DOI:
10.1039/D4DT00580E
(Paper)
Dalton Trans., 2024,
53, 6264-6274
Voltage-induced modulation of the magnetic exchange in binuclear Fe(III) complex deposited on Au(111) surface†
Received
27th February 2024
, Accepted 15th March 2024
First published on 15th March 2024
Abstract
We present a complete computational study devoted to the deposition of a magnetic binuclear complex on a metallic surface, aimed to obtain insight into the interaction of magnetically coupled complexes with their supporting substrates, as well as their response to external electrical stimuli applied through a surface-molecule-STM molecular junction-like architecture. Our results not only show that the deposition is favorable in two of the four studied orientations, but also, that the magnetic coupling is only slightly perturbed once the complex is adsorbed. We observe that the effects of the applied bias voltage on the magnetic coupling strongly depend on the molecule orientation with respect to the surface and the voltage polarity. Further analysis shows that this behavior is attributable to the stabilization/destabilization of the d-type singly occupied orbitals of the iron centers, reinforced by the strong local electric fields and induced charge densities only present in certain orientations of the deposited molecule and applied voltage polarity.
Introduction
Aviram and Ratner proposed in 1974 a molecular rectifier that predicted the feasibility of building a functional molecular device using single-molecular components as active elements.1 Since this seminal work, researchers have made great efforts to replicate the functions performed by silicon-based microelectronic devices by single molecules, giving rise to the field of molecular electronics.
The advent of spintronics during the 80s, triggered by the discovery of the giant magnetoresistance (GMR) effect,2,3 sparked the interest of the molecular magnetism community, which immediately commenced to envisage how single molecules could reproduce the functions of the first mesoscopic spin valves (three-layer structures whose conductivity can be modulated through its overall magnetic state), giving rise to a new branch in the molecular electronics field, i.e., molecular spintronics.4 To this day, technological developments such as the scanning tunneling microscope (STM)5 or the technique known as mechanically controllable break junction (MCBJ)6 have allowed the addressing and manipulation of the magnetic and transport properties of single molecules, or even atoms, paving the way to the fabrication of commercial spintronic devices in the near future. Due to their inherent chemical versatility, which allows the fine-tuning of sought properties, organometallic complexes are natural candidates to act as magnetically active elements in these hypothetical molecular devices. In fact, nowadays, it is possible to study and characterize the magnetic and transport properties of metallic complexes deposited over different substrates, such as metallic surfaces7–9 or graphene,10–12 or even encapsulated inside single-walled nanotubes.13 Moreover, it has been demonstrated that it is feasible to induce the switching of the spin state of deposited spin crossover complexes14–16 through an external electric field, employing an STM tip, with different voltage values between the tip and surface.17–20 Regarding the magnetic properties of the magnetic units, polynuclear metallic complexes offer a higher degree of flexibility due to the almost unlimited number of combinations of coordination ligands, metal ions, and molecular bridges between them, allowing chemists to obtain tailored compounds adjusted to their needs.21–23 The magnetic coupling between the centers (characterized by the magnetic coupling constant J), ferromagnetic (FM) or anti-ferromagnetic (AF), determines the complex's spin ground state. Several polynuclear magnetic complexes have been deposited and characterized over different substrates,24–28 where in some systems, the interaction of the complex with the substrate could drastically modify the magnetic coupling between the metal centers.29–31 Thus, the magnetic coupling is a critical parameter to control at the time of synthesizing new complexes and once they interact with supporting substrates. On the other hand, the electric-induced switching of the magnetic coupling in polynuclear complexes has been scarcely studied, chiefly reduced to model systems.32–34 An important step in this way was made in 2013, when Wagner and co-workers studied the transport properties of a magnetically coupled binuclear Co2+ complex anchored to two Au electrodes in an MCBJ junction setup.35 In this work, the magnetic coupling was successfully modulated by applying different bias voltages, experimentally showing that a voltage-triggered switch from the ferromagnetic to the anti-ferromagnetic state is feasible.
In this context, it is crucial to obtain a deeper insight into the mechanisms underlying the measured magnetic properties of magnetically coupled molecules interacting with their surroundings. To address this challenge, we performed a rigorous computational study focused on the deposition of a binuclear magnetic complex over a metallic surface. Although isolated polynuclear magnetic molecules have been extensively studied through sophisticated wave function based methods (WFT),36–40 this is no longer possible once they are deposited on a substrate due to the extensive size of the systems. In this sense, we employed Density Functional Theory (DFT) based methods, widely used to determine the magnetic properties of metallic complexes interacting with different substrates,41–46 and even their transport properties in single molecule junctions architectures.47–52 After a series of rigorous calibration tests, we stablished a DFT-based methodology that allowed us to accurately determine the electronic and magnetic properties of the deposited complex at an affordable computational cost. In addition, we designed a suite of molecular-junction-type architectures, where the binuclear complex is sandwiched between the gold surface and a gold STM tip, to probe the effects of an applied bias voltage on the molecular magnetic coupling.
We focus on the complex (C38H32N8)Fe2O, (complex 1, Fig. 1), synthesized by Sessler and co-coworkers.53 The system consists of an “accordion porphyrin”, a dipyrromethane-based macrocycle, with accordion-like aliphatic chains bridging two dipyrromethene moieties, and two Fe(III) centers bridged by an oxygen atom. To the best of our knowledge, the magnetic properties of this system have not been previously studied either experimentally or theoretically.
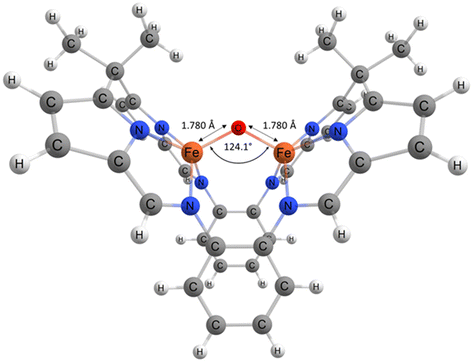 |
| Fig. 1 Complex 1 molecular structure experimentally determined by X-ray diffraction in ref. 36. | |
We proceed to deposit 1 over an Au(111) surface in four different orientations, followed by the complete geometry optimization of the systems in order to find the most stable configurations as well as the impact that the deposition has on the magnetic properties of 1. Subsequently, we constructed a series of molecular junctions featuring 1 as the magnetic active element, using as electrodes, the Au(111) surface and an STM tip, aimed to explore the impact of the applied voltage on the intramolecular magnetic coupling. Our results indicate that the isolated molecule presents an AF magnetic coupling between the Fe(III), with an amplitude of 207 cm−1, in line with reported values for similar μ-oxo bridged dinuclear Fe(III) complexes.
The molecule interacts strongly with the Au(111) surface. Two of the four explored are particularly stabilized by more than 1.4 eV, but this strong molecule-surface interaction has almost no effect on the magnetic coupling constant. When an external voltage is applied through an STM tip, different behaviors of J vs. V are obtained depending on the orientation of the molecule with respect to the surface and the polarity of the voltage. We were able to rationalize these effects based on the Hay, Thibeault, and Hoffmann approach, which relates the observed J vs. V behavior with the stabilization/destabilization of the d-type Fe orbitals. Further analysis suggested that these effects could be caused by the strong local electric fields experienced by the Fe–O–Fe moiety, resulting from the inhomogeneous electrostatic potential and induced charge densities over different regions of the molecular junction, that are not observed under the influence of a homogeneous electric field.
Computational details
Electronic structure and magnetic characterization of complex 1
Using the molecular structure determined by X-ray diffraction,53 we evaluated the electronic structure and magnetic properties of 1 through DFT and WFT methods.
The WFT calculations were carried out with the ORCA software package54 using the complete active space self-consistent field (CASSCF) methodology,55 along with the N-Electron Valence State Perturbation Theory (NEVPT2)56 to consider the effects of the dynamic correlation. The def2-TZVP57 basis set was chosen to represent all atoms, with the exception of the Fe(III) ions, where a larger def2-QZVP57 basis set was employed. In addition, the resolution of the identity (RI)58 approach was adopted to increase the efficiency of all calculations. The active space utilized in the CASSCF calculations consists of 10 electrons in 10 orbitals corresponding to 10 electrons occupying the five 3d-type orbitals centered on each of the two Fe(III) ions (Fig. S1.1†).
All DFT calculations were performed with the Quantumwise ATK program suite,59 determining the nature and strength of the magnetic coupling through the Broken Symmetry (BS) formalism (ESI S1.2.1†). For all atoms, the valence electrons were represented by a double-ζ-polarized (DZP) basis set, along with PseudoDojo norm-conserving pseudopotentials60 to model the core electrons. Based on a series of calibration tests (ESI S1.2.2†), we decided to employ the Perdew–Burke–Ernzerhof (PBE)61 exchange–correlation (XC) functional in combination with a Hubbard Ueff correction62–64 (PBE+U) to the Fe(III) d orbitals (Ueff = 4.0 eV). This scheme allowed us to accurately determine the electronic structure and magnetic properties of 1 in the gas phase, deposited on the Au(111) surface, and as the active element in the molecular junction architecture at a consistent level of theory and at an affordable computational cost.
Deposition of the complex over the Au(111) surface and interaction with the STM tip
The deposition of 1 over the Au(111) surface was studied employing the Quantumwise ATK program suit utilizing the same basis set, convergence parameters, and methodology (PBE+U) established for the isolated complex (ESI S1.2.2†), exploring four possible orientation of the molecule with respect to the surface (Fig. 2, left panel). Subsequently, to probe the effects an applied bias voltage would have on the deposited complex, we simulated a surface-molecule-STM molecular-junction-like setup, modeling an open system through the combination of the DFT with the Keldysh non-equilibrium Green's function formalism (DFT-NEGF),65 featuring complex 1 as magnetic active element, and employing the Au(111) surface as one of the electrodes and the gold tip of an STM as the other (Fig. 2, right panel). A full description of the methodology and utilized models can be found in sections S2 and S3 of the ESI.†
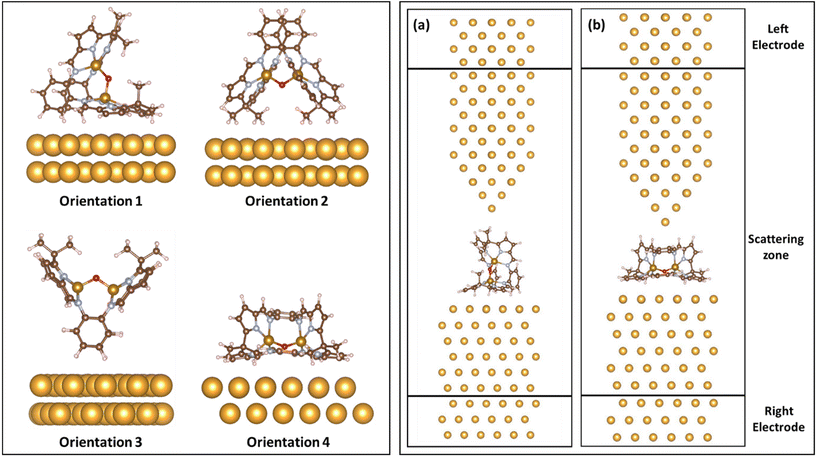 |
| Fig. 2 (Left panel) Optimized geometry adopted by complex 1 over the Au(111) surface in the four studied orientations. (Right panel) Depiction of the employed surface-complex-STM molecular junction-type setups featuring complex 1 as magnetic active element in (a) orientation 1 and (b) orientation 4 as magnetic active element. | |
Results and discussion
Electronic structure and magnetic properties of complex 1
The molecular structure of complex 1 consists of a μ-oxo bis Fe(III) moiety coordinated to a tetradentate macrocycle formed by two dipyrromethene units linked by two phenylene diamines. In this environment, each iron center is pentacoordinated in a distorted square pyramid geometry (Fig. 1). In this ligand field, the local spin moment of each Fe(III) ion is S = 5/2.
Although there is no experimental magnetic characterization available for 1, the geometry of the Fe–O–Fe unit suggests an antiferromagnetic interaction between the Fe centers, as observed in similar oxo bridged Fe(III) dinuclear complexes.66
Employing the experimental geometry reported for 1,53 we determine the electronic structure and magnetic properties of the complex through DFT and WFT-based methods (see Computational details).
Though both methodologies predicted an antiferromagnetic coupling, they differ in its magnitude.
Employing the DFT-based Broken Symmetry (BS) methodology, we obtained a J value of −214.02 cm−1, in line with the experimental values observed in similar μ-oxo bridged dinuclear Fe(III) pentacoordinated complexes, with experimental J values ranging from −160 to −220 cm−1.67–70
CASSCF(10,10)/NEVPT2 calculations yield a value of J = −113.3 cm−1. Regarding the active CASSCF orbitals (Fig. S1.1†), it can be inferred that this antiferromagnetic coupling is mainly governed by the dz2, dyz, dxz Fe orbitals, through a superexchange mechanism through the 2p orbitals of the bridging oxygen atom. The amplitude of the coupling at this level is apparently underestimated with respect to other dinuclear Fe(III) pentacoordinated complexes. It is well known that CASSCF calculations based on a minimal active space, i.e., only the singly occupied d-type orbitals and their corresponding electrons, tend to underestimate the strength of the coupling, even once the dynamic correlation is considered through second order perturbation theory, NEVPT2 in the present case.71 As previously reported, it is possible to improve the results by extending the active space with the Fe double d-shell and the 2p occupied orbitals of the bridging oxygen atom.71 Alternatively, and resting on a minimal CAS, it is possible to obtain more accurate results through a variational methodology such as the difference dedicated configuration interaction (DDCI) approach,72 or even through a perturbative approach using a convenient optimized set of molecular orbitals.73,74 Unfortunately, due to the extension of the complex and the size of the minimal active space (10,10), none of these procedures are feasible at an affordable computational cost in this case.
Deposition of complex 1 over Au(111)
We probe four different initial orientations of 1 with respect to the Au(111) surface (Fig. 2 and Fig. S2.1†). The adsorption energies, the magnetic moment of each Fe(III) ion and bridging oxygen atom, and the computed J value of 1 in the four orientations after a complete geometry relaxation of the surface-molecule system are reported in Table 1.
Table 1 Adsorption energy (Eads), coupling constant value (J), effective magnetic moments (μeff), Fe–O bond distances (d), and Fe–O–Fe bridge angle (Θ) computed for complex 1 deposited over the Au(111) surface in the four studied orientations
Orientation |
E
ads (eV) |
J (cm−1) |
Θ (°) |
d (Å) |
μ
eff (Fe1, Fe1, O) (μβ) |
1 |
−1.466 |
−204.60 |
121.7 |
1.79 |
4.43, 4.43, 0.5 |
2 |
−0.176 |
−197.27 |
120.7 |
1.80 |
4.42, 4.42, 0.5 |
3 |
0.286 |
−200.02 |
119.2 |
1.80 |
4.42, 4.42, 0.5 |
4 |
−1.568 |
−196.89 |
115.8 |
1.79 |
4.41, 4.42, 0.49 |
Isolated |
— |
−214.02 |
124.1 |
1.78 |
4.43, 4.43, 0.5 |
The molecule is stable on the surface for three of the four explored orientations. Two orientations are clearly preferred, and we will focus only on them hereafter: orientation 1, where the Fe–O–Fe axis is perpendicular to the surface plane, and orientation 4, where this axis is parallel to the surface. In the former case, the complex interacts with the surface through one of the dipyrromethene moieties, with both pyrrole subunits nearly parallel to the surface (Fig. 2 and S2.1†), and the hydrogen atoms of the two phenylene diamines, in this case, are perpendicular to the surface. In orientation 4, the deposition is carried out through one pyrrole group of each one of the dipyrromethene units, parallel to the surface, together with the closest phenylene diamine to the surface, also parallel to the plane of the Au(111) surface (Fig. 2 and S2.1†).
Regarding the magnetic coupling, in all four explored orientations, the J value after deposition is practically unchanged. In fact, crucial geometrical parameters that determine the magnetic coupling in 1, like the Fe–O–Fe angle and the Fe–N bond distances, experiment little change once the complex is deposited (Table 1). The only exception is the final Fe–O–Fe angle in orientation 4, decreasing around 8 degrees, reflected in a slight decrease of the J amplitude.
The shape and energy positions (with respect to the Fermi level) of the frontier orbitals of the deposited molecule could determine crucial properties, such as, the total conductance or the spin filter efficiency. To obtain further insight into the deposition's effects on complex 1 electronic structure, we computed the molecular projected self-consistent Hamiltonian (MPSH) states of the complex over the surface. These states are obtained by diagonalizing the molecular part of the full self-consistent Hamiltonian of the molecule-surface system, and to some extent could be considered as the molecular orbitals of the complex under the influence of the metallic surface. We observed that the frontier orbitals drastically change once the complex is adsorbed (Fig. 3). For orientation 1, all the orbitals delocalized over both dipyrromethene moieties in the isolated complex case (ESI section S4†), are now localized on only one of these units (ESI section S5†). This behavior is expected, since in this orientation one dipyrromethene fragment is much closer to the surface than the other. It is worth mentioning that for the frontier virtual orbitals with contributions from the Fe atoms (in the HS solution), the electronic density is still evenly delocalized on both centers, despite the asymmetric distance of the magnetic centers to the surface. A similar localization effect is observed in orientation 4, but in this case, the orbitals are localized over just one of the pyrrole groups. Of special interest are the spin up LUMO and LUMO+1, which exhibit a large electronic density in regions near the surface, experiencing an important stabilization with respect to their counterparts in orientation 1 (Fig. S3.2†).
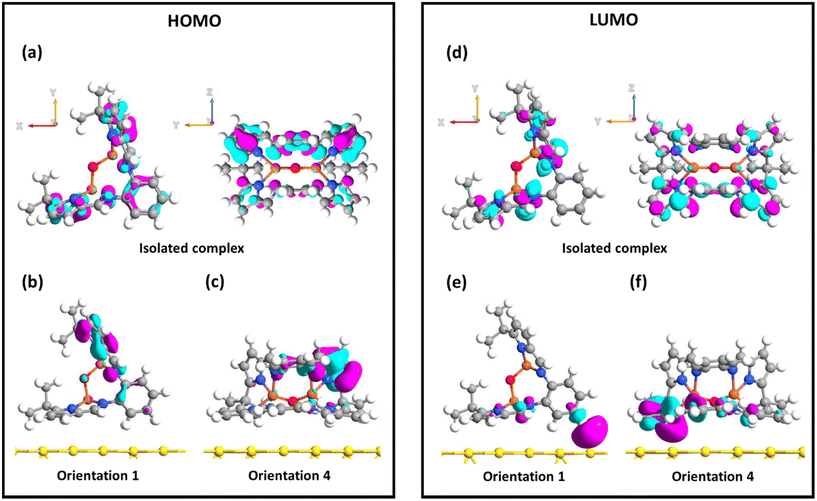 |
| Fig. 3 Comparation between complex 1 HOMO/LUMO in (a)/(d) the gas phase and once is deposited over the Au(111) surface in (b)/(e) orientation 1 and (c)/(f) orientation 4. | |
Effects of the bias voltage in Au(111)-complex-STM molecular junction
To evaluate the effects that an applied bias voltage would have in the magnetic coupling, we constructed a series of molecular junctions recreating a surface-molecule-STM-like setup, featuring the complex deposited over the Au(111) surface as the magnetic active element, in both orientations 1 and 4 (Fig. 2, right panel). Then, we compute the J value through the BS methodology, considering the effects that the applied bias voltage has on the magnetic coupling in a self-consistent way through the NEGF-DFT methodology. In this way, we were able to explicitly include the effects that the induced charge density, resulting from the potential difference between the electrodes, has on the effective potential at different regions of the molecule in a non-isotropic way. Thus, we did not have to resort to approximations that simulate a homogenous electric field, that do not necessarily reproduce the actual potential profile in the junction. The J values obtained in each orientation for an applied bias voltage from −1 V to 1 V are reported in Fig. 4(a). In all cases, the chemical potential of the left electrode (μL), corresponding to the extension of the Au(111) surface, was held fixed. A positive voltage corresponds to a decrease in the right electrode chemical potential (μR) by V·e (where e is the electron charge and V the applied voltage value), and a negative one to an increase of μR by −V·e. With this definition, a negative voltage induces an electron flow from the tip to the sample and a positive one from the ample to the tip. By the inspection of Fig. 4(a), a significant increase in the antiferromagnetic coupling can be observed in orientation 4, but only when a negative voltage is applied. However, the J coupling is not voltage-dependent for orientation 1.
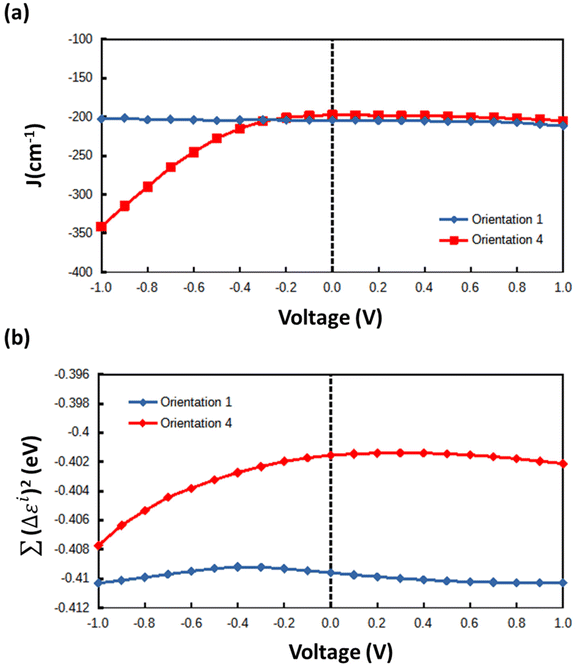 |
| Fig. 4 (a) Coupling constant J computed through the BS methodology in the two studied molecular junctions applying different bias voltages. (b) Voltage dependence of the sum of the energy differences between the corresponding symmetric (ψs) and antisymmetric (ψa) d-type molecular orbitals employed in the Hay, Thibeault and Hoffmann (HTH) analysis. | |
To obtain further insight into the mechanisms underlying the behavior of J, we performed the molecular orbital-based analysis introduced by Hay, Thibeault, and Hoffmann (HTH),75 (ESI S3.3†) utilized on multiple occasions, especially to establish magneto-structural correlations in bimetallic complexes.76–79 In this model, the antiferromagnetic contribution to J is proportional to the square of the energy difference between the in-phase (ψs = ψdA + ψdB) and out-of-phase (ψa = ψdA − ψdB) combinations of the magnetic orbitals of the complex.
In this case, we computed the energy differences between the corresponding five d-like pairs
of the MPSH states projected in the Fe–O–Fe moiety of the complex (Fig. S3.3 and S3.4†), in the molecular junction systems, and at each of the studied voltage values. Plots of (Δεi)2 against the applied voltage values can be found in Fig. S3.5.† Remarkably, for both orientations, the plot of the sum of the five d-type orbital contributions
(Fig. 4(b)) follows the same trends that the corresponding J vs. V curves. This suggests that the increase of the antiferromagnetic coupling in orientation 4, when a negative voltage is applied, results from the voltage-dependent stabilization/destabilization of the singly-occupied d-type orbitals. For both orientations, if we inspect the individual (Δεi)2vs. V plots (Fig. S3.5†), it can be seen that the orbitals with a larger contribution to the overall AF coupling are those labeled as dz2, dxz, and dyz, as expected, since they have large contributions of the 2p orbitals of the bridging oxygen atom, favoring the super exchange mechanism. Particularly, in orientation 4 case, it can be seen that Δεi in the dxz and dyz cases increase with the increasing negative bias voltage, whereas in the dz2 the Δεi decreases. Thus, although the magnitude of (Δεi)2 is greater for dz2 the combined increase in (Δεi)2 for the dxz and dyz orbitals result in an overall rise of the antiferromagnetic coupling. Regarding the stabilization/destabilization of the in- and out- of phase combinations of the dz2, dxz, and dyz orbitals, it is instructive to plot their evolution as the voltage increases with respect to their corresponding values at zero bias (Fig. S3.6†). In the first place, we can see that all six orbitals show a parabolic behavior, with each curve minima located at a negative voltage. Three of them, dz2(a), dxz(a), and dyz(s), present a very similar trend, all presenting their minima at 0.7 V, all of them with contribution from the bridging oxygen atom. Next, we observed that the two orbitals most stabilized by the voltage, dxz(s) and dyz(a), correspond to the d-type orbital combinations with no contribution from the oxo-bridge and have their minima at 0.7 and 0.8 V, respectively. Finally, the dz2(s) orbital is the least stabilized by the applied bias, presenting its minimum at only 0.4 V, and presenting a small contribution from the 2s orbital of the oxygen atom.
One of the possible causes of the observed different behaviors of J vs. V, with respect to the complex orientation and voltage polarity, could be the different electric fields experienced by the Fe–O–Fe fragment in each case. The impact of an homogeneous electric field on the magnetic coupling in dinuclear Cu2 compounds has been recently analyzed by Guihéry and co-workers,80 showing that the orientation of the field with respect to the Cu–Cl–Cu fragment is a key factor of the J vs. E dependence. To check this effect in our systems, we applied a homogeneous electric field to complex 1, parallel to the normal of the Au(111) surface (the same orientation of the field produced by the applied voltage in the junction), employing the geometry adopted after the deposition in both orientations (Fig. S3.7†). Only the complex is explicitly taken into account in these calculations. As can be seen in Fig. S3.7,† a homogeneous electric field of about ∼104 kV has little influence on both the computed J values and (Δεi)2, as observed in ref. 62 for this orientation of the molecule with respect to the applied field.
Then, a homogeneous electric field is not responsible for the observed J-vs.-V behavior. To elucidate if the asymmetry of the J vs. V profiles could stem from inhomogeneous electric fields produced in the actual junctions, we compute the induced charge density and potential profiles, obtained by the DFT-NEGF calculations, of the two systems when voltages of −1 V and 1 V are applied (Fig. 5). As can be seen in Fig. 5, for orientation 4 at 1 V and orientation 1 at both −1 V and 1 V the voltage drop is relatively smooth over the molecular region of the scattering zone, similar to that observed in the case of the applied homogeneous field (Fig. S3.7†), becoming more pronounced at approaching the STM tip. This almost linear drop produces a homogeneous redistribution of charge in the complex, along with a moderate electric field on the spatial region occupied by the complex. Conversely, when a negative voltage is applied to the junction with the complex deposited in orientation 4, an abrupt potential drop is observed between the surface and the lower part of the complex (just where the Fe–O–Fe moiety is located), followed by a plateau in the remaining part of the molecule, to finally steeply drop again in the zone near the gap between the molecule and the STM tip. This pronounced potential drop over a short space extension gives rise to strong local and non-homogeneous electric fields on the metal centers and bridging oxygen atom, accompanied by a marked charge redistribution in this fragment, which at the end can be responsible of the enhancement of the antiferromagnetic contribution to the coupling.
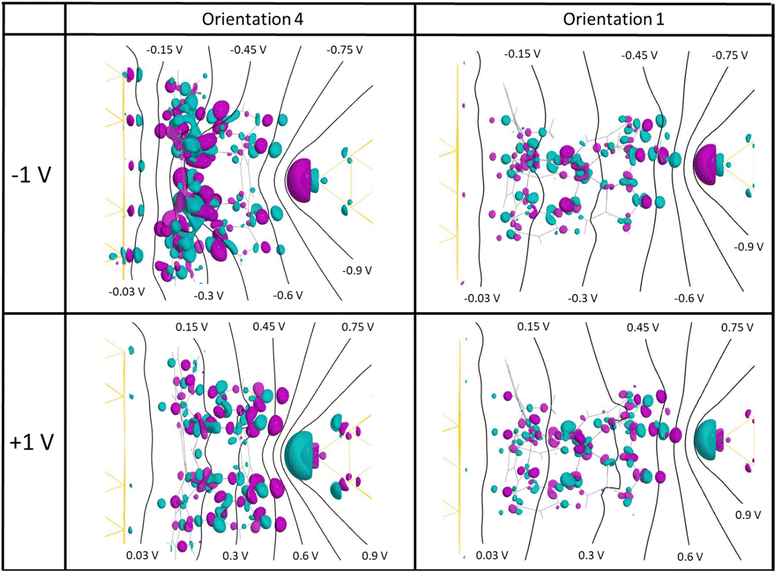 |
| Fig. 5 Induced charge densities and electrostatic potential isolines in the junctions featuring complex in HS configuration applying a bias voltage of −1 V and +1 V. In the plots, magenta/cyan represent excess/deficiency of charge with respect to respective systems at zero bias. All the iso surfaces were set to 3.5 × 10−4 1 bohr−3. | |
Conclusions
In this work, we theoretically studied the magnetic exchange on complex 1 in the gas phase, deposited over an Au(111) surface, and interacting with a STM tip. Our results indicate that coupling between the Fe(III) ions in complex 1 is antiferromagnetic, with a value of J = −207.89 cm−1, in line with the experimental values obtained for similar μ-oxo bridged binuclear Fe(III) complexes. Of the four orientations explored for the deposition, two were favored by around −1.5 eV, where the computed J value of the adsorbed complex shows only slight deviations from the value obtained in the gas phase. The effects of an applied voltage bias on J were probed through DFT-NEGF calculations performed on a series of molecular junction-like setups. We observed orientation and voltage polarity dependence on the J vs. V profiles. We were able to rationalize the mechanisms underlying this behavior employing the HTH methodology, in base of the voltage-dependent stabilization/destabilization of the single occupied d-type orbitals, stemming from the different electrostatic potential landscapes and induced electron densities in each case, depending on the molecule orientation and bias polarity. The presented findings could result useful at the time of rationalizing future STM measurements featuring this type of complexes as active molecular elements.
Conflicts of interest
The authors have no conflicts to disclose.
Acknowledgements
N. M. P. thanks to the financial support of “ANID Postdoctorado FONDECYT 3210181”. This research was partially supported by the supercomputing infrastructure of the NLHPC (ECM-02) of the Universidad de Chile. C. J. C. acknowledge the financial support through grant PID2021-127674NB-I00 funded by MCIN/AEI/10.13039/501100011033 and by “ERDF_A way of making Europe”. The technical support of the Supercomputing Team of the Centro Informático Científico de Andalucía (CICA) and the access to the computational facilities of the “Centro de Servicios de Informática y Redes de Comunicaciones” (CSIRC, Universidad de Granada, Spain) are also acknowledged. G. C. J. thank the financial support of ANID/Chile under Project FONDECYT 1221072.
References
- A. Aviram and M. A. Ratner, Molecular rectifiers, Chem. Phys. Lett., 1974, 29(2), 277–283 CrossRef CAS.
- M. N. Baibich, J. M. Broto, A. Fert, F. N. Van Dau, F. Petroff, P. Etienne, G. Creuzet, A. Friederich and J. Chazelas, Giant Magnetoresistance of (001)Fe/(001)Cr Magnetic Superlattices, Phys. Rev. Lett., 1988, 61(21), 2472–2475 CrossRef CAS PubMed.
- G. Binasch, P. Grünberg, F. Saurenbach and W. Zinn, Enhanced magnetoresistance in layered magnetic structures with antiferromagnetic interlayer exchange, Phys. Rev. B: Condens. Matter Mater. Phys., 1989, 39(7), 4828–4830 CrossRef CAS PubMed.
- M. Sun and W. Mi, Progress in organic molecular/ferromagnet spinterfaces: towards molecular spintronics, J. Mater. Chem. C, 2018, 6(25), 6619–6636, 10.1039/C8TC01399C.
- L. A. Bumm, J. J. Arnold, M. T. Cygan, T. D. Dunbar, T. P. Burgin, L. Jones, D. L. Allara, J. M. Tour and P. S. Weiss, Are Single Molecular Wires Conducting?, Science, 1996, 271(5256), 1705 CrossRef CAS.
- M. A. Reed, C. Zhou, C. J. Muller, T. P. Burgin and J. M. Tour, Conductance of a Molecular Junction, Science, 1997, 278(5336), 252 CrossRef CAS.
- S. K. Karuppannan, A. Martín-Rodríguez, E. Ruiz, P. Harding, D. J. Harding, X. Yu, A. Tadich, B. Cowie, D. Qi and C. A. Nijhuis, Room temperature conductance switching in a molecular iron(III) spin crossover junction, Chem. Sci., 2021, 12(7), 2381–2388, 10.1039/D0SC04555A.
- B. W. Heinrich, L. Braun, J. I. Pascual and K. J. Franke, Tuning the Magnetic Anisotropy of Single Molecules, Nano Lett., 2015, 15(6), 4024–4028 CrossRef CAS PubMed.
- S. Bhandary, B. Brena, P. M. Panchmatia, I. Brumboiu, M. Bernien, C. Weis, B. Krumme, C. Etz, W. Kuch and H. Wende,
et al., Manipulation of spin state of iron porphyrin by chemisorption on magnetic substrates, Phys. Rev. B: Condens. Matter Mater. Phys., 2013, 88(2), 024401 CrossRef.
- Y. Zheng, L. Huang, Z. Zhang, J. Jiang, K. Wang, L.-M. Peng and G. Yu, Sensitivity enhancement of graphene Hall sensors modified by single-molecule magnets at room temperature, RSC Adv., 2017, 7(4), 1776–1781, 10.1039/C6RA27673C.
- F. Paschke, P. Erler, V. Enenkel, L. Gragnaniello and M. Fonin, Bulk-Like Magnetic Signature of Individual Fe4H Molecular Magnets on Graphene, ACS Nano, 2019, 13(1), 780–785 CrossRef CAS PubMed.
- B. de la Torre, M. Švec, P. Hapala, J. Redondo, O. Krejčí, R. Lo, D. Manna, A. Sarmah, D. Nachtigallová and J. Tuček,
et al., Non-covalent control of spin-state in metal-organic complex by positioning on N-doped graphene, Nat. Commun., 2018, 9(1), 2831 CrossRef PubMed.
- J. Villalva, A. Develioglu, N. Montenegro-Pohlhammer, R. Sánchez-de-Armas, A. Gamonal, E. Rial, M. García-Hernández, L. Ruiz-Gonzalez, J. S. Costa and C. J. Calzado,
et al., Spin-state-dependent electrical conductivity in single-walled carbon nanotubes encapsulating spin-crossover molecules, Nat. Commun., 2021, 12(1), 1578 CrossRef CAS PubMed.
- K. Senthil Kumar and M. Ruben, Emerging trends in spin crossover (SCO) based functional materials and devices, Coord. Chem. Rev., 2017, 346, 176–205 CrossRef CAS.
- M. Gruber and R. Berndt, Spin-Crossover Complexes in Direct Contact with Surfaces, Magnetochemistry, 2020, 6(3), 35 CrossRef CAS.
- D. Le, T. Jiang, M. Gakiya-Teruya, M. Shatruk and T. S. Rahman, On stabilizing spin crossover molecule [Fe(tBu2qsal)2] on suitable supports: insights from ab initio studies, J. Phys.: Condens. Matter, 2021, 33(38), 385201 CrossRef CAS PubMed.
- T. Jasper-Toennies, M. Gruber, S. Karan, H. Jacob, F. Tuczek and R. Berndt, Robust and Selective Switching of an FeIII Spin-Crossover Compound on Cu2N/Cu(100) with Memristance Behavior, Nano Lett., 2017, 17(11), 6613–6619 CrossRef CAS PubMed.
- T. G. Gopakumar, F. Matino, H. Naggert, A. Bannwarth, F. Tuczek and R. Berndt, Electron-Induced Spin Crossover of Single Molecules in a Bilayer on Gold, Angew. Chem., Int. Ed., 2012, 51(25), 6262–6266 CrossRef CAS PubMed.
- K. Bairagi, O. Iasco, A. Bellec, A. Kartsev, D. Li, J. Lagoute, C. Chacon, Y. Girard, S. Rousset and F. Miserque,
et al., Molecular-scale dynamics of light-induced spin cross-over in a two-dimensional layer, Nat. Commun., 2016, 7(1), 12212 CrossRef CAS PubMed.
- A. Köbke, F. Gutzeit, F. Röhricht, A. Schlimm, J. Grunwald, F. Tuczek, M. Studniarek, D. Longo, F. Choueikani and E. Otero,
et al., Reversible coordination-induced spin-state switching in complexes on metal surfaces, Nat. Nanotechnol., 2020, 15(1), 18–21 CrossRef.
- R. Sessoli, D. Gatteschi, A. Caneschi and M. A. Novak, Magnetic bistability in a metal-ion cluster, Nature, 1993, 365(6442), 141–143 CrossRef CAS.
- P. Román, C. Guzmán-Miralles, A. Luque, J. I. Beitia, J. Cano, F. Lloret, M. Julve and S. Alvarez, Influence of the Peripheral Ligand Atoms on the Exchange Interaction in Oxalato-Bridged Nickel(II) Complexes: An Orbital Model. Crystal Structures and Magnetic Properties of (H3dien)2[Ni2(ox)5]·12H2O and [Ni2(dien)2(H2O)2(ox)]Cl2, Inorg. Chem., 1996, 35(13), 3741–3751 CrossRef PubMed.
- R. J. Majeste and E. A. Meyers, Crystal and molecular structure of bisbipyridyl-.mu.-dihydroxo-dicopper(II) nitrate, J. Phys. Chem., 1970, 74(19), 3497–3500 CrossRef CAS.
- R. S. Berkley, Z. Hooshmand, T. Jiang, D. Le, A. F. Hebard and T. S. Rahman, Characteristics of Single-Molecule Magnet Dimers ([Mn3]2) on Graphene and h-BN, J. Phys. Chem. C, 2020, 124(51), 28186–28200 CrossRef CAS.
- D. Mitcov, A. H. Pedersen, M. Ceccato, R. M. Gelardi, T. Hassenkam, A. Konstantatos, A. Reinholdt, M. A. Sørensen, P. W. Thulstrup and M. G. Vinum,
et al., Molecular multifunctionality preservation upon surface deposition for a chiral single-molecule magnet, Chem. Sci., 2019, 10(10), 3065–3073, 10.1039/C8SC04917C.
- M. Mannini, F. Pineider, P. Sainctavit, C. Danieli, E. Otero, C. Sciancalepore, A. M. Talarico, M.-A. Arrio, A. Cornia and D. Gatteschi,
et al., Magnetic memory of a single-molecule quantum magnet wired to a gold surface, Nat. Mater., 2009, 8(3), 194–197 CrossRef CAS PubMed.
- L. Gragnaniello, F. Paschke, P. Erler, P. Schmitt, N. Barth, S. Simon, H. Brune, S. Rusponi and M. Fonin, Uniaxial 2D Superlattice of Fe4 Molecular Magnets on Graphene, Nano Lett., 2017, 17(12), 7177–7182 CrossRef CAS.
- V. Heß, F. Matthes, D. E. Bürgler, K. Y. Monakhov, C. Besson, P. Kögerler, A. Ghisolfi, P. Braunstein and C. M. Schneider, Adsorption phenomena of cubane-type tetranuclear Ni(II) complexes with neutral, thioether-functionalized ligands on Au(111), Surf. Sci., 2015, 641, 210–215 CrossRef.
- M. Mannini, P. Sainctavit, R. Sessoli, C. Cartier dit Moulin, F. Pineider, M.-A. Arrio, A. Cornia and D. Gatteschi, XAS and XMCD Investigation of Mn12 Monolayers on Gold, Chem. – Eur. J., 2008, 14(25), 7530–7535 CrossRef CAS PubMed.
- A. Saywell, A. J. Britton, N. Taleb, M. del Carmen Giménez-López, N. R. Champness, P. H. Beton and J. N. O'Shea, Single molecule magnets on a gold surface: in situ electrospray deposition, X-ray absorption and photoemission, Nanotechnology, 2011, 22(7), 075704 CrossRef PubMed.
- V. Lanzilotto, L. Malavolti, S. Ninova, I. Cimatti, L. Poggini, B. Cortigiani, M. Mannini, F. Totti, A. Cornia and R. Sessoli, The Challenge of Thermal Deposition of Coordination Compounds: Insight into the Case of an Fe4 Single Molecule Magnet, Chem. Mater., 2016, 28(21), 7693–7702 CrossRef CAS.
- A. Palii, S. Aldoshin, B. Tsukerblat, J. M. Clemente-Juan, A. Gaita-Ariño and E. Coronado, Electric field controllable magnetic coupling of localized spins mediated by itinerant electrons: a toy model, Phys. Chem. Chem. Phys., 2017, 19(38), 26098–26106, 10.1039/C7CP03872K.
- T. Saygun, J. Bylin, H. Hammar and J. Fransson, Voltage-Induced Switching Dynamics of a Coupled Spin Pair in a Molecular Junction, Nano Lett., 2016, 16(4), 2824–2829 CrossRef CAS PubMed.
- M. S. Leiva, S. A. Díaz and A. S. Nunez, Origin of the magnetoelectric couplings in the spin dynamics of molecular magnets, Phys. Rev. B, 2023, 107(9), 094401 CrossRef.
- S. Wagner, F. Kisslinger, S. Ballmann, F. Schramm, R. Chandrasekar, T. Bodenstein, O. Fuhr, D. Secker, K. Fink and M. Ruben,
et al., Switching of a coupled spin pair in a single-molecule junction, Nat. Nanotechnol., 2013, 8(8), 575–579 CrossRef CAS PubMed.
- C. J. Calzado, C. Angeli, D. Taratiel, R. Caballol and J.-P. Malrieu, Analysis of the magnetic coupling in binuclear systems. III. The role of the ligand to metal charge transfer excitations revisited, J. Chem. Phys., 2009, 131(4), 044327 CrossRef PubMed.
- D. Maganas, J. Krzystek, E. Ferentinos, A. M. Whyte, N. Robertson, V. Psycharis, A. Terzis, F. Neese and P. Kyritsis, Investigating Magnetostructural Correlations in the Pseudooctahedral trans-[NiII{(OPPh2)(EPPh2)N}2(sol)2] Complexes (E = S, Se; sol = DMF, THF) by Magnetometry, HFEPR, and ab Initio Quantum Chemistry, Inorg. Chem., 2012, 51(13), 7218–7231 CrossRef CAS PubMed.
- P. Sharma, D. G. Truhlar and L. Gagliardi, Magnetic Coupling in a Tris-hydroxo-Bridged Chromium Dimer Occurs through Ligand Mediated Superexchange in Conjunction with Through-Space Coupling, J. Am. Chem. Soc., 2020, 142(39), 16644–16650 CrossRef CAS PubMed.
- M.-A. Bouammali, N. Suaud, N. Guihéry and R. Maurice, Antisymmetric Exchange in a Real Copper Triangular Complex, Inorg. Chem., 2022, 61(31), 12138–12148 CrossRef CAS PubMed.
- Z. Sandoval-Olivares, E. Solis-Céspedes and D. Páez-Hernández, Antiferromagnetic Coupling Supported by Metallophilic Interactions: Theoretical View, Inorg. Chem., 2022, 61(3), 1401–1417 CrossRef CAS PubMed.
- A. Zhao, Q. Li, L. Chen, H. Xiang, W. Wang, S. Pan, B. Wang, X. Xiao, J. Yang and J. G. Hou,
et al., Controlling the Kondo Effect of an Adsorbed Magnetic Ion Through Its Chemical Bonding, Science, 2005, 309(5740), 1542 CrossRef CAS.
- X. Meng, J. Möller, M. Mansouri, D. Sánchez-Portal, A. Garcia-Lekue, A. Weismann, C. Li, R. Herges and R. Berndt, Controlling the Spin States of FeTBrPP on Au(111), ACS Nano, 2023, 17(2), 1268–1274 CrossRef CAS PubMed.
- X. Wang, L. Yang, L. Ye, X. Zheng and Y. Yan, Precise Control of Local Spin States in an Adsorbed Magnetic Molecule with an STM Tip: Theoretical Insights from First-Principles-Based Simulation, J. Phys. Chem. Lett., 2018, 9(9), 2418–2425 CrossRef CAS PubMed.
- N. Montenegro-Pohlhammer, R. Sánchez-de-Armas and C. J. Calzado, Deposition of the Spin Crossover FeII–Pyrazolylborate Complex on Au(111) Surface at the Molecular Level, Chem. – Eur. J., 2021, 27(2), 712–723 CrossRef CAS PubMed.
- R. Sánchez-de-Armas, N. Montenegro-Pohlhammer, A. Develioglu, E. Burzurí and C. J. Calzado, Spin-crossover complexes in nanoscale devices: main ingredients of the molecule–substrate interactions, Nanoscale, 2021, 13(44), 18702–18713, 10.1039/D1NR04577F.
- X. Wang, Q. Zhuang, P. Wu, L. Liu, F. Wang, X. Zhang, X. Li and X. Zheng, Tweezer-like magnetic tip control of the local spin state in the FeOEP/Pb(111) adsorption system: a preliminary exploration based on first-principles calculations, Nanoscale, 2023, 15(5), 2369–2376, 10.1039/D2NR04379C.
- R. Frisenda, G. D. Harzmann, J. A. Celis Gil, J. M. Thijssen, M. Mayor and H. S. J. van der Zant, Stretching-Induced Conductance Increase in a Spin-Crossover Molecule, Nano Lett., 2016, 16(8), 4733–4737 CrossRef CAS PubMed.
- A. C. Aragonès, D. Aravena, J. I. Cerdá, Z. Acís-Castillo, H. Li, J. A. Real, F. Sanz, J. Hihath, E. Ruiz and I. Díez-Pérez, Large Conductance Switching in a Single-Molecule Device through Room Temperature Spin-Dependent Transport, Nano Lett., 2016, 16(1), 218–226 CrossRef PubMed.
- D. Aravena and E. Ruiz, Coherent Transport through Spin-Crossover Single Molecules, J. Am. Chem. Soc., 2012, 134(2), 777–779 CrossRef CAS PubMed.
- E. Burzuri, A. Garcia-Fuente, V. Garcia-Suarez, K. Senthil Kumar, M. Ruben, J. Ferrer and H. S. J. van der Zant, Spin-state dependent conductance switching in single molecule-graphene junctions, Nanoscale, 2018, 10(17), 7905–7911 RSC.
- N. Montenegro-Pohlhammer, R. Sánchez-de-Armas, C. J. Calzado, M. Borges-Martínez and G. Cárdenas-Jirón, A photo-induced spin crossover based molecular switch and spin filter operating at room temperature, Dalton Trans., 2021, 50(19), 6578–6587, 10.1039/D1DT00078K.
- D. Li, Y. Tong, K. Bairagi, M. Kelai, Y. J. Dappe, J. Lagoute, Y. Girard, S. Rousset, V. Repain and C. Barreteau,
et al., Negative Differential Resistance in Spin-Crossover Molecular Devices, J. Phys. Chem. Lett., 2022, 13(32), 7514–7520 CrossRef CAS PubMed.
- J. M. Veauthier, W.-S. Cho, V. M. Lynch and J. L. Sessler, Calix[4]pyrrole Schiff Base Macrocycles. Novel Binucleating Ligands for μ-Oxo Iron Complexes, Inorg. Chem., 2004, 43(4), 1220–1228 CrossRef CAS PubMed.
- F. Neese, The ORCA program system, Wiley Interdiscip. Rev.: Comput. Mol. Sci., 2012, 2(1), 73–78 CAS.
- B. O. Roos, P. R. Taylor and P. E. M. Sigbahn, A complete active space SCF method (CASSCF) using a density matrix formulated super-CI approach, Chem. Phys., 1980, 48(2), 157–173 CrossRef CAS.
- C. Angeli, R. Cimiraglia and J.-P. Malrieu, N-electron valence state perturbation theory: a fast implementation of the strongly contracted variant, Chem. Phys. Lett., 2001, 350(3), 297–305 CrossRef CAS.
- F. Weigend and R. Ahlrichs, Balanced basis sets of split valence, triple zeta valence and quadruple zeta valence quality for H to Rn: Design and assessment of accuracy, Phys. Chem. Chem. Phys., 2005, 7(18), 3297–3305, 10.1039/B508541A.
- F. Neese, An improvement of the resolution of the identity approximation for the formation of the Coulomb matrix, J. Comput. Chem., 2003, 24(14), 1740–1747 CrossRef CAS PubMed.
- S. Smidstrup, T. Markussen, P. Vancraeyveld, J. Wellendorff, J. Schneider, T. Gunst, B. Verstichel, D. Stradi, P. A. Khomyakov and U. G. Vej-Hansen,
et al., QuantumATK: an integrated platform of electronic and atomic-scale modelling tools, J. Phys.: Condens.Matter, 2019, 32(1), 015901 CrossRef PubMed.
- M. J. van Setten, M. Giantomassi, E. Bousquet, M. J. Verstraete, D. R. Hamann, X. Gonze and G. M. Rignanese, The PseudoDojo: Training and grading a 85 element optimized norm-conserving pseudopotential table, Comput. Phys. Commun., 2018, 226, 39–54 CrossRef CAS.
- J. P. Perdew, K. Burke and M. Ernzerhof, Generalized Gradient Approximation Made Simple [Phys. Rev. Lett. 77, 3865 (1996)], Phys. Rev. Lett., 1997, 78(7), 1396–1396 CrossRef CAS.
- V. I. Anisimov, J. Zaanen and O. K. Andersen, Band theory and Mott insulators: Hubbard U instead of Stoner I, Phys. Rev. B: Condens. Matter Mater. Phys., 1991, 44(3), 943–954 CrossRef CAS PubMed.
- A. I. Liechtenstein, V. I. Anisimov and J. Zaanen, Density-functional theory and strong interactions: Orbital ordering in Mott-Hubbard insulators, Phys. Rev. B: Condens. Matter Mater. Phys., 1995, 52(8), R5467–R5470 CrossRef CAS PubMed.
- V. I. Anisimov, A. I. Poteryaev, M. A. Korotin, A. O. Anokhin and G. Kotliar, First-principles calculations of the electronic structure and spectra of strongly correlated systems: dynamical mean-field theory, J. Phys.: Condens. Matter, 1997, 9(35), 7359 CrossRef CAS.
- J. Taylor, H. Guo and J. Wang, Ab initio modeling of quantum transport properties of molecular electronic devices, Phys. Rev. B: Condens. Matter Mater. Phys., 2001, 63(24), 245407 CrossRef.
- H. Weihe and H. U. Güdel, Angular and Distance Dependence of the Magnetic Properties of Oxo-Bridged Iron(III) Dimers, J. Am. Chem. Soc., 1997, 119(28), 6539–6543 CrossRef CAS.
- R. N. Mukherjee, T. D. P. Stack and R. H. Holm, Angle dependence of the properties of the [Fe2X]4+ bridge unit (X = O, S): structures, antiferromagnetic coupling, and properties in solution, J. Am. Chem. Soc., 1988, 110(6), 1850–1861 CrossRef CAS.
- F. E. Mabbs, V. N. McLachlan, D. McFadden and A. T. McPhail, Magnetic properties and crystal and molecular structure of μ-oxo-bis[bis-(2-methyl-8-hydroxyquinolinato)iron(III)]–chloroform, J. Chem. Soc., Dalton Trans., 1973,(19), 2016–2021, 10.1039/DT9730002016.
- P. Coggon, A. T. McPhail, F. E. Mabbs and V. N. McLachlan, Crystal and molecular structure and magnetic anisotropy of μ-oxo-bis-[NN′-ethylenebis(salicylideneiminato)iron(III)]–dichloromethane, J. Chem. Soc. A, 1971, 1014–1019, 10.1039/J19710001014.
- J. T. Landrum, D. Grimmett, K. J. Haller, W. R. Scheidt and C. A. Reed, Imidazolate- and oxo-bridged metalloporphyrins, J. Am. Chem. Soc., 1981, 103(10), 2640–2650 CrossRef CAS.
- J. P. Malrieu, R. Caballol, C. J. Calzado, C. de Graaf and N. Guihery, Magnetic interactions in molecules and highly correlated materials: physical content, analytical derivation, and rigorous extraction of magnetic Hamiltonians, Chem. Rev., 2014, 114(1), 429–492 CrossRef CAS PubMed.
- J. Miralles, O. Castell, R. Caballol and J.-P. Malrieu, Specific CI calculation of energy differences: Transition energies and bond energies, Chem. Phys., 1993, 172(1), 33–43 CrossRef CAS.
- M. Spivak, C. Angeli, C. J. Calzado and C. de Graaf, Improving the calculation of magnetic coupling constants in MRPT methods, J. Comput. Chem., 2014, 35(23), 1665–1671 CrossRef CAS.
- C. Angeli and C. J. Calzado, The role of the magnetic orbitals in the calculation of the magnetic coupling constants from multireference perturbation theory methods, J. Chem. Phys., 2012, 137(3), 034104 CrossRef PubMed.
- P. J. Hay, J. C. Thibeault and R. Hoffmann, Orbital interactions in metal dimer complexes, J. Am. Chem. Soc., 1975, 97(17), 4884–4899 CrossRef CAS.
- E. Ruiz, P. Alemany, S. Alvarez and J. Cano, Toward the Prediction of Magnetic Coupling in Molecular Systems: Hydroxo- and Alkoxo-Bridged Cu(II) Binuclear Complexes, J. Am. Chem. Soc., 1997, 119(6), 1297–1303 CrossRef CAS.
- M. Fondo, J. Doejo, A. M. García-Deibe, J. Sanmartín-Matalobos, R. Vicente, M. S. El-Fallah, M. Amoza and E. Ruiz, Predetermined Ferromagnetic Coupling via Strict Control of M–O–M Angles, Inorg. Chem., 2016, 55(22), 11707–11715 CrossRef CAS.
- D. Venegas-Yazigi, D. Aravena, E. Spodine, E. Ruiz and S. Alvarez, Structural and electronic effects on the exchange interactions in dinuclear bis(phenoxo)-bridged copper(II) complexes, Coord. Chem. Rev., 2010, 254(17), 2086–2095 CrossRef CAS.
- N. Montenegro-Pohlhammer, D. Páez-Hernández, C. J. Calzado and R. Arratia-Pérez, A theoretical study of the super exchange mechanism and magneto-structural relationships in the [Mn(iii)2(μ-F)F4(Me3tacn)2](PF6) coordination compound, New J. Chem., 2018, 42(16), 13847–13855, 10.1039/C8NJ02793E.
- B. Pradines, B. Cahier, N. Suaud and N. Guihéry, Impact of the electric field on isotropic and anisotropic spin Hamiltonian parameters, J. Chem. Phys., 2022, 157(20), 204308 CrossRef CAS PubMed.
|
This journal is © The Royal Society of Chemistry 2024 |