DOI:
10.1039/C3RA47116K
(Paper)
RSC Adv., 2014,
4, 8360-8364
A near-infrared fluorescent sensor for selective detection of cysteine and its application in live cell imaging†
Received
28th November 2013
, Accepted 14th January 2014
First published on 14th January 2014
Abstract
Biological thiols, including cysteine (Cys), homocysteine (Hcy) and glutathione (GSH), play important roles in maintaining the appropriate redox status of biological systems. The discrimination between them is of great importance because of their different biological roles. Herein, we present a new near-infrared (NIR) fluorescent sensor Cy-NO2 for selective detection of Cys over Hcy/GSH. The nitrothiophenol group is introduced to quench the fluorescence through photo-induced electron transfer (PET). The sensor undergoes displacement of nitrothiophenol with thiol to turn on the fluorescence. The amino groups of Cys/Hcy further replace the thiolate to form amino-substituted products, which exhibit dramatically different photophysical properties compared to the sulfur-substituted product from the reaction with GSH. By means of more rapid intramolecular displacement of sulfur with the amino group of Cys than Hcy, the discrimination of Cys is achieved. Moreover, Cy-NO2 was successfully applied for bioimaging Cys in living cells.
Introduction
Biothiols, such as cysteine (Cys), homocysteine (Hcy) and glutathione (GSH), are involved in a number of biological processes and play crucial roles in maintaining the appropriate redox status of biological systems. Their abnormal levels can cause a variety of diseases. A depressed level of Cys is associated with slowed growth, hair depigmentation, liver damage, and weakness.1 At elevated levels in plasma, Hcy is a risk factor for Alzheimer's and cardiovascular diseases.2 GSH plays a critical role in controlling oxidative stress in order to maintain the redox homeostasis for cell growth and function.3 Therefore, the detection of biothiols consistently attracts a great deal of attention for various biochemical investigations as well as the diagnosis of related diseases.
Fluorescence imaging has emerged as one powerful technique to monitor targets and biological processes in the context of a living system with high temporal and spatial resolution. When the goal is to quantitate or image thiols in vivo, technologies based on fluorescent probes are often the methodologies of choice because of their demonstrated selectivities and sensitivities.4 Significant effort has been made to develop fluorescent sensors for biothiol detection.5 Most of the existing sensors utilize the strong nucleophilicity of thiol group, which involve some specific reaction mechanisms, such as Michael addition,6 cleavage of disulphide7 and sulfonamide,8 etc. These sensors could selectively detect biothiols from other amino acids. However, discriminating among thiol-containing molecules, with their similar structures and reactivities, remains a challenge. Therefore, it has received a lot of attention to distinguish between these three thiols.5a Utilizing the cyclization of Cys/Hcy with aldehydes or acrylates, the discrimination of Cys/Hcy over GSH can be achieved.9 Recently our group has developed a ratiometric fluorescent sensor for the selective detection of GSH over Cys/Hcy.10 The chlorine of the monochlorinated boron dipyrromethene (BODIPY) can be rapidly replaced by thiolate. The amino groups of Cys/Hcy, but not of GSH further replace the thiolate to form amino-substituted BODIPY. The significantly different photophysical properties of sulfur and amino substituted BODIPY enable the discrimination of GSH over Cys/Hcy. However, most of the reported selective sensors required the detection wavelength in the visible range, in which the detection would be interfered with autofluorescence from the cell and not suitable for deep tissue imaging.
Cyanine, as a classical NIR dye, has been widely used as NIR fluorescent labels for biological application.11 They have unique advantages of tracing molecular activity in vivo because their NIR photons can penetrate relatively deeply into tissues with a low auto-fluorescence background, as well as less damage to biological samples.12 We have successfully expanded our previously reported intramolecular displacement mechanism to new chromophores, including cyanine dye.13 Herein, we develop a NIR fluorescent sensor based on cyanine dyes for the selective turn-on detection of Cys. We introduced nitrothiophenol to quench the fluorescence through photo-induced electron transfer (PET), which could be blocked by the remove of nitrothiophenol group in the presence of thiols. By means of more rapid intramolecular displacement of sulfur with amino group of Cys than Hcy and GSH, the discrimination of Cys is achieved.
Results and discussion
As shown in Scheme 1a, the reaction of IR-780 with nitrothiophenol in the presence of triethylamine yields Cy-NO2, which was characterized by 1H NMR, 13C NMR, and mass spectrometry (MALDI-TOF-MS). As we expected, the nitrothiophenol moiety with strong reduction potential incurs the PET to quench the fluorescence, resulted in the faint fluorescence. In preliminary experiments, we study the reaction of Cy-NO2 with methyl mercaptoacetate. The reaction product was identified as Cy-S, which exhibited strong fluorescence (Fig. S1 in ESI†). This result indicated that methyl mercaptoacetate substituted the nitrothiophenol group, which blocked the PET process and led to a fluorescence enhancement (Scheme 1b). We tested the time course response of Cy-NO2 to methyl mercaptoacetate under pseudo 1st reaction conditions (Fig. S2 in ESI†). The observed rate constant is found to be 1.9 × 10−2 s−1 (t 1/2 = 37 s), which indicated Cy-NO2 showed fast response to thiols.
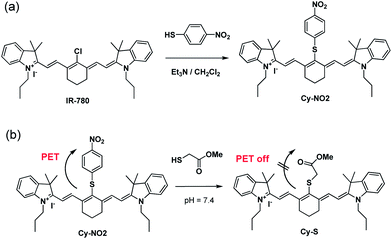 |
| Scheme 1 (a) Synthesis of Cy-NO2; (b) reaction of Cy-NO2 with thiol. | |
We measured the time-dependent fluorescence response of Cy-NO2 in the presence of Cys in DMSO–HEPES buffer (1
:
4, v/v, 20 mM, pH 7.4) at 37 °C. As shown in Fig. 1, free Cy-NO2 exhibited weak fluorescence centered at 830 nm. Upon addition of Cys, a new emission band centered at 750 nm emerged and the emission intensity increased gradually, suggesting nitrothiophenol group was replaced and turned off the PET quenching. We tested the fluorescence responses of Cy-NO2 as function of concentrations of Cys (Fig. S3 in ESI†). A marked fluorescence enhancement with the increasing concentration of Cys was observed. The emission intensity of 750 nm showed a good linear relationship with Cys concentrations (Fig. 2). The detection limit was determined to be 1.26 × 10−6 M (S/N = 3).
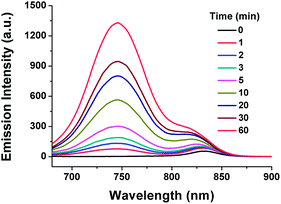 |
| Fig. 1 Time-dependent emission spectra of Cy-NO2 (10 μM) in the presence of 100 equiv. of Cys in DMSO–HEPES buffer (1 : 4, v/v, 20 mM, pH 7.4) at 37 °C. λex = 650 nm. | |
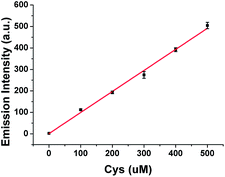 |
| Fig. 2 Emission intensity of Cy-NO2 (10 μM) at 750 nm as a function of the concentration of Cys in DMSO–HEPES buffer (1 : 4, v/v, 20 mM, pH 7.4) at 37 °C. Each spectrum was recorded 60 min after addition. λex = 650 nm. | |
We also measured the absorption and fluorescence changes of Cy-NO2 in the present of GSH and Hcy (Fig. 3, Fig. S4 in ESI†). Upon addition of GSH, the fluorescence increased at 830 nm, which is in agreement with the emission of Cy-S. In the case of Hcy, a dual emission at both 745 nm and 820 nm was observed. To validate the reaction mechanism of Cy-NO2 with the three thiols, Cy-N was also synthesized by reacting Cy-NO2 with butylamine, which showed similar emission properties with Cy–Cys (Fig. S5 in ESI†). Therefore, the emission at about 750 nm and 830 nm were corresponding to the amino- and sulfur-substituted cyanine, respectively. It revealed that only sulfur-substituted product was formed in the presence of GSH. In the case of Hcy, the amino-substituted product could be observed, however, at much slower reaction rate compared to Cys. Fig. 3 showed that Cy-NO2 could detect Cys over GSH/Hcy.
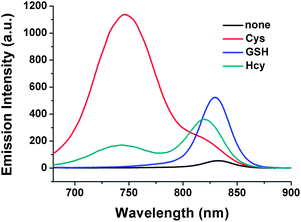 |
| Fig. 3 Emission spectra of Cy-NO2 (10 μM) in the presence of 100 equiv. of Cys, Hcy and GSH in DMSO–HEPES buffer (1 : 4, v/v, 20 mM, pH 7.4) at 37 °C. Each spectrum was recorded 60 min after addition. λex = 650 nm. | |
Control experiments were performed in order to demonstrate the reaction mechanism.14 The reaction of Cy-NO2 with 2-mercaptoethylamine (MEA), which has similar structure with Cys but lacks the carboxyl group, resulted in the amino-substituted product. By contrast, in the reaction of Cy-NO2 with N-acetylcysteine which lacks an amino group, only the formation of the sulfur-substituted product was observed (Fig. S5 in ESI†). In addition, the reaction of Cy-NO2 with amino acids without sulfhydryl groups (e.g., Lys and Arg) was not observed because of the protonation of amino group under physiological conditions (pH 7.4). It revealed that both sulfur and adjacent amino groups are necessary for the formation of amino-substituted product, which is accordance with the two-step reaction in our previous work.10,13,15
On the basis of above observations and our previous work,10,13,14 we proposed the reaction mechanism of Cy-NO2 in the presence of Cys, Hcy and GSH (Scheme 2). At physiological conditions, deprotonation of the thiol yields active nucleophile, thiolate. The nitrothiophenyl group of Cy-NO2 is replaced by thiolate to generate the kinetic sulfur-substituted product. For Cys and Hcy, the primary amine allows further intramolecular displacement of sulfur by five- or six-membered cyclic transition state to yield the thermodynamic amino-substituted product. As we known, five-membered ring is the preferred conformation, which can explain the faster reaction rate in the presence of Cys rather than Hcy. For GSH, no favored transition state could be formed due to the unavailable adjacent amine group. Thus, the sulfur-substituted product is stable and maintains its structure.
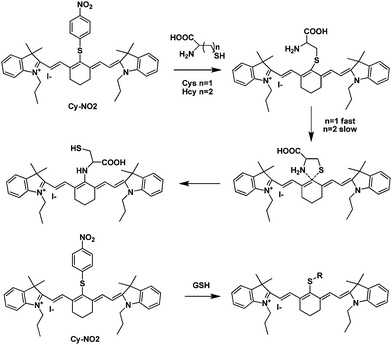 |
| Scheme 2 Proposed mechanism for the reaction of Cy-NO2 with Cys, Hcy and GSH. | |
The selectivity of Cy-NO2 was testified in the presence of other physiologically relevant amino acids (e.g., Gly, Ala, Val, Leu, Ile, Phe, Trp, Tyr, Asp, His and Asn) at identical conditions. As shown in Fig. 4, no visible changes in the spectra were observed upon the addition of 100 equiv. of other amino acids. The results demonstrated that Cy-NO2 showed high selectivity towards Cys over Hcy/Hcy and other amino acids.
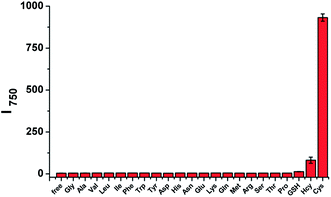 |
| Fig. 4 Emission responses at 750 nm of Cy-NO2 (10 μM) upon addition of 100 equiv. of various amino acids and GSH. Each data were acquired 60 min after addition of different amino acids in DMSO–HEPES buffer (1 : 4, v/v, 20 mM, pH 7.4) at 37 °C. | |
To access the capability of Cy-NO2 for Cys detection in living cells, the confocal imaging was studied (Fig. 5). HeLa cells were pretreated with 500 μM Cys for 15 min and then incubated with Cy-NO2 (10 μM) for 60 min, strong fluorescence was observed. A control experiment was performed by removing intracellular thiols using 1 mM N-methylmaleimide (NEM, a thiol-blocking reagent) for 15 min before incubation of the cells with Cy-NO2 (10 μM) for 60 min, leading to almost no fluorescence. The result exhibits that Cy-NO2 can show turn-on response to Cys in living cells. In vitro cytotoxicity of Cy-NO2 was valuated with HeLa cells using CCK8 assay.16 No obvious adverse effect of Cy-NO2 was found on HeLa cells viability (Fig. S6†).
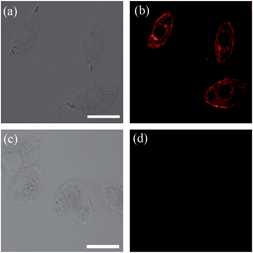 |
| Fig. 5 Confocal fluorescence and bright-field images of living HeLa cells: (a) bright field and (b) fluorescence images of HeLa cells pretreated with 500 μM Cys for 15 min and then incubated with Cy-NO2 (10 μM) for 60 min; (c) bright field and (d) fluorescence images of HeLa cells pretreated with 1 mM NEM for 15 min and then incubated with Cy-NO2 (10 μM) for 60 min. Scale bar = 20 μm. | |
Conclusions
In summary, we have developed a new NIR fluorescent sensor Cy-NO2 for selective detection of Cys in living cells. Cy-NO2 is non-fluorescent due to the PET from the nitro group. The nitrothiophenol group can be substituted by thiolate and result in turn-on fluorescence. The discrimination of Cys from Hcy and GSH is achieved through more rapid intramolecular displacement of sulfur with amino group of Cys rather than that of Hcy and GSH. Furthermore, Cy-NO2 has been successfully used to image Cys in living cells.
Experimental section
Materials and instruments
IR-780 iodide were purchased from Alfa Aesar. 4-Nitrothiophenol were purchased from Aladdin Reagant. The amino acids and GSH were purchased from Sigma-Aldrich. Other reagents were purchased from commercial suppliers and used without further purifications. 1H NMR spectra were recorded on an Advance Bruker 400M spectrometer and referenced to solvent signals. Mass spectra were obtained on Bruker Apex IV Fourier Transform Mass Spectrometer. Fluorescence spectra were determined on a Hitachi 4500 spectrophotometer. Absorption spectra were determined on a Shimadzu UV-1601PC UV-Visible spectrophotometer.
Spectral measurements
Cy-NO2 was prepared as a 1 mM stock solution in DMSO. Accurately weighted amount of Cys were dissolved in water to obtain the stock solutions (1–50 mM). The amino acids (Cys, Hcy, Gly, Ala, Val, Leu, Ile, Phe, Trp, Tyr, Asp, His, Asn, Gln, Met, Arg, Ser, Thr and Pro), GSH, MEA, N-acetylcysteine stock solution were prepared in water. The Cy-NO2 stock solution (20 μL) and the analyte stock solution (<10 μL) were added to solvent mixture of DMSO (380 μL) and HEPES buffer (1.6 mL, 20 mM, pH 7.4) to obtain according Cy-NO2 (10 μM) in DMSO–HEPES buffer (1
:
4, v/v, 20 mM, pH 7.4).
Synthesis of Cy-NO2
IR-780 iodide (100 mg, 0.15 mmol) was dissolved in dichloromethane (20 mL) and 4-nitrothiophenol (55 mg, 0.3 mmol) and one drop of Et3N was added. The mixture was stirred at room temperature for 2 h, and the solvent was evaporated under reduced pressure. The crude residue was purified by column chromatography over silica (methanol/dichloromethane = 1/20 as eluent) to give Cy-NO2 (65 mg, 63%). 1H NMR (CDCl3, 400 MHz): δ 8.52 (d, J = 14.4 Hz, 2H), 8.12 (d, J = 8.8 Hz, 2H), 7.34–7.39 (m, 4H), 7.30 (t, J = 6.4 Hz, 2H), 7.22 (t, J = 7.2 Hz, 2H), 7.14 (d, J = 7.6 Hz, 2H), 6.37 (d, J = 14.4 Hz, 2H), 4.20 (t, J = 7.2 Hz, 4H), 2.86 (t, J = 5.6 Hz, 4H), 2.09 (t, J = 5.6 Hz, 2H), 1.85–1.94 (m, 4H), 1.46 (s, 12H), 1.06 (t, J = 7.2 Hz, 6H). 13C NMR (100 MHz,CDCl3): 172.6, 147.0, 146.9, 145.4, 145.0, 142.3, 141.1, 134.2, 128.8, 125.9, 125.5, 124.6, 122.2, 111.1, 102.4, 49.2, 46.3, 27.9, 26.7, 21.0, 20.8, 11.6. MALDI-TOF-MS: calculated for [C42H48N3O2S] 658.4, found 658.3.
Cell culture and fluorescence imaging
HeLa cells were pretreated with 500 μM Cys for 15 min, washed three times with phosphate buffered saline (PBS), and incubated with 10 μM Cy-NO2 at 37 °C under 5% CO2 for 60 min, then were washed three times with PBS. For the control experiment, the cells were treated with 1 mM NEM in culture media for 15 min at 37 °C in a humidified incubator. After washing with PBS, the cells were further incubated with 10 μM of Cy-NO2 in culture media for 60 min. Fluorescent images were acquired on Olympus FV1000 confocal laser-scanning microscope 100 × oil-immersion objective lens. Fluorescence was excited at 635 nm and emission was collected at 700–800 nm.
In vitro cytotoxicity
HeLa cells were maintained in DMEM, supplemented with 10% FBS, penicillin (100 units per mL), and streptomycin (100 μg mL−1). Cells (2 × 103) were seeded in 96-well plates and incubated overnight to allow the cells to attach to the surface of the wells. The cells were then exposed to Cy-NO2 solutions with various concentrations and incubated at 37 °C in a humidified atmosphere of 5% CO2 for 24 h. After that, cells were washed with phosphate buffer and stained with CCK8. The absorbance was measured at 450 nm using an EnSpire® Multimode Plate Reader (PerkinElmer, U.S.A.). The data represented the means of triplicate measurements.
Acknowledgements
This work was financially supported by the 973 Program (2013CB933800), the National Science Foundation of China (Grant 21102155, 21222210 and 91027041) and the Chinese Academy of Sciences (100 talents program). We thank Prof. P. Wang (TIPC, CAS) for providing HeLa cells and Dr M. Wu for help in the cytotoxicity test.
Notes and references
-
(a) S. Shahrokhian, Anal. Chem., 2001, 73, 5972 CrossRef CAS;
(b) S. A. Lipton, Y. B. Choi, H. Takahashi, D. X. Zhang, W. Z. Li, A. Godzik and L. A. Bankston, Trends Neurosci., 2002, 25, 474 CrossRef CAS;
(c) E. Weerapana, C. Wang, G. M. Simon, F. Richter, S. Khare, M. B. D. Dillon, D. A. Bachovchin, K. Mowen, D. Baker and B. F. Cravatt, Nature, 2010, 468, 790 CrossRef CAS PubMed.
-
(a) S. Seshadri, A. Beiser, J. Selhub, P. F. Jacques, I. H. Rosenberg, R. B. D'Agostino, P. W. F. Wilson and P. A. Wolf, N. Engl. J. Med., 2002, 346, 476 CrossRef CAS PubMed;
(b) S. Seshadr, J. Alzheimer's Dis., 2006, 9, 393 Search PubMed.
-
(a) H. J. Forman, H. Q. Zhang and A. Rinna, Mol. Aspects Med., 2009, 30, 1 CrossRef CAS PubMed;
(b) T. P. Dalton, H. G. Shertzer and A. Puga, Annu. Rev. Pharmacol. Toxicol., 1999, 39, 67 CrossRef CAS PubMed.
-
(a) Y. Yang, Q. Zhao, W. Feng and F. Li, Chem. Rev., 2013, 113, 192 CrossRef CAS PubMed;
(b) J. Du, M. Hu, J. Fan and X. Peng, Chem. Soc. Rev., 2012, 41, 4511 RSC.
-
(a) H. S. Jung, X. Chen, J. S. Kim and J. Yoon, Chem. Soc. Rev., 2013, 42, 6019 RSC;
(b) H. Peng, W. Chen, Y. Cheng, L. Hakuna, R. Strongin and B. Wang, Sensors., 2012, 12, 15907 CrossRef CAS PubMed;
(c) X. Chen, Y. Zhou, X. Peng and J. Yoon, Chem. Soc. Rev., 2010, 39, 2120 RSC.
-
(a) C. Yin, F. Huo, J. Zhang, R. Martinez-Manez, Y. Yang, H. Lv and S. Li, Chem. Soc. Rev., 2013, 42, 6032 RSC;
(b) D. Kand, A. M. Kalle, S. J. Varma and P. Talukdar, Chem. Commun., 2012, 48, 2722 RSC;
(c) Y. Sun, M. Chen, J. Liu, X. Lv, J. Li and W. Guo, Chem. Commun., 2011, 47, 11029 RSC;
(d) L. Yi, H. Li, L. Sun, L. Liu, C. Zhang and Z. Xi, Angew. Chem., Int. Ed., 2009, 48, 4034 CrossRef CAS PubMed;
(e) S. Lim, S. Lee, S. Park and H. Kim, Tetrahedron Lett., 2011, 52, 3902 CrossRef CAS PubMed;
(f) S. Girouard, M. H. Houle, A. Grandbois, J. Keillor and S. W. Michnick, J. Am. Chem. Soc., 2005, 127, 559 CrossRef CAS PubMed.
-
(a) M. H. Lee, J. H. Han, P.-S. Kwon, S. Bhuniya, J. Y. Kim, J. L. Sessler, C. Kang and J. S. Kim, J. Am. Chem. Soc., 2012, 134, 1316 CrossRef CAS PubMed;
(b) C. S. Lim, G. Masanta, H. J. Kim, J. H. Han, H. M. Kim and B. R. Cho, J. Am. Chem. Soc., 2011, 133, 11132 CrossRef CAS PubMed;
(c) X. Cao, W. Lin and Q. Yu, J. Org. Chem., 2011, 76, 7423 CrossRef CAS PubMed;
(d) C. Ding, H. Li, X. Li and S. Zhang, Chem. Commun., 2010, 46, 7990 RSC.
-
(a) L. Yuan, W. Lin, S. Zhao, W. Gao, B. Chen, L. He and S. Zhu, J. Am. Chem. Soc., 2012, 134, 13510 CrossRef CAS PubMed;
(b) J. Shao, H. Sun, H. Guo, S. Ji, J. Zhao, W. Wu, X. Yuan, C. Zhang and T. D. James, Chem. Sci., 2012, 3, 1049 RSC;
(c) X.-D. Jiang, J. Zhang, X. Shaoa and W. Zhao, Org. Biomol. Chem., 2012, 10, 1966 RSC;
(d) X. Li, S. Qian, Q. He, B. Yang, J. Li and Y. Hu, Org. Biomol. Chem., 2010, 8, 3627 RSC.
-
(a) P. Wang, J. Liu, X. Lv, Y. Liu, Y. Zhao and W. Guo, Org. Lett., 2012, 14, 520 CrossRef CAS PubMed;
(b) H. Wang, G. Zhou, H. Gai and X. Chen, Chem. Commun., 2012, 48, 8341 RSC;
(c) P. Das, A. K. Mandal, N. B. Chandar, M. Baidya, H. B. Bhatt, B. Ganguly, S. K. Ghosh and A. Das, Chem. – Eur. J., 2012, 18, 15382 CrossRef CAS PubMed;
(d) Z. Guo, S. Nam, S. Park and J. Yoon, Chem. Sci., 2012, 3, 2760 RSC;
(e) H. Li, J. Fan, J. Wang, M. Tian, J. Du, S. Sun, P. Sun and X. Peng, Chem. Commun., 2009, 5904 RSC;
(f) X. Yang, Y. Guo and R. M. Strongin, Angew. Chem., Int. Ed., 2011, 50, 10690 CrossRef CAS PubMed;
(g) O. Rusin, N. N. St. Luce, R. A. Agbaria, J. O. Escobedo, S. Jiang, I. M. Warner, F. B. Dawan, K. Lian and R. M. Strongin, J. Am. Chem. Soc., 2004, 126, 438 CrossRef CAS PubMed.
- L.-Y. Niu, Y.-S. Guan, Y.-Z. Chen, L.-Z. Wu, C.-H. Tung and Q.-Z. Yang, J. Am. Chem. Soc., 2012, 134, 18928 CrossRef CAS PubMed.
- L. Yuan, W. Lin, K. Zheng, L. He and W. Huang, Chem. Soc. Rev., 2013, 42, 622 RSC.
-
(a) F. Yu, P. Li, G. Li, G.-J. Zhao, T.-S. Chu and K.-L. Han, J. Am. Chem. Soc., 2011, 133, 11030 CrossRef CAS PubMed;
(b) F. Kong, R. Liu, R. Chu, X. Wang, K. Xu and B. Tang, Chem. Commun., 2013, 49, 9176 RSC;
(c) K. Xu, S. Sun, J. Li, L. Li, M. Qiang and B. Tang, Chem. Commun., 2012, 48, 684 RSC;
(d) N. Karton-Lifshin, E. Segal, L. Omer, M. Portnoy, R. Satchi-Fainaro and D. Shabat, J. Am. Chem. Soc., 2011, 133, 10960 CrossRef CAS PubMed;
(e) K. Kundu, S. F. Knight, N. Willett, S. Lee, W. R. Taylor and N. Murthy, Angew. Chem., Int. Ed., 2009, 48, 299 CrossRef CAS PubMed;
(f) W. Hao, A. McBride, S. McBride, J. P. Gao and Z. Y. Wang, J. Mater. Chem., 2011, 21, 1040–1048 RSC;
(g) L. Shang, J. Yin, J. Li, L. Jin and S. Dong, Biosens. Bioelectron., 2009, 25, 269–274 CrossRef CAS PubMed.
- L.-Y. Niu, H.-R. Zheng, Y.-Z. Chen, L.-Z. Wu, C.-H. Tung and Q.-Z. Yang, Analyst 10.1039/C3AN01849K.
- At first, we considered following the reaction process of Cy-NO2 with GSH/Cys/Hcy by HPLC, but our effort has been stymied by the extreme difficulty isolating and purifying the reactive intermediates.
- L.-Y. Niu, Y.-S. Guan, Y.-Z. Chen, L.-Z. Wu, C.-H. Tung and Q.-Z. Yang, Chem. Commun., 2013, 49, 1294 RSC.
- M. Wu, J. Shi, D. Fan, Q. Zhou, F. Wang, Z. Niu and Y. Huang, Biomacromolecules, 2013, 14, 4032 CrossRef CAS PubMed.
Footnote |
† Electronic supplementary information (ESI) available: Synthetic details characterizations of compounds Cy-S, Cy-N; time course of the response of Cy-NO2 to methyl mercaptoacetate; fluorescence responses of Cy-NO2 to different concentrations of Cys; absorption spectra of Cy-NO2 in the presence of Cys, Hcy and GSH; In vitro cytotoxicity test of Cy-NO2. See DOI: 10.1039/c3ra47116k |
|
This journal is © The Royal Society of Chemistry 2014 |
Click here to see how this site uses Cookies. View our privacy policy here.