DOI:
10.1039/C9TA05441C
(Paper)
J. Mater. Chem. A, 2019,
7, 17306-17314
Metallovesicles as smart nanoreactors for green catalytic synthesis of benzimidazole derivatives in water†
Received
22nd May 2019
, Accepted 14th June 2019
First published on 15th June 2019
Abstract
Metallovesicles are an emerging class of soft nanomaterials where spherical bilayer membranes, resulting from self-aggregation of amphiphilic metal complexes, amalgamate the advantages of metal specific catalytic properties and small hydrophobic cavities serving as nanoreactors. The confinement of substrates in these vesicle bilayers, on account of hydrophobic interactions in aqueous media, encourage their application in catalysis, particularly, where preclusion of organic solvents is of prime concern without compromising desired reaction rates and product yields. In the present work, novel amphiphilic metallosurfactant complex [Cu(C12H25NH2)2]Cl2 has been self-assembled to achieve spherical bilayer structures known as copper metallovesicles (CuMVs). DLS, TEM and FESEM analyses revealed the formation of spherical multivesicular vesicles in the size range 160–200 nm. The multivesicular structure of CuMVs was further supported by small angle X-ray scattering (SAXS) results. The as-synthesized CuMVs were further assessed for their potential as aqueous catalytic system for the synthesis of important therapeutic agents, benzimidazoles. The co-existence of hydrophobic reactants inside the metal-adorned vesicle bilayers, affords high product yield in short times. Facile synthesis of metallosurfactants, self-assembly to metallovesicles, aqueous reaction media, low metal concentration, low E-factor values, stability and recyclability of metallovesicles are the features that establish metallovesicular catalysis as a promising multifaceted approach for greener catalysis of benzimidazole synthesis and many other significant synthetic reactions.
1. Introduction
In recent years, the scientific community has become more sensitive towards environmental issues and has worked illustriously in developing such protocols for synthesis of organic molecules, which not only, are environment friendly but sustainable as well.1 All the catalytic approaches, whether they involve homogeneous or heterogeneous catalysts, are critically examined on the scale of green chemistry.2 Besides the cost and energy factor, the major factor that decides the green nature of a catalytic protocol is the choice of reaction media.3 Many organic syntheses that involve harmful organic solvents as reaction media are high on E-factor value which is a prime concern in developing eco-friendly and sustainable protocols.4 Water, for its inexpensive, non-toxic and non-flammable nature, has intrigued scientists to develop methods that favor organic syntheses of molecules in water.5 While heterogeneous catalysis in water requires high temperatures (preferably microwaves), the homogeneous catalysis in presence of water poses difficulties in separation of products and even contamination of water for reuse.6 The challenge of combining the better selectivity of homogeneous catalysis with the recoverability and reusability factor of heterogeneous catalysis puts forward the need of developing innovative and smart materials that smartly address the demands of green catalysis.
Nanoreactors, comprising the non-covalent self-assemblies of amphiphilic molecules, have emerged as excellent alternatives to combine the features of homogeneous and heterogeneous catalysis in one-pot.7 The amphiphilic nature of these self assemblies resolve the solubility issues of substrates in water, which are held together in hydrophobic pockets of these self-assembled nanoreactors. The compartmentalization of substrates results in their increased proximity and concentration around active catalytic sites.8 In literature, a vast variety of amphiphilic aggregates like micelles, vesicles, microemulsions, liposomes, polymerosomes etc. of suitable morphology are known to enhance the reaction rates of many organic reactions.9–13 Vesicles, for instance, have better catalytic performance in comparison to micelles and other aggregates14–16 because of the following four factors17 (i) they possess chemical aspects of biological membranes and show tremendous selectivity (ii) they increase the proximity between the reagents by decreasing the effective volume where the reaction takes place (iii) their structural complexity provides necessary stearic-hindrance to avoid side reactions (iv) they provide reaction centers with different polarity in comparison to the bulk solvent. Depending upon reaction requirements, the fourth factor can be altered by incorporation of a metal ion into the vesicular structure. The metallovesicles, formed as a result of functionalization of traditional vesicles with suitable metal ions, are adorned with notable catalytic properties.18,19 Though there are numerous applications of vesicular systems in drug delivery, model protocells, vaccination and reaction promoters,20–24 the metallovesicular systems are very less explored for their potential as efficient catalytic systems.
The aim of the present work is two-fold; one, to fabricate metallovesicles (CuMVs) from amphiphilic metallosurfacatnt complex, bisdodecylaminecopper(II) chloride and assess their structural details. And two, to efficiently use the as-fabricated CuMVs, as catalyst, in synthesis of benzimidazole derivatives in milder reaction conditions with water as solvent. Benzimidazole and its derivatives are important building blocks of pharmaceutical industries owing to their various biological activities such as antiarrhythmic,25 antiulcer,26 ionotropic,27 antihelmintic,28 anticancer29 and antimicrobial activities.30 Furthermore, these compounds have significant industrial applications in UVB filters, optical devices, paints, and fuel cell membranes.31–34 Many reports are available in literature to synthesize benzimidazoles by the condensation of arylaldehydes with ortho-phenylenediamines using variety of homogeneous and heterogeneous catalysts, for e.g. acetic acid,35 trimethylsilyl chloride,36 oxone,37 sulphamic acid,38 PhI(OAc)2,39 KHSO4,40 K3PO4,41 Amberlite,42L-proline,43 CoCl2,44 PTSA,45 metal triflates,46 heteropoly acids,47 solid support catalysts48 and ionic liquids49etc. Unfortunately, many of these methods suffer from drawbacks such as environmentally hazardous solvents/reagents, drastic reaction conditions, low yields, tedious workup procedures, low atom economy and various side products. Nanoparticles have also been utilized as heterogeneous catalysts in this synthesis but most of the high-performing nanoparticles catalysts include expensive transition metals, hazardous oxidants generating toxic by-products.50–56 As a consequence, the introduction of an efficient and mild method is still needed to overcome these limitations. The present catalytic system, comprising of CuMVs, offers a room temperature synthesis of 2-aryl-1H-benzimidazole derivatives at low metal concentration in aqueous media which is economic, environmentally benign, recyclable and energy efficient. The intriguing results obtained with CuMVs in the present synthetic reaction with low E-factor values, expand their scope as catalysts in other reactions as well as gives direction in designing similar catalytic structures with different functionalities.
2. Experimental
2.1. Materials
Copper(II) chloride (99%), dodecylamine (98.6%), o-phenylenediamine (99%), cholesterol (99%) and DMSO-d6 were purchased from Sigma Aldrich. Chloroform and diethyl ether were purchased from Fischer Scientific. o-Phenylenediamine (99%) was purchased from Sigma Aldrich and various aromatic aldehydes (>98%) were purchased from Himedia. All the chemicals were used as received without any further purification. Polycarbonate microfilters (0.25 micron pore size) were purchased from Millipore.
2.2. Designing the catalyst
2.2.1. Fabrication of copper metallovesicles (CuMVs).
We are hereby proposing a catalyst design which adheres to all the prerequisites for the synthesis of benzimidazole derivatives. In our previous report, we have described the synthesis of a metallosurfactant complex, bisdodecylaminecopper(II) chloride [Cu(C12H25NH2)2]Cl2 which is used as precursor for the fabrication of CuMVs in present report.57
The CuMVs were prepared by employing conventional chloroform film method.58 Equimolar amounts of [Cu(C12H25NH2)2]Cl2 (5 mmol) and cholesterol (5 mmol) were dissolved in CHCl3 (20 ml). The solution was stirred at 333 K in a round bottom flask for 30 minutes followed by slow evaporation of CHCl3 under reduced pressure. The resulting thin film on the surface of round bottom flask was dried in vacuum desiccator at room temperature for 24 h and further hydrated with deionized water (100 ml). The obtained solution was repeatedly sonicated, subjected to vortex shaker for about 4 h and obtruded through polycarbonate microfilters (0.25 micron pore size) for at least 10 times which resulted in uniform particle size distribution as monitored by Dynamic Light Scattering (DLS). Different volumes of this solution were further used for catalytic synthesis of benzimidazole derivatives.
2.2.2. Characterization techniques.
The formation of CuMVs was confirmed by different characterization techniques such as DLS, Atomic Force Microscopy (AFM), Confocal Laser Scanning Microscopy (CLSM), Transmission Electron Microscopy (TEM), Field Emission Scanning Electron Microscopy (FESEM) and Small Angle X-ray Scattering (SAXS). Size measurements of CuMVs were done by DLS experiments performed on ALV-5000 with Nd:YAG laser (wavelength 532 nm) at room temperature. For TEM analysis, samples of CuMVs were dispersed on a carbon coated copper grid and air dried grid was analyzed using Hitachi H 7500 instrument operated at 80–110 kV. For FESEM images were taken with Hitachi (SU8010) at various operating voltages (3–20 kV). AFM images were obtained on Nanoscope “V-multimode 8”. The instrument was operated in the contact mode with an operating frequency of 312.13 kHz. Confocal Laser Scanning Microscope (CLSM) images were taken on Nikon Eclipse Ti(C2Si) inverted microscope (100× objective) with attached Nikon camera. Nikon ECLIPSE E100 with attached camera was used to obtain optical micrographs of CuMVs. SAXS studies were performed on SAXSpace by Anton Paar operated on line collimation mode at 40 kV. Quartz capillary liquid cell of 1 mm diameter was used to hold the sample. The scattering pattern was recorded using CCD detector having scattering vector q ranges from 0.01 nm−1 to 8 nm−1. All the measured intensity was normalised and the background scattering contributions from capillary/solvent were corrected. The data were evaluated with General Inverse Fourier Transformation (GIFT) software. The phase transition temperature of metallosurfacatnt complex was found through Differential Scanning Calorimetry (DSC), performed on DSC-Q20 (TA instrument, USA) at scanning range of 10 °C min−1. ICP-MS analyses were done on ICP-MS Agilent 7800 to determine concentration of Cu in metallovesicle solution.
2.3. Catalytic synthesis of 2-aryl-1H-benzimidazoles
2.3.1. General procedure.
A mixture of o-phenylenediamine (1 mmol) and aromatic aldehyde (1 mmol) was added to a round bottom flask containing CuMVs solution (20 ml, containing 2 ppm Cu2+) (Scheme 1). The mixture was stirred well at 303 K, and the progress of the reaction was monitored by thin layer chromatography at regular time intervals. As the reaction proceeded to completion, the precipitation of product took place. After completion, the reaction mixture was filtered to separate the product and obtained solid was recrystallized in ethanol. Benzimidazole derivatives, which could not be crystallized, were purified with the help of column chromatography.
 |
| Scheme 1 Synthesis of 2-substituted benzimidazoles R = H, 2-OCH3, 4-OCH3, 4-NO2, 3-NO2, 4-Cl, 4-Br, 4-CN, 4-CH3. | |
2.3.2. Characterization of products.
The identification of all the synthesized benzimidazole derivatives was done through 1H NMR using DMSO as a solvent. The physical and spectral data of all the products (entries 1a–1i, Table 1) is given in ESI (Section S1, ESI†).
Table 1 Effect of substituent (on aromatic aldehyde) on reaction ratea
Entry |
Diamine |
Aromatic aldehyde |
Product |
Time (min) |
Yieldb (%) |
E-factor |
Reaction conditions: o-phenylenediamine (1 mmol), aromatic aldehyde (1 mmol), CuMVs (2 ppm, 20 ml), 303 K.
Isolated yield.
|
1a
|
|
|
|
70 |
94 |
0.175 |
1b
|
|
|
|
85 |
90 |
0.212 |
1c
|
|
|
|
80 |
93 |
0.173 |
1d
|
|
|
|
45 |
95 |
0.142 |
1e
|
|
|
|
50 |
93 |
0.166 |
1f
|
|
|
|
60 |
95 |
0.148 |
1g
|
|
|
|
75 |
95 |
0.130 |
1h
|
|
|
|
60 |
96 |
0.138 |
1i
|
|
|
|
80 |
91 |
0.206 |
2.3.3. Recycling the catalyst.
The CuMVs were collected as filtrate after filtration of reaction mixture and were purified with diethyl to extract traces of unreacted substrates (if any). The as-collected free aqueous CuMVs solution was used in next catalytic cycles to check their recycling efficiency.
3. Results and discussion
This article engulfs a green and highly efficient catalytic synthesis of benzimidazole derivatives using specially designed aqueous CuMVs. Recyclable CuMVs have a dual role to play i.e. apart from behaving as nano-vessels to accommodate reactants within them and increase their physical proximity; they also provide acidic sites integrated within the aggregated structure that expeditiously catalyzes the model reaction.
An amphiphilic metallosurfactant complex of copper equipped with two long hydrocarbon chains viz [Cu(C12H25NH2)2]Cl2 was synthesized and further used for the preparation of CuMVs using film hydration method. The thin film as described in Section 2.2.1 consists of sediments of multiple bilayers which were sonicated and mechanically vortexed with deionised water at a temperature above the phase transition temperature (333 K) of metallosurfactant complex (illustrated by DSC, Fig. S1, ESI†). This resulted in swelling and folding of these lamellar bilayer structures into spherical CuMVs. The addition of equimolar amounts of cholesterol to metallosurfactant complex was done to meliorate rigidity and stability of CuMVs.59 The notably polydisperse CuMVs were repeatedly extruded (at least 10 times) through polycarbonte microfilters resulting in monodisperse CuMVs as monitored by DLS.60 DLS analyses of CuMVs before and after extrusion are given in (Fig. S2, ESI†) respectively. The polydispersity index of CuMVs was reduced from 0.367 to 0.132 after extrusion. The average size of extruded CuMVs was found to be 220 nm. It is to be noted that amount of water used for hydration process has no effect on CuMVs' morphology. However, it results in the variation of concentration of resulting CuMVs in solution.
Morphological details of CuMVs were obtained by different microscopic techniques. Preliminary structural examination of CuMVs was done with conventional light microscope that showed spherical structures (Fig. S3(a), ESI†). Further detailed examination of size, morphology and topography of the CuMVs was carried out using TEM, CLSM, FESEM and AFM techniques. TEM, CLSM and FESEM analysis gave a fairer insight into the size, morphology and lamellarity whereas AFM studies showed topographical analysis of the prepared CuMVs. TEM image (Fig. 1(a)) shows the formation of multivesicular metallovesicles (large number of small vesicles inside a giant vesicle) in the size range 160–200 nm. Inset of Fig. 1(a) shows high resolution TEM image of a multivesicular vesicle (180 nm). The spherical shape of CuMVs is further confirmed through CLSM images (Fig. 1(b)). Also, the FESEM results affirm with those of TEM showing spherical shaped structures (Fig. S3(b)†).58 AFM images show height and phase topographies of CuMVs (Fig. S3(c)58 and (d),† respectively). Color contrast in height images reveals the average particle size of CuMVs in the range of 180–200 nm however vesicles are found to be vertically compressed due to high local force applied by the tip of the cantilever. Colour contrast in phase image shows distinct phase boundaries as we move from one metallovesicle to other, showing segregated distribution of CuMVs. Careful analysis of the phase images shows colour contrast within the same vesicle illustrating the presence of different phases in accordance with multivesicular topography.
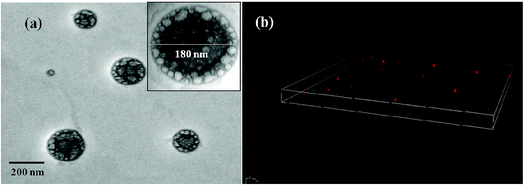 |
| Fig. 1 Structural investigation of CuMVs showing (a) TEM micrograph, inset: single multivesicular vesicle (b) CLSM image. | |
Further structural investigation of CuMVs was done using SAXS. Fig. 2(a) shows experimental SAXS scattering profile of CuMVs in which scattering intensities I(q) are plotted as a function of scattering vector q, which is defined as 4π
sin
θ/λ; where θ is half the scattering angle and λ is wavelength of X-rays. The absence of distinct diffraction maxima in SAXS profile suggested the lack of periodicity in the structures.61 This was indicative of multivesicular structure of CuMvs consisting of non-concentric bilayers. Also, the power law (q−1) of scattering curve in line collimation mode suggested vesicular structures.61–63 The interpretation of scattering curve in real space was done using General Inverse Fourier Transformation (GIFT) which generated Pair Distance Distribution Function (PDDF), P(r). Fig. 2(b) and (c) show the approximated SAXS scattering data fit with experimental data after carrying out indirect fourier transformation and the corresponding PDDF, respectively. It can be seen that the data fits well with experimental data and the PDDF curve i.e. P(r) vs. r shows asymmetry with alternate signs of distribution function. Since P(r) is calculated by weighting two electron density values connected by distance r, the asymmetry in signs of P(r) points towards inhomogenous interior of CuMVs.
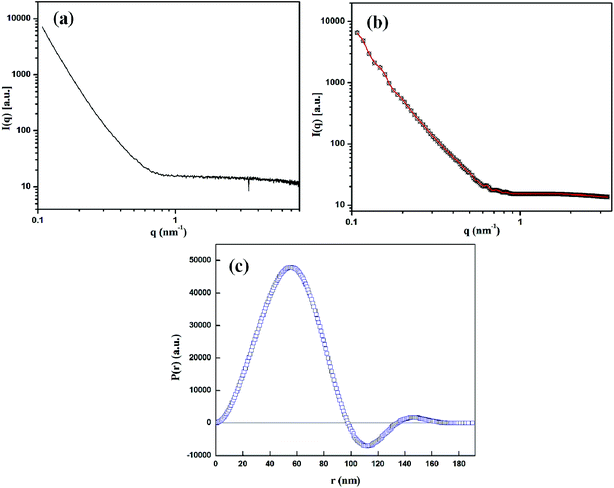 |
| Fig. 2 (a) SAXS scattering profile of CuMVs (b) approximated scattering curve (red) after carrying out Indirect Fourier Transformation and (c) the corresponding PDDF. | |
3.1. Catalytic synthesis of 2-aryl-1H-benzimidazoles using CuMVs
The CuMVs were tested for their catalytic efficiency in synthesis of various benzimidazole derivatives (entries 1a–1i, Table 1). The optimum reaction conditions were obtained by choosing a model reaction between o-phenylenediamine and 4-chlorobenzaldehyde was chosen (Scheme 2) to obtain the optimum reaction conditions by varying different reaction parameters like catalyst dose and temperature. In all the experiments, the progress of the reaction was monitored by thin layer chromatography and % product yield were noted.
 |
| Scheme 2 Model reaction between o-phenylenediamine and 4-chlorobenzaldehyde. | |
Initially, a blank experiment was run where the model reaction was performed in the absence of CuMVs. The reaction did not show any progress even after 4 h suggesting the need of catalyst for successful conversion of reactants to product.
In order to optimize the catalyst dose for the reaction, the CuMVs solution was initially assessed for concentration of catalytically active sites (Cu2+) using ICP-MS analysis that suggested it to be 2 ppm. The model reaction was then carried out with different volumes of CuMVs solution. Fig. 3(a) summarizes the time for reaction completion and isolated product yield in each case. An increase in the volume of CuMVs solution led to faster completion of reaction with increased product yield.
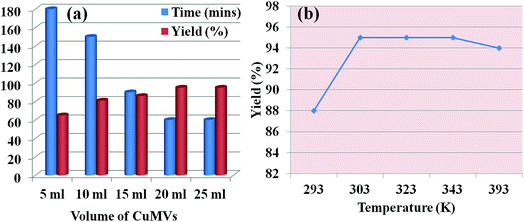 |
| Fig. 3 (a) Effect of catalytic dose (b) variation of product yield with reaction temperature. Reaction conditions (a): o-phenylenediamine (1 mmol), 4-chlorobenzaldehyde (1 mmol), CuMVs (2 ppm), 303 K (b) 20 ml. *Isolated yield. | |
The results could be understood in terms of increase in number of catalytically active Cu2+ sites. Increasing the volume of solution ensured more number of CuMVs and hence faster reactions with better yields. The constant results beyond 20 ml of catalyst volume suggest the complete encapsulation of reactants in the CuMVs. Introduction of anymore amount of CuMVs did not affect the reaction rate as there was no further encapsulation of reactants in newly introduced CuMVs. The catalytic reaction was also performed at higher temperatures to understand the effect of temperature on the reaction performance. It was found that increasing the reaction temperature had negligible effect on product yield (Fig. 3(b)). Based on these results, the optimum reaction temperature for the reaction was fixed to be 303 K.
3.2. Substrate scope
The designed catalytic system has wide applicability as it covers a large number of substrates for the synthesis of various benzimidazole derivatives. The reaction was performed with different aromatic aldehydes carrying different substituents and good to excellent product yields were obtained in all the cases (Table 1). The reaction times are greatly influenced by the electron releasing or withdrawing nature of substituents attached to the aromatic ring. As expected from the first step of mechanism which is nucleophillic attack of o-phenylenediamine on aromatic aldehyde, electron withdrawing groups increase the electrophilicity of carbonyl carbon favouring the nucleophillic attack. This accounts for the lower reaction times for electron withdrawing groups as compared to electron releasing groups. However, for same group longer reaction times were observed for the ortho and meta substitutions due to stearic reasons.
3.3.
E-factor calculations
The environmental impact of the catalytic protocol was assessed through E-factor calculations which measures the amount of waste generated during a synthetic process. The calculations were done using eqn (1) as follows: |  | (1) |
It is to be noted that since the CuMVs were recovered during the process for further usage, mass of CuMVs have been omitted from the E-factor calculations. The values of E-factor have been calculated for synthesis of all the benzimidazole derivatives and are given in Table 1. It can be seen from Table 1 that E-factor values are very small ranging between 0.14 and 0.21 which advocates the green nature of the protocol. This is attributed to minimum waste generation during the process which occurs in water as reaction medium in presence of highly active recyclable CuMVs.
3.4. Mechanistic aspects of catalysis with CuMVs
The CuMVs act as small nanoreactors bearing catalytically active Cu2+ sites. The hydrophobic pockets inside the multivesicular structure of CuMVs encapsulate the substrates and increase their concentration at catalytically active Cu2+ sites resulting in faster catalysis. This encapsulation of substrates in hydrophobic regions is evidenced through CLSM image of CuMVs encapsulated with reactant (4-bromobenzaldehyde) (Fig. 4).
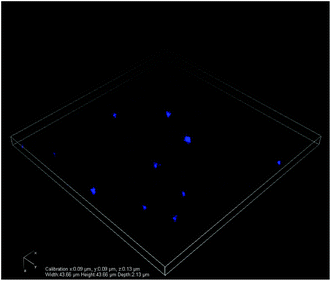 |
| Fig. 4 CLSM micrograph of CuMVs loaded with 4-bromobenzaldehyde. | |
The proposed mechanism for the reaction taking place inside the CuMVs is depicted in Scheme S1 (ESI†). The Cu2+ sites in the CuMVs activate the carbonyl carbon of the aromatic aldehyde for nucleophilic attack by –NH2 group of o-phenylenediamine. The resulting imine is further attacked by second –NH2 to form dihydroimidazole followed by aromatization in presence of air to give 2-aryl-1H-benzimidazoles as product.
3.5. Reusability tests
The developed catalytic system was assessed for its recycling efficiency. Firstly, the CuMVs solution recycled after 1st reaction cycle was investigated for structural integrity of CuMVs using FESEM and light microscope (Fig. 5). It can be seen from FESEM image (Fig. 5(a)) and optical micrographs (Fig. 5(b)) that the recycled CuMVs maintained their structural integrity.
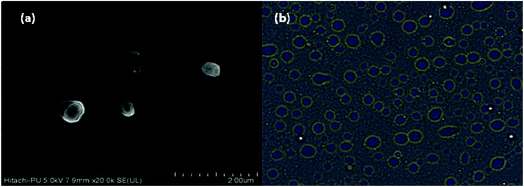 |
| Fig. 5 (a) FESEM image and (b) optical micrograph of CuMVs recovered after 1st cycle of catalytic reaction. | |
The recycled CuMVs solution was subjected to further catalytic cycles and the reaction performance was noted in each case. It was found that the CuMVs maintained their catalytic performance for up to 6 reaction cycles (Fig. 6). The performance is attributed to mechanical strength and stability of metallovesicular structures.
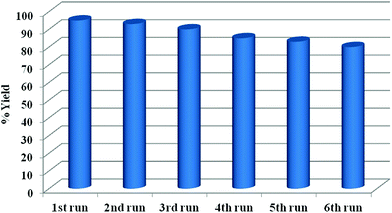 |
| Fig. 6 Recycling efficiency of CuMVs. | |
The decline in the performance of the catalytic system can be explained on the basis of rupture of metallovesicle structure on repeated use beyond 6th cycle (Fig. S4, ESI†).
4. Advantage over other catalytic systems and future prospects
The synthesis of 2-aryl-1H-benzimidazole derivatives has been achieved with a number of catalysts in literature. The reaction conditions and their catalytic performance are tabulated in Table 2. It can be seen that most of the catalysts, apart from their own toxic nature, require high temperatures, toxic solvents as reaction medium and tedious work-up procedures to separate the products. The transition metal oxide nanoparticles (In2O3),50 though prove to be an efficient alternative in terms of their activity and recoverability, compromise with the cost factor which cannot be ignored for the implementation of the protocol on large scale. The present system is found to qualify all the significant conditions of an efficient catalytic system like high activity, environment benignity, cost effectiveness, recyclability and ease of product separation. The catalytic systems consisting of metallovesicles have a promising scope in future of metal-assisted catalysis of organic reactions and transformations which, otherwise, require traditional organic solvents and expensive metal catalysts.
Table 2 Comparison of catalytic performance of present catalytic system with literature reports for the synthesis of 2-substituted benzimidazoles
S. no. |
Catalyst |
Catalyst dose |
Time/yield (%) |
Solvent/temperature |
Ref. |
1 |
VOSO4 |
3 mol% |
1 h/92% |
EtOH/rt |
64
|
2 |
TiCl3OTf |
10 mol% |
50 min/86% |
EtOH/rt |
65
|
3 |
Ce(NO3)3·6H2O |
30 mol% |
1.5–2 h/93% |
DMF/80 °C |
66
|
4 |
PhSiH3 |
4 equivalent |
2 h/95% |
DMF/120 °C |
67
|
5 |
Co(OH)2 |
10 mol% |
4–7 h/82–96% |
EtOH/rt |
68
|
6 |
ClSO3H |
10 mol% |
1.8 h/93% |
2-Propanol/rt |
69
|
7 |
CuFe2O4 NPs |
20 mol% |
24 h/89% |
Toluene/O2 |
51
|
8 |
Mesoporous TiO2–Fe2O3 |
20 mg |
3 h/97% |
H2O/O2 |
52
|
9 |
Pt/TiO2 |
1 mol% |
1 h/78% |
Mesitylene |
53
|
10 |
Fe3O4–SiO2–(NH4)6Mo7O24 nanocomposite |
220 mg |
0.5 h/90% |
EtOH/H2O2 |
54
|
11 |
α-MoO3 nanobelts |
2 mol% |
0.5 h/93% |
t-BuOOH |
55
|
12 |
CuI Nps |
10 mol% |
1 h/96% |
CH3CN/O2 |
56
|
13 |
CuMVs |
0.03 mol% |
1 h/96% |
H2O |
Present work |
5. Conclusions and outlook
In conclusion, novel metallovesicles, CuMVs have been fabricated using metallosurfactant complex, bisdodecylaminecopper(II) chloride. The as-fabricated metallovesicles have been employed as nanoreactors for the catalytic synthesis of 2-aryl-1H-benzimidazole. The successful condensation of o-phenylenediamine with aromatic aldehydes in aqueous metallovesicles solutions under mild reaction conditions to give excellent product yields, establish the claim of these novel metallovesicles as small nanoreactors possessing active catalytic sites. The notable catalytic activity, harmless aqueous reaction medium, ambient reaction conditions promote the as-developed catalytic approach as effective and greener approach for benzimidazole synthesis. The strength and stability of metallovesicular structures impart yet another attractive feature to the catalytic approach in the form of recyclability. Effective catalytic action followed by facile recovery of metallovesicles enables them to be reused in further reaction cycles. The efficiency of CuMVs as catalysts in the present protocol opens up the way to diverse applications of these materials in the field of organic synthesis which requires a dire need to shift to efficient, greener and sustainable catalytic approaches.
Conflicts of interest
Authors declare no conflicts of interest.
Acknowledgements
Ganga Ram Chaudhary and Sesha Srinivasan would like to acknowledge the support of UGC, India under INDO-US 21st Century Knowledge Initiative project [F. No. 194-2/2016 (IC)]. Navneet Kaur is thankful to UGC for Senior Research Fellowship. Gurpreet Kaur acknowledges DST, India for Inspire Faculty award (IFA-12-CH-41). All the authors acknowledge the support of DST PURSE II grant for this work.
References
- Y. Liu, W. Wang, X. Xu, J. P. Veder and Z. Shao, J. Mater. Chem. A, 2019, 7, 7280–7300 RSC.
- M. J. Mulvihill, E. S. Beach, J. B. Zimmerman and P. T. Anastas, Annu. Rev. Environ. Resour., 2011, 36, 271–293 CrossRef.
- V. Polshettiwar and R. S. Varma, Acc. Chem. Res., 2008, 41(5), 629–639 CrossRef CAS PubMed.
- N. Kaur, G. Kaur, A. Bhalla, J. Dhau and G. R. Chaudhary, Green Chem., 2018, 20, 1506–1518 RSC.
- M. B. Gawande, V. D. Bonifácio, R. Luque, P. S. Branco and R. S. Varma, Chem. Soc. Rev., 2013, 42(12), 5522–5551 RSC.
- F. H. M. Dekker, A. Bliek, F. Kapteijn and J. A. Moulijn, Chem. Eng. Sci., 1995, 50, 3573–3580 CrossRef CAS.
- M. T. De Martino, L. K. Abdelmohsen, F. P. Rutjes and J. C. van Hest, Beilstein J. Org. Chem., 2018, 14(1), 716–733 CrossRef CAS PubMed.
- S. H. A. M. Leenders, R. Gramage-Doria, B. de Bruin and J. N. H. Reek, Chem. Soc. Rev., 2015, 44, 433–448 RSC.
- T. Dwars, E. Paetzold and G. Oehme, Angew. Chem., Int. Ed., 2005, 44, 7174–7199 CrossRef CAS PubMed.
- K. Tonova and Z. Lazarova, Biotechnol. Adv., 2008, 26(6), 516–532 CrossRef CAS PubMed.
- M. Akbarzadeh, Z. Moosavi-Movahedi, A. Shockravi, R. Jafari, K. Nazari, N. Sheibani and A. A. Moosavi-Movahedi, J. Mol. Catal. A: Chem., 2016, 424, 181–193 CrossRef CAS.
- Y. Jiang, D. Wang, Z. Pan, H. Ma, M. Li, J. Li, A. Zheng, G. Lv and Z. Tian, Front. Chem. Sci. Eng., 2018, 12(1), 32–42 CrossRef CAS.
- M. Hirose, T. Ishigami, K. Suga and H. Umakoshi, Langmuir, 2015, 31(47), 12968–12974 CrossRef CAS PubMed.
- T. Kunitake, Y. Okabata, R. Ando, S. Shinkai and S. Hirakawa, J. Am. Chem. Soc., 1980, 102, 7877–7881 CrossRef CAS.
- M. V. Scarpa, P. S. Araujo, S. Schreier, A. Sesso, A. G. Oliveira, H. Chaimovich and I. M. Cuccovia, Langmuir, 2000, 16, 993–999 CrossRef CAS.
- J. Perez-Juste, F. Hollfelder, A. J. Kirby and J. B. F. N. Engberts, Org. Lett., 2000, 2, 127–130 CrossRef CAS PubMed.
- P. Walde, H. Umakoshi, P. Stano and F. Mavelli, Chem. Commun., 2014, 50, 10177–10197 RSC.
- T. Rispens and J. B. F. N. Engberts, Org. Lett., 2001, 3, 941–943 CrossRef CAS PubMed.
- B. Gruber, E. Kataev, J. Aschenbrenner, S. Stadlbauer and B. König, J. Am. Chem. Soc., 2011, 133(51), 20704–20707 CrossRef CAS PubMed.
- J. W. Szostak, D. P. Bartel and P. L. Luisi, Nature, 2001, 409, 387–390 CrossRef CAS PubMed.
- V. Noireaux and A. Libchaber, Proc. Natl. Acad. Sci. U. S. A., 2004, 101, 17669–17674 CrossRef CAS PubMed.
- P. Walde, BioEssays, 2010, 32, 296–303 CrossRef CAS PubMed.
- P. Stano, P. Carrara, Y. Kuruma, T. P. de Souza and P. L. Luisi, J. Mater. Chem., 2011, 21, 18887–18902 RSC.
- S. Matosevic, BioEssays, 2012, 34, 992–1001 CrossRef CAS PubMed.
- D. A. Horton, G. T. Bourne and M. L. Smythe, Chem. Rev., 2003, 103, 893–930 CrossRef CAS PubMed.
- S. D. Undevia, F. Innocenti, J. Ramirez, L. House, A. A. Desai, L. A. Skoog, D. A. Singh, T. Karrison, H. L. Kindler and M. Ratain, Eur. J. Cancer, 2008, 44, 1684–1692 CrossRef CAS PubMed.
-
Z. Lixin and L. D. Arnold, Natural Products: Drug Discovery and Therapeutic Medicine, Humana Press, Totowa, NJ, 2005, p. 341 Search PubMed.
-
H. D. Jakubke and N. Sewald, Peptides from A to Z: A Concise Encyclopedia, Wiley-VCH Verlag GmbH & Co. KGaA, Weinheim, Germany, 2008, p. 378 Search PubMed.
-
Encyclopedia of Parasitology, ed. H. Mehlhorn, Springer-Verlag, Berlin, 2008, vol. 3, p. 386 Search PubMed.
- R. Sarges, H. R. Howard, R. G. Browne, L. A. Lebel, P. A. Seymour and B. K. Koe, J. Med. Chem., 1990, 33, 2240–2254 CrossRef CAS PubMed.
-
P. Fritsch, in Dermatologie und Venerologie, Springer-Verlag, Berlin, 2004, vol. 2 Search PubMed.
-
H. M. Smith, High Performance Pigments, Wiley-VCH Verlag GmbH & Co. KGaA, Weinheim, Germany, 2002, pp. 135–158 Search PubMed.
-
B. Meuthen and A. S. Jandel, Coil Coating, 2. Auflage, Friedr, Vieweg & Sohn Verlag, Wiesbaden, Germany, 2008, p. 65 Search PubMed.
-
G. G. Scherer, Fuel Cells II, Springer-Verlag, Berlin, 2008, pp. 65–120 Search PubMed.
- D. Azarifari, M. Pirhayati, B. Maleki, M. Sanginabadi and R. N. Yami, J. Serb. Chem. Soc., 2010, 75, 1181–1189 CrossRef.
- J. P. Wan, S. F. Gan, J. M. Wu and Y. Pan, Green Chem., 2009, 11, 1633–1637 RSC.
- P. L. Beaulieu, B. Hache and V. E. Moon, Synthesis, 2003, 11, 1683–1692 CrossRef.
- M. Chakrabarty, S. Karmakar, A. Mukherji, S. Arima and Y. Harigaya, Heterocycles, 2006, 16, 4169–4173 Search PubMed.
- L. H. Du and Y. G. Wang, Synthesis, 2007, 5, 675–678 Search PubMed.
- H. Ma, Y. Wang and A. Wang, Heterocycles, 2006, 68, 1669–1673 CrossRef CAS.
- C. Xie, X. Han, J. Gong, D. Li and C. Ma, Org. Biomol. Chem., 2017, 15, 5811–5819 RSC.
- S. D. Sharma and D. Konwar, Synth. Commun., 2009, 39, 980–991 CrossRef.
- R. Varala, A. Nasreen, R. Enugala and S. R. Adapa, Tetrahedron Lett., 2007, 48, 69–72 CrossRef CAS.
- A. T. Khan, T. Parvin and L. H. Choudhury, Synth. Commun., 2009, 39, 2339–2346 CrossRef CAS.
- X. Han, H. Ma and Y. Wang, ARKIVOC, 2007, 13, 150–154 Search PubMed.
-
(a) P. Torabi, J. Azizian and J. Noei, Tetrahedron Lett., 2016, 57, 185–188 CrossRef;
(b) T. Itoh, K. Nagata, H. Ishikawa and A. Ohsawa, Heterocycles, 2004, 63, 2769–2783 CrossRef CAS;
(c) R. Trivedi, S. K. De and R. A. Gibbs, J. Mol. Catal. A: Chem., 2005, 245, 8–11 CrossRef.
- M. M. Heravi, S. Sadjadi, H. A. Oskooie, R. H. Shoar and F. F. Bamoharram, Catal. Commun., 2008, 8, 504–507 CrossRef.
- A. Ben-Alloum, S. Bakkas and M. Soufiaoui, Tetrahedron Lett., 1998, 39, 4481–4484 CrossRef CAS.
- H. Q. Ma, Y. L. Wang, J. P. Li and J. Y. Wang, Heterocycles, 2007, 71, 135–140 CrossRef CAS.
- S. Santra, A. Majee and A. Hajra, Tetrahedron Lett., 2012, 53, 1974–1977 CrossRef CAS.
- D. Yang, X. Zhu, W. Wei, N. Sun, L. Yuan, M. Jiang, J. You and H. Wang, RSC Adv., 2014, 4, 17832–17839 RSC.
- S. Roy, B. Banerjee, N. Salam, A. Bhaumik and S. M. Islam, ChemCatChem, 2015, 7, 2689–2697 CrossRef CAS.
- C. Chaudhari, S. M. A. Hakim Siddiki and K. Shimizu, Tetrahedron Lett., 2015, 56, 4885–4888 CrossRef CAS.
- G. Bai, X. Lan, X. Liu, C. Liu, L. Shi, Q. Chen and G. Chen, Green Chem., 2014, 16, 3160–3168 RSC.
- M. Jafarpour, A. Rezaeifard, M. Ghahramaninezhad and T. Tabibi, New J. Chem., 2013, 37, 2087–2095 RSC.
- P. Linga Reddy, R. Arundhathi, M. Tripathi and D. S. Rawat, RSC Adv., 2016, 6, 53596–53601 RSC.
- G. Kaur, P. Singh, S. K. Mehta, S. Kumar, N. Dilbaghi and G. R. Chaudhary, Appl. Surf. Sci., 2017, 404, 254–262 CrossRef CAS.
- B. Kaur, G. Kaur and G. R. Chaudhary, J. Mater. Chem. B, 2019, 7, 3679–3691 RSC.
- P. Orathai, S. Chavadej and R. Rujiravanit, J. Biosci. Bioeng., 2011, 112, 102–106 CrossRef PubMed.
-
Z. Hongwei, Liposomes, Humana Press, New York, NY, 2017, pp. 17–22 Search PubMed.
- M. J. Moghaddam, L. de Campo, M. Hirabayashi, P. A. Bean, L. J. Waddington, J. A. Scoble, G. Coiac and C. Drummondd, J. Biomater. Sci., Polym. Ed., 2014, 2, 924–935 RSC.
- M. Malinda Salim, W. F. N. W. Iskandar, M. Patrick, N. I. Zahid and R. Hashim, Langmuir, 2016, 32, 5552–5561 CrossRef PubMed.
-
Anton Paar GmBH, SAXS-Data Classification 01-Basics, http://www.msg.ucsf.edu/XRayLab/SAXS-Lit/SAXS-Data%20Classification%20%26%Evaluation.pdf Search PubMed.
- C. S. Digwal, U. Yadav, A. P. Sakla, P. V. S. Ramya, S. Aaghaz and A. Kamal, Tetrahedron Lett., 2016, 57, 4012–4016 CrossRef CAS.
- J. Azizian, P. Torabi and J. Noei, Tetrahedron Lett., 2016, 57, 185 CrossRef CAS.
- G. M. Martins, T. Puccinelli, R. A. Gariani, F. R. Xavier, C. C. Silveira and S. R. Mendes, Tetrahedron Lett., 2017, 58, 1969–1972 CrossRef CAS.
- J. Zhu, Z. Zhang, C. Miao, W. Liu and W. Sun, Tetrahedron, 2017, 73, 3458–3462 CrossRef CAS.
- M. A. Chari, D. Shobha and T. Sasaki, Tetrahedron Lett., 2011, 52, 5575–5580 CrossRef.
- N. V. Shitole, K. S. Niralwad, B. B. Shingate and M. S. Shingare, Arabian J. Chem., 2016, 9, S858–S860 CrossRef CAS.
Footnote |
† Electronic supplementary information (ESI) available. See DOI: 10.1039/c9ta05441c |
|
This journal is © The Royal Society of Chemistry 2019 |