DOI:
10.1039/C8AN02414F
(Paper)
Analyst, 2019,
144, 2523-2530
Ratiometric fluorescent nanosensors for ultra-sensitive detection of mercury ions based on AuNCs/MOFs†
Received
13th December 2018
, Accepted 19th February 2019
First published on 19th February 2019
Abstract
Ratiometric fluorescent nanosensors were developed to detect mercury ions (Hg2+) using enhanced dual emissions from glutathione stabilized gold nanoclusters/indium-based metal–organic frameworks modified with cysteine (AuNCs/MIL-68(In)-NH2/Cys). The nanosensors exhibited bright pink fluorescence with AuNCs evenly distributed on MIL-68(In)-NH2. Under 370 nm excitation, the obtained sensor presented double fluorescence emission around 438 nm and 668 nm, which was attributed to MIL-68(In)-NH2 and GSH-AuNCs, respectively. The fluorescence emission was remarkably enhanced after modification with Cys. In the presence of Hg2+, the red fluorescence peak at 668 nm was quenched, while the blue fluorescence peak at 438 nm was slightly altered. The prepared AuNCs/MIL-68(In)-NH2/Cys nanosensors exhibited two linear ranges for the detection of Hg2+, namely from 20 pM to 0.2 μM and 0.2 μM to 60 μM, with a detection limit of 6.7 pM. They also presented high selectivity towards other ions and good performance in real water samples. Moreover, a radial star-shaped microfluidic paper-based analytical device (μPAD), as a straightforward and convenient platform, was successfully fabricated for the visual detection of Hg2+ with a wide detection range from 5 nM to 50 μM.
1. Introduction
Heavy metal pollution has become an increasingly serious environmental problem. The mercury ion (Hg2+), as a typical heavy metal ion, can be enriched and transmitted in the food chain, causing serious damage to the environment and human health.1,2 Owing to the affinity of Hg2+ with thiol groups, toxic Hg2+ can cause dysfunctions, like destruction of the central nervous system and the endocrine system.3–5 As recorded in the data,6 the World Health Organization (WHO) has set the maximum contamination level of Hg2+ in drinking water as 6 ppb and the U.S. Environmental Protection Agency (EPA) has set the limit of Hg2+ as 2 ppb. Until now, a series of analysis techniques for the determination of Hg2+ have been developed, such as inductively coupled plasma mass spectrometry,7 atomic absorption spectroscopy,8 atomic emission spectrometry,9 and surface-enhanced Raman scattering.10 Due to the requirements of bulky and expensive instruments and well-trained operators, it is difficult to apply them in on-site applications. Furthermore, it is necessary for researchers to develop a convenient and sensitive method to detect Hg2+. In recent years, fluorescence analysis methods based on AuNCs for Hg2+ have emerged on account of the rapid response and high sensitivity of AuNCs towards Hg2+. Fluorescent gold nanoclusters (AuNCs) with a size smaller than 3 nm exhibit molecule-like properties and show discrete electronic states.11,12 By taking advantage of the 5d10–5d10 interaction between Hg2+ and Au+, AuNCs with different protecting agents were developed as the fluorescent materials to detect Hg2+.13 For example, Shang et al. rapidly synthesized dihydrolipoic acid (DHLA) capped fluorescent gold nanoclusters (DHLA-AuNCs) by the microwave-assisted method for the detection of Hg2+.14 Yang and co-workers utilized folic-acid-capped gold nanoclusters (FA-AuNCs) for the detection of mercury (Hg2+) and methylmercury (CH3Hg+).12 Zhang's group employed inexpensive chicken egg white (EW) as a reducing/capping reagent to develop the chicken egg white-protected gold nanoclusters (EW@AuNCs) with ultra-sensitive detection of Hg2+.15
Besides, metal–organic frameworks (MOFs), which coordinate metal ions with organic ligands, are a class of crystalline microporous materials. MOFs possess fascinating features like high surface areas, tunable pore sizes and controllable surface functionalities; thus, more and more researchers exploit countless applications of MOFs including gas storage and separation, selective catalysis, drug delivery, and sensing.16–20 Until now, various types of MOFs have been widely applied to fabricate different kinds of sensors for analysis and detection. Lin and co-workers encapsulated branched poly-(ethylenimine)-capped carbon quantum dots (BPEI-CQDs) into the zeolitic imidazolate framework materials (ZIF-8) for the detection of Cu2+.21 Bai's group synthesized cysteine-functionalized MIL-101(NH2) for efficient enrichment of N-linked glycopeptides in cell lysate.22 Yi et al. employed a Cy5-labeled 8–17 DNAzyme strand and a Cy3-labeled substrate strand to form a duplex probe adsorbing on the ZIF-8 surface for ratiometric fluorescence imaging of miRNAs.23 Optically functionalized MOFs are the most attention-deserving because the inorganic or organic components can generate fluorescence, and other dimensional fluorescence functionalities could be combined with them to form novel materials.17 Inspired by these studies, combining MIL-68(In)-NH2 with AuNCs, the dual-emission ratiometric fluorescent sensor was proposed. MOFs, as an internal standard, provided a superior surface area, porous structure and fluorescence properties. Simultaneously, the AuNCs, as the response signal fluorophore, presented the sensing selectivity and fluorescence features. MOFs, as a desirable carrier, can enhance the amount of AuNC loading and amplify the signal intensity of AuNCs.16 To further enhance the sensitivity of the sensor for the detection of Hg2+, cysteine (Cys) was introduced to improve the fluorescence intensity of the sensor. It was worth noting that Cys could decrease the surface defects of AuNCs and played an important role in the sensing process due to its thiol groups (–SH).24 Therefore, the dual-emission ratiometric fluorescence sensing platform, constructed of AuNCs/MIL-68(In)-NH2/Cys, may present high sensing sensitivity and selectivity to various analytes.
Ratiometric fluorescent sensors are very suitable for the construction of small special equipment, which is easy to prepare, cheap, convenient, and highly sensitive.25 Currently, microfluidic paper analytical devices (μPADs), first introduced by the Whitesides group in 2007,26 as an innovative low-cost platform, have found applications in multiplexed analyte detection. μPADs are remarkable since porous hydrophilic paper as the three-dimensional (3D) microfluidic substrate makes liquid flow by capillary forces without the need to pump.27,28 Meanwhile, hydrophobic barriers could identify liquid flow routes by depositing a hydrophobic material.29 To confine liquid flow pathways, various paper designing methods, which have different channels, have been reported, such as inkjet printing,30 wax printing31 and screen-printing.32 Wax printing, as the most attractive technique, possesses simply patterned paper and is of relatively low cost. After the reagents are modified and flowed through the hydrophilic region and the liquid has dried, the μPAD can be used for qualitative and quantitative chemical analyses, like high-throughput ion screening.
In this work, AuNCs/MIL-68(In)-NH2/Cys were first synthesized by conjugating GSH-AuNCs and MIL-68(In)-NH2, with a double fluorescence emission peak, which was accompanied by fluorescent AuNCs incorporated into fluorescent MIL-68(In)-NH2 resulting in light pink fluorescence emission. The produced nanosensors after modification by cysteine further enhanced fluorescence emission at 668 nm of GSH-AuNCs and presented bright pink fluorescence. The sensor greatly reduced background signals and extremely improved sensitivity and selectivity. Meanwhile, the nanosensors had the advantages of Hg2+ detection (Scheme 1A). The ratiometric fluorescent sensor exhibited a lower LOD of 6.7 pM with a wide linear range of 20 pM–0.2 μM and 0.2 μM–60 μM for Hg2+ detection. Based on this, we developed a microfluidic paper-based analytical device (μPAD)26 to achieve straightforward selection of different ions and detection of different concentrations of Hg2+ (Scheme 1B). Correspondingly, the μPAD was designed to be radially star-shaped with twelve detection reservoirs and six detection reservoirs. Furthermore, the time required for selective screening and Hg2+ detection did not exceed 10 s. The ratiometric fluorescent μPAD presented obvious color changes and had a wide Hg2+ concentration detection range from 5 nM to 50 μM. The observed good selectivity and sensitivity confirmed that the ratiometric fluorescent sensor can be further applied as a low-cost and attractive ion-screening μPAD to achieve further faster ion detection.
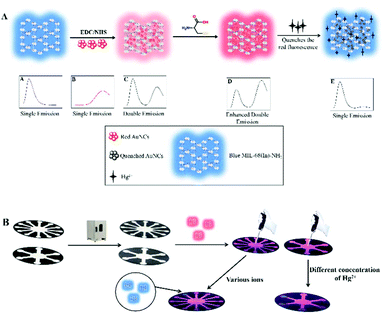 |
| Scheme 1 (A) Schematic illustration of ratiometric fluorescent composites and the principles of the detection of Hg2+. (B) The fabrication process of the μPAD for the detection of various ions and different concentrations of Hg2+. | |
2. Experimental section
2.1 Chemicals and materials
Chloroauric acid (HAuCl4·4H2O), Cysteine (Cys), anhydrous methanol (CH3OH, ≥99.5%), Nitric acid (HNO3, ≥99.5%) and N,N-dimethylformamide (DMF, ≥99.5%) were purchased from Shanghai Sinopharm Chemical Reagent Co. Ltd (Shanghai, China). 2-Aminoterephthalic acid (H2ATA, 99%) was received from Energy Chemical. N-Hydroxysuccinimide (NHS, 98%) was purchased from Sigma-Aldrich. 1-(3-Dimethylaminopropyl)-3-ethylcarbodiimide hydrochloride (EDC, 99%), Glutathione (GSH) and Indium nitrate hydrate (In(NO3)3·xH2O, 99.99%) were obtained from Aladdin. Phosphate buffer solutions (PBS, pH 5.0–11.0, 50 mM) were freshly prepared by varying the ratio of NaH2PO4·2H2O to Na2HPO4·12H2O before use. All chemicals and solvents were of analytical grade and were used without further purification. Double distilled water was used throughout.
2.2 Instrumentation and characterization
UV-vis absorption spectroscopy was performed on a UV-2600 spectrophotometer (Shimadzu Co.). The Fluoromax-4 fluorescence spectrofluorometer (Horiba, USA) was applied for fluorescence (FL) measurements. Transmission electron microscopy (TEM) measurements were conducted on a JEM-2100 transmission electron microscope (JEOL Ltd). Scanning electron microscopy (SEM) images were obtained using a FEI Sirion 200 scanning electron microscope. Zeta potential measurements were performed with a Marlvern Zetasizer (Nano ZS). The FT-IR spectrum was recorded using a Nicolet 5700 (USA) IR spectrometer in the range of 400–4000 cm−1. The designed μPADs were printed by using a wax printer (ColorQube 8580N, Xerox, CT) on the filter paper (210 mm × 297 mm).
2.3 Synthesis of GSH-Au NCs
According to our previous work, the glutathione-stabilized gold nanoclusters (GSH-AuNCs) were synthesized with a slight modification.33 The mixture of GSH (15 mM, 5 mL), and HAuCl4·4H2O (1%, 2.06 mL) was under stirring for 2 min. After the addition of NaOH (1 M, 1 mL), the solution was incubated at 37 °C for 24 h. After washing with anhydrous methanol, the products were dried under vacuum, redispersed in double distilled water and stored at 4 °C for later use.
2.4 Synthesis of MIL-68(In)-NH2
The indium-based metal–organic framework (MIL-68(In)-NH2) was prepared by a solvothermal method by a little modification of the previous report.34 In brief, the mixture of In(NO3)3·xH2O and H2ATA (the molar ratio between In(NO3)3·xH2O and H2ATA was 3
:
1) was added into DMF (6.2 mL), and the reaction solution was maintained at 125 °C for 5 h. After cooling to room temperature and washing several times with anhydrous methanol to remove the guest molecules in the pores, the samples were dried under vacuum at 100 °C for 12 h.
2.5 Synthesis of Au NCs/MIL-68(In)-NH2 hybrid and cysteine enhanced the fluorescence
For the synthesis of the dual-emission ratiometric fluorescent nanocomposites from GSH-Aul, NCs and MIL-68(In)-NH2 (AuNCs/MIL-68(In)-NH2), the red emissive AuNCs were subsequently conjugated to MIL-68(In)-NH2. Firstly, EDC/NHS solution was added to activate the carboxylic groups on the GSH-AuNC surface. Then, the MIL-68(In)-NH2 suspension was supplied (the quality ratio between GSH-AuNCs and MIL-68(In)-NH2 was 100
:
1). Next, the mixture was kept shaking for 8 h in the dark. The dual-emission ratiometric fluorescent composites from AuNCs/MIL-68(In)-NH2 modified with Cys (AuNCs/MIL-68(In)-NH2/Cys) could be enhanced, which resulted from the thiol groups (–SH) of Cys that were bonded to the surface of AuNCs. Typically, the Cys solution (the quality ratio between AuNCs/MIL-68(In)-NH2 and Cys was 1
:
0.8) was added into the above solution and incubated at 40 °C under static conditions without stirring for 40 min. After cooling down, the AuNCs/MIL-68(In)-NH2/Cys hybrid was dialyzed for 24 h with a dialysis membrane (100
000 MWCO). The visual color changes could be observed under a UV lamp (excitation wavelength at 365 nm). Followed by dialysis with deionized water, the sample could be taken directly for the detection of Hg2+ and visual detection on the paper could be achieved.
2.6 Detection of Hg2+ ions
To obtain a high sensitivity for Hg2+ detection, the pH value range from 5 to 11 was optimized through monitoring the emission intensity of the produced AuNCs/MIL-68(In)-NH2 and AuNCs/MIL-68(In)-NH2/Cys. Because Hg2+ tends to hydrolyze strongly in water, the solution of Hg2+ was prepared by dissolving an appropriate amount of Hg(NO3)2 in 0.1 M HNO3 for the experiments.35 All the fluorescence detection procedures were conducted in phosphate buffer solution (PBS, 5 mM, pH = 7.4, the most suitable condition) at room temperature. Comprehensively, adding different concentrations of Hg2+ solution into a series of 200 μL of the above dispersion makes the concentration gradient of the Hg2+ range from 0 μM to 60 μM. The final volume of the sample was adjusted to 500 μL with double distilled water, and then the mixture was shaken gently for 30 s, and the fluorescence spectra of the system were measured at an excitation wavelength of 370 nm.
2.7 Selectivity and interference experiments
To examine the selectivity of AuNCs/MIL-68(In)-NH2/Cys probes to Hg2+, the fluorescence intensity ratios (FL668 nm/FL438 nm) of AuNCs/MIL-68(In)-NH2/Cys exposed to other competitive metal ions, such as Cd2+, Zn2+, Cu2+, Fe3+, Co2+, Pb2+, Cr3+, Fe3+, Mg2+, Sn2+, and Ni2+, were studied under the same conditions. A volume of 200 μL of the above AuNCs/MIL-68(In)-NH2/Cys solution was mixed with 50 μL PBS solution (50 mM), followed by addition of a series of competitive metal cations. After that, deionized water was added to adjust the final volume (500 μL); the final solution was incubated for 60 s. Among them, the concentrations of Hg2+ were 60 μM and the concentrations of other metal ions were 150 μM. Finally, the mixture was added to a spectrophotometer trace quartz cuvette ready for fluorescence spectra recording. The visual color changes were observed under a UV lamp (excitation wavelength at 365 nm).
2.8 Practical detection of Hg2+ ions in lake and tap water
To assess the capability of the probes, the local lake sample, obtained from the Jiulong Lake, and the tap water have been tested. All water samples had the suspension removed by using 0.22 μm filters before use. After pretreatment, Hg2+ in water samples was detected by spiking known amounts of Hg2+ at four different concentrations (0.05, 40, 1 × 103, 2 × 104 μM) using AuNCs/MIL-68(In)-NH2/Cys as the ratiomeric fluorescent sensor.
2.9 Visual detection of Hg2+ on the μPAD
The microfluidic paper-based analytical device (μPAD) in a 38 mm × 38 mm circle was designed using Adobe Illustrator. A sample reservoir was located at the center and twelve detection reservoirs were arranged regularly to detect various ions. A sample reservoir located at the center but with six star-shaped detection reservoirs was used to detect different concentrations of Hg2+. Details of the design of the μPAD are presented in the ESI (Fig. S12†). As reported by Carrilho and co-workers,31 the designed μPADs were printed on a sheet of filter paper (210 mm × 297 mm) by using a wax printer, and then dried at 150 °C for 50 s in an oven. The drying process caused the wax to pass through the paper, forming a 3D hydrophobic barrier to control the flow of liquid. As shown in Scheme 1B, each well-designed μPAD was cut down, and appropriate volumes of the AuNCs/MIL-68(In)-NH2/Cys were added on the center reservoir until liquid diffused into each detection reservoir. After drying in an oven, the μPAD with 12-branch detection reservoirs was used for selectivity experiments. Namely, 5 μL of different metal ions was dropped on the twelve detection reservoirs. Meanwhile, the μPAD with 6-branch detection reservoirs was used for testing Hg2+ with various concentrations (0, 0.005, 0.05, 0.5, 5 and 50 μM), and the volume was also 5 μL. The visual fluorescence color can be easily observed under a 365 nm UV lamp. The fluorescence photos were taken by using a smartphone (iphone 6s plus). In the process of the experiment, a sheet of filter paper (210 mm × 297 mm) can produce 12 devices simultaneously at a cost of $ 0.0397 each.
3. Results and discussion
3.1 Characterization of GSH-Au NCs, MIL-68(In)-NH2
The prepared red-emitting GSH-AuNCs were characterized by transmission electron microscopy (TEM), which showed clearly both the size and surface morphology. The TEM image in Fig. S1A† revealed that GSH-AuNCs had tiny spherical structures with decent dispersion. Meanwhile, it was clearly observed from HRTEM that the lattice spacing of GSH-AuNCs was 0.231 nm. As shown in Fig. S1B,† the mean diameter was calculated to be 1.6 nm. Such a result was highly consistent with the previous report that the sizes of GSH-AuNCs were observed to be smaller than 2 nm.36–38 Fig. S2† displays a broad absorption before 400 nm but no absorbance peak around 500 nm existed, which demonstrated that highly purified AuNCs have been obtained without big particles or bulk metals as by-products.33 Meanwhile, the product showed a red, broad emission peak centered at 678 nm (Fig. S3†).
The blue-emitting MIL-68(In)-NH2 was characterized by scanning electron microscopy (SEM). The image showed that the composites showed a well-dispersed and willow leaf-like structure, which may be attributed to the formation of building blocks of the MIL-68(In)-NH2 crystals during the synthesis (Fig. S4†).39 The FTIR spectra (Fig. S5†) can confirm successful formation of MIL-68(In)-NH2. The bands at 3490 and 3367 cm−1 were assigned to the symmetric and asymmetric stretching of primary amines. Furthermore, the peak at 1256 cm−1 was attributed to the stretching vibration of N–C.40 Fig S6† depicts that MIL-68(In)-NH2 had a blue fluorescence emission peak centered at 428 nm.
3.2 Characterization of AuNCs/MIL-68(In)-NH2/Cys
The TEM image proved the successful preparation of AuNCs/MIL-68(In)-NH2. Fig. 1A shows the morphology of AuNCs/MIL-68(In)-NH2, which indicated that MIL-68(In)-NH2 maintained a willow leaf-like structure after the incorporation of AuNCs into the MOF framework and the AuNCs were highly dispersed on the MIL-68(In)-NH2 substrate. The successful fabrication of composite materials was certified through zeta potential (Fig. S7†). MIL-68(In)-NH2 synthesized from 2-aminoterephthalic acid retained significant amino groups out of the framework, which induced positive charges at the surface of MIL-68(In)-NH2, and showed a positive charge of 14.1 ± 0.5 mV. Moreover, GSH-AuNCs showed a negative charge of −24.1 ± 1.2 mV. Combining with them, the zeta potential changed to −8.41 ± 2.6 mV. The zeta potential demonstrated that besides the role of covalent binding there might be possible electrostatic binding between GSH-AuNCs and MIL-68(In)-NH2. Moreover, the fluorescence intensity of AuNCs was gradually enhanced with the concentration of MIL-68(In)-NH2 slowly increased (Fig. S8†). Thus, the optimal ratio of AuNCs versus MIL-68(In)-NH2 was 100
:
1. As illustrated in Fig. 1B, the strong fluorescence emission peak of MIL-68(In)-NH2 centered at 428 nm (curve a), and the weak fluorescence emission peak of GSH-AuNCs centered at 678 nm (curve b). Predictably, AuNCs/MIL-68(In)-NH2 showed two obvious fluorescence peaks, the emission intensity of MIL-68(In)-NH2 decreased accompanied by the red shift to 438 nm, whereas the emission intensity of GSH-AuNCs increased double significantly with the blue shift to 663 nm (curve c). The porous structure and the large specific surface area of the MIL-68(In)-NH2, result in the fluorescence enhancement of AuNCs. Besides, the inset images of Fig. 1B showed the red AuNCs, the blue MIL-68(In)-NH2 and the light pink AuNCs/MIL-68(In)-NH2 under UV (365 nm) illumination (from left to right corresponding to the three products). After cysteine (Cys) functionalization, as demonstrated in Fig. 1C, the TEM images of AuNCs/MIL-68(In)-NH2/Cys verified that the framework showed no obvious change, while the size of AuNCs was clearly enlarged with good dispersion. It also proved the successful integration of AuNCs and cysteine. Fig. 1D shows that the peak of GSH-AuNCs at 668 nm was enhanced 2.6 times remarkably followed by the red shift 5 nm under the same excitation of 370 nm, while the emission intensity of MIL-68(In)-NH2 at 438 nm remained stable and slightly enhanced. The formed AuNCs/MIL-68(In)-NH2/Cys exhibited bright pink fluorescence (inset of Fig. 1D). Because of –SH of Cys bonded with the Au+ in the AuNCs, Cys adsorbed on the surface of AuNCs, resulting in a further dramatic increase of the fluorescence intensity, while Cys can be tied to the amino-group on MIL-68(In)-NH2 by its carboxyl group terminal, which induced slight strengthening of fluorescence emission at 438 nm via the restriction of intramolecular rotation (RIR) mechanism.41 The two emission peaks were well separated by a wavelength distance of 230 nm, enhancing possibilities and sensitivity for ratiometric fluorescence detection.
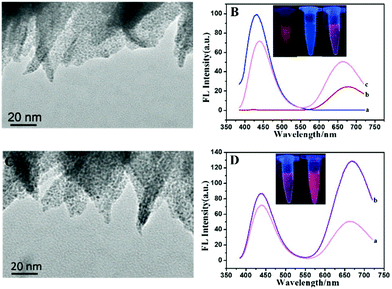 |
| Fig. 1 (A) TEM image and (B) fluorescence emission spectra of AuNCs/MIL-68(In)-NH2. Inset: Photographs of AuNCs, MIL-68(In)-NH2 and AuNCs/MIL-68(In)-NH2 under UV light (365 nm). (C) TEM image and (D) fluorescence emission spectra of AuNCs/MIL-68(In)-NH2/Cys. Inset: Photographs of Au NCs/MIL-68(In)-NH2 and AuNCs/MIL-68(In)-NH2/Cys under UV light (365 nm). | |
By monitoring the fluorescence emission intensity of the produced AuNCs/MIL-68(In)-NH2/Cys, the reaction time, temperature and the ratio of reactants were optimized, respectively. The results showed that incubation for 40 min at 40 °C of AuNCs/MIL-68(In)-NH2 with cysteine served as the optimal conditions for the synthesis of AuNCs/MIL-68(In)-NH2/Cys (Fig. S9†). The fluorescence intensity gradually stabilized with the increase of incubation time. However, after 40 °C, the fluorescence intensity decreased with the increase of temperature. The phenomenon can be attributed to higher temperature which was harmful to the reaction between cysteine and AuNCs, while the GSH on the surface of AuNCs would denature and aggregate when the reaction temperature exceeded 70 °C.24 It could be also found that the ratio at 1
:
0.8 of AuNCs/MIL-68(In)-NH2 to cysteine served as the optimal condition for the synthesis of AuNCs/MIL-68(In)-NH2/Cys (Fig. S10†).
3.3 Detection of Hg2+ using the AuNCs/MIL-68(In)-NH2/Cys
In the absence of Hg2+, the AuNCs/MIL-68(In)-NH2/Cys emitted double well-resolved fluorescence emission (peak at 438 nm and 668 nm) under a single wavelength excitation in optimized phosphate buffer solution (PBS, 5 mM, pH = 7.4) (Fig. S11†). Next, the dose–response of AuNCs/MIL-68(In)-NH2/Cys to Hg2+ has been analyzed, when a certain concentration of Hg2+ was added, the red fluorescence was quenched and blue fluorescence of MIL-68(In)-NH2 was only presented. Regarding the fluorescence quenching mechanism, it was mainly caused by the heavy metal ion effect between Hg2+ (4f145d10) and Au+ (4f145d10).42–45 Moreover, owing to Cys that reacted with thiophilic Hg2+ and generated stable HS-Hg2+,46 the fluorescence intensity at 668 nm of the sensors could also be reduced. Fig. 2A shows that in the presence of Hg2+ at the concentration ranging from 0 to 60 μM, the red fluorescence peak at 668 nm was continuously quenched, while the blue fluorescence peak at 438 nm was kept almost steady. When the concentration of Hg2+ was close to 60 μM, the emission intensity at 668 nm was quenched completely. Meanwhile, the fluorescence emission intensity at 438 nm was changed very slightly, which might be affected by thiophilic Hg2+ reaction with cysteine. As depicted in Fig. 2B, the fluorescence intensity ratios FL668 nm/FL438 nm displayed a curve relationship versus the concentration of Hg2+ in the range of 0–60 μM, where FL668 nm and FL438 nm represented the FL intensities at 668 nm and 438 nm, respectively. The calibration curve was constructed under optimum conditions. Fig. 2C shows a good linearity response ranging from 20 pM to 200 nM with a correlation coefficient (R2) of 0.993. The equation of linear regression could be expressed as: Y = 1.577 − 2.269X. Fig. 2D also presents a good linearity curve between 200 nM and 60 μM with R2 at 0.998. The equation presented as: Y = 1.125 − 0.014X. In the above equations, X stands for the concentration of Hg2+ and Y stands for FL668 nm/FL438 nm. The limit of detection (LOD) was estimated to be 6.7 pM, at a signal-to-noise ratio (S/N) of 3, which is much lower than that of the maximum contamination level of Hg2+ in drinking water permitted by the World Health Organization (WHO, 6 ppb) and the U.S. Environmental Protection Agency (EPA, 2 ppb).6 The methods of detection of Hg2+ by other fluorescent probes are shown in Table 1. The resulting dual-emission ratiometric fluorescent composites provided a wide linear range and a low LOD for the detection of Hg2+, which were much better than those previously reported,15,47–52 especially, the detection of Hg2+ adopted various AuNCs.
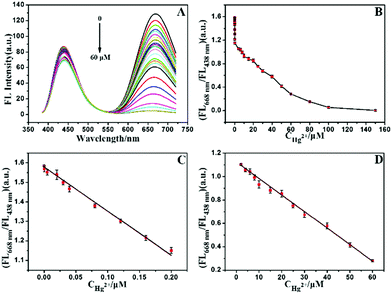 |
| Fig. 2 (A) Fluorescence emission spectra of AuNCs/MIL-68(In)-NH2/Cys in the presence of Hg2+ with different concentrations (0, 2 × 10−4, 5 × 10−3, 0.02, 0.03, 0.04, 0.08, 0.12, 0.16, 0.2,2, 4, 6, 8, 10, 15, 20, 25, 30, 40, 50 and 60 μM). (B) Plot of the fluorescence intensity ratio (FL668 nm/FL438 nm) versus the concentration of Hg2+. (C) The linear relationship between FL668 nm/FL438 nm and the concentration of Hg2+ ranging from 20 pM to 0.2 μM. (D) The linear relationship between FL668 nm/FL438 nm and the concentration of Hg2+ ranging from 0.2 μM to 60 μM. The error bars represent the standard deviation of three measurements (λex = 370 nm, slit: 5/5 nm). | |
Table 1 Comparisons of the linear range and detection limit for Hg2+ with other materials
Materials |
Linear ranges (nM) |
Detection limit (LOD) (nM) |
Ref. |
EW@AuNCs |
0.0446–5.9 |
0.02 |
15
|
BSA-AuNCs |
1–20 |
9.8 × 10−3 |
47
|
Metal ion-TSDRs |
0.1–100 |
0.065 |
48
|
Lysozyme-AuNCs |
10–5 × 103 |
10 |
49
|
Mn-CDs |
100–5 × 104 |
1.6 |
50
|
BCDs and TGA-CdTe |
10–1.4 × 103 |
4.6 |
51
|
Thymine-CDs |
0–1.0 × 106 |
3.5 × 10−8 |
52
|
AuNCs/MIL-68(In)-NH2/Cys |
0.02–200, 200–6 × 104 |
1.7 × 10−3 |
Our method |
3.4 Selectivity of Au NCs/MIL-68(In)-NH2/Cys for detecting metal ions
When applied in real analysis, the selectivity of AuNCs/MIL-68(In)-NH2/Cys to the target metal ions over other pertinent metal ions (including Cd2+, Zn2+, Cu2+, Fe3+, Co2+, Pb2+, Cr3+, Fe3+, Mg2+, Sn2+, and Ni2+) was essential.53 In the experiment, other metal ions were 150 μM and Hg2+ was 60 μM under maximum concentration. The respective nitrate or chloride salts were prepared for stock solutions of other metal ions. The results of Fig. 3A depicted that only Hg2+ can induce a drastic decrease (FL668 nm/FL438 nm) of AuNCs/MIL-68(In)-NH2/Cys. In contrast, other ions led to little fluorescence intensity change, even the concentration of other ions was higher than that of Hg2+. Photographs of the aqueous solutions of the AuNCs/MIL-68(In)-NH2/Cys after the addition of various ions under irradiation by UV light at 365 nm are given in Fig. 3B. Obviously, only Hg2+ could quench the red fluorescence and show the blue fluorescence. The experimental results showed that the proposed probe in this paper has high selectivity for distinguishing Hg2+.
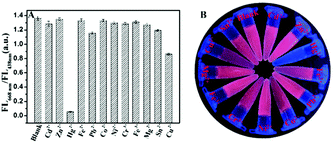 |
| Fig. 3 (A) Fluorescence intensity ratios (FL668 nm/FL438 nm) of AuNCs/MIL-68(In)-NH2/Cys to various metal ions. The bars represent the addition of different ions. The concentrations of different metal ions were 150 μM, except Hg2+ at 60 μM. (B) The image shows the corresponding fluorescence colors under a UV lamp (365 nm). | |
3.5 Application in real samples
To assess the practicality of the proposed method to real samples, standard addition and recovery experiments were performed via the proposed sensing probe. Hg2+ standard solutions at known concentrations were spiked into the lake and tap samples, respectively. As listed in Table 2, the recoveries of all samples are in the range of 91.3–110.2% with a satisfactory consequence. In addition, the RSD definitely also indicated that the high accuracy and reliability could be achieved by the present sensing method. The fluorescent sensor based on AuNCs/MIL-68(In)-NH2/Cys confirmed that the proposed method could achieve practical applications to detect Hg2+ in real samples.
Table 2 Results of the detection of Hg2+ in Hg2+-spiked real water samples using the ratiometric fluorescent probe AuNCs/MIL-68(In)-NH2/Cys
Sample |
Hg2+ spiked (nM) |
Hg2+ found (nM) |
Recovery (%) |
RSD (%) |
Lake |
0.05 |
0.0546 |
109.2 |
2.6 |
40 |
0.039 × 103 |
97.5 |
2.3 |
1 × 103 |
1.102 × 103 |
110.2 |
3.4 |
|
Tap |
2 × 104 |
1.994 × 104 |
99.7 |
1.5 |
0.05 |
0.0482 |
96.4 |
2.8 |
40 |
0.043 × 103 |
107.5 |
3.7 |
1 × 103 |
0.913 × 103 |
91.3 |
2.5 |
2 × 104 |
1.927 × 104 |
96.4 |
1.9 |
3.6 Visual detection of Hg2+ on the μPAD
To further achieve rapidity and simplicity for detection, the new detection platform, microfluidic paper-based analytical device (μPAD), was selected to visually detect ions (Fig. S12†). The μPAD with twelve detection reservoirs (Fig. S13A†) was used for detecting various ions. Fig. S13B† shows that the μPAD presented light yellow after AuNCs/MIL-68(In)-NH2/Cys was added and dried. Apparently, the μPAD with six detection reservoirs (Fig. S13C†), which was used for detecting Hg2+ at different concentrations, possessed the same light yellow color after treatment (Fig. S13D†). Under a 365 nm UV lamp, Fig. 4A and B present the selectivity of AuNCs/MIL-68(In)-NH2/Cys on the μPAD to the target metal ions over other pertinent metal ions under the same conditions. Obviously, only treated with Hg2+, the detection reservoir showed red fluorescence quenching and retained blue fluorescence. Fig. 4C and D indicate that the fluorescence conversion of AuNCs/MIL-68(In)-NH2/Cys towards different concentrations of Hg2+. With the increasing concentration of Hg2+ (0, 0.005, 0.05, 0.5, 5 and 50 μM), the red fluorescence appeared regularly quenching and there was increasingly strong blue fluorescence. All of these results confirmed that the μPAD provided a convenient and straightforward fluorescence platform for Hg2+ detection with high selectivity and sensitivity.
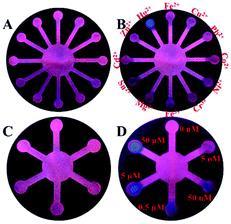 |
| Fig. 4 Photographs of the μPAD (A) with the AuNCs/MIL-68(In)-NH2/Cys, (B) and with the AuNCs/MIL-68(In)-NH2/Cys responding to various metal ions under 365 nm UV light. The types of the ions are Fe2+, Cu2+, Pb2+, Co2+, Ni2+, Cr3+, Fe3+, Mg2+, Sn2+, Cd2+, Zn2+, and Hg2+ along the clockwise direction. Photographs of the μPAD (C) with the AuNCs/MIL-68(In)-NH2/Cys under 365 nm UV light, (D) and with AuNCs/MIL-68(In)-NH2/Cys responding to Hg2+ under 365 nm UV light. The concentrations of the Hg2+ solutions are 0, 0.005, 0.05, 0.5, 5 and 50 μM along the clockwise direction. | |
4. Conclusion
In summary, the ratiometric fluorescent nanosensors were successfully developed, and AuNCs/MIL-68(In)-NH2/Cys was constructed by AuNCs incorporated into MIL-68(In)-NH2, and then further modified with Cys. Because MIL-68(In)-NH2 has high porosity and specific surface area and Cys could reduce surface defects of AuNCs, the as-prepared AuNCs/MIL-68(In)-NH2/Cys displayed bright pink fluorescence with double fluorescence emission peaks at 438 nm and 668 nm, and well-dispersed AuNCs. The fluorescence emission at 668 nm was enhanced 5.2 times remarkably compared to GSH-AuNCs. It was found that the enhanced pink fluorescence of AuNCs/MIL-68(In)-NH2/Cys could be quenched by Hg2+ and two wide linear ranges from 20 pM to 0.2 μM and 0.2 μM to 60 μM with a lower detection limit of 8.7 nM were obtained. The sensor was successfully applied to recognize Hg2+ in real water samples with high levels of recoveries (91.3–110.2%). Additionally, the microfluidic paper-based analytical device (μPAD) was successfully fabricated to rapidly screen different ions with excellent selectivity and has been successfully applied to detect different concentrations of Hg2+ with the detection range from 5 nM–50 μM. Furthermore, we believe that the developed dual-emission fluorescent sensor and ratiometric fluorescent μPAD, with its satisfactory simplicity and sensitivity, would be propitious for applications in practical environmental analysis and biosensors.
Conflicts of interest
There are no conflicts to declare.
Acknowledgements
This work was supported by the National Key Research and Development Program of China (2017YFA0700404), the National Natural Science Foundation of China (21535003 and 21575022), the Key Research & Development Plan of Jiangsu Province (BE2018617), Qing Lan Project and Key Laboratory of Optic-electric Sensing and Analytical Chemistry for Life Science, MOE, Qingdao University of Science and Technology (STAM201801).
Notes and references
- Y.-X. Qi, M. Zhang, A. Zhu and G. Shi, Analyst, 2015, 140, 5656–5661 RSC.
- Y.-C. Lu, J. Chen, A.-J. Wang, N. Bao, J.-J. Feng, W. Wang and L. Shao, J. Mater. Chem. C, 2015, 3, 73–78 RSC.
- G. Sener, L. Uzun and A. Denizli, Anal. Chem., 2014, 86, 514–520 CrossRef CAS PubMed.
- D. T. Quang and J. S. Kim, Chem. Rev., 2010, 110, 6280–6301 CrossRef CAS PubMed.
- D. W. Boening, Chemosphere, 2000, 40, 1335–1351 CrossRef CAS PubMed.
- Z.-X. Wang and S.-N. Ding, Anal. Chem., 2014, 86, 7436–7445 CrossRef CAS PubMed.
- J. Allibone, E. Fatemian and P. J. Walker, J. Anal. At. Spectrom., 1999, 14, 235–239 RSC.
- H. Erxleben and J. Ruzicka, Anal. Chem., 2005, 77, 5124–5128 CrossRef CAS PubMed.
- Y. Yu, Z. Du, M. Chen and J. Wang, Angew. Chem., 2008, 120, 8027–8030 CrossRef.
- Y. Ding, S. Wang, J. Li and L. Chen, TrAC, Trends Anal. Chem., 2016, 82, 175–190 CrossRef CAS.
- E. Saouter and B. Blattmann, Anal. Chem., 1994, 66, 2031–2037 CrossRef CAS.
- J.-Y. Yang, T. Yang, X.-Y. Wang, M.-L. Chen, Y.-L. Yu and J.-H. Wang, Anal. Chem., 2018, 90, 6945–6951 CrossRef CAS PubMed.
- L.-Y. Chen, C.-W. Wang, Z. Yuan and H.-T. Chang, Anal. Chem., 2015, 87, 216–229 CrossRef CAS PubMed.
- L. Shang, L. Yang, F. Stockmar, R. Popescu, V. Trouillet, M. Bruns, D. Gerthsen and G. U. Nienhaus, Nanoscale, 2012, 4, 4155–4160 RSC.
- Y. Zhang, J. Jiang, M. Li, P. Gao, L. Shi, G. Zhang, C. Dong and S. Shuang, Sens. Actuators, B, 2017, 238, 683–692 CrossRef CAS.
- X. Yang, Y.-Q. Yu, L.-Z. Peng, Y.-M. Lei, Y.-Q. Chai, R. Yuan and Y. Zhuo, Anal. Chem., 2018, 90, 3995–4002 CrossRef CAS PubMed.
- Y. Cui, Y. Yue, G. Qian and B. Chen, Chem. Rev., 2012, 112, 1126–1162 CrossRef CAS PubMed.
- N. L. Rosi, J. Eckert, M. Eddaoudi, D. T. Vodak, J. Kim, M. Keeffe and O. M. Yaghi, Science, 2003, 300, 1127 CrossRef CAS PubMed.
- M. D. Allendorf, C. A. Bauer, R. K. Bhakta and R. J. T. Houk, Chem. Soc. Rev., 2009, 38, 1330–1352 RSC.
- F. Cao, E. Ju, C. Liu, W. Li, Y. Zhang, K. Dong, Z. Liu, J. Ren and X. Qu, Nanoscale, 2017, 9, 4128–4134 RSC.
- X. Lin, G. Gao, L. Zheng, Y. Chi and G. Chen, Anal. Chem., 2014, 86, 1223–1228 CrossRef CAS PubMed.
- W. Ma, L. Xu, X. Li, S. Shen, M. Wu, Y. Bai and H. Liu, ACS Appl. Mater. Interfaces, 2017, 9, 19562–19568 CrossRef CAS PubMed.
- J.-T. Yi, T.-T. Chen, J. Huo and X. Chu, Anal. Chem., 2017, 89, 12351–12359 CrossRef CAS PubMed.
- M.-L. Cui, J.-M. Liu, X.-X. Wang, L.-P. Lin, L. Jiao, L.-H. Zhang, Z.-Y. Zheng and S.-Q. Lin, Analyst, 2012, 137, 5346–5351 RSC.
- J. F. da Silveira Petruci and A. A. Cardoso, Anal. Chem., 2016, 88, 11714–11719 CrossRef PubMed.
- A. W. Martinez, S. T. Phillips, M. J. Butte and G. M. Whitesides, Angew. Chem., Int. Ed., 2007, 46, 1318–1320 CrossRef CAS PubMed.
- H. Liu and R. M. Crooks, J. Am. Chem. Soc., 2011, 133, 17564–17566 CrossRef CAS PubMed.
- T. Akyazi, A. Tudor, D. Diamond, L. Basabe-Desmonts, L. Florea and F. Benito-Lopez, Sens. Actuators, B, 2018, 261, 372–378 CrossRef CAS.
- Y. S. Kim, Y. Yang and C. S. Henry, Sens. Actuators, B, 2018, 255, 3654–3661 CrossRef CAS PubMed.
- K. Abe, K. Suzuki and D. Citterio, Anal. Chem., 2008, 80, 6928–6934 CrossRef CAS PubMed.
- E. Carrilho, A. W. Martinez and G. M. Whitesides, Anal. Chem., 2009, 81, 7091–7095 CrossRef CAS PubMed.
- S. Wang, L. Ge, X. Song, J. Yu, S. Ge, J. Huang and F. Zeng, Biosens. Bioelectron., 2012, 31, 212–218 CrossRef CAS PubMed.
- C.-L. Zheng, Z.-X. Ji, J. Zhang and S.-N. Ding, Analyst, 2014, 139, 3476–3480 RSC.
- L. Wu, M. Xue, S.-L. Qiu, G. Chaplais, A. Simon-Masseron and J. Patarin, Microporous Mesoporous Mater., 2012, 157, 75–81 CrossRef CAS.
- J. Yao, K. Zhang, H. Zhu, F. Ma, M. Sun, H. Yu, J. Sun and S. Wang, Anal. Chem., 2013, 85, 6461–6468 CrossRef CAS PubMed.
- Y. Yu, Z. Luo, D. M. Chevrier, D. T. Leong, P. Zhang, D.-E. Jiang and J. Xie, J. Am. Chem. Soc., 2014, 136, 1246–1249 CrossRef CAS PubMed.
- Z. Luo, V. Nachammai, B. Zhang, N. Yan, D. T. Leong, D.-E. Jiang and J. Xie, J. Am. Chem. Soc., 2014, 136, 10577–10580 CrossRef CAS PubMed.
- R. Jin, Nanoscale, 2010, 2, 343–362 RSC.
- Y. Pi, X. Li, Q. Xia, J. Wu, Z. Li, Y. Li and J. Xiao, Nano Res., 2017, 10, 3543–3556 CrossRef CAS.
- R. Liang, L. Shen, F. Jing, W. Wu, N. Qin, R. Lin and L. Wu, Appl. Catal., B, 2015, 162, 245–251 CrossRef CAS.
- T. Du, H. Zhang, J. Ruan, H. Jiang, H.-Y. Chen and X. Wang, ACS Appl. Mater. Interfaces, 2018, 10, 12417–12423 CrossRef CAS PubMed.
- P. Pyykkö, Angew. Chem., Int. Ed., 2004, 43, 4412–4456 CrossRef PubMed.
- J. Xie, Y. Zheng and J. Y. Ying, Chem. Commun., 2010, 46, 961–963 RSC.
- X. Xu, Y.-F. Li, J. Zhao, Y. Li, J. Lin, B. Li, Y. Gao and C. Chen, Analyst, 2015, 140, 7841–7853 RSC.
- J.-J. Feng, H. Huang, D.-L. Zhou, L.-Y. Cai, Q.-Q. Tu and A.-J. Wang, J. Mater. Chem. C, 2013, 1, 4720–4725 RSC.
- K. Severin, R. Bergs and W. Beck, Angew. Chem., Int. Ed., 1998, 37, 1634–1654 CrossRef PubMed.
- I. Onyido, A. R. Norris and E. Buncel, Chem. Rev., 2004, 104, 5911–5930 CrossRef CAS PubMed.
- S. Wang, X. Li, J. Xie, B. Jiang, R. Yuan and Y. Xiang, Sens. Actuators, B, 2018, 259, 730–735 CrossRef CAS.
- H. Wei, Z. Wang, L. Yang, S. Tian, C. Hou and Y. Lu, Analyst, 2010, 135, 1406–1410 RSC.
- Q. Xu, R. Su, Y. Chen, S. Theruvakkattil Sreenivasan, N. Li, X. Zheng, J. Zhu, H. Pan, W. Li, C. Xu, Z. Xia and L. Dai, ACS Appl. Nano Mater., 2018, 1, 1886–1893 CrossRef CAS.
- F. Ghasemi, M. R. Hormozi-Nezhad and M. Mahmoudi, Sens. Actuators, B, 2018, 259, 894–899 CrossRef CAS.
- Y. Li, Z.-Y. Zhang, H.-F. Yang, G. Shao and F. Gan, RSC Adv., 2018, 8, 3982–3988 RSC.
- C. Zou, M. F. Foda, X. Tan, K. Shao, L. Wu, Z. Lu, H. S. Bahlol and H. Han, Anal. Chem., 2016, 88, 7395–7403 CrossRef CAS PubMed.
Footnote |
† Electronic supplementary information (ESI) available. See DOI: 10.1039/c8an02414f |
|
This journal is © The Royal Society of Chemistry 2019 |