DOI:
10.1039/C8AN01801D
(Tutorial Review)
Analyst, 2019,
144, 2480-2497
Fluorescent organic nanoparticles (FONs) as convenient probes for metal ion detection in aqueous medium
Received
18th September 2018
, Accepted 21st February 2019
First published on 25th February 2019
Abstract
Recently, water contamination caused by metal ions has become one of the most serious problems as it has caused several deaths and socioeconomic problems around the world. Hence, the fast and accurate detection of metal ions in aqueous media has become the most important area of research; from time to time, new probes have been designed for this purpose. Among the previously reported sensors, probes based on fluorescent organic nanoparticles (FONs) have been gaining tremendous attention due to their ease of preparation/fabrication, synthetic diversity according to targeted metal ions, quick response, high selectivity toward different analytes at lower concentrations, tenable optical properties, and less toxicity. This review comprises two main sections, wherein we have tried to summarize the key progresses made in this field. The first section summarizes the literature dealing with FON-based chemosensors, which are used for the detection of transition metal ions of silver, copper, chromium, cadmium, mercury, iron, and zinc. The second section focuses on FON-based chemosensors that have been used for the detection of main group metal ions, namely, cesium, aluminum, strontium, lithium, and tin. Further, this review provides an adequate amount of information about the mechanism of metal ion sensing with FONs. It is expected that this review can provide sufficient information about this area of research and will be useful in fruitful progress in this field in the future.
1. Introduction
Metal ions play a very important role in life processes and they are used by all types of organisms to perform essential life functions.1,2 It is estimated that in the human body, nearly 30% of enzymes use metal ions in some way.3 In vital biological processes such as transmission of nerve impulses,4 muscle contraction,5,6 cell activity,7 and other processes,8,9 sodium, magnesium, potassium, and calcium play very important roles. In medicine, it is imperative to regulate the serum levels of lithium in patients under treatment for manic depression10 and potassium in cases of high blood pressure.11 The toxicity of aluminum has long been recognized and there is a controversy about its possible implication in Alzheimer's disease.12,13 In chemical oceanography, it has been verified that certain nutrients are absolutely necessary for the survival of microorganisms in seawater, such as zinc, iron, and manganese.14,15 In spite of the fact that metal ions are very essential for sustaining life, their extensive use can cause serious health and environmental issues. For example, certain heavy metal ions such as Fe3+, Zn2+, Cu2+, Co2+, Mn2+, and Mo6+ and their higher concentrations can mainly cause several serious health problems.16,17 Further, there are certain other heavy metal ions, namely, Hg2+, Cd2+, Pb2+, and As3+, which are investigated as highly toxic ions and are responsible for numerous health issues.18 Accordingly, the determination of trace amounts of metal ions is of increasing interest in several fields such as environmental analysis, process control, biology, medicine, etc.
So far, several methods such as atomic absorption/emission spectroscopy,19–21 inductively coupled plasma mass spectroscopy,22,23 inductively coupled plasma atomic emission spectroscopy,24 electrochemical assays,25 Auger electron spectroscopy,26 polarography,27 and recently formulated colorimetric methods28,29 have been developed for the detection of metal ions. Their practical applications are inadequate because these techniques need sophisticated instrumentation; however, in the last two decades, fluorescent-mediated probes to detect metal ions have gained tremendous attention owing to their promising photophysical properties. For instance, their fluorophores can be altered by several ways: charge transfer, electron transfer, energy transfer, influence of metal ions, and destabilization of nonemissive n–π* excited states.30,31
Subsequently, many fluorophores have been used for sensor improvement in the past, but problems like narrow excitation spectra and broad emission spectra are associated with such fluorophores;32 further, these probes work exceptionally well in organic solvents, but have limited applications in aqueous media.33 Sensor activities in nonaqueous media sometimes restrict their use in real-life applications, for example, in environmental and biological samples. However, in recent times, fluorescent organic nanoparticles (FONs) have been progressively used to overcome the shortcomings of traditional fluorogenic sensors for cations. FON-based probes are more beneficial as compared to traditional fluorophores due to their tenable photoluminescence, promising assortment of flexibility in material synthesis, good water solubility, availability of organic molecules, superiority of biodegradation, biocompatibility, photostability, and optical properties depending upon the size of the nanoparticles.34,35 Further, most of the fluorescent chemosensors are used in solution because of which they suffer from problems such as blinking, photobleaching, and intractable self-quenching. By comparison, FONs are a new class of chemosensors that provide additional results as compared to molecule-based probes. Some FONs show the aggregation-induced emission enhancement (AIEE) effect. The active AIEE molecules have a generic feature in which they exhibit low or no fluorescence when freely dissolved in a solvent. However, upon the addition of a solvent (which reduces their solubility), the fluorescence intensity drastically increases as a result of aggregate formation.36 These nanosensors are easy to fabricate and require less synthesis labor as compared to traditional water-soluble sensors. Normally, these nanosensors are fabricated by employing a single-step re-precipitation technique, but other important approaches such as emulsion polymerization, sol–gel phase transition, laser ablation, stratification deposition, and solvent evaporation have also been employed.37–41 By realizing the importance of FONs as sensors for metal ions in aqueous media, we aim to provide a brief understanding of this field. We have tried to cover the major reports of these types of sensing platforms and hope that this will facilitate the progress in this field.
2. Mechanism of sensing metal ions using FONs
Metal ions when detected by FONs via the technique of fluorescence necessitate certain obvious changes in the fluorescence emission by the fluorophore. In order to achieve this, there are three important ways: (i) fluorescence enhancement, (ii) fluorescence quenching, and (iii) shift in emissions. Fluorescence enhancement involves the employment of a fluorophore that enhances its fluorescence or becomes fluorescent in the presence of metal ions and has little or no fluorescence in the absence of metal ions. The increase in the fluorescence of such a receptor fluorophore is mainly due to the induced conformational restriction that results from the binding of metal ions. Fluorescence quenching involves the selection of molecules that exhibit high fluorescence in the absence of metal ions but their fluorescence suddenly vanishes or considerably reduces in the presence of the target metal ions. Finally, emission changes mainly depend upon the shift in the emission band of the fluorophore upon interaction with specific metal ions; the resultant shift in the band may be at a higher or lower wavelength. In this case, the change in the emission maximum is mainly due the reaction of the metal ions with the fluorophore and results in new molecules with new emission maxima.1,9 These changes may be attributed to photoinduced electron transfer (PET), intramolecular charge transfer (ICT), and photoinduced charge transfer (PCT). The enhancement in fluorescence is termed as chelation-enhanced fluorescence (CHEF); contrarily, the decrease in fluorescence is termed as chelation-enhanced fluorescence quenching (CHEQ). As the metal ions from the main groups possess high charge density and high electropositivity, when these ions are complexed with the receptor, it causes an enhancement in the fluorescence of the receptor42via the inhibition of PET; however, most of the transition metals are known to be fluorescence quenchers because they have the ability to quench fluorescence by the energy transfer mechanism. There are other more frequent mechanisms observed in metal detection via fluorescence changes. The other common mechanisms observed during sensing metal ions are Förster resonance energy transfer (FRET), fluorescence resonance energy transfer (FRET), resonance energy transfer (RET), and electronic energy transfer (EET). He et al. described additional details about the mechanisms involved in sensing metal ions.43 Such mechanisms for sensing depend upon the interaction of metal ions with the binding sites of sensors; therefore, the mechanism of sensing metal ions with FONs is the same as that when using traditional sensors; however, in some cases, metal ions adsorb on the surface of the nanoparticles, which results in the alteration of the photophysical properties of the molecule and a change in the fluorescence signatures is observed. Further, the self-assembly of these nanoaggregates allows the formation of binding sites for metals on the surface of the nanoparticles, which facilitates the increased efficiency and sensitivity of these nanosensors towards metal ions as compared to those of traditional water-soluble sensors.
3. FON-based chemosensors for transition metal ions
3.1 FON-based chemosensors for silver ions
Silver is considered to be a silent ion that does not have any spectroscopic signals due to which it is difficult to discriminate it from other correlated metal ions.44 Recently, only fluorescence detection techniques have been preferred for the detection of such silent hazardous metal ions.45 Yan et al. synthesized molecular probe 1 based on triazolo-thiadiazole; the synthesized probe was fabricated into nanoparticles to maximize its use in aqueous environments (Fig. 1).46 After the addition of excess Ag+ (6.7 equiv.) in the aqueous solution of 1, a 1.95-fold emission enhancement was observed, whereas no fluorescence increase was observed for other interfering metal ions (Fig. 2). The obvious change in the fluorescence of 1 upon interaction with silver ions was due to the formation of a complex between the silver ions and 1, which resulted in the inhibition of the PET phenomenon. The proposed nanoreceptor showed high sensitivity toward silver ions with the limit of detection (LOD) of 2.58 × 10−7 M. Similarly, for the detection of silver ions in aqueous media, a ratiometric dipodal nanosensor based on imine-linked 1,8-naphthalimide 2 was fabricated by Sharma et al.47 The addition of Ag ions caused selective ratiometric changes (quenching at 522 nm and enhancement at 627 nm) in the emission profile of nanoreceptor 2 in the presence of other interfering metal ions (Fig. 4). The ratiometric changes in this nanoreceptor were caused by the interaction of Ag ions with the naphthalimide rings of the nanoreceptor, which causes π–π stacking and results in the formation of “excimers.” Furthermore, the receptor displayed a linear response toward silver ions over a large range of pH (2.3–12.3), with a lower LOD value (15.5 nm) and shorter response time (84 s).
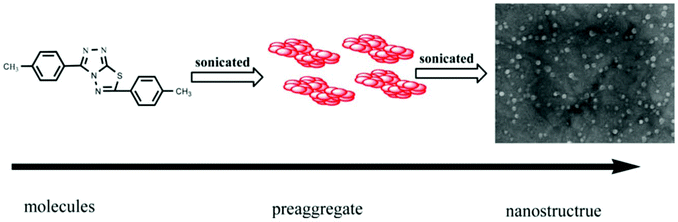 |
| Fig. 1 Schematic representation of the formation process of nanoscale materials of 1. Adapted with permission from ref. 46. Copyright 2011 Elsevier Ltd. | |
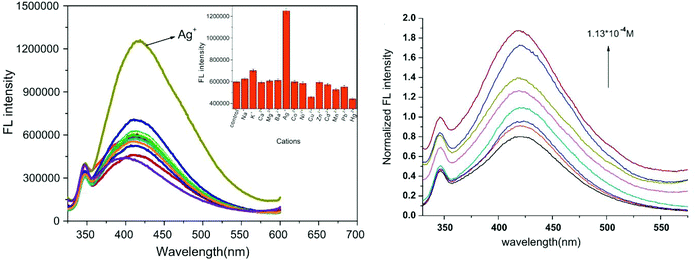 |
| Fig. 2 Fluorescence emission spectra of FONs (7.5 × 10−6 M) with or without various cations and plot of the fluorescent intensity as a function of Ag+ concentration. Adapted with permission from ref. 46. Copyright 2011 Elsevier Ltd. | |
According to the hard and soft acids and bases (HSAB) principle, Ag ions prefer to bind with soft nucleophiles;48,49 based on this strategy, several fluorescent probes have been designed for the detection of Ag ions, most of which are based on thiourea derivatives.28 This is because thiocarbonyl present in the thiourea moiety acts as a soft site for the binding of Ag ions. Most of the times, Hg2+ —a soft electrophile— causes interference in the determination of Ag+. For example, thiourea containing naphthalene–thiadiazole 3-based FONs displayed selective fluorescence quenching upon interaction with silver ions with marginal interference from Hg2+ (Fig. 4). Quenching in the fluorescence of the nanoreceptor can be attributed to the formation of small Ag nanoparticles on the surface of the nanoreceptor. The proposed nanosensor exhibited a linear response over a wide concentration range of silver ions with LOD of 4.7 × 10−8 M (5 ppb) (Fig. 3).50
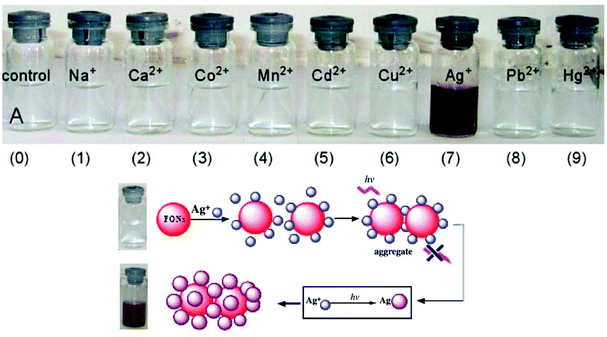 |
| Fig. 3 Photographs of FON solutions after adding 5 × 10−4 M of the indicated metal ions after 24 h and the schematic illustration of the possible structure of Ag-nanoparticle-coated 3 aggregates. Adapted with permission from ref. 50. Copyright 2008 Elsevier Ltd. | |
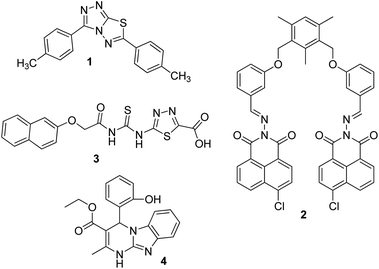 |
| Fig. 4 Structures of FONs for the detection of silver ions. | |
Recently, Navneet Kaur and co-workers fabricated a highly efficient, noninvasive, and economical fluorescent chemosensor mediated on organic nanoparticles (ONPs) of Biginelli compound 4 for the selective detection of Ag+ spermidine cations in biological samples (urine) and in environmental samples (river water) by quenching of fluorescence emissions (Fig. 4).51 The LOD was observed to be 7.9 nM for Ag+. The nanosensor involving Biginelli compound 4 could be applied in a broad spectrum of pH (3–12); hence, it was suitable for ion investigations in physiological and environmental conditions.
3.2 FON-based chemosensors for copper ions
Copper is an important micronutrient that is imbibed by the human body along with food and water: a daily intake of 2 mg of copper has been recommended by the U.S. Food and Drug Administration (FDA). Besides the advantages of copper, its extensive use can result in gastrointestinal problems and kidney failure.52 Because of the paramagnetic nature of Cu2+, most of the times, it causes quenching of fluorescence emission of chemosensors and leads to a turn-off signal.53 Compound 5 based on rigid conjugated pyridinylthiazole derivatives was synthesized and fabricated into nanoparticles for the selective detection of Cu2+ ions in aqueous media by Hou et al.54 The fluorescent intensity of the nanoreceptor showed an inverse relationship with the addition of Cu2+ ions; however, it remained unchanged with other competing metal ions (Fig. 5). Compound 5 nanoparticles, as compared to their monomers, displayed additional fluorescence sensitivity toward Cu2+ ions. The dynamic working of Cu2+ ions is exhibited by the proposed nanosensor ranges from 0.02 to 0.50 μM having LOD of 10 nM; however, the direct measurement of Cu2+ ions in the sample of drinking water has been expressed by planned chemosensors with satisfactory results.
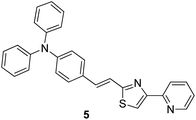 |
| Fig. 5 Structure of nanoreceptor (E)-N,N-diphenyl-4-(2-(4-(pyridin-2-yl)thiazol-2-yl)vinyl)aniline 5. | |
Recently, certain polymer-based fluorescent nanoprobes have been gaining considerable attention because of their possible use as detectors for metal ions.55 Based on this, Chen et al.56 proposed a multifunctional fluorescent nanosensor based on FRET. Fluorescent polymer nanoparticles were synthesized via the copolymerization of estrone, vinylbenzyl chloride, and fluorescent vinylic cross-linking monomer (fluorescein-o,o-bis-propene (FBP)) in oil-in-water mini-emulsion, stabilized with cationic surfactant (dodecyltrimethylammonium bromide (DTAB)). Finally, 1,4,7,10-tetraazacyclododecane (cyclen) was grafted onto the surface of the nanoparticles, which acts as a ligand for potential binding with metal ions. The fabricated polymeric nanoparticles exhibited very selective “on–off” response toward Cu2+ among 11 other common interfering metal ions in a pure aqueous medium. The selectivity of the proposed polymeric nanoreceptor toward Cu2+ was due to FRET between the FBP and Cu2+-cyclen complex. The polymeric nanosensor exhibited a very sensitive response toward Cu2+ ions and responded to very low concentrations up to 340 nM. A similar approach was reported by Su et al. who investigated a “turn-off” nanoprobe based on polydihydroxyphenylalanine nanoparticles (PDNPs) for the fluorescent detection of Cu2+ in aqueous media. Under optimized conditions, the nanoprobe showed a selective response toward Cu2+via a decrease in the fluorescence intensity at 520 nm; no other common interfering cations caused any changes in the emission profile of the nanoreceptor. The nanoreceptor exhibited high sensitivity toward Cu2+, with LOD of 0.10 μM, which is well below the safety limit of Cu2+ in drinking water permitted by the U.S. Environmental Protection Agency (EPA). Furthermore, the scope of detection of the nanosensor toward Cu2+ in river water samples was extended with satisfactory results.57
3.3 FON-based chemosensors for chromium ions
Usually, chromium exists in the form of two oxidation states, i.e., trivalent and hexavalent states. Here, Cr3+ is a vital trace element, and it plays an important role in the action of insulin and glucose, protein, and lipid metabolism. However, Cr6+ is dangerous to health and can cause damage to the human gastrointestinal, hematological, respiratory, immunological, developmental, and reproductive systems.58 Recently, the detection of this hazardous metal ion via fluorescence changes has become pivotal because this ion may induce changes in the photophysical properties of the receptors when they are complexed with it. These two suspected ions can be selectively detected in the presence of each other. A fluorescent sensor based on 3-aminopropylmethyl (tetra phenyl) silole 6 nanoparticles was synthesized by Trogler and co-workers (Fig. 7)59 for the selective detection of Cr6+ in aqueous media. When excited at 360 nm, the nanosensor exhibited an emission at 485 nm. The fluorescence of the sensor selectively declines with Cr6+ with a marginal effect of As5+. The mechanism of quenching was supposed to be a result of electron transfer from the excited state of the silole to the analyte. Not surprisingly, since AsO43− is a weaker oxidant than CrO42−, it is a weaker quencher, too (standard reduction potentials vs. NHE are −0.68 and −0.13 V, respectively; at pH 7, they are +0.15 and +0.56 V, respectively).
Dalavi et al.60 fabricated a very efficient fluorescent nanosensor based on cetyltrimethylammonium bromide (CTAB)-stabilized perylene nanoparticles (PNPs) for the selective detection of Cr6+. Under the most opportune conditions, the fluorescence intensity of PNPs examined at an excitation wavelength of λmax = 382 nm was quenched by the consecutive addition of growing concentrations of dichromate ions (Fig. 6). Fluorescence quenching was a result of the alteration of the surface of monodisperse nanoparticles that was restricted with dichromate ions by electrostatic interactions to form stable nonfluorescent micelle complexes in the ground state. The sensor could detect target cations with concentrations as high as 0.008 mg mL−1. Furthermore, this method of sensing was highly appreciable with promising results for the detection of contents of Cr6+ in synthetic and environmental samples.
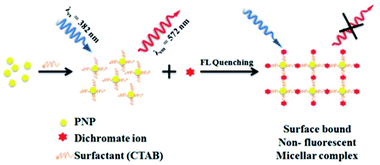 |
| Fig. 6 Proposed mechanism for the formation of CTAB PNPs and surface-bound stable nonfluorescent micellar complexes with dichromate ions. Adapted with permission from ref. 60. Copyright 2014 the Royal Society of Chemistry. | |
Similarly, Wang et al. carried out the fabrication of ONPs based on anthracene/polyacrylamide (AN/PAM) for the selective determination of Cr6+ in aqueous media. Under optimum conditions, the excitation spectra of AN/PAM nanoparticles have their maximal peak at 345 nm, which shifts to 405 nm with a concomitant increase in intensity after the addition of Cr6+ and linearly reduces with the addition of higher concentrations of Cr6+. Under optimum conditions, numerous common cations such as Na+, K+, Mg2+, Al3+, Fe3+, Ca2+, Zn2+, Cr3+, Hg2+, Cu2+, Co2+, Ni2+, Ag+, Mn2+, and Sn2+ were tested for their possible interference, but none of the metal ions posed any interference in the determination of Cr6+. In addition, from these fluorescence studies, it was evident that the planned nanoprobe could detect Cr6+ with LOD as low as 0.02 μg ml−1. The subsequent addition of Cr6+ causes the gradual oxidation of PAM and reduction of Cr6+ into Cr(OH)3. The oxidative product of PAM reduces the fluorescence intensity; further, Cr(OH)3, which enveloped the nanoparticles, also decreased a portion of the fluorescence, which eventually resulted in the reduction in fluorescence with an increase in Cr6+ concentration.61 A nanoprobe based on 1,8-naphthalic anhydride and triethylenetetramine ligand 7 was synthesized and fabricated into its nanoparticles by Saini and co-workers for the selective detection of Cr3+ in aqueous media (Fig. 7). The proposed nanosensor exhibited selective enhancement in the emission intensity in the presence of targeted metal ions. The enhancement in the emission was due to the complexation of Cr3+ with the nitrogen part of triethylenetetramine, which resulted in the inhibition of PET. Furthermore, the nanoprobe showed high selectivity toward Cr3+ with LOD of 64 nM.62
 |
| Fig. 7 Structure of chemosensor for the fluorescent detection of chromium ions. | |
Pyrene and its derivatives are well known for their brilliant optical properties,63 and they have been used in several sensing platforms as a fluorophore core.42,64 On the basis of the importance of pyrene as a fluorophore, Wang et al.65 converted 1-pyrenemethylamine 8 into its nanoparticles for the selective detection of Cr6+ in water. The sensor displayed the maximum excitation and emission at 238, 283, 351, 383, and 400 nm, but only λex = 238 and λem = 400 nm were selected for the recognition studies (Fig. 7). The fluorescence of the nanosensor was quenched with the successive addition of Cr6+, but remained unchanged with other common interfering metal ions. From these fluorescence studies, it was evident that the sensor could detect Cr6+ ions up to concentrations of 2.8 × 10−6 mol L−1. In 2018, Viviana et al. developed an efficient technique for the recognition of Cr3+ in water and multivitamin formulations by employing fluorescent nanosensors mediated on N,N′-bis(5-bromo-2-thiophenylmethyl)ethylenediimine (BTED 9).66 BTED-based nanosensors selectively and sensitively identify Cr3+ cations without detecting other coexisting metal cations in a H2O bath and aqueous vitamin tablets. The LOD was observed to be 7.3 μM.
Recently, imine-linked fluorophores have gained considerable attention for metal ion recognition due to the ease of operation afforded by excited state intramolecular proton transfer (ESIPT), high fluorescence yield, and dual channel emission with keto–enol tautomerism (Fig. 8).67,68 Based on this strategy, Kaur et al.32 designed the synthesis of glutathione-based tripeptide and imine-linked fluorescent sensor 10via the condensation reaction between salicylaldehyde and tripeptide glutathione (GSH). The synthesized fluorescent sensor was fabricated into nanoparticles for the selective detection of Cr3+ in an aqueous bath. When excited at 324 nm, because of keto–enol tautomerism, the sensors exhibited two emission peaks at 369 and 400 nm (Fig. 9). The emission peak at 369 nm revealed enhancement in the fluorescence intensity upon the addition of Cr3+; however, the emission peak at 490 nm gets quenched. Other metal ions have a negligible influence on the sensing system under the same conditions. The fluorescence enhancement at one wavelength (369 nm) and quenching at the other (400 nm) can be explained on the basis of local field enhancement and radiationless energy transfer process between the metal and the host. When the metal is attached to the host, the local field causes the stimulation of molecules, which consequently leads to enhancement in fluorescence intensity. Further, when the energy loss occurs toward the metal, fluorescence quenching takes place. The nanosensor displayed a linear response for Cr3+ over a wide concentration range (0.55–50 μM) with LOD of 0.25 μM.
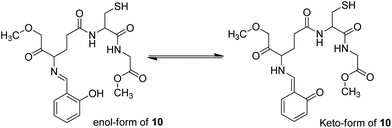 |
| Fig. 8 Keto–enol tautomers of probe 10. | |
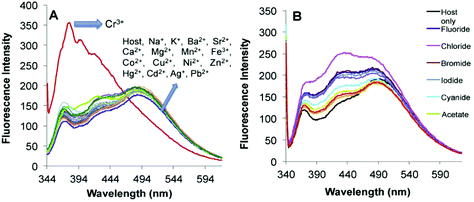 |
| Fig. 9 Changes in the fluorescence spectrum of 10 (FONs) (20 M) in aqueous medium upon the (A) addition of 50 M of a particular metal nitrate salt in an aqueous medium and (B) addition of 50 M of a particular anion as tetrabutylammonium salt in an aqueous medium. Adapted with permission from ref. 32. Copyright 2015 Elsevier Ltd. | |
3.4 FON-based chemosensors for cadmium ions
Cadmium concentration in drinking water is 0.005 mg L−1 or 4.4 × 10−8 M, which is declared by the EPA. Malfunctions may be caused by the long-term exposure to cadmium for the abovementioned levels. Mahajan et al.69 demonstrated that N-methyl isatin nanoparticle (N-MINPs) 11 was used as selective fluorescent sensors for Cd2+ ions in aqueous media (Fig. 10). Under optimized conditions, the emission of the nanosensor increased with the addition of Cd+ ions; however, a contradictory response was observed for other metal ions, namely, Ni2+, Cu2+, Hg2+, Sn2+, Pb2+, Ca2+, Zn2+, Na+, K+, Co2+, and Mg2+. Cadmium is a soft metal and forms a complex with N-MINP ligands by electrostatic forces originating from oxygen that functions as the donor group of carbonyl functionality (Fig. 11). The formation of a rigid complex leads toward the restriction in free rotation of the carbon in the carbonyl group with respect to the indole ring, ultimately causing an increase in the fluorescence intensity of the receptor. The fabricated nanosensor was found to be very sensitive toward Cd2+ as it could detect ion concentrations as high as 1.33 μg mL−1 (Fig. 12).
 |
| Fig. 10 Structure of fluorescent chemosensor 1-methylindoline-2,3-dione 11. | |
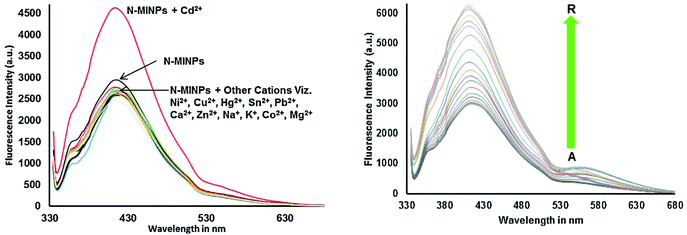 |
| Fig. 11 Selectivity of 11 toward Cd2+ shows fluorescence enhancement as compared to other cations, and the fluorescence spectra of N-MINP suspension (1 × 10−5 M) in the presence of different concentrations of Cd2+ ions. Adapted with permission from ref. 69. Copyright 2015 Elsevier Ltd. | |
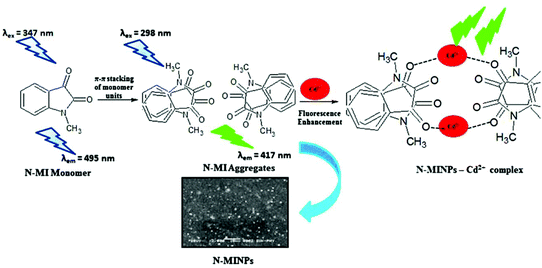 |
| Fig. 12 Proposed graphic for the formation of 11 and its complexation with Cd2+ ions. Adapted with permission from ref. 67. Copyright 2015 Elsevier Ltd. | |
3.5 FON-based chemosensors for mercury ions
Bhardwaj et al. proposed the synthesis and fabrication of dipodal-rhodamine-based fluorescent nanoprobe 12 for the selective detection of Hg2+ ions in aqueous media (Fig. 6). When excited at 530 nm, the nanoprobe did not exhibit any fluorescence with most metal ions; however, a new band at 547 nm appeared when Hg2+ (10 μM) was added, with almost 100 times increase in the fluorescence intensity (Fig. 13).70 The sensor displayed a linear response toward Hg2+ over a wide concentration range (0 μM to 730 μM) with LOD of 0.1 nm. The enhancement in the fluorescence of the receptor can be attributed to the spirolactam ring opening assisted by the coordination of Hg2+ (Fig. 14).
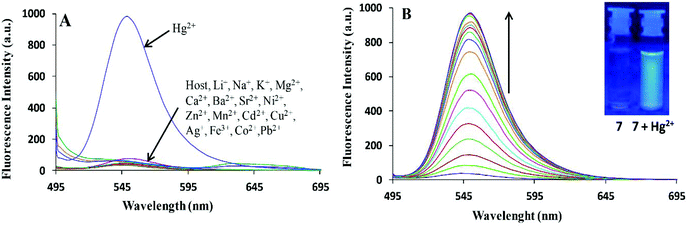 |
| Fig. 13 (A) Changes in the fluorescence emission spectra of 195 nM solution of 12 (FON) upon the addition of 10 μM of different metal ion salts in 15 aqueous media; (B) changes in the fluorescence emission spectra of 195 nM solution of 12 (FON) upon the successive addition of 0 to 10 μM of Hg2+ in an aqueous medium. Adapted with permission from ref. 70. Copyright 2013 the Centre National de la Recherche Scientifique (CNRS) and the Royal Society of Chemistry. | |
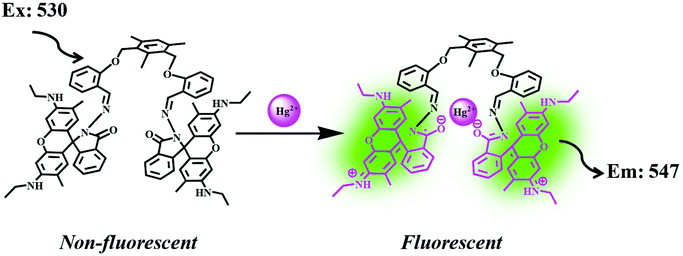 |
| Fig. 14 Process of sensing through the coordination of Hg2+. Adapted with permission from ref. 70. Copyright 2013 CNRS and the Royal Society of Chemistry. | |
Thiourea-based tripodal nanoreceptor 13 was fabricated by Singh and co-workers for the detection of Hg2+ in aqueous media (Fig. 16).71 The addition of Hg2+ ions cause selective enhancement in the fluorescence of nanoreceptors in the presence of other common interfering metal ions. This can be attributed to the binding ability of Hg2+ with a nanoreceptor that rearranges the framework into static conformation through multivalent interactions, ultimately yielding PET. The nanoreceptor exhibited a linear response toward Hg2+ over a wide concentration range of 0–10 μM with LOD of 2.4 nM (Fig. 15). Similarly, Li and Yan72 proposed a ratiometric fluorescent probe based on thiourea–thiadiazole–pyridine-linked 14 FONs for the selective recognition of Hg2+ ions (Fig. 16). Upon binding with Hg2+, the nanoreceptor exhibited a 46-fold increase in the fluorescence quantum yield along with a 98 nm red-shift in the fluorescence emission. The observed variation in fluorescence can be attributed to the potential of Hg2+ to convert theorem into urea.73 Under optimum conditions, the sensor showed LOD of 1.13 × 10−7 M (22.6 ppb); however, other metal ions, namely, Ni2+, Cd2+, Ca2+, Na+, Cu2+, Ag+, Pb2+, Mg2+, and Mn2+, did not induce any significant variation in the emission profile of the sensor.
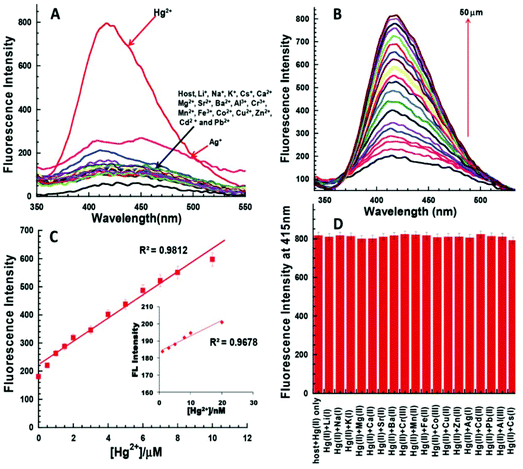 |
| Fig. 15 (A) Changes in the emission profile of nanoaggregates 13 (10 μM) in water upon the addition of 50 μM of a particular metal nitrate salt; (B) changes in the emission profile of nanoaggregates 13 (10 μM) upon the successive addition of Hg2+ (0–50 μM); (C) linear regression graph between the concentration of added mercury ions (0–10 μM) and increase in the fluorescence intensity of nanoaggregates 13 (25 μM). Inset: Linear regression plot of nanoaggregates 13 (10 μM) and addition of Hg2+ in the range of 0–20 nM; (D) influence of other metal ions on Hg2+-based changes in the emission profile of nanoaggregates 13. Adapted with permission from ref. 71. Copyright 2014 the Royal Society of Chemistry. | |
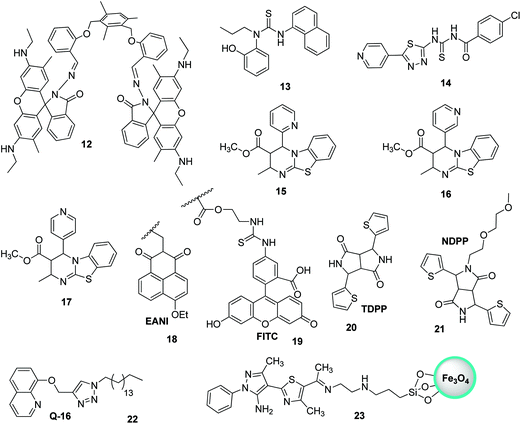 |
| Fig. 16 Structures of FON-based chemosensors for the detection of mercury ions. | |
Biginelli-based compounds 15, 16, and 17 were synthesized by Singh et al.74 and fabricated into nanoparticles to test as a selective sensor for metal ions in aqueous media (Fig. 16). Upon the addition of suspected cation solution (100 μM) to the aqueous solution of nanoreceptors (20 μM), only nanosensor 16 exhibited a selective response to Hg2+ ions. The addition of Hg2+ resulted in the quenching of the emission band at λmax = 456 nm and an enhancement in λmax to 440 nm. The binding of Hg2+ with the nanoreceptor caused a disturbance in the electron cloud of the fluorophore, thereby resulting in PET; this ultimately resulted in a decrease in the emission with a blue-shift. The nanosensor displayed a very sensitive response toward Hg2+ ions over a wide concentration range (0–100 μM) with LOD of 1 nM.
The nanoparticles of a fluorescent polymer based on 2-vinylnaphthalene and acrylic acid were fabricated by Wang et al.75via ultrasonic radiation. These polymer-based nanoparticles were used for the detection of Hg2+ in aqueous media. The nanoprobes presented excellent sensing potential toward Hg2+ ions, since the probes could detect suspected ions as high as 0.01 μg ml−1. The utility of the nanosensor was also evaluated with artificial samples, and the sensor yielded satisfactory results. Similarly, Wang et al. developed polymer-mediated fluorescent ratiometric nanoprobe NP3 for the selective detection of Hg2+ ions in water. The NP3 nanoprobe contains a reference fluorescent dye (EANI viz. 4-ethoxy-9-allyl-1,8-naphthalimide 18) that resides in the nucleus of the nanosensor, and the Hg2+ identification system (FITC, i.e., fluorescein derivative 19) is present on the exterior of the nanosensor (Fig. 16).76 The nanoprobe exhibits excellent long-term photostability, promising H2O dispersibility, and highly sensitive and selective ratiometric recognition of Hg2+ ions in H2O relative to other interfering cations, namely, Fe2+, Ag+, Cd2+, Fe3+, Ca2+, K+, Mn2+, Co2+, Zn2+, Mg2+, Na+, and Cu2+, as well as reasonably low LOD (∼75 nM). In 2017, Nie et al. investigated the fluorescent recognition of Hg2+ ions in water by using two organic DPP-mediated sensors, namely, TDPP- and NDPP-mediated loose micelle-type nanosensors.77 Commercially available TDPP 20 having NH entity can detect Hg2+ ions in water via the molecular aggregation and formation of the TDPP–Hg–TDPP complex (Fig. 16). NDPP 21, on the other hand, operated more efficiently on account of the development of surfactant-free micelle-type ONPs in H2O. NDPP nanosensors are simple to prepare as compared to other micelle-mediated ONPs. Further, two organic DPP-mediated sensors exhibited excellent selectivity toward Hg2+ ions relative to other ions and appropriate solubility in H2O. In addition, the two sensors exhibited LOD of around 11 nM.
Liu et al. recently described ONP mediated on 22 for the fluorescent recognition of Hg2+ ions in H2O.78 Upon the addition of Hg2+, the nanoparticles of 22 exhibited fluorescence quenching without interference from other metal cations in H2O (Fig. 16). The LOD was observed to be 4.42 nM for Hg2+. Very recently, pyrazole-based silica-coated magnetite fluorescent nanoprobe 23 was fabricated and investigated for ion detection by Noshin Mir and co-workers (Fig. 16).79 The investigation of ion detection by synthesized nanoprobe 23 revealed that Hg2+ ions in H2O exhibited one of the highest capacity for fluorescence quenching. Nanoprobe 23 could sense Hg2+ with LOD of 7.5 nM and it could be simply isolated from H2O by means of a bar magnet.
3.6 FON-based chemosensors for iron ions
Iron is the most vital metal ion in the biological system and it plays a crucial role in a variety of chemical processes, i.e., cofactor in enzymatic reactions, cellular metabolism, and transport of oxygen through haem. Its deficiency and excess cause serious disorders in living systems, such as damage to vital organs, anemia, heart failure, and diabetes.80 Its detection by using the fluorescence technique mainly involves the quenching of emission intensity of the sensor via the electron transfer mechanism. However, in some reported cases, fluorescence enhancement is also observed in the fluorophore, which may be caused by the chelation of Fe3+/Fe2+ with the fluorophore sensor.81,82 For the selective sensing of Fe3+ in an aqueous medium, a branched nanoreceptor with a triazole as a core and benzothiadiazide derivative as branches (24) was fabricated by Wang and co-workers (Fig. 19).83 The proposed nanosensor exhibited high selectivity and sensitivity toward the targeted ions via transformation in the emission profile. The emission of the nanoreceptor quenched by the addition of Fe3+ ions was unaffected by the other metal ions. The Fe–organic complex is responsible for such sensing behavior. These selective and promising properties of the nanoreceptor are the key reasons for its application as Fe3+ impurity detector in a promising cathode material (LiFePO4) in lithium-ion batteries. The fluorescence intensity of 24 NPs does not show quenching in pure LiFePO4 dissolved in an acid solution, but it gets dramatically quenched when Fe3+ impurity is present. The nanosensor can detect Fe3+ ions up to 1 × 10−7 M concentration. Similarly, for the detection of Fe3+, naphthalimide FON 25 with aggregation-induced emission enhancement properties were designed and synthesized by Cuiping Han et al. (Fig. 19).84 When excited at 513 nm in a semi-aqueous medium (H2O: 70%; CH3CN: 30%), the receptor displayed an emission peak at 523 nm. Fe3+ caused an increase in the intensity along with an 8 nm blue-shift in the emission maxima of the nanoreceptor (Fig. 17). The enhancement in the fluorescent intensity along with this blue-shift could be attributed to the inhibition of the PET effect. In the absence of Fe3+, PET takes place from an electron-rich diethylenetriamine unit to an electron-deficient naphthalimide group of receptors; the binding of Fe3+ with the lone pair of N from the diethylenetriamine portion resulted in the inhibition of PET and increase in fluorescence. The nanoreceptor exhibited a linear response toward Fe3+ ions over a wide concentration range (1 nM to 100 μM) with LOD of 0.35 nM.
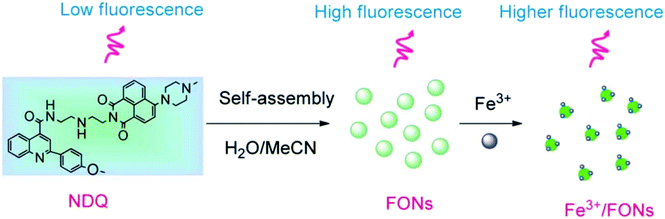 |
| Fig. 17 Schematic illustration of the synthesis of 25 and strategy for Fe3+ detection. Adapted with permission from ref. 84. Copyright 2014 the Royal Society of Chemistry. | |
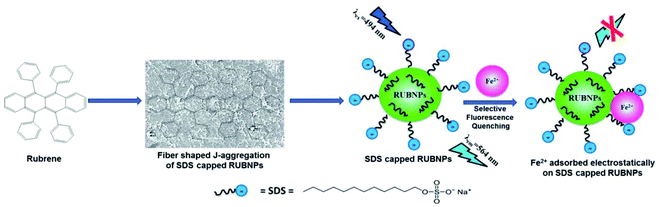 |
| Fig. 18 Plausible fluorescence quenching mechanism based on Fe2+ ion adsorption on the surface of SDS-capped RUBNPs. Adapted with permission from ref. 86. Copyright 2015 the Royal Society of Chemistry. | |
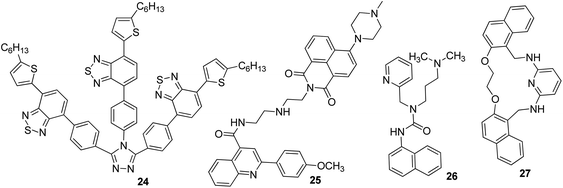 |
| Fig. 19 Structures of FON-based chemosensors for the detection of iron ions. | |
Tripodal receptor 26 based on organic fluorescent nanoparticles was synthesized by Shweta Chopra et al. (Fig. 19).85 They reported that the fabricated nanoparticles could be used as a Fe3+-selective sensor in aqueous media. Selective fluorescence quenching was observed upon the addition of Fe3+ ions with no interference from other metal ions. The lowest detectable concentration of the receptor for Fe3+ ions was found to be 1.66 μM. The selective quenching of fluorescence was a result of the inhibition of PET via 1
:
1 26-Fe3+ complex formation. Further, it was observed that spermidine (a biologically active amine) could selectively replace Fe3+ ions from 26-Fe3+ and ultimately cause an increase in the fluorescence intensity. On the basis of these observations, a selective detection of spermidine was carried out with LOD of 3.68 μM.
A fluoroionophore sensor based on the sodium dodecyl sulphate (SDS)-capped rubrene nanoparticles (RUBNPs) was reported as a Fe2+-selective chemosensor by Mahajan and co-workers (Fig. 18).86 The presence of Fe2+ in an aqueous suspension of RUBNPs induced fluorescence quenching at 564 nm, and the quenching results fit into a conventional Stern–Volmer relation in the concentration range of 0–80 μg mL−1. The sensor exhibited a good linear relationship toward Fe2+ with LOD of 0.015 μg mL−1. Further, the utility of the projected nanosensor was verified through the Fe2+ content in a pharmaceutical tablet.
Very recently, Azadbakht and co-workers described a highly stable fluorescent organic sensor mediated on nanoparticles of 27.87 This 27-based chemosensor with a particle size of around 35 nm possesses high selectivity and sensitivity toward Fe3+ cations in aqueous buffer solutions as compared to other metal cations such as Ag+, Hg2+, Cd2+, Zn2+, Cu2+, Ni2+, Co2+, Fe2+, Mn2+, Pb2+, Al3+, Ba2+, Ca2+, Mg2+, Cs+, K+, and Na+ by fluorescence emission quenching at a wavelength of 358 nm. The LOD of this 27-based sensor was found to be 5.57 nM for Fe3+.
3.7 FON-based chemosensors for zinc ions
Zinc plays an essential role in pathophysiological processes because of the advantages offered by its structural properties, such as gene expression, cell apoptosis, enzymatic adjustment, and neurotransmission. Some neurological problems like Alzheimer's disease and Parkinson's disease and the pathology of intellectual development is caused by the break-up of zinc homeostasis.88–90 For the selective detection of zinc ions in an aqueous medium, ONPs with ligand N,N′-bis(pyridyl-2yl-methyl)ethylenediamine 28 were fabricated by Huerta-Aguilar et al. (Fig. 20).91,92 The fluorescence intensity of the nanoreceptor increases with the subsequent addition of Zn ions, whereas no enhancement was observed with other common interfering metal ions. The selective response of Zn ions toward nanoprobe 28 was attributed to the inhibition of PET, which was the result Zn-28 complex formation. The sensor displayed a linear response toward Zn2+ (0.0–0.1 mM) with LOD of 470.6 ppb. Further, the scope of the nanosensor was utilized in the determination of Zn2+ ions in commercially available multivitamin tablets (Fig. 21). Based on a similar approach, the same group designed the synthesis and fabrication of nanoreceptor 29 for the recognition of Zn2+ and Al3+ ions in an aqueous medium (Fig. 20). The fluorescence intensity of 29 rose with an increase in the concentration of Zn2+. Interestingly, when Al3+ ions were added to 29-ONPs/Zn2+ in a sequential order (addition of Zn2+ to 29-ONPs, followed by Al3+), a further enhancement in the fluorescence with a 50 nm red-shift was observed. The addition of Al3+ further activated Zn2+/29-ONPs system and induced an increase in fluorescence. The HOMO energy of 29-Zn2+ system is lowered as compared to that of nanoreceptor 29, which results in the inhibition of PET from the HOMO (donor) to the fluorophore, resulting in an increase in fluorescence. When Al3+ is added to the 29-Zn2+ system, the HOMO energy of the receptor gets further stabilized; further, the addition of Al3+ causes an increase in the planarity of the complex, leading to strong PET from the receptor, resulting in a further increase in fluorescence.91,92
 |
| Fig. 20 Structures of FON-based chemosensors for detection of zinc ions. | |
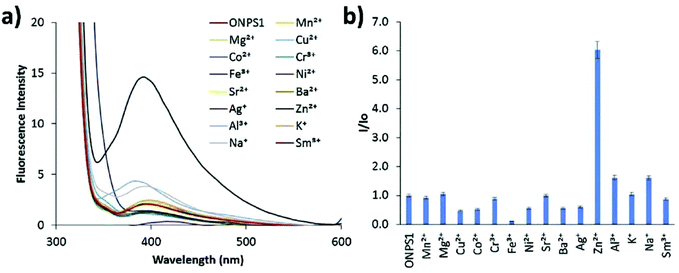 |
| Fig. 21 Fluorescence studies: metal ion binding test for nanoprobe 28 (0.1 mM) in an aqueous medium for the recognition of metal ions; (a) change in fluorescence spectra with respect to different metal ion additions; (b) relative fluorescence intensity at 410 nm against different metal ions. Adapted with permission from ref. 92. Copyright 2016 Elsevier Ltd. | |
4. FON-based chemosensors for the main group metal ions
4.1 FON-based chemosensors for cesium ions
In a struggle for the development of a Cs+ determination technique, 30 was synthesized by Chopra et al. by the simple condensation of dipicolinic acid hydrazide with catechol carbaldehyde. In addition, the receptor was fabricated into its nanoparticles to study the ability toward the recognition of Cs+ in aqueous media (Fig. 23).93 Once excited at 309 nm, it showed an emission peak at 412 nm. The emission spectra of the nanoreceptors remained unaffected with most of the other metal ions, i.e., Hg2+, Fe3+, Pb2+, Cd2+, Al3+, Co3+, Zn2+, Ag+, Li+, Sr2+, Ni2+, Cu2+, Mg2+, Ba2+, Mn2+, Na+, K+, Al3+, and Cr3+; however, the addition of Cs+ ions caused enhanced emission in the solution with receptor 30 (Fig. 22). This enhancement is expected because of the formation of 1-Cs+ complex, which inhibits the PET effect. The sensitivity experiment showed that the proposed nanosensors could detect Cs+ concentrations of up to 70 nm.
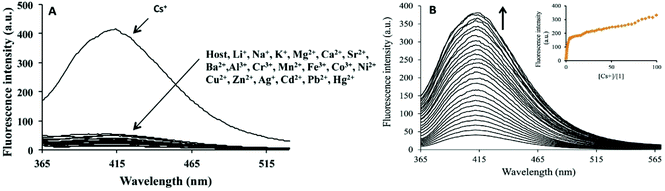 |
| Fig. 22 (A) Changes in fluorescence spectrum of FONs of 30 (1.5 mM) in aqueous media upon the addition of 100 mM of a particular metal nitrate salt in the aqueous medium. (B) Changes in the fluorescence spectrum of FONs of 30 upon the successive addition of Cs+; inset shows the fluorescence intensity as a function of Cs+. Adapted with permission from ref. 93. Copyright 2014 Elsevier Ltd. | |
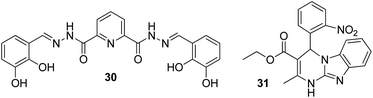 |
| Fig. 23 Structures of FON-based chemosensors for the detection of cesium and lithium ions. | |
4.2 FON-based chemosensors for lithium ions
Due to its smaller size, lithium exhibits high heat of hydration and poor coordination capability; these properties complicate the process of developing selective fluorescent probes for detection.94,95 However, it possesses high charge density (hard acid), and it can bind with hard donor atoms (oxygen and nitrogen) of the receptor, which may induce changes in the photophysiological properties of the receptor. Therefore, the production of an optical sensor grounded on Biginelli compound 31 fabricated into ONPs via the bottom-up technique was formulated by Kaur and co-workers (Fig. 23).96 There was not a single sort of intrusion from any of the conceivable interfering cations to the proposed nanosensor; however, a stable, rapid, and selective response was exhibited by the nanosensor toward lithium ions. When excited at 300 nm, the nanoreceptor exhibited an emission peak at 353 nm. The emission peak remained unchanged with most of the other metal ions such as Al3+, Na+, Ag+, K+, Cd2+, Cs2+, Pb2+, Mg2+, Hg2+, Ca2+, Zn2+, Sr2+, Cu2+, Ba2+, Co2+, Cr2+, Fe3+, and Mn2+, but a considerable enhancement in the fluorescence intensity was evident after the addition of Li+ ions: the LOD was up to 122 nM. The enhancement in fluorescence of the nanoreceptor could be attributed to the ability of Li+ to bind with highly electronegative oxygen and nitrogen atoms of the receptor. Moreover, in the practical samples, the working ability of the sensor was also analyzed through lake and tap waters, and it was observed that the sensor could effectively detect Li+ ions with an average recovery percentage of 96.5%.
4.3 FON-based chemosensors for strontium ions
Besides many beneficial uses of strontium,97,98 its one isotope, i.e., radioactive strontium-90, is considered to be one of the “most hazardous” when considering radioactive fallout.99 There are a few reports on fluorescent probes for the selective detection of strontium.100,101 Kaur et al.102 synthesized a series of three compounds (32, 33, and 34), and they fabricated them into ONPs for fluorescent receptor development for detecting cations in aqueous media (Fig. 24). When the synthesized compounds were tested for the detection of different metal ions, only probe 34 displayed an increase in emission when Sr2+ was added as a suspected cation. Therefore, for the development of a chemosensor, the deciding factor is the position of the substituent in the organic compound. The fluorescence intensity of the nanosensor increases because the guest molecule binds to the oxygen atom of the carbonyl group (C
O) of compound 34, which leads to the reduction in the PET process, subsequently leading to fluorescence enhancement. The nanosensor proved to be very sensitive toward Sr2+: it could detect Sr2+ concentrations as low as 184 μM. Moreover, commercial applications of the sensor were also investigated via the determination of Sr2+ amount present in toothpastes and oral gels.
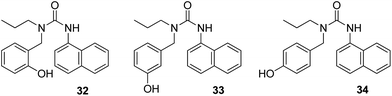 |
| Fig. 24 Structures of FON-based chemosensors for the detection of strontium ions. | |
4.4 FON-based chemosensors for aluminum ions
Poor coordination ability and lack of spectroscopic characteristics are the two major difficulties in the design of fluorescent probes for the detection of Al3+. The probes that contain strong coordinating groups have been designed for the recognition of Al3+. Based on this, nanoreceptor 36 was synthesized by Saini et al.103 by treating 1,8-naphthalimide with diethylenetriamine (Fig. 25). When excited at 340 nm, the receptor displayed two emission peaks at 340 and 494 nm, respectively. Among the various tested metal ions, Al3+ displayed selective enhancement in the emission peak present at 494 nm. The nanosensor exhibited high sensitivity toward Al3+ with LOD of 667 nM. Upon the addition of Al3+ into a solution containing receptor 36, Al3+ binds with the lone pair of central nitrogen of the diethylenetriamine part of receptor 36 and consumes the excess electron. This results in the reduction of PET and causes an increase in the fluorescence intensity. Based on this same mechanism, Azadbakht and co-workers104 designed a nanosensor based on macrocycle compound 37. This nanosensor exhibited an “on–off”-type mode with high selectivity toward Al3+ ions (Fig. 25). Upon binding of Al3+ with the nanoparticles, a 15-fold fluorescence enhancement at 359 nm was observed. The LOD of 37 for Al3+ was as low as 2.8 × 10−7 M. In 2017, Kim and co-workers synthesized water-soluble onion-type ONPs 35 and investigated them for their applicability toward ion detection (Fig. 26).105 Nanoparticles 35 were in the size range of 4–8 nm and allowed the precise recognition of Al3+ ions, even in the presence of higher concentrations of other metal cations: the LOD was 0.76 μM.
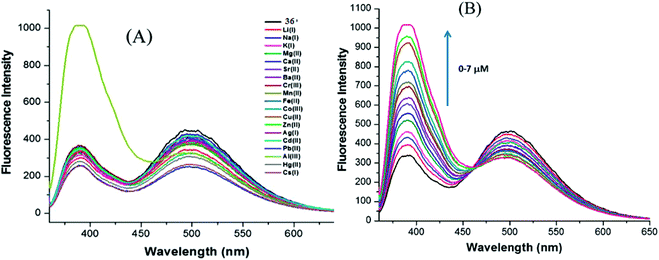 |
| Fig. 25 Changes in the emission profile of nanoaggregates (0.1 mM) in water upon the addition of 20 mM of particular metal nitrate salts. (B) Changes in the emission profile of nanoaggregates (0.1 mM) upon the successive addition of Al3+ (0–7 mM). Adapted with permission from ref. 103. Copyright 2014 CNRS and the Royal Society of Chemistry. | |
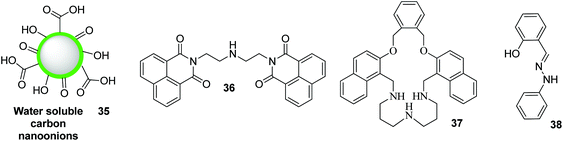 |
| Fig. 26 Structures of FON-based chemosensors for the detection of aluminum and tin ions. | |
4.5 FON-based chemosensors for tin ions
In 2017, Patil and co-workers reported a highly stable fluorescent organic chemosensor based on the nanoparticles of (E)-2-((2-phenylhydrazono)methyl)phenol 38 (Fig. 26).106 The synthesized PHP-NPs (38) were found to have an average size of 93 nm with spherical-shaped morphology. Nanosensors 38 exhibited high selectivity and sensitivity toward the detection of Sn2+ cations in H2O with an increase in fluorescence intensity relative to other cations such as Zn2+, Hg2+, Pb2+, Ca2+, NH4+, Ni2+, Fe2+, Fe3+, and Cu2+, which actually appear to quench the fluorescence of these nanosensors. The LOD was found to be 0.0027 μg mL−1. Further, nanosensors 38 could be utilized to formulate a fluorometric technique for the recognition of Sn2+ cations in environmental liquids taken from residential areas. The recognition of Sn2+ ions by PHP-NPs yields a purely economical and practical method.
5. Summary of FON properties and comparison with conventional fluorescent chemosensors
The performance summary of FONs is listed in Table 1. Veale and co-workers reviewed conventional fluorescent chemosensors for ions based on organic structures.107 A comparison between conventional organic chemosensors and nanoparticle-based organic chemosensors revealed that FONs are more valuable and efficient in terms of fluorescence quantum yield, detection limit, decomposition stability, solubility, and thermal stability. From Table 1, it is evident that FONs have lower LOD (0.35 nM), which facilitate high selectivity toward ions even at very low concentrations as compared to other conventional organic chemosensors. The appropriate enhancements in wavelength-shifted fluorescence intensity (238–564 nm) in the case of FONs provided increased amplification of the recognition event. Further, the feature of small-sized nanoparticles (5–222 nm) makes FONs cheaper, noncorrosive, nonflammable, and highly thermally stable as compared to conventional organic chemosensors. Moreover, in comparison with conventional organic chemosensors, FONs have the strongest affinity with the relevant target (binding selectivity); because of this good binding selectivity, the fluorescence signal can avoid environmental interference (signal selectivity), yielding advantages such as sensor ion concentration, photobleaching, environment around the sensor (temperature, polarity, pH, etc.), and stability under illumination. In addition, FONs are capable of detecting a large spectrum of ions. On the other hand, traditional chemosensors have a limited range, i.e., there are very rare reports about the use of conventional organic chemosensors in the detection of chromium, strontium, tin, and cesium ions. However, there is still a considerable scope to increase the efficiency and capability of FONs to satisfy various criteria such as fluorescence bioimaging capacity, high biocompatibility, and fast chelation.
Table 1 Summary of sensitivity of various FON-based sensors
Metal ion |
Functionalizing agent |
Size of nanoparticle (nm) |
Detection wavelength (nm) |
Mechanism |
L.O.D (nM) |
Ref. |
L.O.D = Limit of detection; nm = nanometer; nM = nanomolar. |
Ag+ |
Triazolo-thiadiazole |
20 |
300 |
PET |
258 |
45
|
Ag+ |
1,8-Naphthalimide |
25 |
522 and 627 |
Formation of excimers |
15.5 |
46
|
Ag+ |
Thiourea |
100 |
355 |
Ag-Nanoparticles on the surface of nanoreceptor |
47 |
48
|
Ag+ |
Biginelli |
22 |
350 |
|
7.9 |
51
|
Cu2+ |
Pyridinylthiazole |
200 |
525 |
Adsorption of Cu2+ ions to the surface of the nanoparticles |
10 |
53
|
Cu2+ |
Cyclen |
33–40 |
540 |
FRET |
340 |
55
|
Cu2+ |
Dihydroxyphenylalanine |
160 |
520 |
The good selectivity may be due to the binding of Cu2+ with N and O atoms on the surface of the PDNPs |
100 |
56
|
Cr6+ |
Silole |
100 |
360 and 485 |
PET |
2.187 |
58
|
Cr6+ |
Perylene |
34 |
382 |
Non-fluorescent micelle complex |
8 × 106 |
59
|
Cr6+ |
Poly-acrylamide |
40 |
345 and 405 |
Oxidation of poly-acrylamide |
2.0 × 104 |
60
|
Cr3+ |
1,8-Naphthalic anhydride |
18 |
500 |
PET |
64 |
61
|
Cr6+ |
1-Pyrenemethylamine |
25 |
238 and 400 |
PET |
2800 |
62
|
Cr3+ |
N,N′-Bis(5-bromo-2-thiophenylmethyl)ethylenediimine |
80 |
373 |
PET |
7.3 × 103 |
66
|
Cr3+ |
Glutathione |
69 |
369 and 400 |
PET |
250 |
32
|
Cd2+ |
Isatin |
112 |
417 and 567 |
Internal charge transfer (ICT) |
1.330 × 106 |
68
|
Hg2+ |
Rhodamine |
— |
530 |
Spiro lactam ring opening |
0.1 |
69
|
Hg2+ |
Thiourea |
66 |
415 |
PET |
2.4 |
70
|
Hg2+ |
Thiourea |
200 |
403 |
PET |
113 |
71
|
Hg2+ |
Biginelli |
— |
456 and 440 |
PET |
1 |
73
|
Hg2+ |
2-Vinylnaphthalene and acrylic acid |
20 |
365 |
Complexation reaction |
1 × 104 |
74
|
Hg2+ |
Hydroxylquinoline |
220 |
400 |
PET |
4.42 |
78
|
Hg2+ |
Pyrazole |
10–20 |
460 |
PET |
7.5 |
79
|
Fe2+ |
Triazole and benzothiadiazide |
140 |
565 |
Formation of complexes between Fe3+ and TAZ-BTTC6 |
100 |
82
|
Fe2+ |
Naphthalimide |
168 |
513 and 523 |
PET |
0.35 |
83
|
Fe2+ |
Urea |
18 |
370 |
PET |
1660 |
84
|
Fe2+ |
RUBNPs |
87.2 |
564 |
Formation of a nonfluorescent complex between a fluorophore and Fe2+ |
1.5 × 104 |
86
|
Fe2+ |
2,6-Diaminopyridine Schiff base |
35 |
358 |
PET |
5.57 |
87
|
Zn2+ |
N,N′-Bis(pyridyl-2yl-methyl)ethylenediamine |
222 |
365 |
PET |
6.721 × 104 |
91
|
Cs+ |
|
65–90 |
412 |
PET |
70 |
92
|
Li+ |
Biginneli |
25 |
353 |
PET |
122 |
95
|
Sr2+ |
Urea |
5–10 |
380 |
PET |
184 000 |
101
|
Al3+ |
1,8-Naphthalimide with diethylenetriamine |
55 |
494 |
PET |
667 |
102
|
Al3+ |
|
50 |
359 |
PET |
280 |
104
|
Sn2+ |
(E)-2-((2-Phenylhydrazono)methyl)phenol |
93 |
532 and 552 |
Excited state complexation formation |
0.218 |
106
|
6. Conclusion and outlook
In conclusion, the toxic effects of metal ions in the environment, particularly in water, have urged chemists and biologists to design probes for monitoring these ions. Due to the ease of preparation, high selectivity and sensitivity, ease of operation, and less toxicity, the fabrication of FONs has proven to be an ideal, effective, and straightforward methodology for the detection of metal ions in aqueous media in the recent years. The objective of this review was to summarize the recent developments in the area of FON-based chemosensors to motivate researchers for planning and constructing a new library of such chemosensors having improved selectivity and sensitivity. Following are some of the properties of an ideal FON-based chemosensor: (i) cheap (ease of preparation/fabrication); (ii) long-lasting stability under humidity and atmospheric oxygen;(III) noncorrosive, nonflammable, and highly thermally stable; (iv) stable toward base and acid hydrolysis actions; (v) excellent solubility in water and other organic solvents; (vi) promising selectivity and sensitivity at lower concentrations; (vii) stable toward oxidative and reductive decompositions; (viii) appropriate detection limit; (ix) appropriate fluorescence quantum yield; (x) ineffective toward impurities in the aqueous media; and (xi) quick response toward ion presence. This area of research has opened up new avenues in the field of nanotechnology/supramolecular chemistry and can attract increased attention from chemists and biologists in the future.
Conflicts of interest
There are no conflicts to declare.
References
- D. T. Quang and J. S. Kim, Chem. Rev., 2010, 110, 6280–6301 CrossRef CAS PubMed.
- G. P. Rao, C. Lu and F. Su, Sep. Purif. Technol., 2007, 58, 224–231 CrossRef CAS.
- F. P. Guengerich, J. Biol. Chem., 2009, 284, 709 CrossRef CAS PubMed.
- J. J. Lacroix, F. V. Campos, L. Frezza and F. Bezanilla, Neuron, 2013, 79, 651–657 CrossRef CAS PubMed.
- E. Boopathi, J. A. Hypolite, S. A. Zderic, C. M. Gomes, B. Malkowicz, H.-C. Liou, A. J. Wein and S. Chacko, Mol. Cell. Biol., 2013, 33, 1085–1102 CrossRef CAS PubMed.
- P. C. Tizioto, C. F. Gromboni, A. R. de Araujo Nogueira, M. M. de Souza, M. de Alvarenga Mudadu, P. Tholon, A. do Nascimento Rosa, R. R. Tullio, S. R. Medeiros and R. T. Nassu, Meat Sci., 2014, 96, 436–440 CrossRef CAS PubMed.
-
M. Csuros and C. Csuros, Environmental sampling and analysis for metals, CRC Press, 2016 Search PubMed.
- J. A. Lemire, J. J. Harrison and R. J. Turner, Nat. Rev. Microbiol., 2013, 11, 371 CrossRef CAS.
- K. P. Carter, A. M. Young and A. E. Palmer, Chem. Rev., 2014, 114, 4564–4601 CrossRef CAS PubMed.
- M. Bauer and S. Döpfmer, J. Clin. Psychopharmacol., 1999, 19, 427–434 CrossRef CAS.
- P. K. Whelton, J. He, J. A. Cutler, F. L. Brancati, L. J. Appel, D. Follmann and M. J. Klag, J. Am. Med. Assoc., 1997, 277, 1624–1632 CrossRef CAS.
- S. C. Bondy, Neurotoxicology, 2016, 52, 222–229 CrossRef CAS PubMed.
- N. D. Barnard, A. I. Bush, A. Ceccarelli, J. Cooper, C. A. de Jager, K. I. Erickson, G. Fraser, S. Kesler, S. M. Levin and B. Lucey, Neurobiol. Aging, 2014, 35, S74–S78 CrossRef.
- K. Hirose, Anal. Sci., 2006, 22, 1055–1063 CrossRef CAS PubMed.
- F. M. M. Morel and N. M. Price, Science, 2003, 300, 944–947 CrossRef CAS PubMed.
- F. Fu and Q. Wang, J. Environ. Manage., 2011, 92, 407–418 CrossRef CAS PubMed.
- F. Ge, M.-M. Li, H. Ye and B.-X. Zhao, J. Hazard. Mater., 2012, 211, 366–372 CrossRef PubMed.
- M. Al-Shannag, Z. Al-Qodah, K. Bani-Melhem, M. R. Qtaishat and M. Alkasrawi, Chem. Eng. J., 2015, 260, 749–756 CrossRef CAS.
- M. Ghaedi, F. Ahmadi and A. Shokrollahi, J. Hazard. Mater., 2007, 142, 272–278 CrossRef CAS.
- M. Arienzo and R. Capasso, J. Agric. Food Chem., 2000, 48, 1405–1410 CrossRef CAS PubMed.
- J. Wu, J. Yu, J. Li, J. Wang and Y. Ying, Spectrochim. Acta, Part B, 2007, 62, 1269–1272 CrossRef.
- R. S. Houk, V. A. Fassel, G. D. Flesch, H. J. Svec, A. L. Gray and C. E. Taylor, Anal. Chem., 1980, 52, 2283–2289 CrossRef CAS.
-
A. Montaser, Inductively coupled plasma mass spectrometry, John Wiley & Sons, 1998 Search PubMed.
- S. Hirata, Y. Umezaki and M. Ikeda, Anal. Chem., 1986, 58, 2602–2606 CrossRef CAS.
- F. J. Hayes, H. B. Halsall and W. R. Heineman, Anal. Chem., 1994, 66, 1860–1865 CrossRef CAS PubMed.
- C. C. Chang, Surf. Sci., 1971, 25, 53–79 CrossRef CAS.
- T. M. Florence, Analyst, 1986, 111, 489–505 RSC.
- J. F. Zhang, Y. Zhou, J. Yoon and J. S. Kim, Chem. Soc. Rev., 2011, 40, 3416–3429 RSC.
- Y.-L. Hung, T.-M. Hsiung, Y.-Y. Chen, Y.-F. Huang and C.-C. Huang, J. Phys. Chem. C, 2010, 114, 16329–16334 CrossRef CAS.
- H. N. Kim, W. X. Ren, J. S. Kim and J. Yoon, Chem. Soc. Rev., 2012, 41, 3210–3244 RSC.
- J. S. Kim and D. T. Quang, Chem. Rev., 2007, 107, 3780–3799 CrossRef CAS PubMed.
- N. Kaur, S. Kaur, R. Mehan, C. A. H. Aguilar, P. Thangarasu and N. Singh, Sens. Actuators, B, 2015, 206, 90–97 CrossRef CAS.
- A. N. Uglov, A. Bessmertnykh-Lemeune, R. Guilard, A. D. Averin and I. P. Beletskaya, Russ. Chem. Rev., 2014, 83, 196 CrossRef.
- B.-K. An, S.-K. Kwon, S.-D. Jung and S. Y. Park, J. Am. Chem. Soc., 2002, 124, 14410–14415 CrossRef CAS PubMed.
- X. Zhang, S. Wang, L. Xu, L. Feng, Y. Ji, L. Tao, S. Li and Y. Wei, Nanoscale, 2012, 4, 5581–5584 RSC.
- Y.-Y. Sun, J.-H. Liao, J.-M. Fang, P.-T. Chou, C.-H. Shen, C.-W. Hsu and L.-C. Chen, Org. Lett., 2006, 8, 3713–3716 CrossRef CAS PubMed.
- A. Patra, C. G. Chandaluri and T. P. Radhakrishnan, Nanoscale, 2012, 4, 343–359 RSC.
- H. Kasai, H. S. Nalwa, H. Oikawa, S. Okada, H. Matsuda, N. Minami, A. Kakuta, K. Ono, A. Mukoh and H. Nakanishi, Jpn. J. Appl. Phys., 1992, 31, L1132 CrossRef CAS.
- Y. Bai, H. Xing, G. A. Vincil, J. Lee, E. J. Henderson, Y. Lu, N. G. Lemcoff and S. C. Zimmerman, Chem. Sci., 2014, 5, 2862–2868 RSC.
- W. Cheng, L. Capretto, M. Hill and X. Zhang, Nanotechnol. Life Sci., 2015, 104, 221–257 Search PubMed.
- T. Asahi, T. Sugiyama and H. Masuhara, Acc. Chem. Res., 2008, 41, 1790–1798 CrossRef CAS.
- Y. Zhou, C.-Y. Zhu, X.-S. Gao, X.-Y. You and C. Yao, Org. Lett., 2010, 12, 2566–2569 CrossRef CAS PubMed.
- L. He, B. Dong, Y. Liu and W. Lin, Chem. Soc. Rev., 2016, 45, 6449–6461 RSC.
- S. Martin and W. Griswold, Environ. Sci. Technol. Briefs Citizens, 2009, 15, 1–6 Search PubMed.
- A. Chatterjee, M. Santra, N. Won, S. Kim, J. K. Kim, S. Bin Kim and K. H. Ahn, J. Am. Chem. Soc., 2009, 131, 2040–2041 CrossRef CAS PubMed.
- H. Yan, H. Su, D. Tian, F. Miao and H. Li, Sens. Actuators, B, 2011, 160, 656–661 CrossRef CAS.
- H. Sharma, V. K. Bhardwaj and N. Singh, Eur. J. Inorg. Chem., 2014, 2014, 5424–5431 CrossRef CAS.
- R. G. Pearson, J. Am. Chem. Soc., 1963, 85, 3533–3539 CrossRef CAS.
- R. G. Pearson and J. Songstad, J. Am. Chem. Soc., 1967, 89, 1827–1836 CrossRef CAS.
- F. Qu, J. Liu, H. Yan, L. Peng and H. Li, Tetrahedron Lett., 2008, 49, 7438–7441 CrossRef CAS.
- G. Kaur, T. Raj, S. Singhal and N. Kaur, Sens. Actuators, B, 2018, 255, 424–432 CrossRef CAS.
- M. B. Gumpu, S. Sethuraman, U. M. Krishnan and J. B. B. Rayappan, Sens. Actuators, B, 2015, 213, 515–533 CrossRef CAS.
- H. Qin, J. Ren, J. Wang and E. Wang, Chem. Commun., 2010, 46, 7385–7387 RSC.
- J. Hou, L.-Y. Wang, D.-H. Li and X. Wu, Tetrahedron Lett., 2011, 52, 2710–2714 CrossRef CAS.
- R. M. Duke, E. B. Veale, F. M. Pfeffer, P. E. Kruger and T. Gunnlaugsson, Chem. Soc. Rev., 2010, 39, 3936–3953 RSC.
- J. Chen, Y. Li, W. Zhong, Q. Hou, H. Wang, X. Sun and P. Yi, Sens. Actuators, B, 2015, 206, 230–238 CrossRef CAS.
- Y. Su, B. Shi, S. Liao, Y. Qin, L. Zhang, M. Huang and S. Zhao, Sens. Actuators, B, 2016, 225, 334–339 CrossRef CAS.
- A. Zhitkovich, Chem. Res. Toxicol., 2011, 24, 1617–1629 Search PubMed.
- S. J. Toal, K. A. Jones, D. Magde and W. C. Trogler, J. Am. Chem. Soc., 2005, 127, 11661–11665 CrossRef CAS PubMed.
- D. K. Dalavi, D. P. Bhopate, A. S. Bagawan, A. H. Gore, N. K. Desai, A. A. Kamble, P. G. Mahajan, G. B. Kolekar and S. R. Patil, Anal. Methods, 2014, 6, 6948–6955 RSC.
- L. Wang, T. Xia, J. Liu, L. Wang, H. Chen, L. Dong and G. Bian, Spectrochim. Acta, Part A, 2005, 62, 565–569 CrossRef PubMed.
- A. Saini, A. K. K. Bhasin, N. Singh and N. Kaur, New J. Chem., 2016, 40, 278–284 RSC.
- X. Zhang, X. Zhang, W. Shi, X. Meng, C. Lee and S. Lee, J. Phys. Chem. B, 2005, 109, 18777–18780 CrossRef CAS PubMed.
- Y. Zhou, F. Wang, Y. Kim, S.-J. Kim and J. Yoon, Org. Lett., 2009, 11, 4442–4445 CrossRef CAS.
- L. Wang, L. Wang, T. Xia, L. DONG, G. BIAN and H. CHEN, Anal. Sci., 2004, 20, 1013–1017 CrossRef CAS.
- P. B. Viviana, C. A. Huerta-Aguilar, N. Singh and T. Pandiyan, Res. Chem. Intermed., 2018, 44, 3179–3197 CrossRef CAS.
- H. J. Jung, N. Singh and D. O. Jang, Tetrahedron Lett., 2008, 49, 2960–2964 CrossRef CAS.
- P. Saluja, V. K. Bhardwaj, T. Pandiyan, S. Kaur, N. Kaur and N. Singh, RSC Adv., 2014, 4, 9784–9790 RSC.
- P. G. Mahajan, D. P. Bhopate, G. B. Kolekar and S. R. Patil, Sens. Actuators, B, 2015, 220, 864–872 CrossRef CAS.
- V. K. Bhardwaj, H. Sharma, N. Kaur and N. Singh, New J. Chem., 2013, 37, 4192–4198 RSC.
- A. Singh, S. Kaur, N. Singh and N. Kaur, Org. Biomol. Chem., 2014, 12, 2302–2309 RSC.
- H. Li and H. Yan, J. Phys. Chem. C, 2009, 113, 7526–7530 CrossRef CAS.
- A. Corsaro and V. Pistara, Tetrahedron, 1998, 54, 15027–15062 CrossRef CAS.
- A. Singh, T. Raj, T. Aree and N. Singh, Inorg. Chem., 2013, 52, 13830–13832 CrossRef CAS.
- L. Wang, L. Dong, G.-R. Bian and T.-T. Xia, Spectrochim. Acta, Part A, 2005, 62, 313–316 CrossRef PubMed.
- H. Wang, P. Zhang, J. Chen, Y. Li, M. Yu, Y. Long and P. Yi, Sens. Actuators, B, 2017, 242, 818–824 CrossRef CAS.
- K. Nie, B. Dong, H. Shi, Z. Liu and B. Liang, Anal. Chem., 2017, 89, 2928–2936 CrossRef CAS PubMed.
- L.-N. Liu, L. He, Y. Qu, N. Lu, Q.-Y. Cao and Z. Yan, Inorg. Chim. Acta, 2018, 474, 128–133 CrossRef CAS.
- N. Mir, A. Heidari, H. Beyzaei, S. Mirkazehi-Rigi and P. Karimi, Chem. Eng. J., 2017, 327, 648–655 CrossRef CAS.
- P. Ponikowski, D. J. Van Veldhuisen, J. Comin-Colet, G. Ertl, M. Komajda, V. Mareev, T. McDonagh, A. Parkhomenko, L. Tavazzi and V. Levesque, Eur. Heart J., 2014, 36, 657–668 CrossRef.
- B. P. Espósito, S. Epsztejn, W. Breuer and Z. I. Cabantchik, Anal. Biochem., 2002, 304, 1–18 CrossRef.
- S. K. Sahoo, D. Sharma, R. K. Bera, G. Crisponi and J. F. Callan, Chem. Soc. Rev., 2012, 41, 7195–7227 RSC.
- J. Wang, X. Xu, L. Shi and L. Li, ACS Appl. Mater. Interfaces, 2013, 5, 3392–3400 CrossRef CAS.
- C. Han, T. Huang, Q. Liu, H. Xu, Y. Zhuang, J. Li, J. Hu, A. Wang and K. Xu, J. Mater. Chem. C, 2014, 2, 9077–9082 RSC.
- S. Chopra, J. Singh, H. Kaur, H. Singh, N. Singh and N. Kaur, New J. Chem., 2015, 39, 3507–3512 RSC.
- P. G. Mahajan, D. P. Bhopate, A. A. Kamble, D. K. Dalavi, G. B. Kolekar and S. R. Patil, Anal. Methods, 2015, 7, 7889–7898 RSC.
- R. Azadbakht, M. Hakimi and J. Khanabadi, New J. Chem., 2018, 42, 5929–5936 RSC.
- T. Kambe, T. Tsuji, A. Hashimoto and N. Itsumura, Physiol. Rev., 2015, 95, 749–784 CrossRef CAS.
- S. M. Y. Kong, B. K. K. Chan, J.-S. Park, K. J. Hill, J. B. Aitken, L. Cottle, H. Farghaian, A. R. Cole, P. A. Lay and C. M. Sue, Hum. Mol. Genet., 2014, 23, 2816–2833 CrossRef CAS.
- P. Jiang and Z. Guo, Coord. Chem. Rev., 2004, 248, 205–229 CrossRef CAS.
- C. A. Huerta-Aguilar, T. Pandiyan, P. Raj, N. Singh and R. Zanella, Sens. Actuators, B, 2016, 223, 59–67 CrossRef CAS.
- C. A. Huerta-Aguilar, T. Pandiyan, N. Singh and N. Jayanthi, Spectrochim. Acta, Part A, 2015, 146, 142–150 CrossRef CAS.
- S. Chopra, N. Singh, P. Thangarasu, V. K. Bhardwaj and N. Kaur, Dyes Pigm., 2014, 106, 45–50 CrossRef CAS.
- S. Rochat, Z. Grote and K. Severin, Org. Biomol. Chem., 2009, 7, 1147–1153 RSC.
- J. Gao, S. Rochat, X. Qian and K. Severin, Chem. – Eur. J., 2010, 16, 5013–5017 CrossRef CAS.
- G. Kaur, A. Singh, P. Venugopalan, N. Kaur and N. Singh, RSC Adv., 2016, 6, 1792–1799 RSC.
- W. E. Cabrera, I. Schrooten, M. E. De Broe and P. C. d'Haese, J. Bone Miner. Res., 1999, 14, 661–668 CrossRef CAS.
- R. H. Wasserman, Clin. Chem., 1998, 44, 437–439 CAS.
-
T. Fukuda, F. Arai and M. Nakajima, Micro-nanorobotic manipulation systems and their applications, Springer Science & Business Media, 2013 Search PubMed.
- H.-F. Ji, Y. Yang, X. Xu and G. Brown, Org. Biomol. Chem., 2006, 4, 770–772 RSC.
- A. Kaur, G. Kaur, A. Singh, N. Singh and N. Kaur, ACS Sustainable Chem. Eng., 2015, 4, 94–101 CrossRef.
- S. Kaur, A. Kaur, N. Kaur and N. Singh, Org. Biomol. Chem., 2014, 12, 8230–8238 RSC.
- A. Saini, J. Singh, R. Kaur, N. Singh and N. Kaur, New J. Chem., 2014, 38, 4580–4586 RSC.
- R. Azadbakht, M. Talebi, J. Karimi and R. Golbedaghi, Inorg. Chim. Acta, 2016, 453, 728–734 CrossRef CAS.
- K. M. Tripathi, T. S. Tran, Y. J. Kim and T. Kim, ACS Sustainable Chem. Eng., 2017, 5, 3982–3992 CrossRef CAS.
- K. S. Patil, P. G. Mahajan and S. R. Patil, Spectrochim. Acta, Part A, 2017, 170, 131–137 CrossRef CAS.
- E. B. Veale and T. Gunnlaugsson, Annu. Rep. Prog. Chem., Sect. B: Org. Chem., 2010, 106, 376–406 RSC.
|
This journal is © The Royal Society of Chemistry 2019 |