DOI:
10.1039/D3QM01190A
(Research Article)
Mater. Chem. Front., 2024,
8, 1611-1618
Robust imidazole-linked Ni-phthalocyanine-based covalent-organic framework for CO2 electroreduction in the full pH range†
Received
8th November 2023
, Accepted 27th January 2024
First published on 30th January 2024
Abstract
The electroreduction of CO2 to value-added chemicals is a promising approach to utilize CO2 and mitigate greenhouse gas emission. Covalent organic frameworks (COFs) with abundant accessible active sites, tunable pore size, and large CO2 adsorption capacity are considered promising electrocatalysts for CO2 conversion. However, most COFs linked by reversible covalent bonds exhibit poor stability, which limits their application for CO2 electroreduction reactions in acidic or alkaline electrolytes. Herein, a Ni-phthalocyanine-based COF linked by stable imidazole building blocks, named NiPc-Im-COF, was synthesized through the condensation reaction of 2,3,9,10,16,17,23,24-octa-aminophthalocyaninato Ni(II) and 4,4′-biphenyl dialdehyde. The obtained NiPc-Im-COF exhibits high chemical stability after soaking in concentrated HCl and KOH. When applied for the electroreduction of CO2, the NiPc-Im-COF exhibits high CO selectivity (>90%) in electrolytes with different pH values. Specifically, the NiPc-Im-COF shows a high CO partial current density of 267 mA cm−2 with a CO selectivity of 90% at −0.8 V vs. the reversible hydrogen electrode (RHE) in 5 M KOH solution, and meanwhile, over 90% of FECO and 6 hours of stability at −1.3 V in 0.5 M K2SO4 (pH = 1). The XRD patterns prove that the structure of the NiPc-Im-COF is not destroyed after CO2 electroreduction at different pH values. This work presents a strategy to improve the stability of COFs via irreversible covalent linkage and offers an efficient CO2RR in the full pH range, which paves a new pathway for the industrial application of COFs in CO2 electroreduction.
Introduction
Today, with the excessive use of fossil fuels, the rapid increase in the content of CO2 in the atmosphere has led to a series of problems, including global warming and rising sea levels.1–3 Various approaches, including photocatalysis, electrocatalysis, and thermal catalysis, have been explored to address this challenge.4–10 Among these methods, the electrocatalytic reduction of CO2 to fossil fuels driven by renewable energy could realize the recycling of carbon resources.11,12 Nowadays, many kinds of electrocatalysts, including metal-based catalysts (Sn-, Ag-, and Cu-based catalysts); Fe-, Co-, Ni-based single-atom catalysts; and metal-free catalysts, were employed to promote the CO2 electroreduction reaction (CO2RR) and produce diverse chemicals and fuels, including CO, HCOOH, CH4, and C2H4.13–23 However, many problems remain to be solved in the CO2RR, such as ambiguous active centers led by complex composition, low stability due to reconstruction of catalysts, low selectivity resulting from a multi-electron transfer process, and the competitive hydrogen evolution reaction (HER).24–29 Hence, it is necessary to design highly efficient electrocatalysts with clear active centers. In addition, as is well known, to achieve highly efficient CO2RR performance with high current density, alkaline and/or acidic electrolytes are usually required. Under alkaline conditions, CO2 molecules readily react with OH− and convert into carbonate/bicarbonate, which results in a low utilization of CO2. Simultaneously, the generated carbonate/bicarbonate will block the pores of the electrode, reduce CO2RR stability, and lead to the leakage of the electrolyte.30 Although these problems can be avoided while using acidic electrolytes, the HER usually occurs predominantly under such conditions and far exceeds the CO2RR. Therefore, it is important to develop and prepare stable electrocatalysts under such harsh conditions for the CO2RR.
Recently, porous crystalline covalent-organic frameworks (COFs) have garnered extensive interest owing to clear and accessible active centers and diverse adjustable chemical and pore structures, which have propelled them into the spotlight within the field of electrocatalysis.31–35 However, to obtain crystalline structures, most COFs are constructed by dynamically reversible covalent bonds, such as C
N imine bonds. These unstable bonds usually lead to the decomposition of the crystalline framework during the CO2RR process under harsh acid or alkaline conditions.36–40 In contrast, the construction of COFs with irreversible covalent bonds could effectively enhance their stability under acid or alkaline conditions.41–43 Ni phthalocyanines with active sites have been shown to promote electrocatalytic CO2RR. However, most Ni phthalocyanine-based COFs are connected with dynamic bonds such as C
N and B–O. It is still challenging to construct crystalline robust metal phthalocyanine-based COFs with irreversible covalent bonds for efficient electrocatalytic CO2RR in gas diffusion electrodes (GDE) with acid and alkaline electrolytes. Recently, nitrogen heterocyclic units were used to construct stable COFs due to the excellent chemical stability of nitrogen heterocycles.44,45 Herein, a Ni-phthalocyanine-based COF linked by the stable imidazole building blocks (NiPc-Im-COF) is synthesized, and the as-prepared NiPc-Im-COF exhibited high selectivity of CO (>90%) from −0.5 V to −0.8 V vs. reversible hydrogen electrode (RHE) and the largest CO partial current density (JCO) of 267 mA cm−2 at −0.8 V when 5 M KOH was employed as the electrolyte in GDE. Besides, the faradaic efficiency of CO (FECO) reached 90%, and the JCO arrived at 87 mA cm−2 at −1.4 V under the conditions of potassium sulfate aqueous solution (pH = 1). The results clearly indicate the significant potential of the stable COF connected via irreversible covalent bonds for the electrocatalytic CO2RR.
Results and discussion
As depicted in Fig. 1a, NiPc-Im-COF was synthesized by the reaction of 2,3,9,10,16,17,23,24-octa-aminophthalocyaninato nickel(II) (NiPc-(NH2)8) and 4,4′-biphenyl dialdehyde (BPDH) in the mixed solvent of N-methyl pyrrolidone (NMP) and mesitylene (MT). The powder X-ray diffraction (PXRD) pattern of NiPc-Im-COF, as shown in Fig. 1b, is in agreement with the simulated profile, suggesting the formation of crystalline NiPc-Im-COF most likely with an AA stacking model (Fig. 1a). Three main peaks located at 3.55°, 7.03°, and 10.6°, could be attributed to the (100), (200), and (300) crystallographic planes, respectively. The peak at 26.6° corresponding to the (001) facet is indicative of the π–π stacking between COF layers. Notably, the main diffraction peak of NiPc-(NH2)8 in the PXRD pattern was located at 5° (Fig. S1, ESI†), which was distinct from that of NiPc-Im-COF. As demonstrated by Material Studio 7.0, the NiPc-Im-COF possessed a unit cell with dimensions a = b = 25.2 Å and an interplane distance of c = 3.35 Å, in agreement with the experimental and the resulting XRD pattern (the Rp is 1.51% and the Rwp is 1.64%). To provide additional validation for the successful synthesis of the COF network structure, Fourier transform infrared spectroscopy (FT-IR), and solid-state 13C nuclear magnetic resonance (13C NMR) spectra were performed. The FT-IR spectra (Fig. S2, ESI†) revealed the band at 1688 cm−1 attributed to the stretching vibration of the aldehyde-based C
O in BPDH, and the bands at 3220 cm−1 and 3334 cm−1, signifying the vibration of –NH2 in NiPc(NH2)8, disappeared after the reaction. Simultaneously, new bands corresponding to the C–H vibration of benzene rings emerged at 2852 cm−1 and 2921 cm−1.46,47 Besides, as illustrated in Fig. 1c, the solid-state 13C NMR spectra of NiPc-Im-COF exhibited a special chemical shift peak at 153 ppm, which was ascribed to the C of the NH–C
N in the imidazole ring in the framework.48 Besides, the ultraviolet-visible (UV-vis) spectra substantiate a red shift in the Q band for NiPc-Im-COF, which suggested the extended conjugation within NiPc-Im-COF (Fig. S3, ESI†). These aforementioned results indicated the successful synthesis of NiPc-Im-COF.
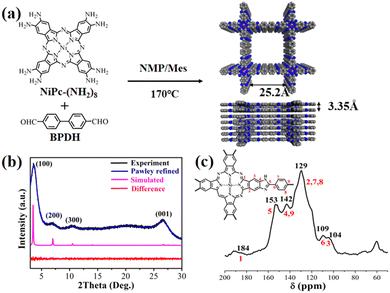 |
| Fig. 1 (a) Schematic illustration for the synthesis of the NiPc-Im-COF, with top and side views of AA stacking. (b) Simulated and experimental NiPc-Im-COF PXRD patterns. (c) Solid-state 13C NMR spectrum. | |
The scanning electron microscopy (SEM) image (Fig. 2a and Fig. S4a, ESI†) revealed that the bulk NiPc-Im-COF comprised stacked layers of nanosheets, aligning with the simulated structure. As shown in Fig. 2b and Fig. S4b, ESI,† the transmission electron microscopy (TEM) image revealed its porous structure. Additionally, high-angle annular dark-field scanning transmission electron microscopy (HAADF-STEM) and the energy-dispersive X-ray spectroscopy (EDS) elemental mapping images (Fig. 2c) illustrated the uniform distribution of C, N, and Ni elements within the NiPc-Im-COF framework, with no discernible Ni nanoparticles (Ni NPs) in TEM images, aligning with PXRD findings. At the same time, atomic force microscopy images also confirmed a sheet-like structure of NiPc-Im-COF with a height of 1.4 nm (Fig. S5, ESI†). The inductively coupled plasma (ICP) optical emission spectrometry demonstrated the Ni content of 3.75 wt% in NiPc-Im-COF, which was consistent with the result of EDS (Tables S1 and S2, ESI†). The porous structure of NiPc-Im-COF was investigated by N2 adsorption–desorption experiments at 77 K. As shown in Fig. 2d and Table S3, ESI,† NiPc-Im-COF exhibited a high Brunauer–Emmer Teller (BET) surface area of 360 m2 g−1 and a total pore volume of 0.32 cm3 g−1. Furthermore, benefiting from the affinity of the imidazole ring for CO2, NiPc-Im-COF displayed an impressive CO2 uptake of 27 cm3 g−1 at 298 K (Fig. 2e), highlighting its favorable CO2 affinity, which is beneficial for enhancing the catalytic performance of CO2RR. To evaluate the stability of NiPc-Im-COF, the NiPc-Im-COF powders were immersed in various solvents and solutions (such as N,N-dimethylformamide (DMF), ethanol (EtOH), tetrahydrofuran (THF), trichloromethane (TM) and acid/base such as concentrated HCl (1 M and 5 M), concentrated KOH (1 M and 5 M)) at room temperature for one week. As demonstrated in Fig. S6, ESI,† the PXRD patterns indicated that NiPc-Im-COF exhibited excellent acid/base and organic solvent stability. Besides, NiPc-Im-COF retained its crystallinity after soaking in THF, TM at 60 °C and DMF at 80 °C for 12 h (Fig. S7, ESI†). Additionally, the thermal stability of NiPc-Im-COF was substantiated by thermogravimetric analysis (TGA), revealing a decomposition temperature exceeding 320 °C (Fig. S8, ESI†).
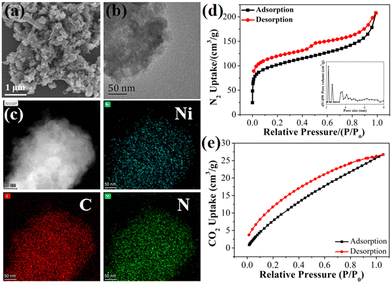 |
| Fig. 2 (a) SEM and (b) TEM images of the NiPc-Im-COF. (c) HAADF-STEM image of the NiPc-Im-COF and elemental mapping images showing the distribution of C, N, and Ni. (d) N2 sorption curves for the samples at 77 K. (e) CO2 adsorption curves for the samples at 298 K. | |
X-ray photoelectron spectroscopy (XPS) survey spectrum revealed the presence of C 1s, N 1s, and Ni 2p core levels in NiPc-Im-COF (Fig. S9a, ESI†). The resonance peak of O in the spectrum might come from guest molecules in the pore or the unreacted aldehyde groups at the edge of the framework, explaining the total atomic mass fraction being less than 100%. In the high-resolution C 1s spectrum (Fig. S9b, ESI†), two prominent peaks were located at 284.8 eV and 285.97 eV, corresponding to C
C/C–C and C
N, respectively.49–51 As shown in Fig. S9c, ESI,† the high-resolution N 1s spectrum displayed peaks at 398.6 eV and 400.16 eV. The peak at 398.6 eV was assigned to the N
C–N bond. Notably, the binding energy is at 400.16 eV, higher than that of the Ni–N bond (399.8 eV) but lower than that of the N–H bond (400.5 eV) in the imidazole ring, suggesting an interaction of Ni–N and N–H.52–54 Moreover, the Ni 2p XPS spectrum in Fig. 3a displays two sets of peaks with binding energies of 872.7 eV and 855.4 eV for Ni 2p1/2 and Ni 2p3/2, respectively, which indicates the presence of Ni(II) species and the absence of Ni nanoparticles.55–57 X-ray absorption spectroscopy (XAS) was performed to determine the coordination environment and electronic structure of Ni sites. As illustrated in Fig. 3b, the X-ray absorption near edge structure (XANES) exhibited nearly identical curves for NiPc-Im-COF and NiPc, indicating minimal alterations in the coordination environment and electronic structure of Ni sites. In addition, the peaks at 8334 eV and 8340 eV were detected in NiPc and NiPc-Im-COF, which could be attributed to the dipole forbidden 1s to 3d transition and the shakedown satellite 1s to 4pz transition.58,59 The XANES curves of NiPc-Im-COF and NiPc closely resembled that of NiO. These results indicated that the valence of Ni sites in NiPc-Im-COF is approximately +2, aligning with the results of XPS. The coordination environment of the Ni sites in the NiPc-Im-COF was analyzed by extended X-ray absorption fine structure (EXAFS). As depicted in Fig. 3c, compared with Ni foil, there was no evidence of a Ni–Ni bond at 2.2 Å in NiPc-Im-COF, indicating that Ni atoms in NiPc-Im-COF existed as single Ni sites, with no presence of Ni NPs. Besides, in comparison to NiO, NiPc and NiPc-Im-COF exhibited a prominent peak at 1.4 Å, indicative of the Ni–N bond, while NiO featured a primary peak at 1.6 Å, corresponding to the Ni–O bond. These results suggest the absence of nickel oxides within NiPc-Im-COF. The EXAFS fitting was carried out to obtain the quantitative structural parameters of Ni sites in NiPc-Im-COF. As shown in Fig. 3d and Table S4, ESI,† the fitting results of NiPc-Im-COF indicated that the average coordination number of Ni–N was 4.2, further demonstrating its Ni–N4 coordination structure.
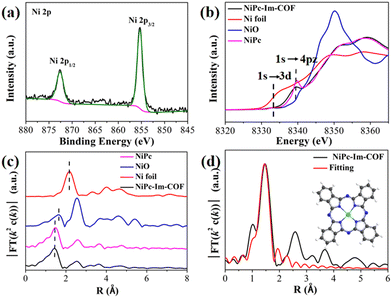 |
| Fig. 3 (a) XPS spectra of Ni in Im-COFs; (b) the normalized Ni K-edge XANES spectra of Im-COFs, NiPc, Ni foil, and NiO; (c) Fourier transform EXAFS spectra of different samples; (d) first-shell fitting of the Fourier transformed EXAFS spectrum of Im-COFs. | |
To evaluate the CO2RR performances of NiPc-Im-COF, the ink comprising NiPc-Im-COF and conductive carbon was drop-casted on 1 cm2 carbon paper electrode. The electrochemical tests were firstly conducted in CO2-saturated 0.5 M KHCO3 aqueous solution within a standard H-type electrochemical cell containing a two-chamber and three-electrode separated by a proton exchange membrane. Fig. 4a illustrates the linear sweep voltammetry (LSV) curves of NiPc-Im-COF, demonstrating a notably higher current density than that of conducted in the corresponding N2-saturated electrolyte. This discrepancy suggested the CO2RR has occurred over NiPc-Im-COF. Besides, the LSV curve of NiPc-Im-COF also displayed a superior current density than that of NiPc-(NH2)8 in CO2-saturated 0.5 M KHCO3 electrolyte, which suggested that the porosity and conductivity of NiPc-Im-COF enhance the mass and electron transfer processes, thereby increasing the current density during the electrocatalytic CO2RR. To further assess the selectivity of NiPc-Im-COF for CO2RR, gas chromatography (GC) was employed to detect the gas products. As shown in Fig. 4b, Fig. S12 and S13, ESI,† NiPc-Im-COF showed high faradaic efficiencies of CO (FECO) (>90%) over a wide potential range from −0.7 V to −1.1 V vs. reversible hydrogen electrode (RHE) and exhibited nearly 100% at −0.7 and −0.8 V. It is important to note that all the potentials mentioned in this work are referenced to the reversible hydrogen electrode (RHE). Furthermore, the CO partial current density (JCO) of NiPc-Im-COF gradually increased with the rising overpotential, reaching 19.3 mA cm−2 at −1.1 V (Fig. 4c). Concurrently, the turnover frequency (TOF) increased with the potential and reached 1880 h−1 at −1.1 V. To evaluate the stability of the catalysts in 0.5 M KHCO3, the time-dependent total current density curves were obtained. The long-term stability of NiPc-Im-COF was analyzed at −0.8 V in CO2-saturated 0.5 M KHCO3 (Fig. S14, ESI†). Notably, the FECO remained consistently above 90% for a duration of 20 hours. Furthermore, the PXRD pattern of NiPc-Im-COF shown in Fig. S15, ESI† remained unchanged, indicating that good crystallinity was maintained even after undergoing the CO2 reduction reaction. Besides, the valence of Ni sites in NiPc-Im-COF was assessed by XPS after the CO2RR in 0.5 M KHCO3. The XPS curves of NiPc-Im-COF demonstrated that bonding energies at 872.7 eV and 855.4 eV are assigned to 2p3/2 and 2p1/2 of Ni(II), respectively (Fig. S16, ESI†), suggesting the excellent stability of NiPc-Im-COF under neutral condition.
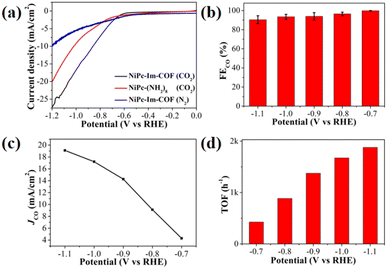 |
| Fig. 4 The CO2RR performances of the NiPc-Im-COF were studied in a H-cell. (a) LSV curves measured in N2-saturated and CO2-saturated 0.5 M KHCO3 solution, scan rate = 10 mv s−1; (b) FE for CO from −0.7 to −1.1 V vs. RHE; (c) CO partial current density; (d) TOF under different potentials. | |
To address the CO2 mass transfer limitation in H-cells, a GDE-based alkaline flow cell was employed. The conductive ink was drop-casted on single carbon paper with an area of 0.8 cm2 and a set of KOH solutions with gradient concentrations (1 M, 3 M, and 5 M) were used as electrolytes. As depicted in Fig. 5a, the LSV curves indicated that the total current density increased with the concentration of KOH, which resulted from the increased ion concentration and CO2 mass transfer. The current density obtained under alkaline GDE conditions far exceeded that of the neutral H-cell. Furthermore, we conducted tests to evaluate the product selectivity under various conditions. As shown in Fig. 5b, the FECO of the catalyst maintained over 90% over a range from −0.5 V to −0.9 V under 1 M KOH. Similarly, the catalyst also showed high FECO in the presence of 3 M and 5 M KOH aqueous solutions. Furthermore, the CO partial current density of the catalyst improved significantly, with JCO reaching 142 mA cm−2 at −0.8 V when 1 M KOH was employed as the electrolyte, which was 7-fold higher than the highest JCO in 0.5 M KHCO3 (Fig. 5c). Similarly, the JCO also increased with the concentration of KOH (3 M and 5 M KOH) and reached 231 mA cm−2 and 267 mA cm−2 at −0.8 V (Fig. 5c), respectively. Compared to previously reported materials of the same type, such a high current density is at the forefront (Fig. S17, ESI†). Likewise, the XPS spectrum shown in Fig. S18, ESI† proves that the valence of Ni sites in NiPc-Im-COF is still maintained at +2 after the CO2RR in all kinds of electrolytes. FECO kept over 90% for a duration of 6 hours when the CO2RR was conducted in 5 M KOH at −0.6 V (Fig. S19, ESI†). At the same time, the PXRD patterns performed after the CO2RR suggested that the structure of NiPc-Im-COF remained stable (Fig. S20, ESI†).
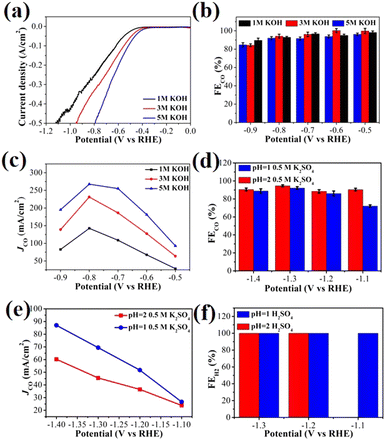 |
| Fig. 5 CO2RR performances for the NiPc-Im-COF were studied in a flow cell. (a) LSV curves measured in KOH solutions with a scan rate of 10 mv s−1; (b) FE for CO from −0.7 to −1.1 V vs. the RHE and (c) CO partial current density in 1 M, 3 M, and 5 M KOH; (d) FE for CO from −1.1 V to −1.4 V vs. the RHE and (e) CO partial current density in 0.5 M K2SO4 (pH = 1 and 2); (f) FE for H2 in 0.5 M K2SO4 (pH = 1 and 2). | |
To improve the utilization of CO2, CO2RR for the robust NiPc-Im-COF was also performed in GDE with acidic electrolyte (0.5 M K2SO4, the pH value was adjusted to 1 and 2 by H2SO4). As shown in Fig. S21, ESI,† the total current density in K2SO4 (pH = 1) was higher than that in K2SO4 (pH = 2) and exhibited ion concentration dependence, with only a slight decrease in the selectivity for CO2RR to CO. As illustrated in Fig. 5d, NiPc-Im-COF also exhibited high selectivity and the FECO exceeded 90% in K2SO4 (pH = 2) from −1.1 V to −1.4 V. When the pH was reduced to 1, FECO remained above 80% within the voltage range of −1.2 V to −1.4 V, and the maximum JCO reached 87 mA cm−2 (Fig. 5e). The electrocatalytic CO2RR was also carried out in H2SO4 (pH = 1 and 2) without added K2SO4, and the current density significantly decreased (Fig. S22, ESI†). Besides, the FEH2 was almost 100% in H2SO4 (Fig. 5f), demonstrating that the presence of K+ in the electrolyte effectively suppressed the HER. The pH value was tested after CO2RR. As shown in Fig. S23, ESI,† the pH values of electrolytes with K2SO4 (pH = 1 and 2) were unchanged, whereas the pH values of electrolytes without K2SO4 (pH = 1 and 2) changed to 1.7 and 2.4. The pH changes further confirmed the dominance of CO2RR in the presence of K+. The valence of Ni sites in NiPc-Im-COF and its crystallinity remained stable after CO2RR, as confirmed by XPS and XRD (Fig. S24 and S25, ESI†). FECO remained stable (about 90%) for 6 hours at −1.3 V in K2SO4 (pH = 1) (Fig. S26, ESI†).
In order to gain a deeper insight into the mechanism of the electrocatalytic activity of NiPc-Im-COF, 13C-labeled CO2 isotope experiments through gas chromatography–mass spectrometry (GC-MS) at −0.8 V in KH12CO3 and KCl electrolytes were conducted. As shown in Fig. 6a and Fig. S27, ESI,† when the CO2RR was conducted under 13C-labeling CO2 in 0.5 M KH12CO3, both the signals of 12CO and 13CO were observed. While only 13CO was observed when KH12CO3 electrolyte was replaced by the KCl electrolyte. These results revealed that the source of carbon in the CO2RR was the CO2 dissolved in the KHCO3 solution. Then, to confirm the intermediates during the CO2RR, in situ attenuated total reflection Fourier transform infrared spectroscopy (ATR-FTIR) at −0.8 V in 0.5 M KHCO3 was performed. As shown in Fig. 6b, the real-time FTIR spectra of CO2RR on NiPc-Im-COF electrodes exhibited two prominent bands at 1400 and 2330–2360 cm−1, which were assigned to the *COOH and adsorption of CO2, respectively.60,61 These results suggested that *COOH plays a crucial role as the key intermediate in the formation of CO.
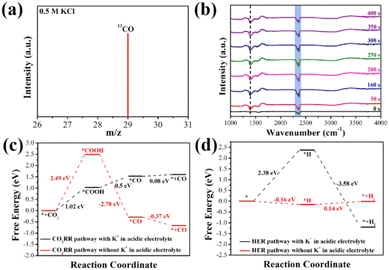 |
| Fig. 6 (a) Mass spectra of CO in the 13CO2-saturated 0.5 M KCl for NiPc-Im-COF; (b) operando ATR-FTIR of NiPc-Im-COF during CO2RR at −0.8 V in 0.5 M KHCO3; (c) free energy diagrams of NiPc-Im-COF for CO2RR and (d) HER pathways with or without K+ in acidic electrolyte. | |
Based on the results of isotope experiments and ATR-FTIR, density functional theory (DFT) calculations were conducted to calculate the free energies of reaction intermediates for CO2RR and HER. In general, the pathway for the formation of CO can be summarized as the adsorption of CO2 to form *CO2, then the generation of *COOH and *CO through the process of electron-coupled proton transfer, and finally, the CO desorption from active sites. From the free energy diagram in Fig. 6c and d, the energy barrier of the *COOH formation decreases from 2.49 eV to 1.02 eV, while the energy barrier of the *H formation increases from −0.16 eV to 2.38 eV when an acidic solution containing K+ is employed as electrolyte. The higher energy barrier of *H formation is consistent with the experimental results that CO is the major product for CO2RR in acidic electrolytes with K+.
Conclusions
In summary, the robust NiPc-Im-COF linked by irreversible covalent bonds was successfully prepared and employed as a highly efficient electrocatalyst for CO2RR. It exhibited a remarkable CO selectivity exceeding 90% within a broad potential range of −0.7 to −1.1 V. Moreover, due to the brilliant stability of the NiPc-Im-COF conferred by the imidazole ring in alkaline and acid solutions, KOH and acidic K2SO4 solutions were employed as electrolytes in GDE to break through the limitation of CO2 mass transfer. NiPc-Im-COF exhibited FECO exceeding 80% in KOH solutions of varying concentrations and the JCO reached 267 mA cm−2 at −0.8 V in 5 M KOH. Furthermore, NiPc-Im-COF exhibited high efficiency in CO2RR, with FECO exceeding 85% and a JCO of 87 mA cm−2 at −1.4 V in acidic K2SO4 (pH = 1), effectively suppressing the hydrogen evolution reaction (HER). Crucially, the structure of NiPc-Im-COF and the valence of Ni sites can remain stable after CO2RR, which is beneficial for the accessibility of active sites. Our work provides an effective strategy to enhance the stability of COFs through the linkage of the imidazole ring for CO2RR. This approach can shed light on the rational design of porous framework materials for the electrocatalytic CO2RR in various environments.
Author contributions
X. Y., H. L., Y. H., and R. C. conceived the project and wrote the manuscript. X. Y. performed the experiments and collected the data. H. L., D. S., and Y. H. polished the manuscript. All authors discussed the results and commented on the manuscript.
Conflicts of interest
The authors declare that they have no conflict of interest.
Acknowledgements
The work was supported by the National Key Research and Development Program of China (2021YFA1501500, 2022YFA1505700), NSFC (U22A20436, 22071245, 22033008, 22220102005, 22201286), and Fujian Science & Technology Innovation Laboratory for Optoelectronic Information of China (2021ZZ103), Open Science Promotion Plan 2023 of CSTCloud. The authors thank the beamline BL14W1 station for XAS measurements at the Shanghai Synchrotron Radiation Facility, China.
References
- A. Eleni, E. H. John, K. M. Edgar, G. L. Foster, A. Ridgwell, G. N. Inglis, R. D. Pancost, D. J. Lunt and P. N. Pearson, Changing atmospheric CO2 concentration was the primary driver of early Cenozoic climate, Nature, 2016, 533, 380–384 CrossRef PubMed.
- C. He, Y.-H. Zou, D.-H. Si, Z.-A. Chen, T.-F. Liu, R. Cao and Y.-B. Huang, A porous metal-organic cage liquid for sustainable CO2 conversion reactions, Nat. Commun., 2023, 14, 3317 CrossRef CAS PubMed.
- J. Liang, Q. Wu, Y. B. Huang and R. Cao, Reticular frameworks and their derived materials for CO2 conversion by thermo-catalysis, EnergyChem, 2021, 3, 100064 CrossRef CAS.
- J. Chen, Y. Zha, B. Liu, Y. Li, Y. Xu and X. Liu, Rationally designed water enriched nano reactor for stable CO2 hydrogenation with near 100% ethanol selectivity over diatomic palladium active sites, ACS Catal., 2023, 13, 7110–7121 CrossRef CAS.
- X. Feng, Y. Pi, Y. Song, C. Brzezinski, Z. Xu, Z. Li and W. Lin, Metal-Organic frameworks significantly enhance photocatalytic hydrogen evolution and CO2 reduction with earth-abundant copper photosensitizers, J. Am. Chem. Soc., 2020, 142, 690–695 CrossRef CAS PubMed.
- C. He, D.-H. Si, Y.-B. Huang and R. Cao, A CO2-masked carbene functionalized covalent organic framework for highly efficient carbon dioxide conversion, Angew. Chem., Int. Ed., 2022, 61, e202207478 CrossRef CAS PubMed.
- N. Li, J.-J. Liu, J.-W. Sun, B.-X. Dong, L.-Z. Dong, S.-J. Yao, Z. Xin, S.-L. Li and Y.-Q. Lan, Calix[8]arene-constructed stable polyoxo-titanium clusters for efficient CO2 photoreduction, Green Chem., 2020, 22, 5325–5332 RSC.
- N. Li, D.-H. Si, Q.-j Wu, Q. Wu, Y.-B. Huang and R. Cao, Boosting electrocatalytic CO2 reduction with conjugated bimetallic Co/Zn polyphthalocyanine frameworks, CCS Chem., 2023, 5, 1130–1143 CrossRef CAS.
- C. H. Vo, C. Mondelli, H. Hamedi, J. Pérez-Ramírez, S. Farooq and I. A. Karimi, Sustainability assessment of thermocatalytic conversion of CO2 to transportation fuels, Methanol, and 1-Propanol, ACS Sustainable Chem. Eng., 2021, 9, 10591–10600 CrossRef CAS.
- P. Zhang, X. Zhan, L. Xu, X. Fu, T. Zheng, X. Yang, Q. Xu, D. Wang, D. Qi, T. Sun and J. Jiang, Mass production of a single-atom cobalt photocatalyst for high-performance visible-light photocatalytic CO2 reduction, J. Mater. Chem. A, 2021, 9, 26286–26297 RSC.
- C. Costentin, M. Robert and J.-M. Savéant, Catalysis of the electrochemical reduction of carbon dioxide, Chem. Soc. Rev., 2013, 42, 2423–2436 RSC.
- S. Nitopi, E. Bertheussen, S. B. Scott, X. Liu, A. K. Engstfeld, S. Horch, B. Seger, I. E. L. Stephens, K. Chan, C. Hahn, J. K. Norskov, T. F. Jaramillo and I. Chorkendorff, Progress and perspectives of electrochemical CO2 reduction on copper in aqueous electrolyte, Chem. Rev., 2019, 119, 7610–7672 CrossRef CAS PubMed.
- Z. Chen, A. Huang, K. Yu, T. Cui, Z. Zhuang, S. Liu, J. Li, R. Tu, K. Sun, X. Tan, J. Zhang, D. Liu, Y. Zhang, P. Jiang, Y. Pan, C. Chen, Q. Peng and Y. Li, Fe1N4-O1 site with axial Fe-O coordination for highly selective CO2 reduction over a wide potential range, Energy Environ. Sci., 2021, 14, 3430–3437 RSC.
- G. Wu, Y. Song, Q. Zheng, C. Long, T. Fan, Z. Yang, X. Huang, Q. Li, Y. Sun, L. Zuo, S. Lei and Z. Tang, Selective electroreduction of CO2 to n-Propanol in two-step tandem catalytic system, Adv. Energy Mater., 2022, 12, 2202054 CrossRef CAS.
- Q.-X. Li, D.-H. Si, W. Lin, Y.-B. Wang, H.-J. Zhu, Y.-B. Huang and R. Cao, Highly efficient electroreduction of CO2 by defect single-atomic Ni-N3 sites anchored on ordered micro-macroporous carbons, Sci. China: Chem., 2022, 65, 1584–1593 CrossRef CAS.
- F. Lü, H. Bao, F. He, G. Qi, J. Sun, S. Zhang, L. Zhuo, H. Yang, G. Hu, J. Luo and X. Liu, Nitrogen dopant induced highly selective CO2 reduction over lotus-leaf shaped ZnO nanorods, Mater. Chem. Front., 2021, 5, 4225–4230 RSC.
- P. Wei, D. Gao, T. Liu, H. Li, J. Sang, C. Wang, R. Cai, G. Wang and X. Bao, Coverage-driven selectivity switch from ethylene to acetate in high-rate CO2/CO electrolysis, Nat. Nanotechnol., 2023, 18, 299–306 CrossRef CAS PubMed.
- C. Long, X. Liu, K. Wan, Y. Jiang, P. Wei, C. Yang, G. Wu, W. Wang, J. Guo, L. Li, K. Pang, Q. Li, C. Cui, S. Liu, T. Tan and Z. Tang, Regulating reconstruction of oxide-derived Cu for electrochemical CO2 reduction toward n-propanol, Sci. Adv., 2023, 9, eadi6119 CrossRef CAS PubMed.
- Y. Yan, L. Ke, Y. Ding, Y. Zhang, K. Rui, H. Lin and J. Zhu, Recent advances in Cu-based catalysts for electroreduction of carbon dioxide, Mater. Chem. Front., 2021, 5, 2668–2683 RSC.
- J. Sang, P. Wei, T. Liu, H. Lv, X. Ni, D. Gao, J. Zhang, H. Li, Y. Zang, F. Yang, Z. Liu, G. Wang and X. Bao, A reconstructed Cu2P2O7 catalyst for selective CO2 electroreduction to multicarbon, Angew. Chem., Int. Ed., 2022, 61, e202114238 CrossRef CAS PubMed.
- J. Zhang, G. Zeng, S. Zhu, H. Tao, Y. Pan, W. Lai, J. Bao, C. Lian, D. Su, M. Shao and H. Huang, Steering CO2 electroreduction pathway toward ethanol via surface-bounded hydroxyl species-induced noncovalent interaction, Proc. Natl. Acad. Sci. U. S. A., 2023, 120, e2218987120 CrossRef CAS PubMed.
- Y. Zhou, F. Che, M. Liu, C. Zou, Z. Liang, P. De Luna, H. Yuan, J. Li, Z. Wang, H. Xie, H. Li, P. Chen, E. Bladt, R. Quintero-Bermudez, T.-K. Sham, S. Bals, J. Hofkens, D. Sinton, G. Chen and E. H. Sargent, Dopant-induced electron localization drives CO2 reduction to C2 hydrocarbons, Nat. Chem., 2018, 10, 974–980 CrossRef CAS PubMed.
- J. Dong, Y. Liu, J. Pei, H. Li, S. Ji, L. Shi, Y. Zhang, C. Li, C. Tang, J. Liao, S. Xu, H. Zhang, Q. Li and S. Zhao, Continuous electroproduction of formate via CO2 reduction on local symmetry-broken single-atom catalysts, Nat. Commun., 2023, 14, 6849 CrossRef CAS PubMed.
- S. Garg, M. Li, A. Z. Weber, L. Ge, L. Li, V. Rudolph, G. Wang and T. E. Rufford, Advances and challenges in electrochemical CO2 reduction processes: an engineering and design perspective looking beyond new catalyst materials, J. Mater. Chem. A, 2020, 8, 1511–1544 RSC.
- N. Kornienko, Y. Zhao, C. S. Kley, C. Zhu, D. Kim, S. Lin, C. J. Chang, O. M. Yaghi and P. Yang, Metal-Organic frameworks for electrocatalytic reduction of carbon dioxide, J. Am. Chem. Soc., 2015, 137, 14129–14135 CrossRef CAS PubMed.
- C. Yan, H. Li, Y. Ye, H. Wu, F. Cai, R. Si, J. Xiao, S. Miao, S. Xie, F. Yang, Y. Li, G. Wang and X. Bao, Coordinatively unsaturated nickel-nitrogen sites towards selective and high-rate CO2 electroreduction, Energy Environ. Sci., 2018, 11, 1204–1210 RSC.
- L. Zhang, Z. J. Zhao and J. Gong, Nanostructured materials for heterogeneous electrocatalytic CO2 reduction and their related reaction mechanisms, Angew. Chem., Int. Ed., 2017, 56, 11326–11353 CrossRef CAS PubMed.
- B. Li, H. Ou, S. Chen, Y.-Q. Su and D. Wang, Recent Advances in CO2 Reduction Reaction to Value-added C1 Products by Single-atom Catalysts, Chem. Res. Chin. Univ., 2023, 39, 527–544 CrossRef CAS.
- Y. Mao, S. Chen, Z. Jia, X. Dong and Q. Mao, CV-etched Nanostructured Ag Foils for Efficient Electrochemical CO2 Reduction, Chem. Res. Chin. Univ., 2023, 39, 1031–1036 CrossRef CAS.
- M. E. Leonard, L. E. Clarke, A. Forner-Cuenca, S. M. Brown and F. R. Brushett, Investigating electrode flooding in a flowing electrolyte, gas-fed carbon dioxide electrolyzer, ChemSusChem, 2020, 13, 400–411 CrossRef CAS PubMed.
- C. S. Diercks, Y. Liu, K. E. Cordova and O. M. Yaghi, The role of reticular chemistry in the design of CO2 reduction catalysts, Nat. Mater., 2018, 17, 301–307 CrossRef CAS PubMed.
- Z. Liang, H.-Y. Wang, H. Zheng, W. Zhang and R. Cao, Porphyrin-based frameworks for oxygen electrocatalysis and catalytic reduction of carbon dioxide, Chem. Soc. Rev., 2021, 50, 2540–2581 RSC.
- C. Wang, Z. Lv, W. Yang, X. Feng and B. Wang, A rational design of functional porous frameworks for electrocatalytic CO2 reduction reaction, Chem. Soc. Rev., 2023, 52, 1382–1427 RSC.
- J. Wang, H. Hu, S. Lu, J. Hu, H. Zhu, F. Duan and M. Du, Conductive metal and covalent organic frameworks for electrocatalysis: design principles, recent progress and perspective, Nanoscale, 2022, 14, 277–288 RSC.
- Q.-J. Wu, J. Liang, Y.-B. Huang and R. Cao, Thermo-, electro-, and photocatalytic CO2 conversion to value-added products over porous metal/covalent organic frameworks, Acc. Chem. Res., 2022, 55, 2978–2997 CrossRef CAS PubMed.
- A. P. Côté, A. I. Benin, N. W. Ockwig, M. O’Keeffe, A. J. Matzger and O. M. Yaghi, Porous, crystalline, covalent organic frameworks, Science, 2005, 310, 1166–1170 CrossRef PubMed.
- P. J. Waller, F. Gándara and O. M. Yaghi, Chemistry of covalent organic frameworks, Acc. Chem. Res., 2015, 48, 3053–3063 CrossRef CAS PubMed.
- Q. Wu, M. J. Mao, Q. J. Wu, J. Liang, Y. B. Huang and R. Cao, Construction of donor-acceptor heterojunctions in covalent
organic framework for enhanced CO2 electroreduction, Small, 2021, 17, e2004933 CrossRef PubMed.
- Q. Wu, R.-K. Xie, M.-J. Mao, G.-L. Chai, J.-D. Yi, S.-S. Zhao, Y.-B. Huang and R. Cao, Integration of strong electron transporter tetrathiafulvalene into metalloporphyrin-based covalent organic framework for highly efficient electroreduction of CO2, ACS Energy Lett., 2020, 5, 1005–1012 CrossRef CAS.
- X. Zhang, Y.-Z. Yuan, H.-F. Li, Q.-J. Wu, H.-J. Zhu, Y.-L. Dong, Q. Wu, Y.-B. Huang and R. Cao, Viologen linker as a strong electron-transfer mediator in the covalent organic framework to enhance electrocatalytic CO2 reduction, Mater. Chem. Front., 2023, 7, 2661–2670 RSC.
- E. Jin, M. Asada, Q. Xu, S. Dalapati, M. A. Addicoat, M. A. Brady, H. Xu, T. Nakamura, T. Heine, Q. Chen and D. Jiang, Two-dimensional sp2 carbon-conjugated covalent organic frameworks, Science, 2017, 357, 673–676 CrossRef CAS PubMed.
- X.-N. Feng, Y. Yang, X. Cao, T. Wang, D.-M. Kong, X.-B. Yin, B. Li and X.-H. Bu, General approach to construct C–C single bond-linked covalent organic frameworks, J. Am. Chem. Soc., 2023, 145, 21284–21292 CrossRef CAS PubMed.
- B. Zhang, M. Wei, H. Mao, X. Pei, S. A. Alshmimri, J. A. Reimer and O. M. Yaghi, Crystalline dioxin-linked covalent organic frameworks from irreversible reactions, J. Am. Chem. Soc., 2018, 140, 12715–12719 CrossRef CAS PubMed.
- J. Liu, T. Yang, Z.-P. Wang, P.-L. Wang, J. Feng, S.-Y. Ding and W. Wang, Pyrimidazole-based covalent organic frameworks: integrating functionality and ultrastability via isocyanide chemistry, J. Am. Chem. Soc., 2020, 142, 20956–20961 CrossRef CAS PubMed.
- P.-F. Wei, M.-Z. Qi, Z.-P. Wang, S.-Y. Ding, W. Yu, Q. Liu, L.-K. Wang, H.-Z. Wang, W.-K. An and W. Wang, Benzoxazole-linked ultrastable covalent organic frameworks for photocatalysis, J. Am. Chem. Soc., 2018, 140, 4623–4631 CrossRef CAS PubMed.
- J. Guo, Y. Xu, S. Jin, L. Chen, T. Kaji, Y. Honsho, M. A. Addicoat, J. Kim, A. Saeki, H. Ihee, S. Seki, S. Irle, M. Hiramoto, J. Gao and D. Jiang, Conjugated organic framework with three-dimensionally ordered stable structure and delocalized π clouds, Nat. Commun., 2013, 4, 2736 CrossRef PubMed.
- M. Wang, M. Ballabio, M. Wang, H.-H. Lin, B. P. Biswal, X. Han, S. Paasch, E. Brunner, P. Liu, M. Chen, M. Bonn, T. Heine, S. Zhou, E. Cánovas, R. Dong and X. Feng, Unveiling electronic properties in metal-phthalocyanine-based pyrazine-linked conjugated two-dimensional covalent organic frameworks, J. Am. Chem. Soc., 2019, 141, 16810–16816 CrossRef CAS PubMed.
- Q. Zhang, F. Zhang, J. Dong, M. Shao, M. Zhu, D. Wang, Y. Guo, J. Zhang and Y. Liu, Controlling the nucleation process to prepare a family of crystalline tribenzimidazole-based covalent organic frameworks, Chem. Mater., 2022, 34, 6977–6984 CrossRef CAS.
- Z. Meng, R. M. Stolz and K. A. Mirica, Two-dimensional chemiresistive covalent organic framework with high intrinsic conductivity, J. Am. Chem. Soc., 2019, 141, 11929–11937 CrossRef CAS PubMed.
- H. Zhong, K. H. Ly, M. Wang, Y. Krupskaya, X. Han, J. Zhang, J. Zhang, V. Kataev, B. Büchner, I. M. Weidinger, S. Kaskel, P. Liu, M. Chen, R. Dong and X. Feng, Phthalocyanine-based layered two-dimensional conjugated metal-organic framework as highly efficient electrocatalyst for oxygen reduction reaction, Angew. Chem., Int. Ed., 2019, 58, 10677–10682 CrossRef CAS PubMed.
- Y. Liu, C. Li, C. Tan, Z. Pei, T. Yang, S. Zhang, Q. Huang, Y. Wang, Z. Zhou, X. Liao, J. Dong, H. Tan, W. Yan, H. Yin, Z.-Q. Liu, J. Huang and S. Zhao, Electrosynthesis of chlorine from seawater-like solution through single-atom catalysts, Nat. Commun., 2023, 14, 2475 CrossRef CAS PubMed.
- X. Han, T. Zhang, X. Wang, Z. Zhang, Y. Li, Y. Qin, B. Wang, A. Han and J. Liu, Hollow mesoporous atomically dispersed metal-nitrogen-carbon catalysts with enhanced diffusion for catalysis involving larger molecules, Nat. Commun., 2022, 13, 2900 CrossRef CAS PubMed.
- L. Lai, J. R. Potts, D. Zhan, L. Wang, C. K. Poh, C. Tang, H. Gong, Z. Shen, J. Lin and R. S. Ruoff, Exploration of the active center structure of nitrogen-doped graphene-based catalysts for oxygen reduction reaction, Energy Environ. Sci., 2012, 5, 7936 RSC.
- Z. Meng, A. Aykanat and K. A. Mirica, Welding metallophthalocyanines into bimetallic molecular meshes for ultrasensitive, low-power chemiresistive detection of gases, J. Am. Chem. Soc., 2018, 141, 2046–2053 CrossRef PubMed.
- F. Pan, H. Zhang, Z. Liu, D. Cullen, K. Liu, K. More, G. Wu, G. Wang and Y. Li, Atomic-level active sites of efficient imidazolate framework-derived nickel catalysts for CO2 reduction, J. Mater. Chem. A, 2019, 7, 26231–26237 RSC.
- S. Wu, H. Chen, C. Jia, L. Liao, K. Chen, S. Ci, Q. Xu and Z. Wen, Bifunctional electroreduction catalysts of NiFe alloy on N-doped carbon toward industrial-level CO2 conversion powered by Zn-air batteries, Inorg. Chem. Front., 2023, 10, 4484–4495 RSC.
- T. Zheng, K. Jiang, N. Ta, Y. Hu, J. Zeng, J. Liu and H. Wang, Large-scale and highly selective CO2 electrocatalytic reduction on nickel single-atom catalyst, Joule, 2019, 3, 265–278 CrossRef CAS.
- N. Han, Y. Wang, L. Ma, J. Wen, J. Li, H. Zheng, K. Nie, X. Wang, F. Zhao, Y. Li, J. Fan, J. Zhong, T. Wu, D. J. Miller, J. Lu, S.-T. Lee and Y. Li, Supported cobalt polyphthalocyanine for high-performance electrocatalytic CO2 reduction, Chem., 2017, 3, 652–664 CAS.
- D. M. Koshy, S. Chen, D. U. Lee, M. B. Stevens, A. M. Abdellah, S. M. Dull, G. Chen, D. Nordlund, A. Gallo, C. Hahn, D. C. Higgins, Z. Bao and T. F. Jaramillo, Understanding the origin of highly selective CO2 electroreduction to CO on Ni, N-doped carbon catalysts, Angew. Chem., Int. Ed., 2020, 59, 4043–4050 CrossRef CAS PubMed.
- N. J. Firet and W. A. Smith, Probing the reaction mechanism of CO2 electroreduction over Ag films via operando infrared spectroscopy, ACS Catal., 2016, 7, 606–612 CrossRef.
- Q.-J. Wu, D. Si, Q. Wu, Y. Dong, R. Cao and Y.-B. Huang, Boosting Electroreduction of CO2 over Cationic Covalent Organic Frameworks: Hydrogen Bonding Effects of Halogen Ions, Angew. Chem., Int. Ed., 2023, 62, e202215687 CrossRef CAS PubMed.
|
This journal is © the Partner Organisations 2024 |