA) Bioplastics from Organic Waste, in Biorefinery, ed. J.-R. Bastidas-Oyanedel and J. E. Schmidt, Springer International Publishing, Cham, 2019, pp. 615–638, Available from: http://link.springer.com/10.1007/978-3-030-10961-5_26 Search PubMed.
-
European Commission, Proposal for a Regulation of the European Parliament and of the Council on minium requirements for water reuse, 2018 Search PubMed.
-
S. Eslamian, Urban water reuse handbook, CRC Press, Boca Raton London New York, 2016, p. 1141 Search PubMed.
-
Wastewater treatment: advanced processes and technologies, ed. D. G. Rao, Environmental Engineering, CRC Press, Boca Raton, Fla, 2013. p. 365 Search PubMed.
- T. Wintgens, T. Melin, A. Schäfer, S. Khan, M. Muston and D. Bixio,
et al. The role of membrane processes in municipal wastewater reclamation and reuse, Desalination, 2005, 178(1–3), 1–11 CrossRef CAS.
- H. Lee and T. P. Tan, Singapore's experience with reclaimed water: NEWater, Int. J. Water Resour. Dev., 2016, 32(4), 611–621 CrossRef.
- F. Zanetti, G. De Luca and R. Sacchetti, Performance of a full-scale membrane bioreactor system in treating municipal wastewater for reuse purposes, Bioresour. Technol., 2010, 101(10), 3768–3771 CrossRef CAS PubMed.
-
S. Judd, B. Kim and G. Amy, Membrane Bio-reactors, in Biological wastewater treatment: principles, modelling and design, IWA Pub, London, 2008 Search PubMed.
- G. K. Pearce, UF/MF pre-treatment to RO in seawater and wastewater reuse applications: a comparison of energy costs, Desalination, 2008, 222(1–3), 66–73 CrossRef CAS.
-
B. Banjoko and C. M. K. Sridhar, Upgrading Wastewater Treatment Systems for Urban Water Reuse, Urban water reuse handbook, 2016 Search PubMed.
- P. Côté, S. Siverns and S. Monti, Comparison of Membrane-based Solutions for Water Reclamation and Desalination, Desalination, 2005, 182(1), 251–257 CrossRef.
-
G. T. Daigger, New Approaches and Technologies for Wastewater Management, The Bridge, 2008, vol. 382, pp. 38–45 Search PubMed.
-
A. I. Stefanakis, Modern Water Reuse Technologists: Tertiary Membrane and Activated Carbon Filtration, in Urban water reuse handbook, 2016 Search PubMed.
-
R. R. Trussel, in Water reuse: potential for expanding the nation's water supply through reuse of municipal wastewater. National Research Council (U.S.), ed. National Research Council (U.S.), National Academies Press, Washington, D.C, 2012, p. 262 Search PubMed.
- M. P. Ormad, N. Miguel, A. Claver, J. M. Matesanz and J. L. Ovelleiro, Pesticides removal in the process of drinking water production, Chemosphere, 2008, 71(1), 97–106 CrossRef CAS PubMed.
- D. Gerrity, S. Gamage, J. C. Holady, D. B. Mawhinney, O. Quiñones and R. A. Trenholm,
et al. Pilot-scale evaluation of ozone and biological activated carbon for trace organic contaminant mitigation and disinfection, Water Res., 2011, 45(5), 2155–2165 CrossRef CAS PubMed.
- X. Qu, J. Zheng and Y. Zhang, Catalytic ozonation of phenolic wastewater with activated carbon fiber in a fluid bed reactor, J. Colloid Interface Sci., 2007, 309(2), 429–434 CrossRef CAS PubMed.
- J. Reungoat, B. I. Escher, M. Macova, F. X. Argaud, W. Gernjak and J. Keller, Ozonation and biological activated carbon filtration of wastewater treatment plant effluents, Water Res., 2012, 46(3), 863–872 CrossRef CAS PubMed.
- X.-J. Gai and H.-S. Kim, The role of powdered activated carbon in enhancing the performance of membrane systems for water treatment, Desalination, 2008, 225(1–3), 288–300 CrossRef CAS.
- H.-S. Kim, S. Takizawa and S. Ohgaki, Application of microfiltration systems coupled with powdered activated carbon to river water treatment, Desalination, 2007, 202(1–3), 271–277 CrossRef CAS.
- O. Sagbo, Y. Sun, A. Hao and P. Gu, Effect of PAC addition on MBR process for drinking water treatment, Sep. Purif. Technol., 2008, 58(3), 320–327 CrossRef CAS.
-
F. Çeçen, Water and Wastewater Treatment: Historical Perspective of Activated Carbon Adsorption and its Integration with Biological Processes, in Activated carbon for water and wastewater treatment: integration of adsorption and biological treatment, 2012 Search PubMed.
- I. Oller, S. Malato and J. A. Sánchez-Pérez, Combination of Advanced Oxidation Processes and biological treatments for wastewater decontamination—A review, Sci. Total Environ., 2011, 409(20), 4141–4166 CrossRef CAS PubMed.
- M. Petrovic, J. Radjenovic and D. Barcelo, Advanced oxidation processes (AOPs) applied for wastewater and drinking water treatment. Elimination of pharmaceuticals, Holistic Approach Environ., 2011, 1(2), 63–74 CAS.
- C. Comninellis, A. Kapalka, S. Malato, S. A. Parsons, I. Poulios and D. Mantzavinos, Advanced oxidation processes for water treatment: Advances and trends for R&D, J. Chem. Technol. Biotechnol., 2008, 83(6), 769–776 CrossRef CAS.
- M. A. Oturan and J.-J. Aaron, Advanced Oxidation Processes in Water/Wastewater Treatment: Principles and Applications. A Review, Crit. Rev. Environ. Sci. Technol., 2014, 44(23), 2577–2641 CrossRef CAS.
- J. L. Wang and L. J. Xu, Advanced Oxidation Processes for Wastewater Treatment: Formation of Hydroxyl Radical and Application, Crit. Rev. Environ. Sci. Technol., 2012, 42(3), 251–325 CrossRef CAS.
- T. E. Agustina, H. M. Ang and V. K. Vareek, A review of synergistic effect of photocatalysis and ozonation on wastewater treatment, J. Photochem. Photobiol., C, 2005, 6(4), 264–273 CrossRef CAS.
- M. Brahmi, N. H. Belhadi, H. Hamdi and A. Hassen, Modeling of secondary treated wastewater disinfection by UV irradiation: Effects of suspended solids content, J. Environ. Sci., 2010, 22(8), 1218–1224 CrossRef CAS.
-
W. J. Masschelein and R. G. Rice, Ultraviolet light in water and wastewater sanitation, Lewis, Boca Raton, 2002, p. 174 Search PubMed.
- M. Guo, H. Hu and W. Liu, Preliminary investigation on safety of post-UV disinfection of wastewater: bio-stability in laboratory-scale simulated reuse water pipelines, Desalination, 2009, 239(1–3), 22–28 CrossRef CAS.
-
J. Jegatheesan, J. Virkutyte, L. Shu, J. Allen, Y. Wang and E. Searston, et al. Removal of Lower-Molecular-Weight Substances from Water and Wastewater: Challenges and Solutions, in Wastewater treatment: advanced processes and technologies, 2013 Search PubMed.
-
Z. Shareefdeen, A. Elkamel and S. Kandhro, Modern Water Reuse Technologies Membrane Bioreactors, in Urban water reuse handbook, 2016 Search PubMed.
- V. Lazarova, P. Savoye, M. L. Janex, E. R. Blatchley and M. Pommepuy, Advanced Wastewater Disinfection Technologies: State of the Art and Perspectives, Water Sci. Technol., 1999, 40(4–5), 203–213 CrossRef CAS.
- L. Yi, W. Jiao, X. Chen and W. Chen, An overview of reclaimed water reuse in China, J. Environ. Sci., 2011, 23(10), 1585–1593 CrossRef.
- X. Garcia and D. Pargament, Reusing wastewater to cope with water scarcity: Economic, social and environmental considerations for decision-making, Resour., Conserv. Recycl., 2015, 101, 154–166 CrossRef.
-
V. Lazarova, T. Asano, A. Bahri and J. Anderson, Milestones in water reuse: the best success stories, IWA Publ, London, 2013, p. 375 Search PubMed.
- E. Van Houtte and J. Verbauwhede, Long-time membrane experience at Torreele's water re-use facility in Belgium, Desalin. Water Treat., 2013, 51(22–24), 4253–4262 CrossRef CAS.
- J. Rook, S. Hillegers and J. P. van der Hoek, From which sources does Amsterdam produce its drinking water after 2020? (in Dutch: Waar haalt Amsterdam na 2020 drinkwater vandaan?), H2O, 2013, 46(7–8), 40–41 Search PubMed.
- F. Pedrero, I. Kalavrouziotis, J. J. Alarcón, P. Koukoulakis and T. Asano, Use of treated municipal wastewater in irrigated agriculture—Review of some practices in Spain and Greece, Agric. Water Manag., 2010, 97(9), 1233–1241 CrossRef.
- J. Fawell, K. Le Corre and P. Jeffrey, Common or independent? The debate over regulations and standards for water reuse in Europe, Int. J. Water Resour. Dev., 2016, 32(4), 559–572 CrossRef.
-
EIA USEIA, International Energy Outlook 2013, 2013 Search PubMed.
- X. Wang, P. L. McCarty, J. Liu, N.-Q. Ren, D.-J. Lee and H.-Q. Yu,
et al. Probabilistic evaluation of integrating resource recovery into wastewater treatment to improve environmental sustainability, Proc. Natl. Acad. Sci. U. S. A., 2015, 112(5), 1630–1635 CrossRef CAS PubMed.
- E. S. Heidrich, T. P. Curtis and J. Dolfing, Determination of the Internal Chemical Energy of Wastewater, Environ. Sci. Technol., 2011, 45(2), 827–832 CrossRef CAS PubMed.
- W. Rulkens, Sewage Sludge as a Biomass Resource for the Production of Energy: Overview and Assessment of the Various Options†, Energy Fuels, 2008, 22(1), 9–15 CrossRef CAS.
- X. Ma, X. Xue, A. González-Mejía, J. Garland and J. Cashdollar, Sustainable Water Systems for the City of Tomorrow—A Conceptual Framework, Sustainability, 2015, 7(9), 12071–12105 CrossRef CAS.
- K. Rabaey and R. A. Rozendal, Microbial electrosynthesis — revisiting the electrical route for microbial production, Nat. Rev. Microbiol., 2010, 8(10), 706–716 CrossRef CAS PubMed.
- B. E. Logan and K. Rabaey, Conversion of Wastes into Bioelectricity and Chemicals by Using Microbial Electrochemical Technologies, Science, 2012, 337(6095), 686–690 CrossRef CAS PubMed.
- H. N. Chang, N.-J. Kim, J. Kang and C. M. Jeong, Biomass-derived volatile fatty acid platform for fuels and chemicals, Biotechnol. Bioprocess Eng., 2010, 15(1), 1–10 CrossRef CAS.
- P. Manara and A. Zabaniotou, Towards sewage sludge based biofuels via thermochemical conversion – A review, Renewable Sustainable Energy Rev., 2012, 16(5), 2566–2582 CrossRef CAS.
-
S. Sohi, E. Loez-Capel, E. Krull and R. Bol, CSIRO Land and Water Science Report: Biochar's roles in soil and climate change: A review of research needs, 2009 Search PubMed.
- M. Goto, T. Nada, A. Kodama and T. Hirose, Kinetic Analysis for Destruction of Municipal Sewage Sludge and Alcohol Distillery Wastewater by Supercritical Water Oxidation, Ind. Eng. Chem. Res., 1999, 38(5), 1863–1865 CrossRef CAS.
- O. Yakaboylu, J. Harinck, K. Smit and W. de Jong, Supercritical Water Gasification of Biomass: A Literature and Technology Overview, Energies, 2015, 8(2), 859–894 CrossRef CAS.
- L. Qian, S. Wang, D. Xu, Y. Guo, X. Tang and L. Wang, Treatment of municipal sewage sludge in supercritical water: A review, Water Res., 2016, 89, 118–131 CrossRef CAS PubMed.
- W. S. Lee, A. S. M. Chua, H. K. Yeoh and G. C. Ngoh, A review of the production and applications of waste-derived volatile fatty acids, Chem. Eng. J., 2014, 235, 83–99 CrossRef CAS.
- H.-S. Lee, W. F. J. Vermaas and B. E. Rittmann, Biological hydrogen production: prospects and challenges, Trends Biotechnol., 2010, 28(5), 262–271 CrossRef CAS PubMed.
- E. E. Muller, A. R. Sheik and P. Wilmes, Lipid-based biofuel production from wastewater, Curr. Opin. Biotechnol., 2014, 30, 9–16 CrossRef CAS PubMed.
- I. K. Muniraj, S. K. Uthandi, Z. Hu, L. Xiao and X. Zhan, Microbial lipid production from renewable and waste materials for second-generation biodiesel feedstock, Environ. Technol. Rev., 2015, 4(1), 1–16 CrossRef CAS.
-
R. Khiewwijit, H. H. M. Rijnaarts, K. J. Keesman and B. G. Temmink, New wastewater treatment concepts towards energy saving and resource recovery, 2016 [cited 2018 Sep 26], Available from: http://edepot.wur.nl/369526 Search PubMed.
- J. B. K. Park, R. J. Craggs and A. N. Shilton, Wastewater treatment high rate algal ponds for biofuel production, Bioresour. Technol., 2011, 102(1), 35–42 CrossRef CAS PubMed.
- H. Gao, Y. D. Scherson and G. F. Wells, Towards energy neutral wastewater treatment: methodology and state of the art, Environ. Sci.: Processes Impacts, 2014, 16(6), 1223–1246 RSC.
- S. A. Tassou, Heat recovery from sewage effluents using heat pumps, Heat Recovery Syst. CHP, 1988, 8(2), 141–148 CrossRef CAS.
- U. Schröder, From Wastewater to Hydrogen: Biorefineries Based on Microbial Fuel-Cell Technology, ChemSusChem, 2008, 1(4), 281–282 CrossRef PubMed.
- P. Clauwaert and W. Verstraete, Methanogenesis in membraneless microbial electrolysis cells, Appl. Microbiol. Biotechnol., 2009, 82(5), 829–836 CrossRef CAS PubMed.
- S. Cheng, D. Xing, D. F. Call and B. E. Logan, Direct Biological Conversion of Electrical Current into Methane by Electromethanogenesis, Environ. Sci. Technol., 2009, 43(10), 3953–3958 CrossRef CAS PubMed.
- M. Villano, S. Scardala, F. Aulenta and M. Majone, Carbon and nitrogen removal and enhanced methane production in a microbial electrolysis cell, Bioresour. Technol., 2013, 130, 366–371 CrossRef CAS PubMed.
-
K. Hartley, Tuning biological nutrient removal plants, IWA Publ, London, 2013, p. 246 Search PubMed.
- K.-J. Chae and J. Kang, Estimating the energy independence of a municipal wastewater treatment plant incorporating green energy resources, Energy Convers. Manage., 2013, 75, 664–672 CrossRef.
- W. Mo and Q. Zhang, Energy–nutrients–water nexus: Integrated resource recovery in municipal wastewater treatment plants, J. Environ. Manage., 2013, 127, 255–267 CrossRef CAS PubMed.
-
F. Schmid, Sewage Water: Interesting Source for Heat Pumps, Zürich, 2008, Available from: https://heatpumpingtechnologies.org/publications/sewage-water-interesting-heat-source-forheat-pumps-and-chillers/ Search PubMed.
- V. Berger, A. Niemann, T. Frehmann and H. Brockmann, Advanced energy recovery strategies for wastewater treatment plants and sewer systems using small hydropower, Water Utility Journal, 2013, 5, 15–24 Search PubMed.
- C. Power, A. McNabola and P. Coughlan, Development of an evaluation method for hydropower energy recovery in wastewater treatment plants: Case studies in Ireland and the UK, Sustain. Energy Technol. Assessments, 2014, 7, 166–177 CrossRef.
- A. McNabola, P. Coughlan, L. Corcoran, C. Power, A. Prysor Williams and I. Harris,
et al. Energy recovery in the water industry using micro-hydropower: an opportunity to improve sustainability, Water Policy, 2014, 16(1), 168–183 CrossRef.
- Y. Gu, Y. Li, X. Li, P. Luo, H. Wang and X. Wang,
et al. Energy Self-sufficient Wastewater Treatment Plants: Feasibilities and Challenges, Energy Procedia., 2017, 105, 3741–3751 CrossRef.
- D. Ganora, A. Hospido, J. Husemann, J. Krampe, C. Loderer and S. Longo,
et al. Opportunities to improve energy use in urban wastewater treatment: a European scale analysis, Environ. Res. Lett., 2019, 14, 4 Search PubMed.
- X. Hao, R. Liu and X. Huang, Evaluation of the potential for operating carbon neutral WWTPs in China, Water Res., 2015, 87, 424–431 CrossRef CAS PubMed.
- A. R. Sheik, E. E. L. Muller and P. Wilmes, A hundred years of activated sludge: time for a rethink, Front Microbiol, 2014, 5, 47 Search PubMed.
-
Phosphorus and nitrogen removal from municipal wastewater: principles and practice, ed. R. Sedlak, 2nd edn, Lewis Publishers, Chelsea, Mich., 1991, p. 240 Search PubMed.
- M. Xie, H. K. Shon, S. R. Gray and M. Elimelech, Membrane-based processes for wastewater nutrient recovery: Technology, challenges, and future direction, Water Res., 2016, 89, 210–221 CrossRef CAS PubMed.
- C. M. Mehta, W. O. Khunjar, V. Nguyen, S. Tait and D. J. Batstone, Technologies to Recover Nutrients from Waste Streams: A Critical Review, Crit. Rev. Environ. Sci. Technol., 2015, 45(4), 385–427 CrossRef.
- C. J. Van Leeuwen, S. H. A. Koop and R. M. A. Sjerps, City Blueprints: baseline assessments of water management and climate change in 45 cities, Environ. Dev. Sustain., 2016, 18(4), 1113–1128 CrossRef.
- H. Kirchmann, G. Börjesson, T. Kätterer and Y. Cohen, From agricultural use of sewage sludge to nutrient extraction: A soil science outlook, Ambio, 2017, 46(2), 143–154 CrossRef CAS PubMed.
- Y. Jaffer, T. A. Clark, P. Pearce and S. A. Parsons, Potential phosphorus recovery by struvite formation, Water Res., 2002, 36(7), 1834–1842 CrossRef CAS PubMed.
- Y. Zhang, E. Desmidt, A. Van Looveren, L. Pinoy, B. Meesschaert and B. Van der Bruggen, Phosphate Separation and Recovery from Wastewater by Novel Electrodialysis, Environ. Sci. Technol., 2013, 47(11), 5888–5895 CrossRef CAS PubMed.
- E. V. Münch and K. Barr, Controlled struvite crystallisation for removing phosphorus from anaerobic digester sidestreams, Water Res., 2001, 35(1), 151–159 CrossRef.
- L. Shu, P. Schneider, V. Jegatheesan and J. Johnson, An economic evaluation of phosphorus recovery as struvite from digester supernatant, Bioresour. Technol., 2006, 97(17), 2211–2216 CrossRef CAS PubMed.
- J. Lin, N. Chen and Y. Pan, Arsenic incorporation in synthetic struvite (NH4MgPO4.6H2O): a synchrotron XAS and single-crystal EPR study, Environ. Sci. Technol., 2013, 47(22), 12728–12735 CrossRef CAS PubMed.
- P. Wilfert, A. I. Dugulan, K. Goubitz, L. Korving, G. J. Witkamp and M. C. M. Van Loosdrecht, Vivianite as the main phosphate mineral in digested sewage sludge and its role for phosphate recovery, Water Res., 2018, 144, 312–321 CrossRef CAS PubMed.
- V. Réveillé, L. Mansuy, É. Jardé and É. Garnier-Sillam, Characterisation of sewage sludge-derived organic matter: lipids and humic acids, Org. Geochem., 2003, 34(4), 615–627 CrossRef.
- H. Li, Y. Jin and Y. Nie, Application of alkaline treatment for sludge decrement and humic acid recovery, Bioresour. Technol., 2009, 100(24), 6278–6283 CrossRef CAS PubMed.
- D. Woolf, J. E. Amonette, F. A. Street-Perrott, J. Lehmann and S. Joseph, Sustainable biochar to mitigate global climate change, Nat. Commun., 2010, 1(5), 1–9 Search PubMed.
- Y. N. Chun, D. W. Ji and K. Yoshikawa, Pyrolysis and gasification characterization of sewage sludge for high quality gas and char production, J. Mech. Sci. Technol., 2013, 27(1), 263–272 CrossRef.
- K. A. Spokas, Impact of biochar field aging on laboratory greenhouse gas production potentials, GCB Bioenergy, 2013, 5(2), 165–176 CrossRef CAS.
- S. Jeffery, F. G. A. Verheijen, M. van der Velde and A. C. Bastos, A quantitative review of the effects of biochar application to soils on crop productivity using meta-analysis, Agric., Ecosyst. Environ., 2011, 144(1), 175–187 CrossRef.
-
C. Korzenowski, M. A. S. Rodrigues and J. Zoppas Ferreira, Electrodialysis for the Recovery of Hexavalent Chromium Solutions, in Electrodialysis and Water Reuse, ed. A. M. Bernardes, M. A. Siqueira Rodrigues and J. Zoppas Ferreira, Springer, 2014, pp. 111–118 Search PubMed.
- J. Elser and E. Bennett, A broken biogeochemical cycle: Phosphorus cycle, Nature, 2011, 478(7367), 29–31 CrossRef CAS PubMed.
-
M. Henze and Y. Comeau Wastewater Characterization, in Biological wastewater treatment: principles, modelling and design, IWA Pub, London, 2008 Search PubMed.
- P. Kuntke, K. M. Śmiech, H. Bruning, G. Zeeman, M. Saakes and T. H. J. A. Sleutels,
et al. Ammonium recovery and energy production from urine by a microbial fuel cell, Water Res., 2012, 46(8), 2627–2636 CrossRef CAS PubMed.
- J. A. Wilsenach, M. Maurer, T. A. Larsen and M. C. M. van Loosdrecht, From waste treatment to integrated resource management, Water Sci. Technol., 2003, 48(1), 1–9 CrossRef CAS PubMed.
- J. P. Van der Hoek, A. Struker and J. E. M. de Danschutter, Amsterdam as a sustainable European metropolis: Integration of water, energy and material flows, Urban Water J., 2015, 14(1), 61–68 CrossRef.
- Y. Chen, S. Jiang, H. Yuan, Q. Zhou and G. Gu, Hydrolysis and acidification of waste activated sludge at different pHs, Water Res., 2007, 41(3), 683–689 CrossRef CAS PubMed.
- M. T. Agler, B. A. Wrenn, S. H. Zinder and L. T. Angenent, Waste to bioproduct conversion with undefined mixed cultures: the carboxylate platform, Trends Biotechnol., 2011, 29(2), 70–78 CrossRef CAS PubMed.
- Z. Ji, G. Chen and Y. Chen, Effects of waste activated sludge and surfactant addition on primary sludge hydrolysis and short-chain fatty acids accumulation, Bioresour. Technol., 2010, 101(10), 3457–3462 CrossRef CAS PubMed.
- S. Longo, E. Katsou, S. Malamis, N. Frison, D. Renzi and F. Fatone, Recovery of volatile fatty acids from fermentation of sewage sludge in municipal wastewater treatment plants, Bioresour. Technol., 2015, 175, 436–444 CrossRef CAS PubMed.
- W. Jie, Y. Peng, N. Ren and B. Li, Volatile fatty acids (VFAs) accumulation and microbial community structure of excess sludge (ES) at different pHs, Bioresour. Technol., 2014, 152, 124–129 CrossRef CAS PubMed.
- R. Kleerebezem, B. Joosse, R. Rozendal and M. C. M. Van Loosdrecht, Anaerobic digestion without biogas?, Rev. Environ. Sci. Bio/Technol., 2015, 14(4), 787–801 CrossRef CAS.
- T. I. M. Grootscholten, K. J. J. Steinbusch, H. V. M. Hamelers and C. J. N. Buisman, Chain elongation of acetate and ethanol in an upflow anaerobic filter for high rate MCFA production, Bioresour. Technol., 2013, 135, 440–445 CrossRef CAS PubMed.
- A. Alloul, R. Ganigué, M. Spiller, F. Meerburg, C. Cagnetta and K. Rabaey,
et al. Capture–Ferment–Upgrade: A Three-Step Approach for the Valorization of Sewage Organics as Commodities, Environ. Sci. Technol., 2018, 52(12), 6729–6742 CrossRef CAS PubMed.
- B. Laycock, P. Halley, S. Pratt, A. Werker and P. Lant, The chemomechanical properties of microbial polyhydroxyalkanoates, Prog. Polym. Sci., 2013, 38(3–4), 536–583 CrossRef CAS.
- H. Moralejo-Gárate, R. Kleerebezem, A. Mosquera-Corral, J. L. Campos, T. Palmeiro-Sánchez and M. C. M. van Loosdrecht, Substrate versatility of polyhydroxyalkanoate producing glycerol grown bacterial enrichment culture, Water Res., 2014, 66, 190–198 CrossRef PubMed.
- C. Fernández-Dacosta, J. A. Posada, R. Kleerebezem, M. C. Cuellar and A. Ramirez, Microbial community-based polyhydroxyalkanoates (PHAs) production from wastewater: Techno-economic analysis and ex-ante environmental assessment, Bioresour. Technol., 2015, 185, 368–377 CrossRef PubMed.
-
J. van Tamis and M. C. M. van Loosdrecht, Resource recovery from organic waste streams by microbial enrichment cultures, 2015 Search PubMed.
- L. Leng, P. Yang, Y. Mao, Z. Wu, T. Zhang and P.-H. Lee, Thermodynamic and physiological study of caproate and 1,3-propanediol co-production through glycerol fermentation and fatty acids chain elongation, Water Res., 2017, 114, 200–209 CrossRef CAS PubMed.
- C. M. Spirito, H. Richter, K. Rabaey, A. J. Stams and L. T. Angenent, Chain elongation in anaerobic reactor microbiomes to recover resources from waste, Curr. Opin. Biotechnol., 2014, 27, 115–122 CrossRef CAS PubMed.
- K. J. J. Steinbusch, H. V. M. Hamelers, C. M. Plugge and C. J. N. Buisman, Biological formation of caproate and caprylate from acetate: fuel and chemical production from low grade biomass, Energy Environ. Sci., 2011, 4(1), 216–224 RSC.
- Y. Liu and J.-H. Tay, The essential role of hydrodynamic shear force in the formation of biofilm and granular sludge, Water Res., 2002, 36(7), 1653–1665 CrossRef CAS PubMed.
- M. K. de Kreuk and M. C. M. van Loosdrecht, Selection of slow growing organisms as a means for improving aerobic granular sludge stability, Water Sci. Technol., 2004, 49(11–12), 9–17 CrossRef CAS PubMed.
- S. Felz, S. Al-Zuhairy, O. A. Aarstad, M. C. M. van Loosdrecht and Y. M. Lin, Extraction of Structural Extracellular Polymeric Substances from Aerobic Granular Sludge, J. Visualized Exp., 2016,(115) Search PubMed Available from: http://www.jove.com/video/54534/extraction-structural-extracellular-polymeric-substances-from-aerobic.
- K. Y. Lee and D. J. Mooney, Alginate: Properties and biomedical applications, Prog. Polym. Sci., 2012, 37(1), 106–126 CrossRef CAS PubMed.
- J.-S. Yang, Y.-J. Xie and W. He, Research progress on chemical modification of alginate: A review, Carbohydr. Polym., 2011, 84(1), 33–39 CrossRef CAS.
-
K. I. Draget, Alginates, in Handbook of hydrocolloids, 2nd edn, 2009, pp. 807–828 Search PubMed.
-
S. Tseggai, Structural Composite Architecture Made from Waste - Material Aware and Circular Design inspired by Nature's Strategies, Master ThesisDelft University of Technology, 2016 Search PubMed.
- S. Matassa, N. Boon, I. Pikaar and W. Verstraete, Microbial protein: future sustainable food supply route with low environmental footprint, Microb. Biotechnol., 2016, 9(5), 568–575 CrossRef PubMed.
- P. Wilfert, A. Mandalidis, A. I. Dugulan, K. Goubitz, L. Korving and H. Temmink,
et al., Vivianite as an important iron phosphate precipitate in sewage treatment plants, Water Res., 2016, 104, 449–460 CrossRef CAS PubMed.
- Wetsul.nl. ViviMag [Internet]. 2019 [cited 2019 May 1], Available from: https://www.wetsus.nl/vivimag.
- T. Prot, V. H. Nguyen, P. Wilfert, A. I. Dugulan, K. Goubitz and D. J. De Ridder,
et al. Magnetic separation and characterization of vivianite from digested sewage sludge, Sep. Purif. Technol., 2019, 224, 564–579 CrossRef CAS.
- NSF, NSF, U.S. Environmental Protection Agency, U.S. Department of Energy. Energy-Positive Water Resource Recovery Workshop Report. Arlington, Virginia, USA, 2015.
- A. A. Zorpas, Sustainable waste management through end-of-waste criteria development, Environ. Sci. Pollut. Res., 2016, 23(8), 7376–7389 CrossRef PubMed.
-
H. Saveyn and P. Eder, Institute for Prospective Technological Studies. End-of-waste criteria for biodegradable waste subjected to biological treatment (compost & digestate): technical proposals, Publications Office, Luxembourg, 2014 [cited 2019 Dec 24]. Available from: http://dx.publications.europa.eu/10.2788/6295.
- M. Kacprzak, E. Neczaj, K. Fijałkowski, A. Grobelak, A. Grosser and M. Worwag,
et al., Sewage sludge disposal strategies for sustainable development, Environ. Res., 2017, 156, 39–46 CrossRef CAS PubMed.
-
A. Dereszewska and S. Cytawa, Sustainability considerations in the operation of Wastewater Treatment Plant ‘Swarzewo’, ed. M. Filipowicz, M. Dudek, T. Olkuski and K. StyszkoE3S, Web Conf., 2016, vol. 10, p. 00014 Search PubMed.
- S. Hukari, L. Hermann and A. Nättorp, From wastewater to fertilisers — Technical overview and critical review of European legislation governing phosphorus recycling, Sci. Total Environ., 2016, 542, 1127–1135 CrossRef CAS PubMed.
-
V. A. Yangali Quintanilla, Rejection of emerging organic contaminants by nanofiltration and reverse osmosis membranes: effects of fouling, modelling and water reuse, CRC Press/Balkema, Leiden, 2010, p. 201 Search PubMed.
- M. Zhou, J. Yang, H. Wang, T. Jin, D. Xu and T. Gu, Microbial fuel cells and microbial electrolysis cells for the production of bioelectricity and biomaterials, Environ. Technol., 2013, 34(13–14), 1915–1928 CrossRef CAS PubMed.
- W.-W. Li, H.-Q. Yu and Z. He, Towards sustainable wastewater treatment by using microbial fuel cells-centered technologies, Energy Environ. Sci., 2013, 7(3), 911–924 RSC.
- M. Villano, F. Aulenta, C. Ciucci, T. Ferri, A. Giuliano and M. Majone, Bioelectrochemical reduction of CO2 to CH4 via direct and indirect extracellular electron transfer by a hydrogenophilic methanogenic culture, Bioresour. Technol., 2010, 101(9), 3085–3090 CrossRef CAS PubMed.
- J. Lin, N. Chen and Y. Pan, Arsenic Incorporation in Synthetic Struvite (NH4MgPO4·6H2O): A Synchrotron XAS and Single-Crystal EPR Study, Environ. Sci. Technol., 2013, 47(22), 12728–12735 CrossRef CAS PubMed.
-
Eurostat, Gas prices for household consumers - bi-annual data (from 2007 onwards), 2019, Available from: http://appsso.eurostat.ec.europa.eu/nui/show.do?dataset=nrg_pc_202.
-
Eurostat, Electricity prices (including taxes) for household consumers, first half 2019, 2019, Available from: https://ec.europa.eu/eurostat/statistics-explained/index.php/Electricity_price_statistics.
-
FAO, World fertilizer trends and outlook to 2018, Food & Agriculture Organization of United Nations, Rome, 2015 Search PubMed.
- Y. Zhou and R. S. J. Tol, Evaluating the costs of desalination and water transport, Water Resour. Res., 2005, 41(3), 1–10 CrossRef.
- S. B. Grant, J.-D. Saphores, D. L. Feldman, A. J. Hamilton, T. D. Fletcher and P. L. M. Cook,
et al., Taking the “Waste” Out of “Wastewater” for Human Water Security and Ecosystem Sustainability, Science, 2012, 337(6095), 681–686 CrossRef CAS PubMed.
- S. Lavrnić, M. Zapater-Pereyra and M. L. Mancini, Water Scarcity and Wastewater Reuse Standards in Southern Europe: Focus on Agriculture, Water, Air, Soil Pollut., 2017, 228(7), 1–12 CrossRef.
- L. Høibye, J. Clauson-Kaas, H. Wenzel, H. F. Larsen, B. N. Jacobsen and O. Dalgaard, Sustainability assessment of advanced wastewater treatment technologies, Water Sci. Technol., 2008, 58(5), 963–968 CrossRef PubMed.
- D. H. Kim, A review of desalting process techniques and economic analysis of the recovery of salts from retentates, Desalination, 2011, 270(1–3), 1–8 CrossRef CAS.
- H. W. H. Menkveld and E. Broeders, Recovery of ammonium from digestate as fertilizer, Water Pract. Tech., 2017, 12(3), 514–519 CrossRef.
- Y. Lin, M. Guo, N. Shah and D. C. Stuckey, Economic and environmental evaluation of nitrogen removal and recovery methods from wastewater, Bioresour. Technol., 2016, 215, 227–238 CrossRef CAS PubMed.
- E. Agrafioti, G. Bouras, D. Kalderis and E. Diamadopoulos, Biochar production by sewage sludge pyrolysis, J. Anal. Appl. Pyrolysis, 2013, 101, 72–78 CrossRef CAS.
- B. D. Martin, S. A. Parsons and B. Jefferson, Removal and recovery of phosphate from municipal wastewaters using a polymeric anion exchanger bound with hydrated ferric oxide nanoparticles, Water Sci. Technol., 2009, 60(10), 2637–2645 CrossRef CAS PubMed.
- S. Donatello and C. R. Cheeseman, Recycling and recovery routes for incinerated sewage sludge ash (ISSA): A review, Waste Manage., 2013, 33(11), 2328–2340 CrossRef CAS PubMed.
- P. Piekema and A. Giesen, Phosphate Recovery by the Crystallisation Process: Experience and Developments, Environ. Technol., 2001, 1–11 Search PubMed.
- G. Zhang, Q. Zhao, Y. Jiao, K. Wang, D.-J. Lee and N. Ren, Efficient electricity generation from sewage sludge using biocathode microbial fuel cell, Water Res., 2012, 46(1), 43–52 CrossRef CAS PubMed.
- J. Werther and T. Ogada, Sewage sludge combustion, Prog. Energy Combust. Sci., 1999, 25(1), 55–116 CrossRef CAS.
- Y. D. Scherson, G. F. Wells, S.-G. Woo, J. Lee, J. Park and B. J. Cantwell,
et al., Nitrogen removal with energy recovery through N2O decomposition, Energy Environ. Sci., 2013, 6(1), 241–248 RSC.
- V. K. Tyagi and S.-L. Lo, Microwave irradiation: A sustainable way for sludge treatment and resource recovery, Renewable Sustainable Energy Rev., 2013, 18, 288–305 CrossRef CAS.
- C. Pastore, A. Lopez, V. Lotito and G. Mascolo, Biodiesel from dewatered wastewater sludge: A two-step process for a more advantageous production, Chemosphere, 2013, 92(6), 667–673 CrossRef CAS PubMed.
- W. Liu, S. Huang, A. Zhou, G. Zhou, N. Ren and A. Wang,
et al., Hydrogen generation in microbial electrolysis cell feeding with fermentation liquid of waste activated sludge, Int. J. Hydrogen Energy, 2012, 37(18), 13859–13864 CrossRef CAS.
- J. Mata-Alvarez, J. Dosta, M. S. Romero-Güiza, X. Fonoll, M. Peces and S. Astals, A critical review on anaerobic co-digestion achievements between 2010 and 2013, Renewable Sustainable Energy Rev., 2014, 36, 412–427 CrossRef CAS.
- H. Ozgun, R. K. Dereli, M. E. Ersahin, C. Kinaci, H. Spanjers and J. B. van Lier, A review of anaerobic membrane bioreactors for municipal wastewater treatment: Integration options, limitations and expectations, Sep. Purif. Technol., 2013, 118, 89–104 CrossRef CAS.
-
D. J. Batstone and P. D. Jensen, Anaerobic Processes, in Treatise on Water Science, Elsevier, 2011, pp. 615–639, Available from: http://linkinghub.elsevier.com/retrieve/pii/B978044453199500097X Search PubMed.
- K. Lutchmiah, A. R. D. Verliefde, K. Roest, L. C. Rietveld and E. R. Cornelissen, Forward osmosis for application in wastewater treatment: A review, Water Res., 2014, 58, 179–197 CrossRef CAS PubMed.
- R. Nerenberg, The membrane-biofilm reactor (MBfR) as a counter-diffusional biofilm process, Curr. Opin. Biotechnol., 2016, 38, 131–136 CrossRef CAS PubMed.
- B. van der Bruggen, Integrated Membrane Separation Processes for Recycling of Valuable Wastewater Streams: Nanofiltration, Membrane Distillation, and Membrane Crystallizers Revisited, Ind. Eng. Chem. Res., 2013, 52(31), 10335–10341 CrossRef CAS.
-
Membrane technology for water and wastewater treatment, energy and environment, ed. A. F. Ismail and T. Matsuura, Sustainable water developments, CRC Press, Boca Raton London New York Leiden, 2016, p. 328 Search PubMed.
- L. Rizzo, A. Della Sala, A. Fiorentino and G. Li Puma, Disinfection of urban wastewater by solar driven and UV lamp – TiO2 photocatalysis: Effect on a multi drug resistant Escherichia coli strain, Water Res., 2014, 53, 145–152 CrossRef CAS PubMed.
- C. Noutsopoulos, E. Koumaki, D. Mamais, M.-C. Nika, A. A. Bletsou and N. S. Thomaidis, Removal of endocrine disruptors and non-steroidal anti-inflammatory drugs through wastewater chlorination: The effect of pH, total suspended solids and humic acids and identification of degradation by-products, Chemosphere, 2015, 119, S109–S114 CrossRef CAS PubMed.
- F. L. Rosario-Ortiz, E. C. Wert and S. A. Snyder, Evaluation of UV/H2O2 treatment for the oxidation of pharmaceuticals in wastewater, Water Res., 2010, 44(5), 1440–1448 CrossRef CAS PubMed.
- S. Ahmed, M. G. Rasul, W. N. Martens, R. Brown and M. A. Hashib, Advances in Heterogeneous Photocatalytic Degradation of Phenols and Dyes in Wastewater: A Review, Water, Air, Soil Pollut., 2011, 215(1–4), 3–29 CrossRef CAS.
- A. Babuponnusami and K. Muthukumar, A review on Fenton and improvements to the Fenton process for wastewater treatment, J. Environ. Chem. Eng., 2014, 2(1), 557–572 CrossRef CAS.
- M. Dular, T. Griessler-Bulc, I. Gutierrez-Aguirre, E. Heath, T. Kosjek and A. Krivograd Klemenčič,
et al., Use of hydrodynamic cavitation in (waste)water treatment, Ultrason. Sonochem., 2016, 29, 577–588 CrossRef CAS PubMed.
-
C. Gottschalk, J. A. Libra and A. Saupe, Ozonation of water and waste water: a practical guide to understanding ozone and its applications. 2nd completely rev. and updated ed, Wiley-VCH, Weinheim, 2010, p. 362 Search PubMed.
- H. Wang and Z. J. Ren, Bioelectrochemical metal recovery from wastewater: A review, Water Res., 2014, 66, 219–232 CrossRef CAS.
- In Activated carbon for water and wastewater treatment: integration of adsorption and biological treatment, ed. F. Çeçen and Ö. Aktaş, Wiley-VCH-Verl, Weinheim, 2012, p. 388 Search PubMed.
- T. M. Huggins, A. Haeger, J. C. Biffinger and Z. J. Ren, Granular biochar compared with activated carbon for wastewater treatment and resource recovery, Water Res., 2016, 94, 225–232 CrossRef CAS.
- M. F. Hamoda, I. Al-Ghusain and N. Z. Al-Mutairi, Sand filtration of wastewater for tertiary treatment and water reuse, Desalination, 2004, 164(3), 203–211 CrossRef CAS.
- M. Xie, L. D. Nghiem, W. E. Price and M. Elimelech, Toward Resource Recovery from Wastewater: Extraction of Phosphorus from Digested Sludge Using a Hybrid Forward Osmosis–Membrane Distillation Process, Environ. Sci. Technol. Lett., 2014, 1(2), 191–195 CrossRef CAS.
- R. Kumar and P. Pal, Assessing the feasibility of N and P recovery by struvite precipitation from nutrient-rich wastewater: a review, Environ. Sci. Pollut. Res., 2015, 22(22), 17453–17464 CrossRef CAS PubMed.
- F. Morgan-Sagastume, F. Valentino, M. Hjort, D. Cirne, L. Karabegovic and F. Gerardin,
et al., Polyhydroxyalkanoate (PHA) production from sludge and municipal wastewater treatment, Water Sci. Technol., 2014, 69(1), 177–184 CrossRef CAS PubMed.
- Y. V. Nancharaiah, S. V. Mohan and P. N. L. Lens, Biological and Bioelectrochemical Recovery of Critical and Scarce Metals, Trends Biotechnol., 2016, 34(2), 137–155 CrossRef CAS PubMed.
- P. Westerhoff, S. Lee, Y. Yang, G. W. Gordon, K. Hristovski and R. U. Halden,
et al., Characterization, Recovery Opportunities, and Valuation of Metals in Municipal Sludges from U.S. Wastewater Treatment Plants Nationwide, Environ. Sci. Technol., 2015, 49(16), 9479–9488 CrossRef CAS PubMed.
|
This journal is © The Royal Society of Chemistry 2020 |