Emerging investigators series: the source and fate of pandemic viruses in the urban water cycle
Received
11th May 2015
, Accepted 30th June 2015
First published on 3rd July 2015
Abstract
Several recent high profile outbreaks such as SARS, MERS, Ebola and avian influenzas draw attention to the continued risk of a deadly viral pandemic. In general, these enveloped viruses are not considered a major threat for the wastewater and water industries due to their assumed low concentrations in municipal wastewater and high susceptibilities to degradation in aqueous environments. A number of clinical reports, however, suggest that certain enveloped viruses are excreted in human feces during infection. Furthermore, survivability studies show that many enveloped viruses are capable of retaining infectivity for days to months in aqueous environments. Here, we examine the potential presence and fate of enveloped viruses in the urban water cycle, with emphasis on coronaviruses (e.g., SARS and MERS) and avian influenza viruses. We identify a number of pressing research questions that must be answered before the water and wastewater industries can confidently assure the public, through the dissemination of evidence-based guidance, that irrigation waters, recreation waters, and drinking water sources are safe during a viral outbreak or pandemic event.
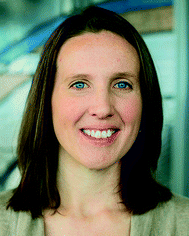
K. R. Wigginton
| Krista Rule Wigginton received her Ph.D. in Environmental Engineering from Virginia Tech under P. J. Vikesland (2008). She conducted postdoctoral research at École Polytechnique Fédérale de Lausanne (2009–2010) under T. Kohn. She was an Assistant Professor of Environmental Engineering at the University of Maryland (2011–2012) and is now an Assistant Professor of Environmental Engineering and the Borchardt and Glysson Water Treatment Faculty Scholar at the University of Michigan. Her main research interests involve the detection and fate of emerging biological contaminants in drinking and wastewater treatment. |
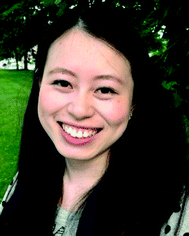
Y. Ye
| Yinyin Ye is a Ph.D. student in Environmental Engineering at the University of Michigan. She received her B.S. degree in Water and Wastewater Treatment Engineering from Tongji University in 2012, and her M.S. degree in Environmental Engineering from the University of Michigan in 2014. Her Ph.D. research under K. Wigginton focuses on the detection and fate of viruses in wastewater. |
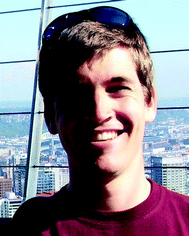
R. M. Ellenberg
| Miles Ellenberg received his B.S. in Civil Engineering from Virginia Tech in 2012. He earned his M.S. in Environmental Engineering from the University of Michigan in 2015. During his M.S. degree, he conducted research with K. Wigginton. Miles currently works for Hazen and Sawyer Consultants in Cincinnati, OH. |
Water impact
Enveloped viruses are generally considered unstable in the aqueous environment and have therefore not been emphasized in waterborne virus research, methods, or regulations. Certain enveloped viruses, however, do survive for long periods of time in wastewaters and surface waters. Avian influenza viruses and some coronaviruses, for example, are relatively stable in water and also occasionally cross over to humans to cause outbreaks of severe disease. This review examines the potential presence and fate of enveloped viruses in municipal wastewater and drinking water. We conclude that future outbreaks or pandemics could involve enveloped viruses that are transmitted via the urban water cycle.
|
Introduction
High-profile viral outbreaks in recent years have heightened concerns of a deadly virus pandemic. These include the severe acute respiratory syndrome coronavirus (SARS-CoV) in 2003, the Middle Eastern respiratory syndrome coronavirus (MERS-CoV) since 2012, the highly pathogenic avian influenzas H5N1 and H797 in 2003 and 2013, respectively, and the recent Ebola outbreak in 2014 (Table 1). The public fear is understandable, as viral pandemics can be devastating; the 1918 influenza pandemic, for example, killed an estimated 50 million people in two years, and one-third of the world contracted the illness.1 Although the number of cases and deaths caused by SARS, MERS, avian influenzas H5N1 and H7N9, and Ebola are orders of magnitudes lower than those caused by the human pandemic influenzas (Table 1), scientists and public health officials carefully monitor for signs that these viruses re-emerge or become more easily transmitted from person to person.
Table 1 Examples of viral diseases that rapidly emerged in humans, likely from an animal source. Viral diseases are listed chronologically with respect to the initial outbreak date
Outbreak/pandemic |
Years |
Most likely animal source |
Deaths |
Approximate case fatality rate |
Genome type |
Ref. |
Data for 2014–15 outbreak only, as of March 24, 2015.
As of December 4, 2014.
In first 12 months of circulation.
As of March 26, 2015.
As of December 10, 2014.
|
“Spanish” pandemic influenza H1N1 |
1918–1920 |
Unresolved |
>40 million |
2–3% |
ssRNA |
1, 5, 6
|
Ebola virus (EBOV) |
1976–present |
Unresolved |
10 353a |
50% |
ssRNA |
7
|
Avian influenza H5N1 |
1997–present |
Birds |
398b |
60% |
ssRNA |
8
|
SARS-CoV |
2002–2003 |
Bats |
774 |
10% |
ssRNA |
9, 10
|
Pandemic influenza H1N1 2009 |
2009–2010 |
Unresolved |
>284 500c |
Up to 0.03% |
ssRNA |
11
|
MERS-CoV |
2012–present |
Unresolved |
456d |
40% |
ssRNA |
7
|
Avian influenza H7N9 |
2013–present |
Poultry |
177e |
40% |
ssRNA |
8
|
Infectious diseases caused by viruses emerge or re-emerge every year, although most do not lead to public health threats as significant as familiar viral pathogens like measles or human seasonal influenza.2 Of the estimated 87 novel pathogens that were first reported in humans over a twenty-five year period (1980–2005), two-thirds were due to viruses.2 RNA viruses, such as SARS and influenza H5N1, made up 85% of the emerging human viruses, primarily due to the high mutation rate of single-strand RNA viruses. Emerging or re-emerging human viruses tend to have animal reservoirs—a phenomenon called zoonosis—which may be exacerbated by increased global travel, deforestation, factory farming, animal markets, and bush meat hunting.3 Climate change may facilitate the spread of some viruses into new geographical regions in the coming years.4
Should a major virus pandemic occur, wastewater and drinking water treatment industries would be under increased scrutiny for serving as a potential means of transmission (Fig. 1). Utilities need to respond rapidly and make decisions that minimize occupational and public health risks based on the cumulative available evidence. Treated wastewater is a common source of recreation, irrigation, and drinking waters, and although wastewater treatment does reduce virus levels, infective human viruses have frequently been detected in wastewater treatment plant effluent.12–14
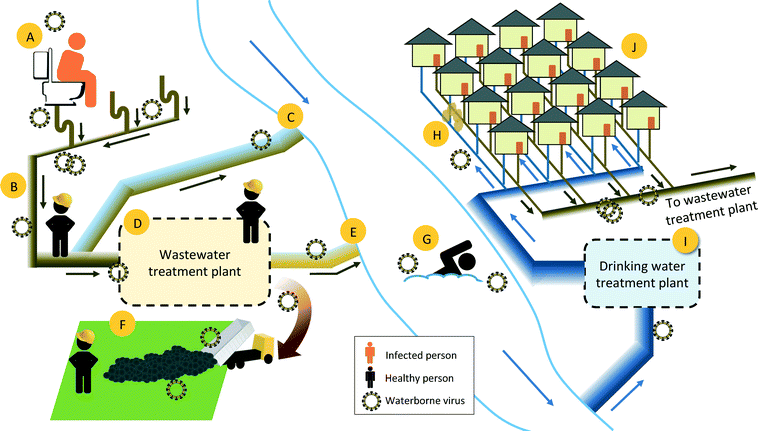 |
| Fig. 1 The fate of infective viruses in the urban water cycle and locations of potential human exposure. A) Viruses that are excreted in feces, urine, and vomit enter the sewage system. Toilet flushing or problems with indoor plumbing systems may form virus-laden aerosols that could result in human exposure. B) Viruses are transported through the municipal sewage system to the wastewater treatment plant (WWTP). Workers servicing sewage systems could be exposed to infective viruses. C) Combined sewage overflow events lead to the release of infective viruses in untreated sewage to surface waters. D) Viruses that enter the municipal WWTP are exposed to physical, biological, and chemical treatment processes. WWTP employees may be exposed to infective viruses present in the untreated and treated wastewater, as well as residual biosolids. E) Wastewater effluent can carry viruses that have survived treatment to surface waters. F) Residual biosolids from WWTP are disposed, often via land-application. Workers or others in close contact with the biosolids may be exposed to infective viruses that have survived the solids treatment processes. G) Recreational activities can lead to exposure to infective viruses present in surface waters. H) Leaky sewage pipes can lead to contamination in the underground drinking water distribution systems. I) Intake water at drinking water treatment plants can contain infective viruses. The water is treated with a range of physical and chemical treatment processes to remove contaminants, including viruses. J) Municipal drinking water consumers are exposed to viruses that either maintain infectivity through drinking water treatment and distribution or enter the distribution system through leaks in underground pipes. | |
If the novel human viruses were shed in the feces, urine, or vomit of infected individuals, it would enter the municipal wastewater system and be transported to the municipal wastewater treatment plants (WWTPs). The 2014 Ebola cases in the U.S. highlighted the lack of data on the presence and fate of emerging viruses in human waste and sewage.15 In this case, government agencies communicated that the Ebola virus was rapidly inactivated outside of the human host and thus municipal wastewater and finished wastewater treatment products did not carry significant occupational and public health risks. At the time, however, there were very few survivability studies of Ebola virus outside of the human host and none in municipal wastewater. Studies on the fate of Ebola virus and Ebola virus surrogates in the environment have since been initiated and results are beginning to appear in the literature.16,17 At this time, the assumptions that were made about the rapid inactivation of Ebola virus outside of the human host have not been validated.
The vast majority of research on the presence and fate of viruses in the urban water cycle has focused on a relatively small set of enteric viruses (i.e., viruses that replicate in the gastrointestinal tract and are readily transmitted via the fecal-oral route; Table 2). Enteric virus particles consist of an RNA or DNA genome that is protected by a protein shell (i.e., capsid). Enteric viruses are considered resistant to heat, acids, and oxidants, and thus survive for long periods of time in the environment. Enveloped viruses, such as influenza viruses, coronaviruses, and Ebola virus, have an additional outer envelope that consists of lipids and proteins. Enveloped viruses are generally not associated with fecal routes of transmission in humans and are considered more susceptible to inactivation in aqueous environments. Recently, however, viral metagenomes of sewage have revealed a large diversity of human viruses including some enveloped viruses.18,19 Although the presence of genes in wastewater does not equate with the presence of infective viruses, the recent wastewater metagenomics studies do prompt a closer look at the preparedness of water industries and government agencies for a pandemic or outbreak event.
Table 2 Human viruses of interest to water and wastewater industries
Virus common name |
Associated illnesses |
Taxonomy |
Genome/capsid types |
Reported concentrations in wastewater |
Norovirus |
Gastroenteritis |
Family Caliciviridae Genus Norovirus |
(+)ssRNA non-enveloped |
≤109 gc L−1 (ref. 13, 37–40) |
|
Sapovirus |
Gastroenteritis |
Family Caliciviridae Genus Sapovirus |
(+)ssRNA non-enveloped |
≤108 gc L−1 (ref. 41) |
|
Adenoviruses |
Respiratory disease, pneumonias, gastroenteritis, keratoconjunctivitis |
Family Adenoviridae |
dsDNA non-enveloped |
≤108 gc L−1 (ref. 13, 38, 42–44) ≤104 IU L−1 (ref. 13) |
|
Astrovirus |
|
Family Astroviridae |
(+)ssRNA non-enveloped |
≤105 gc L−1 (ref. 41) |
|
Rotavirus |
Gastroenteritis |
Family Reoviridae |
dsRNA non-enveloped |
≤107 gc L−1 (ref. 43, 45) ≤103 PFU L−1 (ref. 46) |
|
Hepatitis A virus |
Hepatitis |
Family Picornaviridae Genus Hepatovirus |
(+)ssRNA non-enveloped |
≤106 gc L−1 (ref. 47) |
|
Enteroviruses |
Respiratory illness, meningitis, flaccid paralysis, etc. |
Family Picornaviridae Genus Enterovirus |
(+)ssRNA non-enveloped |
≤106 gc L−1 (ref. 13, 40, 41) ≤103 PFU L−1 (ref. 13, 46) |
|
Torque Teno virus |
Unclear |
Family Anelloviridae |
ssDNA non-enveloped |
≤105 gc L−1 (ref. 41, 44, 48) |
|
Hepatitis E virus |
Hepatitis |
Family: Hepeviridae Genus: Orthohepevirus |
(+)ssRNA non-enveloped |
≤105 gc L−1 (ref. 49) |
|
Aichi virus |
Gastroenteritis |
Family: Picornaviridae Genus: Kobuvirus |
(+)ssRNA non-enveloped |
≤107 gc L−1 (ref. 43) |
|
Klassevirus/Salivirus |
Unclear |
Family Picornaviridae |
(+)ssRNA non-enveloped |
≤107 gc L−1 (ref. 50) |
|
Polyomavirus |
Unclear |
Family Polyomaviridae |
dsDNA non-enveloped |
≤108 gc L−1 (ref. 41, 43, 44, 51) |
|
Ebolavirus |
Ebola |
Family Filoviridae |
(−)ssRNA enveloped |
NA |
|
SARS coronavirus |
Severe Acute Respiratory Syndrome |
Family Coronaviridae Genus: Betacoronavirus |
(+)ssRNA enveloped |
NA |
|
MERS coronavirus |
Middle Eastern Respiratory Syndrome |
Family Coronaviridae Genus: Betacoronavirus |
(+)ssRNA enveloped |
NA |
|
Avian influenza |
Influenza |
Family Orthomyxoviridae Genus: Influenza virus A |
(−)ssRNA enveloped |
NA |
Here, we examine the literature on emerging viruses in wastewater with the intention of informing and preparing wastewater and drinking water treatment industries for future virus pandemics. We apply a definition of pandemic based on the World Health Organization guidelines for identifying an influenza pandemic, or the emergence of a new virus that causes serious illness in humans and experiences easy and sustained human-to-human transmission.20 This is in contrast to an outbreak event, wherein the incidence of a disease rises above levels considered normal for a specific area. Two types of enveloped viruses are particularly important for wastewater and drinking water communities to consider—coronaviruses and avian influenza viruses—due to their confirmed presence in feces and their survival characteristics in aqueous environments. Coronaviruses include the viruses responsible for the recent SARS and MERS global outbreaks. Avian influenza viruses usually cause illness in birds, but occasionally cross over to humans and result in serious illnesses. Although typically considered respiratory viruses, certain coronaviruses (e.g., SARS) and avian influenza viruses can be transmitted via fecal matter in water; they have also been responsible for recent outbreaks with particularly high mortality rates (Table 1). As mentioned above, these enveloped viruses are structurally dissimilar to the enteric viruses that have historically been the focus of waterborne virus studies and are thus believed to behave differently in aqueous environments.
Potential pandemic viruses in wastewater
Human viruses do not replicate in the environment; therefore, for a virus to be transferred via the urban water cycle, it must be introduced to water through human bodily fluids and then retain its infectivity until another person comes into contact with the water (Fig. 1). Studies on the presence and fate of viruses in aqueous environments are often limited to enteric viruses, as are regulations, such as those specified by the USEPA Surface Water Treatment Rule and Groundwater Treatment Rule.21 Wastewater can contain a number of human viruses outside of the common enteric viruses; untreated wastewater sludge and Class B biosolids, for example, contained genes from coronaviruses in more that 80% of the samples, and rubella virus at lower frequencies.19
Human viruses enter municipal wastewater when they are shed in the feces and urine of infected individuals. A number of human enteric viruses have been quantified in municipal wastewater by qPCR and culture methods, with reported concentrations as high as 109 genome copies per liter (Table 2). For those viruses that have not been studied in wastewater, existing information on their presence in human fecal and urine samples may shed light on their potential importance in the water cycle. Feces and urine samples are rarely collected for illnesses perceived as non-enteric, but genes of the respiratory viruses RSV, human rhinovirus, coronaviruses, and seasonal influenza have been detected in stool samples.22–25 In many cases, the presence of respiratory virus genes in feces is thought to stem from a patient swallowing virus-laden nasal secretions. Unfortunately, the PCR techniques commonly used to detect viruses in human samples do not relay infectivity information. To be of concern in the urban water cycle, an entire infective virus particle, and not just pieces of viral genome, would need to be present in wastewater. Indeed, infective SARS-CoV and avian influenza virus particles have been detected in fecal or intestinal tract samples of infected individuals.26–28
Influenza viruses
Influenza viruses are enveloped viruses with segmented, single-stranded RNA genomes. They infect humans and other animals, such as birds and pigs. Influenza viruses have been responsible for four human pandemics in the last century, including the 1918 H1N1 “Spanish Flu” pandemic, the 1957–58 H2N2 “Asian Flu” pandemic, the 1968–69 H3N2 “Hong Kong Flu” pandemic, and the 2009 H1N1 “Swine Flu” pandemic. These pandemics were instigated by the emergence of novel strains to which humans lacked immunity. Novel strains emerge in humans when animal influenza viruses either mix with human viruses or cross directly to humans (e.g., avian influenza viruses).
Human influenza viruses
Seasonal human influenza viruses constantly circulate in human populations around the globe causing seasonal epidemics. The viruses slowly mutate as they circulate via a phenomenon called antigenic drift. The illnesses associated with the seasonal influenza viruses typically involve respiratory tract symptoms and fever. Gastrointestinal symptoms are occasionally experienced, especially in children.29 Transmission of seasonal human influenza occurs through droplet, airborne, and contact transmission modes; however, the relative importance of these three modes of transmission remains unclear.30 As for the potential transmission of human seasonal influenza via wastewater, viral RNA has been detected in human stool samples24,31–33 and has also been detected in municipal wastewater.34,35 Levels in feces ranged from 4.9 × 103 to 8.0 × 107 PCR copies per gram of stool. In one rare case, infective human influenza virus was cultured from human feces,36 but this was suspected to be due to the patient swallowing virus-laden nasopharyngeal secretions, or the spillover of viruses in the blood to other organs.32 Influenza virus receptors are not present on normal human intestinal cells, thus it is unlikely that the viruses infect and replicate in gut cells.32 Based on the available evidence in the literature, high concentrations of infective human seasonal influenza viruses are unlikely in municipal wastewater, even during large outbreaks.
Avian influenza viruses
Birds can be infected by either low pathogenic influenza or highly pathogenic influenza. Whereas low pathogenic avian influenza viruses cause minor illness in birds and are rarely fatal, highly pathogenic avian influenza viruses spread rapidly in birds and are highly virulent. On rare occasions, the highly pathogenic avian influenza viruses have crossed directly over to humans, often when humans were in close proximity to large numbers of domesticated birds. Avian influenza virus infections in humans have had high associated fatality rates (Table 1), but recent avian influenza viruses in humans have not undergone sustained human-to-human transmission. There are serious concerns that an avian influenza will cross over to humans in the future and mutate in a way that allows the virus to spread easily from human to human. This hypothetical event could lead to a deadly avian influenza pandemic in humans like the Spanish influenza pandemic in the early 20th century.
Unlike seasonal human influenza, the highly pathogenic avian influenza strains are transmitted via the fecal-oral route in birds and bind with receptors on avian gut tissues (i.e., gastrointestinal tract infections).52 When the highly pathogenic H5N1 virus crossed over from birds to humans in 2004, many patients experienced severe diarrhea,28,53 viruses were present in stool samples,28 and the viruses infected and replicated in human gut tissues.54 Genes of the novel avian influenza H7N9 virus that emerged in humans in 2013 were also frequently detected in stool samples.8,55 Although not identified as an avian influenza, the H1N1 illness in humans also exhibited unusually high gastrointestinal symptoms,36,56 infective virus particles in feces,57 and efficient replication in intestinal cells.58
Taken together, the frequency of gastrointestinal symptoms, the prevalence of virus particles in the patient stool samples, and the ability of the viruses to replicate in gastrointestinal tract cells, highlights the potential importance of the urban water cycle in the control of avian influenza viruses in humans. Thus far, humans infected with avian influenza viruses, such as H5N1 and H7N9, have been limited in their ability to transmit the illness to other humans. Public health officials, however, fear that a new strain will emerge in humans that spreads easily from person to person; should this occur, humans would not have immunity to the illness and effective vaccines would take time to prepare.59 It seems likely that under potential outbreak or pandemic scenarios, avian influenza virus particles would enter municipal wastewater, possibly at high concentrations.
Coronaviruses
SARS-CoV emerged in Hong Kong in 2003 and caused severe lower respiratory illness that exhibited high mortality rates. During one cluster of 319 cases at an apartment complex in Hong Kong,8,60 the outbreak spread via aerosolized fecal particles in the air ducts of the apartment complex. Despite being associated primarily with respiratory illness, SARS-CoV RNA was often detected in the feces of infected individuals, with the detection frequencies in different cohorts ranging from 16% to 97%.26,61 Diarrhea symptoms were experienced in 23% to 73% of patients, with higher frequency during the first week of the illness.26,62,63 SARS-CoV RNA levels peaked in fecal samples at 9 to 14 days after the onset of the illness,61 and in one case, fecal samples tested positive for up to 73 days following the illness onset.26 The presence of SARS-CoV in feces may be due to its ability to replicate in the small and large intestines, as evidenced by infective viruses in lower intestine samples.26
In addition to SARS-CoV, there are five other known human coronaviruses. MERS-CoV emerged in Saudi Arabia in 2012, and at the time of this writing, has resulted in 1110 confirmed cases and 456 deaths.7 MERS-CoV RNA was detected at low levels in the stool and urine of one infected individual (~103 gene copies per mL),64 but absent in stool samples from two other infected individuals.65 Likewise, human coronavirus HKU1 (HKU1-CoV) RNA has also been detected in stool samples,66 in some cases from patients exhibiting gastrointestinal symptoms.67 In a recent metagenomic study of wastewater, HKU1-CoV was identified in sludge samples collected from different wastewater treatment plants in the U.S., although confirmation with PCR was not conducted.19 Unfortunately, the infectivity state of the HKU1 viruses in stool and wastewater samples cannot be assessed, as there is no tissue culture system compatible with HKU1. The remaining three human coronaviruses (HCoVNL63, HCoV-OC43, and HCoV-229E), have been detected in stool samples25,67,68 but are not believed to play a significant role in infections of the gastrointestinal tract. Based on the available data, the human coronaviruses that are circulating at this point are unlikely to pose significant threats in the urban water cycle. That being said, it is quite plausible that a highly virulent new coronavirus like SARS-CoV will emerge in the future and pose challenges for the water and wastewater industries.
Fate of enveloped viruses through the urban water cycle
The major components of the urban water cycle include drinking water supply, wastewater disposal, and stormwater runoff. Viruses excreted in feces, urine, or vomit enter the urban water cycle in human sewage. We therefore focus most of our discussion on enveloped virus fate in the urban water cycle on wastewater and wastewater treatment. We also include a discussion on the very limited data on enveloped virus fate in natural waters and in drinking water treatment processes.
Virus concentrations in wastewater
In a pandemic scenario, virus concentrations in wastewater would depend on the number of people infected in the community and the rate at which infected individuals shed the viruses. Most data on human virus titers in wastewater are based on enteric viruses and qPCR measurements (Table 2). Concentrations as high as 108–109 genome copies per liter (gc L−1) have been reported in wastewater influent. Relatively few studies have assessed the levels of infective virus particles in wastewater, likely due to limited cell culture techniques and issues with virus extraction methods. Enteroviruses, adenoviruses, and rotaviruses have been monitored in wastewater influent, and reported concentrations are generally less than 104 plaque forming units (PFU) or infective units (IU) per liter.13,46,69 Viruses released in feces tend to be in aggregated states and virus extraction methods have notoriously low recovery rates;51,70 therefore, the reported PFU L−1 values may greatly underestimate the number of infective viral particles in wastewater.
When data on virus loads in wastewater are absent, virus loads in human stool or urine samples may help predict levels in wastewater during an outbreak event. As expected, viruses associated with enteric disease have higher viral loads in human stool samples than those associated with respiratory disease. Norovirus concentrations in human fecal samples can be greater than 1010 gc g−1,71,72 compared to concentrations as high as 109 gc L−1 in sewage during outbreaks.39 Human polyomaviruses (JCPyV and BKPyV) in human urine reach concentrations higher than 1010 gc L−1 and are present in wastewater at levels up to 108 gc L−1.44 Influenza viral loads as high as 8.0 × 107 gc g−1 stool33,57 and SARS virus loads of 107 gc mL−1 in diarrhea73,74 and 2.5 × 104 gc mL−1 in urine73 have been reported. As these are the highest reported levels, they could be used to predict worst-case scenarios for wastewater concentrations.
Survival in municipal wastewater
To be of concern in the urban water cycle, a virus that is excreted in feces or urine must retain its infectivity in sewage until it comes into contact with humans (Fig. 1). Human waste that enters the sewage system is carried through a complex underground pipe system to the municipal wastewater treatment plant. Although the majority of municipal wastewater produced in urban settings in developed countries reaches the wastewater treatment plants, a portion of the wastewater leaks into the subsurface or is released untreated to surface waters during major wet weather events. The untreated wastewater released from leaky pipes to the subsurface can potentially penetrate drinking water distribution pipes and thus result in drinking water exposure. Untreated wastewater released to surface waters can lead to exposure through recreational activities and can contaminate groundwater that is under the direct influence of surface water.
The hydraulic residence times of sewage networks and wastewater treatment plants are typically less than one day, combined.82,83 Outside of the human body, viruses exhibit a range of susceptibilities to environmental factors, with T90 values (i.e., time until 90% of viruses inactivated) in aqueous environments ranging from minutes to years (Table 3). Data on survivability is limited to viruses that can be assayed in vitro; the survivability of nonculturable viruses is often predicted based on the survivability of similar viruses that can be cultured.
Table 3 Time for enveloped viruses to reach 90% inactivation (T90) in aqueous environments compared to time for non-enveloped poliovirus to reach 90% inactivation
Virus |
T
90 (days) |
Temp. (°C) |
Matrix |
Ref. |
Avian influenza virus H5N1 |
84 |
20 |
Distilled water |
75
|
Avian influenza virus H5N1 |
508 |
10 |
Distilled water |
75
|
Avian influenza virus H5N1 |
19 |
20 |
Surface water |
75
|
Avian influenza virus H5N1 |
61 |
10 |
Surface water |
75
|
SARS-CoV |
9 |
RT |
Serum-free culture media |
76
|
HCoV 229E |
<1 |
RT |
Serum-free culture media |
76
|
HCoV 229E |
2 |
RT |
Dechlorinated tap water |
77
|
FIPV (feline coronavirus) |
<1 |
RT |
Primary wastewater effluent |
77
|
TGEV (swine coronavirus) |
11 |
25 |
Reagent-grade water |
78
|
TGEV |
110 |
4 |
Reagent-grade water |
78
|
TGEV |
4 |
25 |
Pasteurized settled wastewater |
78
|
TGEV |
24 |
4 |
Pasteurized settled wastewater |
78
|
MHV (murine coronavirus) |
9 |
25 |
Reagent-grade water |
78
|
MHV |
>365 |
4 |
Reagent-grade water |
78
|
MHV |
3 |
25 |
Pasteurized settled wastewater |
78
|
MHV |
35 |
4 |
Pasteurized settled wastewater |
78
|
Hantavirus |
3 |
20 |
Cell culture media |
79
|
HIV |
<1 |
25 |
Primary wastewater effluent |
80
|
Poliovirus |
4 |
23 |
Primary wastewater effluent |
77
|
Poliovirus |
56 |
23 |
Mineral water |
81
|
Poliovirus |
342 |
4 |
Mineral water |
81
|
Viruses with lipid envelopes are often assumed to readily lose infectivity in aqueous environments. Indeed, viruses such as Human Immunodeficiency Virus (HIV) do rapidly lose their infectivity in aqueous environments, with reported T90 values in water at room temperature in the range of 1–2 hours.84 For comparison, the enteric non-enveloped poliovirus has a T90 value of 56 days in water at room temperature.81 Interestingly, not all enveloped viruses rapidly lose their infectivity (Table 3). Avian influenza H5N1, for example, has a T90 value of approximately 100 days at room temperature in distilled water75,85 and SARS-CoV has a T90 value of 9 days in culture media at room temperature. Inactivation rates are highly dependent on the water temperature and matrix. Higher temperatures are associated with higher inactivation rates,86 as is increased salinity.85 In two studies of human coronaviruses in water,77,78 temperature was found to have the most pronounced effect of a range of variables on survivability, with up to an order of magnitude difference in decay rates between samples at 4 °C and room temperature. The water composition, such as the presence of proteins or microorganisms, also influences virus survivability. Viruses in sterilized wastewater, for example, are inactivated at different rates than viruses in non-sterilized wastewater.87 The presence of suspended solids and organic material increased the survivability of enteric viruses in aqueous environments.88 Likewise, coronaviruses survived longer in unfiltered primary effluent than in filtered primary effluent.77 The enhanced survival in the presence of sediment and organic material may be due to protection from chemical or biological inactivating agents in the water. Extraneous material can also cause faster inactivation, as was the case for coronaviruses in pasteurized settled sewage versus distilled water.78 Based on the collective data, matrix effects on virus inactivation are complex and vary significantly amongst different viruses and environmental samples.
Highly related strains can have significantly different environmental stabilities (Table 3). SARS-CoV, for example, lost its infectivity in culture media at a much slower rate than human coronavirus 229E under the same conditions (Table 3).76 Likewise similar avian influenza viruses had T90 values ranging from 58 to 171 days under the same experimental conditions.85 A mechanistic understanding of this difference in susceptibility is unknown. The virus lipid membranes may play a role, as seasonal influenza virus grown in different cell types, and thus having different sources of their lipid membranes, had varied stabilities in water.89 Based on the cumulative survivability data, it is plausible that an enveloped virus excreted in human feces or urine could survive in aqueous environments for periods of time that are relevant to the wastewater and drinking water treatment fields. Higher levels of infective viruses would be expected in a wastewater treatment plant influent when there is a large incidence rate in the community and when wastewater temperatures are cooler.
Wastewater treatment
To our knowledge, there are no reports on the fate of enveloped viruses through wastewater treatment trains. The extent of non-enveloped human virus removal and inactivation depends on the type of virus and on the treatment processes employed at a particular WWTP. Enteroviruses, adenoviruses, and reoviruses are poorly removed from primary wastewater treatment (i.e., settling), with less than 1-log reduction reported.90,91 Enteroviruses and adenoviruses are more effectively removed in secondary treatment, with 1–4 log reduction reported,90–92 but reovirus reduction was less than 1 log.91 The mechanism of removal in activated sludge has been attributed to adsorption to solids that settle in secondary clarifiers, followed by inactivation of the adsorbed viruses.90,93 The final disinfection step is critical for reducing the number of infective viruses in effluent,94 but even disinfected effluent can contain infective viruses.91,95 A survey of ten plants of various size and treatment processes found that wastewater treatment lead to between 0- and 2-log reduction of infective enteroviruses and 2- to >3-log reduction of infective adenoviruses, although effluent samples were collected prior to disinfection.51 A different survey of five WWTPs found that the log reduction from the influent to the disinfected effluent ranged from 1.9 to 5.0 and infective viruses were detected in the effluent of every plant.42
In solids treatment, digestion has varying effects on non-enveloped viruses, with thermophilic aerobic digestion being much more effective than mesophilic digestion.96 Infectious enteroviruses and adenoviruses were frequently detected in Class B biosolids treated with mesophilic digestion.42,97 Concentrations of enteroviruses and adenoviruses in the mesophilic digestion biosolids were in the range of 101–103 and 102–104 most probable number (MPN) per gram, respectively. Treating biosolids with heat pelletization and composting processes resulted in significantly lower indicator virus levels in final products compared to mesophilic and thermophillic digestion processes.98 Once applied to land, viruses adsorbed to the biosolids can leach out.99 There is also evidence that viruses in biosolids are aerosolized during land-application, leading to a significant risk of airborne exposure.100 It should again be noted that all of these studies on virus fate in wastewater treatment have focused on non-enveloped viruses.
Survival in natural waters
Infective viruses present in WWTP effluent or in combined sewage overflow are usually released into surface waters. In surface waters, viruses are exposed to a number of potentially inactivating stresses, including sunlight, oxidative chemicals, predation by microorganisms, etc.
Research on enveloped viruses in natural waters has focused primarily on avian influenza viruses, due to the likelihood that natural waters serve as environmental reservoirs for influenza viruses. Avian influenza virus survival times in surface waters samples collected from Lake Constance were in the range of 10–100 days at temperatures above freezing (Table 3).75 In this case, the samples were not exposed to sunlight. Avian influenza viruses survived several months to years in frozen natural water samples.75,101 The viruses are most stable between pH 7.4 and 8.2, with rapid inactivation below pH 6.5 and above pH 10.102 There remains a lack of published data on avian influenza inactivation by sunlight.102
Drinking water treatment
Studies exploring the fate of enveloped viruses in drinking water treatment demonstrate the need for additional experiments. The coagulation-flocculation-settling process was not effective or consistent in removing influenza H5N1.31 Ultrafiltration was effective at removing influenza H5N1, and bacteriophage MS2 was a conservative surrogate for this process.31 In general, enveloped viruses are more susceptible to common drinking water disinfectants than non-enveloped viruses. Influenza H5N1 was readily inactivated with UV254 treatment, with greater than 5-log inactivation observed after a dose of 25 mJ cm−2; for comparison, bacteriophage MS2, a surrogate for enteric viruses, underwent less than 2-log inactivation at the same dose.31 Low pathogenicity influenza H5N2, a surrogate for high pathogenicity influenza H5N1, was also very susceptible to UV.34 SARS-CoV, on the other hand, was persistent in experiments with UV disinfection.103 Chlorination readily inactivated influenza in aqueous samples.31,34 With monochloramine disinfection, influenzas H1N1 and H5N1 required monochloramine CT values (i.e., products of disinfectant dose and contact time) of less than 60 mg L−1 min−1 for 4-log inactivation at ambient temperature;31 EPA recommends a monochloramine CT value higher than 700 mg L−1 min−1 for 4-log virus inactivation at 20 °C. More experiments are needed on enveloped viruses in water quality engineering processes before major conclusions can be drawn on their fate in wastewater and drinking water treatment.
Detecting enveloped viruses in water
Research on enveloped virus presence and fate in water is hindered by the lack of proven detection methods. Detecting and quantifying viruses in environmental samples requires first concentrating the viruses in the sample into a smaller volume to improve detection limits. Most available methods were designed and optimized for non-enveloped enteric viruses, and despite efforts, there remains no consensus concentration method.104 The published enteric virus concentration methods focus on combinations of filtration,14,70 ultracentrifugation,70 and PEG precipitation,105 with a range of pH values, chemicals added, filter types, and centrifuge speeds. Envelopes render the viruses more sensitive to organic solvents, temperature, and pH;76 therefore many extraction and purification methods used for non-enveloped enteric viruses are not optimal for enveloped viruses. Protocol steps with chloroform or cesium chloride solutions, for example, destroy the lipid outer layer and should therefore be avoided.106 A mere 1.02% recovery of SARS coronaviruses was achieved from hospital sewage with an electropositive filtration and aluminum hydroxide precipitation method.107 In comparison, non-enveloped viruses have been recovered from wastewater at rates as high as 80%,108 although recovery rates are often much lower. In one of the few attempts to optimize enveloped virus recovery from environmental samples, Deboosere et al. achieved an 8% recovery for influenza A viruses in lake water using glass wool filtration and polyethylene glycol (PEG) precipitation.109 Even when purification steps are included, virus concentrates often contain material that interferes with the detection methods, but these can be minimized by dilution or the addition of quenching agents.110–112 Future successful environmental monitoring efforts will depend on the development and wide acceptance of effective extraction and purification techniques.
Viruses present in sample concentrates are typically identified or quantified by genomic- and culture-based methods. PCR methods detect virus genes and are presently used most often for detecting specific viruses in environmental and clinical samples. The prevalence of PCR methods is due to the fact that they tend to be much faster than culture methods, they provide species and strain-specific identification, and they detect viruses that are not presently culturable in vitro. Drawbacks of PCR methods are that they do not relay virus infectivity and they require the development of appropriate primers; consequently, viruses may go undetected if primers are improperly designed or if the virus has mutated in the targeted genome region. Metagenomic methods, which aim to sequence all of the viruses present in a sample, are increasingly employed for virus detection and discovery, and do not require a priori knowledge of the viruses present. Both RNA and DNA viruses have been sequenced in fecal and environmental samples.113 RNA viruses are sequenced by first reverse transcribing the RNA with random primers, and then sequencing the cDNA. At this time, high-throughput sequencing is not appropriate for routine virus measurements, due to high sample costs, large data sets, and the lack of absolute quantification. Additionally, the detection limits for a specific virus using metagenomic analyses is typically poorer than PCR due to the high abundance of genetic material in samples.114 Following sequencing, PCR is often used to confirm the presence of the detected viruses of interest.113,114 It should be noted that the methods used to concentrate the viruses and separate them from other organisms can influence the viral metagemonic dataset.115
Virus culture methods, such as plaque assays or cytopathic effect assays, continue to play a critical role in environmental virology due to their ability to characterize the infectivity state of the virus—this is not possible with PCR or sequencing techniques. For example, when the fate of human viruses through environmental or engineering processes is of interest, or when the risk a sample poses to human health needs to be assessed, culture methods remain the only reliable detection approach. Unfortunately, a number of important human viruses cannot be cultured in vitro, such as MERS-CoV. For those viruses, molecular detection methods remain the best and only option.
PCR-based and culture-based methods are both employed to detect influenza viruses in human or environmental samples. The matrix (M) gene is often targeted in PCR to detect all influenza subtypes simultaneously, whereas the hemaglutinin (HA), neuraminidase (NA), and other genes are targeted to detect specific influenza subtypes.116,117 Potential novel strains are detected by a positive influenza A assay result followed by negative subtype-specific assay results; this approach led to the identification of the 2009 H1N1 pandemic influenza.118 Influenza A viruses are readily cultured and enumerated in embryonated chicken eggs and a number of cell lines, with the Madin-Darby canine kidney (MDCK) cell line preferred.119 Viruses cultured in MDCK cells can be enumerated via plaque assay or 50% tissue culture infectious dose (TCID50) assays.
For PCR detection of coronaviruses, primers that target conserved regions in the polymerase gene can detect most, if not all coronaviruses, with a limit of detection on the order of 5 × 101–5×103 gene copies.120–122 Degenerate primers are also used to detect a range of coronaviruses.68 Specific coronavirus primers have targeted the S glycoprotein or nucleocapsid protein genes.68,123,124 Due to their varied cell tropisms, human coronaviruses cannot be cultured in the same type of cell systems. SARS-CoV and HCoV-NL63, for example, are readily culturable in common cell lines such as CaCO-2 (SARS) and LLC-MK2 (HCoV-NL63).125,126 HCoV-229E and HCoV-OC43 are also culturable in vitro, but are much more fastidious, and replicate in a narrow range of hosts. The remaining two known human coronaviruses, HCoV-HKU1 and MERS-CoV, are not readily culturable in available cell lines. Consequently, their infectivity state and ultimate fate in environmental samples cannot be easily assayed.
Conclusions
At this time, major knowledge gaps exist on the potential role of the urban water cycle in the spread of enveloped viruses, particularly for avian influenza viruses and coronaviruses. In order to address this, we suggest environmental engineers, virologists, and public health researchers work together to address the following research needs:
(1) Methods need to be developed and optimized for extracting and purifying enveloped viruses from complex sample matrices such as wastewater, residual biosolids, and surface waters. During testing, samples should be spiked with a range of enveloped viruses, as well as with enteric viruses or enteric virus surrogates, in order to develop a better understanding of how extraction recoveries vary with virus physical properties.
(2) Research should address the variability of enveloped virus survivability in aqueous environments. What structural characteristics of certain avian influenza strains, for example, allow them to remain infective in water for longer periods of time than other enveloped viruses?
(3) Research should address the presence and fate of enveloped viruses during wastewater and drinking water treatment processes, as well as when they are released into the environment via wastewater effluent or land-applied biosolids. Whenever possible, these should focus on viruses that are culturable in vitro so that infective virus concentrations, as well as gene copy concentrations, are reported.
(4) More research should focus on the environmental fate of the highly pathogenic viruses, such as avian influenza strains. As demonstrated by the data in Table 3, similar viruses can exhibit very different survivability characteristics and surrogates may not accurately predict the environmental fate of a highly pathogenic virus. This type of work requires access to BSL3 and BSL4 facilities. As most environmental virologists do not have access to or training for this level of biosafety, collaborative efforts will be required.
(5) Quantitative risk assessments should be conducted for highly pathogenic enveloped viruses in wastewater, recreational waters, and drinking waters.
These research questions are critical to prepare the environmental engineering and public health communities in the event that enveloped viruses causing a deadly outbreak or pandemic enter the urban water cycle.
References
- J. K. Taubenberger and D. M. Morens, Annu. Rev. Biomed. Eng., 2006, 17, 69–79 Search PubMed.
- M. Woolhouse and E. Gaunt, Crit. Rev. Microbiol., 2007, 33, 231–242 CrossRef PubMed.
- N. D. Wolfe, P. Daszak, A. M. Kilpatrick and D. S. Burke, Emerging Infect. Dis., 2005, 11, 1822–1827 CrossRef PubMed.
- S. Altizer, R. S. Ostfeld, P. T. Johnson, S. Kutz and C. D. Harvell, Science, 2013, 341, 514–519 CrossRef CAS PubMed.
- J. K. Taubenberger, A. H. Reid, R. M. Lourens, R. Wang, G. Jin and T. G. Fanning, Nature, 2005, 437, 889–893 CrossRef CAS PubMed.
- G. Vana and K. M. Westover, Mol. Phylogenet. Evol., 2008, 47, 1100–1110 CrossRef CAS PubMed.
-
ECDC, Communicable Disease Threats Report Week 13, 22–28 March 2015.
-
ECDC, Communicable Disease Threats Report Week 50, 7–13 December 2014.
-
World Health Organization, Global Alert and Response: Summary of probable SARS cases with onset of illness from 1 November 2002 to 31 July 2003, 2004.
- X. Y. Ge, J. L. Li, X. L. Yang, A. A. Chmura, G. Zhu, J. H. Epstein, J. K. Mazet, Ben Hu, W. Zhang, C. Peng, Y. J. Zhang, C. M. Luo, B. Tan, N. Wang, Y. Zhu, G. Crameri, S. Y. Zhang, L. F. Wang, P. Daszak and Z. L. Shi, Nature, 2014, 503, 535–538 CrossRef PubMed.
- F. S. Dawood, A. D. Luliano, C. Reed, M. I. Meltzer, D. K. Shay, P. Cheng, D. Bandaranayake, R. F. Breiman, W. A. Brooks, P. Buchy, D. R. Feikin, K. B. Fowler, A. Gordon, N. T. Hien, P. Horby, Q. S. Huang, M. A. Katz, A. Krishnan, R. Lal, J. M. Montgomery, Ka. Mølbak, R. Pebody, A. M. Presanis, H. Razuri, A. Steens, Y. O. Tinoco, J. Wallinga, H. Yu, S. Vong, J. Bresee and M. Widdowson, Lancet, 2012, 12, 687–695 CrossRef.
- D. Hot, O. Legeay, J. Jacques, C. Gantzer, Y. Caudrelier, K. Guyard, M. Lange and L. Andreoletti, Water Res., 2003, 37, 4703–4710 CrossRef CAS.
- J. Hewitt, M. Leonard, G. E. Greening and G. D. Lewis, Water Res., 2011, 45, 6267–6276 CrossRef CAS PubMed.
- A. R. Petrinca, D. Donia, A. Pierangeli, R. Gabrieli, A. M. Degener, E. Bonanni, L. Diaco, G. Cecchini, P. Anastasi and M. Divizia, J. Appl. Microbiol., 2009, 106, 1608–1617 CrossRef CAS PubMed.
- K. Bibby, L. W. Casson, E. Stachler and C. N. Haas, Environ. Sci. Technol. Lett., 2015, 2, 2–6 CrossRef CAS.
- B. Cook, T. Cutts, A. Nikiforuk, P. Poliquin, D. Court, J. Strong and S. Theriault, Viruses, 2015, 7, 1975–1986 CrossRef PubMed.
- L. M. Casanova and S. R. Weaver, Environ. Sci. Technol. Lett., 2015, 2, 76–78 CrossRef CAS.
- P. G. Cantalupo, B. Calgua, G. Zhao, A. Hundesa, A. D. Wier, J. P. Katz, M. Grabe, R. W. Hendrix, R. Girones and D. Wang, mBio, 2011, 2, e00180–11 CrossRef PubMed.
- K. Bibby and J. Peccia, Environ. Sci. Technol., 2013, 47, 1945–1951 CrossRef CAS PubMed.
- R. Collier, Can. Med. Assoc. J., 2009, 180, E95–E96 CrossRef PubMed.
- A. Bosch, Int. J. Microbiol., 2010, 1, 191–196 Search PubMed.
- M. von Linstow, J. Eugen-Olsen, A. Koch, T. N. Winther, H. Westh and B. Hogh, Eur. J. Med. Res., 2006, 11, 329–335 Search PubMed.
- H. Harvala, C. L. McIntyre, N. J. McLeish, J. Kondracka, J. Palmer, P. Molyneaux, R. Gunson, S. Bennett, K. Templeton and P. Simmonds, J. Med. Virol., 2012, 84, 536–542 CrossRef CAS PubMed.
- C. Arena, J. P. Amoros, V. Vaillant, K. Balay, R. Chikhi-Brachet, L. Varesi, J. Arrighi, T. Blanchon, F. Carrat and T. Hanslik, Virol. J., 2012, 9, 116 CrossRef PubMed.
- M. Jevšnik, A. Steyer, T. Zrim, M. Pokorn, T. Mrvič, Š. Grosek, F. Strle, L. Lusa and M. Petrovec, Virol. J., 2013, 10, 46 CrossRef PubMed.
- W. K. Leung, K.-F. To, P. K. Chan, H. L. Chan, A. K. Wu, N. Lee, K. Y. Yuen and J. J. Sung, Gastroenterol., 2003, 125, 1011–1017 CrossRef PubMed.
- K. H. Chan, L. L. Poon, V. C. Cheng, Y. Guan, I. F. Hung, J. Kong, L. Y. Yam, W. H. Seto, K. Y. Yuen and J. S. Peiris, Emerging Infect. Dis., 2004, 10, 294–299 CrossRef PubMed.
- M. D. de Jong, V. C. Bach, T. Q. Phan, M. H. Vo, T. T. Tran, B. H. Nguyen, M. Beld, T. P. Le, H. K. Truong, V. V. C. Nguyen, T. H. Tran, Q. H. Do and J. Farrar, N. Engl. J. Med., 2005, 352, 686–691 CrossRef CAS PubMed.
- V. Peltola, T. Ziegler and O. Ruuskanen, Clin. Infect. Dis., 2003, 36, 299–305 CrossRef PubMed.
- T. P. Weber and N. I. Stilianakis, Am. J. Infect. Dis., 2008, 57, 361–373 Search PubMed.
- D. Lenes, N. Deboosere, F. Menard-Szczebara, J. Jossent, V. Alexandre, C. Machinal and M. Vialette, Water Res., 2010, 44, 2473–2486 CrossRef CAS PubMed.
- M. C. Chan, N. Lee, P. K. Chan, K. F. To, R. Y. Wong, W. S. Ho, K. L. Ngai and J. J. Sung, Emerging Infect. Dis., 2011, 17, 2038–2042 Search PubMed.
- M. C. Chan, N. Lee, P. K. Chan, T. F. Leung and J. J. Sung, J. Clin. Virol., 2009, 45, 208–211 CrossRef CAS PubMed.
- A. Lucio-Forster, D. D. Bowman, B. Lucio-Martínez, M. P. Labare and M. A. Butkus, Environ. Eng. Sci., 2006, 23, 897–903 CrossRef CAS.
- L. Heijnen and G. Medema, J. Water Health, 2011, 9, 434–442 CrossRef CAS PubMed.
- B. A. Pinsky, S. Mix, J. Rowe, S. Ikemoto and E. J. Baron, Emerging Infect. Dis., 2010, 16, 1165–1167 CrossRef PubMed.
- J. Nordgren, A. Matussek, A. Mattsson, L. Svensson and P. E. Lindgren, Water Res., 2009, 43, 1117–1125 CrossRef CAS PubMed.
- F. G. Masclaux, P. Hotz, D. Friedli, D. Savova-Bianchi and A. Oppliger, Water Res., 2013, 47, 5101–5109 CrossRef CAS PubMed.
- A. K. da Silva, J. C. Le Saux, S. Parnaudeau, M. Pommepuy, M. Elimelech and F. S. Le Guyader, Appl. Environ. Microbiol., 2007, 73, 7891–7897 CrossRef CAS PubMed.
- M. Kitajima, Y. Tohya, K. Matsubara, E. Haramoto, E. Utagawa and H. Katayama, Lett. Appl. Microbiol., 2010, 51, 119–121 CAS.
- A. Hata, M. Kitajima and H. Katayama, J. Appl. Microbiol., 2012, 114, 545–554 CrossRef PubMed.
- F. J. Simmons and I. Xagoraraki, Water Res., 2011, 45, 3590–3598 CrossRef CAS PubMed.
- M. Kitajima, B. C. Iker, I. L. Pepper and C. P. Gerba, Sci. Total Environ., 2014, 488, 290–296 CrossRef PubMed.
- I. A. Hamza, L. Jurzik, K. Überla and M. Wilhelm, Water Res., 2011, 45, 1358–1368 CrossRef CAS PubMed.
- T. M. Fumian, J. P. G. Leite, T. L. Rose, T. Prado and M. P. Miagostovich, Water Res., 2011, 45, 5755–5763 CrossRef CAS PubMed.
- W. J. Lodder and A. M. de Roda Husman, Appl. Environ. Microbiol., 2005, 71, 1453–1461 CrossRef CAS PubMed.
- L. M. Villar, V. S. de Paula, L. Diniz-Mendes, F. R. Guimarães, F. F. M. Ferreira, T. C. Shubo, M. P. Miagostovich, E. Lampe and A. M. C. Gaspar, Lett. Appl. Microbiol., 2007, 45, 168–173 CrossRef CAS PubMed.
- A. Carducci, P. Morici, F. Pizzi, R. Battistini, E. Rovini and M. Verani, Water Sci. Technol., 2008, 58, 893–897 CrossRef CAS PubMed.
- F. G. Masclaux, P. Hotz, D. Gashi, D. Savova-Bianchi and A. Oppliger, Environ. Res., 2014, 133, 260–265 CrossRef CAS PubMed.
- E. Haramoto, M. Kitajima and M. Otagiri, Appl. Environ. Microbiol., 2013, 79, 3529–3532 CrossRef CAS PubMed.
- J. Hewitt, G. E. Greening, M. Leonard and G. D. Lewis, Water Res., 2013, 47, 6750–6761 CrossRef CAS PubMed.
-
WHO, Review of latest available evidence on risks to human health through potential transmission of avian influenza (H5N1) through water and sewage, 2006, http://www.who.int/water_sanitation_health/emerging/h5n1background.pdf.
- J. H. Beigel, J. Farrar, A. M. Han, F. G. Hayden, R. Hyer, M. D. De Jong, S. Lochindarat, T. K. Nguyen and T. H. Nguyen, N. Engl. J. Med., 2005, 353, 1374–1385 CrossRef PubMed.
- Y. Shu, C. K. F. Li, Z. Li, R. Gao, Q. Liang, Y. Zhang, L. Dong, J. Zhou, J. Dong and D. Wang, J. Infect. Dis., 2010, 201, 1173–1177 CrossRef CAS PubMed.
- Y. Hu, S. Lu, Z. Song, W. Wang, P. Hao, J. Li, X. Zhang, H.-L. Yen, B. Shi and T. Li, Lancet, 2013, 381, 2273–2279 CrossRef.
- S. J. Yoo, S. J. Moon, E. Y. Kuak, H. M. Yoo, C. K. Kim, M. J. Chey and B. M. Shin, J. Clin. Microbiol., 2010, 48, 2314–2315 CrossRef PubMed.
- K. K. To, K.-H. Chan, I. W. Li, T.-Y. Tsang, H. Tse, J. F. Chan, I. F. Hung, S.-T. Lai, C.-W. Leung and Y.-W. Kwan, J. Med. Virol., 2010, 82, 1–7 CrossRef PubMed.
- H. Zeng, C. Pappas, J. M. Katz and T. M. Tumpey, J. Virol., 2011, 85, 686–696 CrossRef CAS PubMed.
-
World Health Organization, Avian Influenza: Assessing the Pandemic Threat, 2005, http://whqlibdoc.who.int/hq/2005/WHO_CDS_2005.29.pdf.
- K. R. McKinney, Y. Y. Gong and T. G. Lewis, J. Environ. Health, 2006, 68, 26–30 Search PubMed.
- P. K. Cheng, D. A. Wong, L. K. Tong, S.-M. Ip, A. C. Lo, C.-S. Lau, E. Y. Yeung and W. W. Lim, Lancet, 2004, 363, 1699–1700 CrossRef.
- C. M. Booth, L. M. Matukas, G. A. Tomlinson, A. R. Rachlis, D. B. Rose, H. A. Dwosh, S. L. Walmsley, T. Mazzulli, M. Avendano and P. Derkach, J. Am. Vet. Med. Assoc., 2003, 289, 2801–2809 CrossRef CAS PubMed.
- J. Peiris, C. M. Chu, V. Cheng, K. S. Chan, I. Hung, L. Poon, K. I. Law, B. Tang, T. Hon and C. S. Chan, Lancet, 2003, 361, 1767–1772 CrossRef CAS.
- C. Drosten, M. Seilmaier, V. M. Corman, W. Hartmann, G. Scheible, S. Sack, W. Guggemos, R. Kallies, D. Muth and S. Junglen, Lancet Infect. Dis., 2013, 13, 745–751 CrossRef.
- B. Guery, J. Poissy, L. el Mansouf, C. Séjourné, N. Ettahar, X. Lemaire, F. Vuotto, A. Goffard, S. Behillil, V. Enouf, V. Caro, A. Mailles, D. Che, J.-C. Manuguerra, D. Mathieu, A. Fontanet and S. Van der Werf, Lancet, 2013, 381, 2265–2272 CrossRef.
- A. Vabret, J. Dina, S. Gouarin, J. Petitjean, S. Corbet and F. Freymuth, Clin. Infect. Dis., 2006, 42, 634–639 CrossRef PubMed.
- F. Esper, Z. Ou and Y. T. Huang, J. Clin. Virol., 2010, 48, 131–133 CrossRef PubMed.
- M. Risku, S. Lappalainen, S. Räsänen and T. Vesikari, J. Clin. Virol., 2010, 48, 27–30 CrossRef PubMed.
- V. Krikelis, N. Spyrou, P. Markoulatos and C. Serie, Can. J. Microbiol., 1985, 31, 24–25 CrossRef CAS.
- T. M. Fumian, J. P. G. Leite, A. A. Castello, A. Gaggero, M. S. L. de Caillou and M. P. Miagostovich, J. Virol. Methods, 2010, 170, 42–46 CrossRef CAS PubMed.
- C.-C. Lai, Y.-H. Wang, C.-Y. Wu, C.-H. Hung, D. D.-S. Jiang and F.-T. Wu, J. Clin. Virol., 2013, 56, 96–101 CrossRef PubMed.
- M. C. Chan, J. J. Sung, R. K. Lam, P. K. Chan, N. L. Lee, R. W. Lai and W. K. Leung, Emerging Infect. Dis., 2006, 12, 1278–1280 CrossRef PubMed.
- I. Hung, V. Cheng, A. Wu, B. Tang, K. H. Chan, C. M. Chu, M. Wong, W. T. Hui, L. Poon and D. Tse, Emerging Infect. Dis., 2004, 10, 1550–1557 CrossRef CAS PubMed.
- L. L. Poon, K. H. Chan, O. K. Wong, T. K. Cheung, I. Ng, B. Zheng, W. H. Seto, K. Y. Yuen, Y. Guan and J. S. Peiris, Clin. Chem., 2004, 50, 67–72 CAS.
- J. Nazir, R. Haumacher, A. Ike, P. Stumpf, R. Böhm and R. E. Marschang, Avian Dis., 2010, 54, 720–724 CrossRef.
- H. F. Rabenau, J. Cinatl, B. Morgenstern, G. Bauer, W. Preiser and H. W. Doerr, Med. Microbiol. Immunol., 2005, 194, 1–6 CrossRef CAS PubMed.
- P. M. Gundy, C. P. Gerba and I. L. Pepper, Food Environ. Virol., 2008, 1, 10–14 CrossRef PubMed.
- L. Casanova, W. A. Rutala, D. J. Weber and M. D. Sobsey, Water Res., 2009, 43, 1893–1898 CrossRef CAS PubMed.
- J. Hardestam, M. Simon, K. O. Hedlund, A. Vaheri, J. Klingstrom and A. Lundkvist, Appl. Environ. Microbiol., 2007, 73, 2547–2551 CrossRef CAS PubMed.
- L. W. Casson, C. A. Sorber, R. H. Palmer, A. Enrico and P. Gupta, Water Environ. Res., 1992, 64, 213–215 CrossRef CAS.
- E. Biziagos, J. Passagot, J. M. Crance and R. Deloince, Appl. Environ. Microbiol., 1988, 54, 2705–2710 CAS.
-
T. Hvitved-Jacobsen, J. Vollertsen and A. H. Nielsen, Sewer Processes: Microbial and Chemical Process Engineering of Sewer Networks, CRC Press, 2013 Search PubMed.
-
G. Tchobanoglous, H. D. Stensel, R. Tsuchihashi and F. Burton, Wastewater Engineering: Treatment and Resource Recovery, Metcalf & Eddy, Inc., McGraw-Hill Science/Engineering/Math, 2013 Search PubMed.
- B. E. Moore, Appl. Environ. Microbiol., 1993, 59, 1437–1443 CAS.
- J. D. Brown, D. E. Swayne, R. J. Cooper, R. E. Burns and D. E. Stallknecht, Avian Dis., 2007, 51, 285–289 CrossRef PubMed.
- C. Lebarbenchon, S. Sreevatsan, T. Lefèvre, M. A. Ramakrishnan, J. D. Brown and D. E. Stallknecht, Proc. R. Soc. B, 2012, 279, 3967–3975 CrossRef PubMed.
- A. M. Nasser, Y. Tchorch and B. Fattal, Water Sci. Technol., 1993, 27, 401–407 Search PubMed.
- E. M. Smith, C. P. Gerba and J. L. Melnick, Appl. Environ. Microbiol., 1978, 35, 685–689 CAS.
- S. Shigematsu, A. Dublineau, O. Sawoo, C. Batéjat, T. Matsuyama and J.-C. Manuguerra, Influenza Other Respir. Viruses, 2014, 8, 123–130 CrossRef PubMed.
- N. A. Clarke, R. E. Stevenson, S. L. Chang and P. W. Kabler, Am. J. Public Health, 1961, 51, 1118–1129 CrossRef CAS PubMed.
- L. G. Irving and F. A. Smith, Appl. Environ. Microbiol., 1981, 41, 51–59 CAS.
- J. F. Malina Jr, K. R. Ranganathan, B. P. Sagik and B. E. Moore, J. - Water Pollut. Control Fed., 1975, 47, 2178–2183 CAS.
- S. W. Funderburg and C. A. Sorber, Water Res., 1985, 19, 547–555 CrossRef.
- W. A. Yanko, Water Environ. Res., 1993, 65, 221–226 CrossRef.
- S. S. Thompson, J. L. Jackson, M. Suva-Castillo, W. A. Yanko, Z. E. Jack, J. Kuo, C. L. Chen, F. P. Williams and D. P. Schnurr, Water Environ. Res., 2003, 75, 163–170 CrossRef CAS.
- M. R. Goddard, J. Bates and M. Butler, Appl. Environ. Microbiol., 1981, 42, 1023–1028 CAS.
- K. Wong, B. M. Onan and I. Xagoraraki, Appl. Environ. Microbiol., 2010, 76, 6441–6448 CrossRef CAS PubMed.
- E. Viau and J. Peccia, Appl. Environ. Microbiol., 2009, 75, 164–174 CrossRef CAS PubMed.
- A. Chetochine, M. Brusseau, C. Gerba and I. Pepper, Appl. Environ. Microbiol., 2006, 72, 665–671 CrossRef CAS PubMed.
- E. Viau, K. Bibby, T. Paez-Rubio and J. Peccia, Environ. Sci. Technol., 2011, 45, 5459–5469 CrossRef CAS PubMed.
- D. Shoham, A. Jahangir, S. Ruenphet and K. Takehara, Influenza Res. Treat., 2012, 2012, 1–11 CrossRef PubMed.
- D. E. Stallknecht, V. H. Goekjian, B. R. Wilcox, R. L. Poulson and J. D. Brown, Avian Dis., 2010, 54, 461–465 CrossRef.
- M. E. Darnell and D. R. Taylor, Transfusion, 2006, 46, 1770–1777 CrossRef PubMed.
- S. Monpoeho, A. Maul, B. Mignotte-Cadiergues, L. Schwartzbrod, S. Billaudel and V. Ferre, Appl. Environ. Microbiol., 2001, 67, 2484–2488 CrossRef CAS PubMed.
- G. D. Lewis and T. G. Metcalf, Appl. Environ. Microbiol., 1988, 54, 1983–1988 CAS.
-
J. E. Lawrence and G. F. Steward, in Manual of Aquatic Viral Ecology. ASLO, ed. S. W. Wilhelm, M. G. Weinbauer and C. A. Suttle, 2010, pp. 166–181 Search PubMed.
- X. Wang, J. Li, T. Guo, B. Zhen, Q. Kong, B. Yi, Z. LI, N. Song, M. Jin and W. Xiao, Water Sci. Technol., 2005, 52, 213–221 CAS.
- H. Amdiouni, L. Maunula, K. Hajjami, A. Faouzi, A. Soukri and J. Nourlil, Curr. Microbiol., 2012, 65, 432–437 CrossRef CAS PubMed.
- N. Deboosere, S. V. Horm, A. Pinon, J. Gachet, C. Coldefy, P. Buchy and M. Vialette, Appl. Environ. Microbiol., 2011, 77, 3802–3808 CrossRef CAS PubMed.
- R. E. Stetler, M. E. Morris and R. S. Safferman, J. Virol. Methods, 1992, 40, 67–75 CrossRef CAS.
- C. J. Hurst and T. Goyke, Appl. Environ. Microbiol., 1983, 46, 133–139 CAS.
- K. E. Gibson, K. J. Schwab, S. K. Spencer and M. A. Borchardt, Water Res., 2012, 46, 4281–4291 CrossRef CAS PubMed.
- N. E. Moore, J. Wang, J. Hewitt, D. Croucher, D. A. Williamson, S. Paine, S. Yen, G. E. Greening and R. J. Hall, J. Clin. Microbiol., 2015, 53, 15–21 CrossRef CAS PubMed.
- L. R. Holtz, S. Cao, G. Zhao, I. K. Bauer, D. M. Denno, E. J. Klein, M. Antonio, O. C. Stine, T. L. Snelling, C. D. Kirkwood and D. Wang, Virology, 2014, 468–470, 556–564 CrossRef CAS PubMed.
- R. J. Hall, J. Wang, A. K. Todd, A. B. Bissielo, S. Yen, H. Strydom, N. E. Moore, X. Ren, Q. S. Huang, P. E. Carter and M. Peacey, J. Virol. Methods, 2014, 195, 194–204 CrossRef CAS PubMed.
- J. B. Mahony, A. Petrich and M. Smieja, Crit. Rev. Clin. Lab. Sci., 2011, 48, 217–249 CrossRef CAS PubMed.
- A. Meijer, A. Beerens, E. Claas, M. Hermans, A. de Jong, R. Molenkamp, H. Niesters, P. Overduin, J. Rossen, R. Schuurman, P. Wolffs, R. Fouchier, A. Osterhaus, M. Schutten and M. Koopmans, J. Clin. Virol., 2009, 45, 179–184 CrossRef PubMed.
- Novel Swine-Origin Influenza A (H1N1) Virus Investigation Team, N. Engl. J. Med., 2009, 360, 2605–2615 CrossRef PubMed.
- J. Liu, X. Shi, R. Schwartz and G. Kemble, Vaccine, 2009, 27, 6460–6463 CrossRef CAS PubMed.
- L. Vijgen, E. Moës, E. Keyaerts, S. Li and M. Van Ranst, Methods Mol. Biol. (N. Y., NY, U. S.), 2008, 454, 3–12 CAS.
- P. C. Woo, S. K. Lau, C.-M. Chu, K.-H. Chan, H.-W. Tsoi, Y. Huang, B. H. Wong, R. W. Poon, J. J. Cai and W.-K. Luk, J. Virol., 2005, 79, 884–895 CrossRef CAS PubMed.
- D. Adachi, G. Johnson, R. Draker, M. Ayers, T. Mazzulli, P. J. Talbot and R. Tellier, J. Virol. Methods, 2004, 122, 29–36 CrossRef CAS PubMed.
- E. R. Gaunt, A. Hardie, E. C. J. Claas, P. Simmonds and K. E. Templeton, J. Clin. Microbiol., 2010, 48, 2940–2947 CrossRef CAS PubMed.
- R. K. Dare, A. M. Fry, M. Chittaganpitch, P. Sawanpanyalert, S. J. Olsen and D. D. Erdman, J. Infect. Dis., 2007, 196, 1321–1328 CrossRef CAS PubMed.
- L. van der Hoek, K. Pyrc, M. F. Jebbink, W. Vermeulen-Oost, R. J. Berkhout, K. C. Wolthers, P. M. Wertheim-van Dillen, J. Kaandorp, J. Spaargaren and B. Berkhout, Nat. Med., 2004, 10, 368–373 CrossRef CAS PubMed.
- M. L. Ng, J. Gen. Virol., 2003, 84, 3291–3303 CrossRef CAS.
|
This journal is © The Royal Society of Chemistry 2015 |