DOI:
10.1039/D4DT00284A
(Paper)
Dalton Trans., 2024,
53, 4962-4967
(TeCl)4(TiCl4) with isolated Te4Cl16 and TiCl4 molecules and second-harmonic-generation†
Received
30th January 2024
, Accepted 16th February 2024
First published on 19th February 2024
Abstract
(TeCl4)4(TiCl4) is obtained by reaction of TeCl4 and TiCl4 at 50 °C with quantitative yield. The compound is composed of isolated, molecular (TeCl4)4 heterocubane-type units as well as isolated, molecular TiCl4 tetrahedra. The (TeCl4)4 heterocubane is arranged like a body-centred cubic cell with TiCl4 tetrahedra occupying 4 of 6 octahedral sites. (TeCl4)4(TiCl4) crystallizes in the space group I
with an unidirectional alignment of the tetrahedral building units. The structure of the compound is obtained from single crystal X-ray diffraction and confirmed by Rietveld refinement of powder diffraction data. Thermogravimetry, optical spectroscopy, infrared and Raman spectroscopy are employed to further characterize the title compound. Second harmonic generation (SHG) is observed with a strong intensity (1.6-times higher than potassium dihydrogen phosphate/KDP). The SHG effect is observed in the visible spectral regime as the band gap, derived from a Tauc plot, is 2.8 eV.
Introduction
Crystalline solid compounds composed of tetrahedral building units often show interesting material features such as nonlinear optical (NLO) properties.1 Quartz with (SiO4) tetrahedra is a well-known example showing piezoelectricity, circular dichroism and second harmonic generation (SHG).2 In particular, SHG materials are highly relevant for application and are widely used for frequency doubling of lasers (e.g., Nd:YAG: 1064 nm → 532 nm; Ti:sapphire: 800 nm → 400 nm).3 A key requirement for NLO properties is the absence of inversion symmetry, which – for tetrahedral arrangements – can occur due to an unidirectional orientation of the tetrahedral building units. While the SHG intensity is comparably weak in quartz, potassium dihydrogen phosphate (KDP) containing [H2PO4]− tetrahedra is known for its very strong SHG effect.4 Other examples include materials like iodates (e.g. Li2Ge(IO3)6), selenites (e.g. Bi3(SeO3)3(Se2O5)F), or tellurites (Ba(MoO2F)2(TeO3)2) with pseudo-tetrahedral [IO3]−, [SeO3]2−, or [TeO3]2− units.5 Beside the absence of inversion symmetry, further material properties determine the intensity of SHG signals and the usefulness of a compound for application. These include the magnitude of the band gap, whether phase matching is possible, the thermal stability, and a high damage threshold when irradiated with high-intensity laser light.6
In principle, tetrahedral arrangements can be easily realized by Lewis-acid–base reactions such as MX3 + M′X4 → [M′X3]+[MX4]− with a halogen transfer from M′ to M (M: element, M′: element with lone electron pair, X: halogen). Following such synthesis routes, several compounds have already been prepared. In many cases, however, the Lewis-acid–base reaction resulted in compounds crystallizing in space groups with inversion symmetry.7 Often inversion symmetry is observed if octahedral building units are formed in addition to a tetrahedral/pseudotetrahedral building unit (e.g. [SeCl3][SbCl6], [TeCl3][NbCl6]).8 Other compounds also crystallize in space groups without inversion symmetry, but potential NLO effects were not examined. Tetrahedral arrangements obtained via Lewis-acid–base reactions often offer several advantages such as: (i) simple synthesis with quantitative yield, (ii) straightforward crystallization and crystal growth, and (iii) a band gap (Eg) at the upper energy edge of the visible spectrum (3.5 eV ≥ Eg ≥ 2.5 eV). Based on the aforementioned considerations, we recently prepared compounds such as [SeCl3]+[GaCl4]−, [TeCl3]+[GaCl4]−,9 or the molecular Cl3SeOGaCl3,10 which show promising SHG intensities up to 10× KDP.
Aiming at a realization of novel tetrahedral arrangements with potential SHG effects via Lewis-acid–base reactions, here, we explored the reaction of TeCl4 and TiCl4. TeCl4 was expected to serve as a Lewis base and TiCl4 as a Lewis acid, hence leading to a formation of [TeCl3]+[TiCl5]− or [TeCl3]+2[TiCl6]2−. In contrast to our expectation, (TeCl4)4(TiCl4) was obtained with isolated molecular, heterocubane-type (TeCl4)4 tetramers as well as isolated molecular TiCl4 tetrahedra. (TeCl4)4(TiCl4) nevertheless crystallizes without inversion symmetry and shows a strong SHG effect with an intensity of about 1.6× KDP.
Results and discussion
Synthesis
(TeCl4)4(TiCl4) was prepared by reaction of TeCl4 and TiCl4 at 50 °C (Fig. 1). Here, the liquid TiCl4 also serves as the solvent (melting point TiCl4: −24 °C). After one week, colourless transparent crystals were obtained with quantitative yield. In contrast to our expectation, (TeCl4)4(TiCl4) was formed instead of the expected Lewis-acid–base adducts, such as [TeCl3]+[TiCl5]− or [TeCl3]+2[TiCl6]2−. Although the anion [TiCl6]2− is well-known (e.g. [NH4]2[TiCl6], Cs2[TiCl6]),11 sixfold coordination of Ti4+ by Cl− is obviously not preferred at our synthesis conditions and the Lewis acidity of TiCl4 is not high enough for Cl− subtraction from TeCl4. The grey colour of the product is noteworthy (Fig. 1). It originates from a small amount of elemental tellurium contained as an impurity in the as-supplied TeCl4. The purity of crystals of the title compound was validated by X-ray powder diffraction (XRD) with a Rietveld refinement of the diffraction data (Fig. 2), Fourier-transform infrared (FT-IR) spectroscopy, and thermogravimetry (TG) (see below). (TeCl4)4(TiCl4) is very sensitive to moisture and needs to be handled and stored under inert conditions.
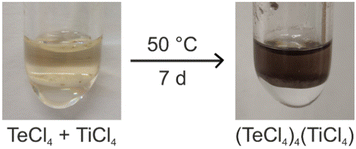 |
| Fig. 1 Scheme illustrating the synthesis of (TeCl4)4(TiCl4) (greyish colour of product due to small amounts of elemental tellurium). | |
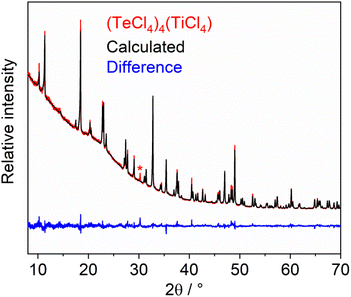 |
| Fig. 2 X-ray powder diffraction of (TeCl4)4(TiCl4) with Rietveld refinement using the data from single-crystal structure analysis as a model: experimental powder diffractogram (red), Rietveld refinement (black), and difference curve (blue) (*single Bragg reflection of elemental tellurium). | |
Structural characterization
According to X-ray structure analysis based on single crystals, (TeCl4)4(TiCl4) crystallizes in the non-centrosymmetric, tetragonal space group I
and consists of isolated, molecular (TeCl4)4 heterocubane-type units (Fig. 3a; ESI: Table S1, Fig. S1†) and isolated, molecular TiCl4 tetrahedra (Fig. 3b). X-ray powder diffraction with Rietveld refinement was used to confirm the crystal structure and space-group symmetry obtained by single-crystal structure analysis (Fig. 2; ESI: Table S2†). The absence of any center of inversion is clearly reflected by the uniformly oriented tetrahedral and heterocubane-type building units (Fig. 3c). The three-dimensional packing of the (TeCl4)4 heterocubanes and the TiCl4 tetrahedra can be illustrated by a reduced representation showing only the center of the respective building unit, i.e. only the center of the (TeCl4)4 heterocubane and only Ti for TiCl4 (ESI: Fig. S2†). Such a representation shows that the (TeCl4)4 heterocubane serves as packing unit of a body-centred cubic (bcc) cell. In this bcc cell, the TiCl4 tetrahedra occupy 4 of 6 octahedral sites.
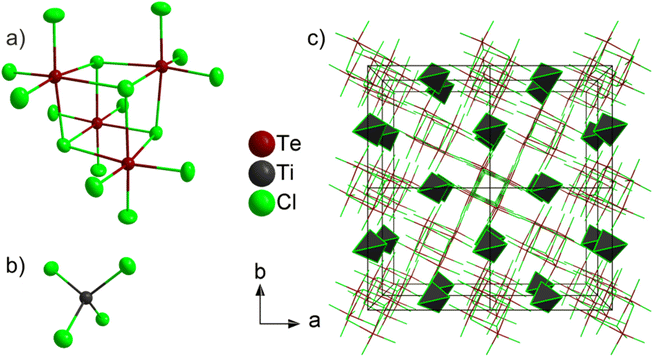 |
| Fig. 3 Crystal structure of (TeCl4)4(TiCl4): (a) isolated (TeCl4)4 heterocubane, (b) isolated TiCl4 tetrahedron; (c) (2 × 2 × 2) supercell showing the identical orientation of all TiCl4 tetrahedra and (TeCl4)4 heterocubanes. | |
Both molecular units in (TeCl4)4(TiCl4) – (TeCl4)4 heterocubanes and TiCl4 tetrahedra – retain structural similarities to the respective starting materials. Thus, the (TeCl4)4 heterocubane exhibits four edge-sharing (TeCl6) octahedra with Te–Cl distances (231.2(2)–295.4(1) pm) and Cl–Te–Cl angles (84.2(1)–95.0(1)°) similar to TeCl4 (Te–Cl: 229.6–295.9 pm, Cl–Te–Cl: 84.0–95.7°).12 Te–Cl distances with terminal Cl (231.2(2)–231.9(1) pm) are as expected significantly shorter than distances involving bridging Cl ligands (289.0(1)–295.4(1) pm). The Cl–Te–Cl angles indicate the slightly distorted octahedral coordination. The Ti–Cl distances (216.4(2) pm) and Cl–Ti–Cl angles (108.7(1)–109.9(1)°) are similar to TiCl4 (Ti–Cl: 216.3–216.5 pm, Cl–Ti–Cl: 108.9–109.9°).13 A mixed molecular compound and co-crystallization of TeCl4 and TiCl4, to the best of our knowledge, is observed here for the first time. An isolated (TeCl4)4 tetramer was not observed in a compound until now (except for pure TeCl4 itself). In addition, only anionic units such as [Te4Cl18]2− were reported before.14 Moreover, isolated molecular TiCl4 tetrahedra without further ligands were up to now only reported in combination with fullerenes (e.g. C60 × 3 TiCl4).15
Spectroscopic characterization
In addition to the X-ray diffraction experiments, the title compound was characterized by vibrational spectroscopy. Fourier-transform infrared (FT-IR) spectroscopy predominately shows an intense ν(Ti–Cl) vibration at 486 cm−1 (Fig. 4a), which is very similar in wavelength and intensity to that observed in TiCl4.16 Moreover, broad less characteristic bands occur at 1000–500 cm−1 and are mainly due to motions of TeCl4.17 The absence of ν(O–H) or ν(C–H) vibrations at 3500–2800 cm−1 confirms the purity of the title compound, which is especially important due to its high moisture-sensitivity. Raman spectra were also recorded (Fig. 4b). Here, the characteristic Raman vibrations of TeCl4 and TiCl4 were observed at 300–400 cm−1 and below 200 cm−1.18 DFPT calculations allow to determine the Eigen-vectors associated with the Raman bands (see ESI†). The two intense bands at 325 and 356 cm−1 are due to an asymmetric and a symmetric stretching vibration of the TeCl3 groups. Eigen-vectors of the low frequency bands involve motions of all constituents and cannot be easily classified.
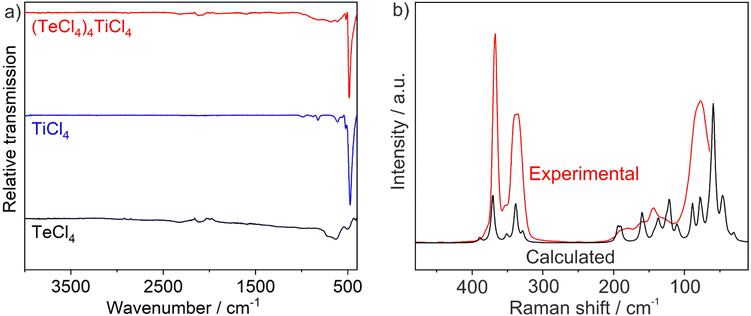 |
| Fig. 4 Vibrational spectroscopy of (TeCl4)4(TiCl4): (a) FT-IR spectra (with TeCl4 and TiCl4 as references), (b) experimental and calculated Raman spectrum. | |
The optical properties of (TeCl4)4(TiCl4) were analysed by UV-Vis spectroscopy (Fig. 5a). The title compound shows a strong absorption at 300–450 nm, which can be assigned to a valence-band to conduction-band transition. This absorption causes the pale yellow colour of crystals of the title compound. Using a Tauc plot, an indirect band gap of 2.8 eV was determined (Fig. 5b). Finally, the thermal properties of (TeCl4)4(TiCl4) were examined via thermogravimetry (ESI: Fig. S3†). Accordingly, (TeCl4)4(TiCl4) quantitatively decomposes into the binary compounds TiCl4 (sublimation at 100–150 °C) and TeCl4 (sublimation at 200–400 °C).
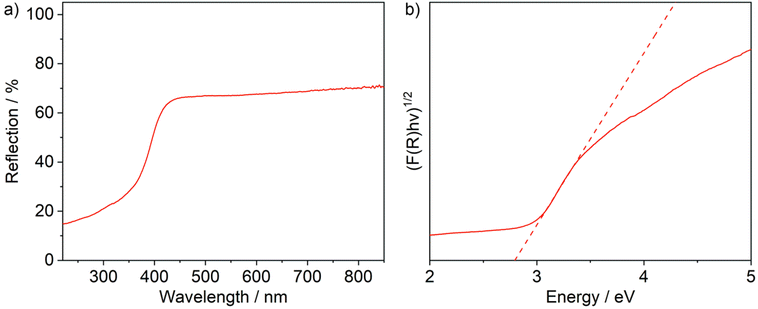 |
| Fig. 5 Optical properties of (TeCl4)4(TiCl4): (a) UV-Vis spectrum, (b) Tauc plot. | |
Second harmonic generation
As (TeCl4)4(TiCl4) crystallizes in the non-centrosymmetric space group I
, NLO effects such as SHG are to be expected, in principle.1,19 The NLO behaviour of the title compound was therefore examined with the Kurtz–Perry method.20 This method is well-suited for rapid characterization of new compounds as the analysis can be performed with microcrystalline powders. With crystallite sizes of 20 to 60 μm, moreover, the individual domains are large enough (>1 μm) to examine enantiomeric or twinned crystals. Due to the relationship between SHG intensity and grain size, the Kurtz–Perry method allows, in principle, to distinguish matchable and non-phase matchable materials. However, the averaged effective SHG coefficient derived from the experiment is associated with a large uncertainty as the grain-size distribution in a powder is difficult to quantify in moisture-sensitive materials, which cannot easily be sieved. Here, we used unsorted powder samples with grain sizes less than 25 μm, which were exposed to laser light at 1064 nm. The converted light was detected at 532 nm with the SHG intensities shown in Table 1.
Table 1 SHG intensities of (TeCl4)4(TiCl4) and specific reference samples
Sample |
Particle size (μm) |
SHG intensities (mV) |
Al2O3 |
9 |
0 (1) |
Quartz |
<5 |
1632 (248) |
5–25 |
3552 (407) |
KDP |
5–25 |
5528 (822) |
KDP |
25–50 |
8860 (1067) |
(TeCl4)4(TiCl4) |
<25 |
8632 (1104) |
As the Kurtz–Perry method does not result in absolute SHG intensities,20 the reference compounds quartz and KDP were examined under similar conditions. KDP is phase matchable (second-order susceptibility: d36 = 0.39 pm V−1) and can yield a SHG signal 5–10-times stronger than that of quartz (d11 = 0.3 pm V−1), which is non-phase-matchable, under optimal measurement conditions.21 In our experiments, however, we had to use a focused laser and could only sample a few small selected spots. Due to this approach, the intensity difference between the two reference samples is significantly reduced. In addition, corundum (α-Al2O3) was analysed as a reference with inversion symmetry and, thus, not showing any SHG effect. In comparison, finally, (TeCl4)4(TiCl4) shows a significant SHG signal, which is about 1.6-times higher than that of KDP (Table 1, Fig. 6).
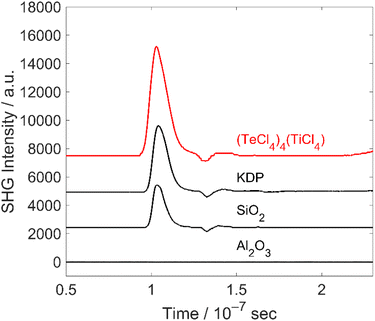 |
| Fig. 6 Representative SHG measurements of (TeCl4)4(TiCl4) and reference samples (quantitative assessment not possible due to limitations of the Kurtz–Perry approach). | |
Conclusions
Aiming at Lewis acid–base reactions to obtain novel compounds with tetrahedral arrangements without inversion symmetry to establish second harmonic generation (SHG), we have reacted TeCl4 and TiCl4 at 50 °C. Against our expectation, no Lewis acid–base reaction to a compound like [TeCl3]+[TiCl5]− or [TeCl3]+2[TiCl6]2− occurred. Instead, (TeCl4)4(TiCl4) was obtained with quantitative yield, which contains isolated, molecular (TeCl4)4 heterocubane-type units and isolated, molecular TiCl4 tetrahedra. Such molecular building units – isolated (TeCl4)4 tetramers and molecular TiCl4 tetrahedra – were rarely observed until now.
(TeCl4)4(TiCl4) crystallizes in the non-centrosymmetric space group I
with (TeCl4)4 heterocubanes arranged like in a body-centred cubic cell and with TiCl4 tetrahedra in 4 of the 6 octahedral sites. In accordance with the unidirectional alignment of the tetrahedral building units, (TeCl4)4(TiCl4) shows a good SHG intensity, which is even 1.6-times stronger than for potassium dihydrogen phosphate (KDP). The SHG effect determined via the Kurtz–Perry method is observed in the visible spectral regime of a narrow-band-gap compound (Eg: 2.8 eV). The compound shows that tetrahedral arrangements with non-linear optic (NLO) effects can be easily obtained in quantitative yield with simple reactions near room temperature (≤100 °C), which also offers many options for further reactions and compounds with promising optical properties.
Experimental section
General considerations
The starting materials TeCl4 (99% Sigma-Aldrich, Germany) and TiCl4 (99.9%, Sigma-Aldrich) were commercially available and used as received. All reactants were filled and stored in argon-filled glove-boxes (MBraun Unilab, Germany, O2/H2O < 1 ppm). The reactions were performed using standard Schlenk techniques and glass ampoules. All glass ware was evacuated three times to <10−3 mbar, heated, and flushed with argon to remove all moisture.
(TeCl4)4(TiCl4)
50 mg (0.19 mmol) of tellurium(IV) chloride and 102 μL (176.0 mg, 0.93 mmol) of titanium(IV) chloride were reacted under argon in a glass ampoule at 50 °C for one week. After cooling to room temperature with a rate of 1 K h−1, pale yellow crystals of (TeCl4)4(TiCl4) were obtained with quantitative yield.
X-ray data collection and structure solution
Selected single crystals of (TeCl4)4(TiCl4) were covered with inert oil (perfluorpolyalkylether, ABCR, Germany) and deposited on a microgripper (MiTeGen, USA). Data collection was performed at 213 K on an IPDS II image plate diffractometer (Stoe, Germany) using Mo-Kα radiation (λ = 71.073 pm, graphite monochromator). Data reduction and absorption correction were performed by the X-AREA software package (version 1.75, Stoe) and Stoe LANA (Version 1.63.1, Stoe).22 For structure solution and refinement, SHELXT and SHELXL were used.23 All atomic displacement parameters were refined anisotropically. Images were generated with DIAMOND.24 Further details related to the crystal structure may also be obtained from the joint CCDC/FIZ Karlsruhe deposition service on quoting the CSD-no. 2328371.
Second harmonic generation
Second harmonic generation (SHG) measurements were performed using the Kurtz–Perry approach20 on microcrystalline powder samples clamped between two glass slides in order to avoid any exposure to air. Quartz, Al2O3 and KH2PO4 (KDP) were used as reference materials. A Q-switched Nd:YAG laser (1064 nm, 5–6 ns, 2 kHz) was used for the generation of the fundamental pump wave. The fundamental infrared light was focused into the sample and the generated second harmonic (532 nm) was separated from 1064 nm using a harmonic separator, a short-pass filter, and an interference filter. The SHG signal was collected with a photomultiplier and an oscilloscope from eight different areas of the sample. On each position, 64 pulses were measured and averaged. Background signals between the laser pulses were used to correct the measured intensities. The SHG measurements were performed under ambient conditions in transmission geometry.
Raman spectroscopy
Raman measurements were carried out with a custom set-up in Frankfurt described in detail elsewhere.17 We used an OXXIUS S.A. Laser-Boxx LMX532 laser (λ = 532 nm) and a spectrograph (Princeton Instruments ACTON SpectraPro 2300i) equipped with a Pixis256E CCD camera. Measurements were performed in reflection geometry with the polarized laser light on the samples which were used for SHG measurements.
Conflicts of interest
There are no conflicts to declare.
Acknowledgements
M. A. B. and C. F. acknowledge the Deutsche Forschungsgemeinschaft (DFG) for funding within the project “Crown-Ether-Coordination-Compounds with Unusual Structural and Optical Properties/Crown I (FE 911/14-1)”. B. W. is grateful for support through the BIOVIA Science Ambassador program. L. B. gratefully acknowledge funding from the DFG (project Ba4020).
References
- E. Garmire, Opt. Express, 2013, 21, 30532–30544 CrossRef PubMed.
- C. J. Winta, S. Gewinner, W. Schöllkopf, M. Wolf and A. Paarmann, Phys. Rev. B, 2018, 97, 094108 CrossRef CAS.
-
R. W. Boyd, Nonlinear Optics, Academic Press, New York, 4th edn, 2020 Search PubMed.
- M. Jia, X. Cheng, M.-H. Whangbo, M. Hong and S. Deng, RSC Adv., 2020, 10, 26479 RSC.
-
(a) J. Chen, C. L. Hu, F. Kong and J. G. Mao, Acc. Chem. Res., 2021, 54, 2775–2783 CrossRef CAS PubMed;
(b) S. Shi, C. Lin, G. Yang, L. Cao, B. Li, T. Yan, M. Luo and N. Ye, Chem. Mater., 2020, 32, 7958–7964 CrossRef CAS;
(c) J. Y. Chung, H. Jo, S. Yeon, H. R. Byun, T.-S. You, J. I. Jang and K. M. Ok, Chem. Mater., 2020, 32, 7318–7326 CrossRef CAS;
(d) M.-L. Liang, Y.-X. Ma, C.-L. Hu, F. Kong and J.-G. Mao, Chem. Mater., 2020, 32, 9688–9695 CrossRef CAS.
- P. S. Halasyamani and W. Zhang, Inorg. Chem., 2017, 56, 12077–12085 CrossRef CAS PubMed.
-
(a) B. A. Stork-Blaisse and C. Romers, Acta Crystallogr., Sect. B: Struct. Crystallogr. Cryst. Chem., 1971, 27, 386–392 CrossRef CAS;
(b) V. B. Rybakov, L. A. Aslanov, S. V. Volkov, Z. A. Fokina and N. I. Timoshchenko, Zh. Neorg. Khim., 1991, 36, 2541–2548 CAS.
-
(a) B. Neumüller, C. Lau and K. Dehnicke, Z. Anorg. Allg. Chem., 1996, 622, 1847–1853 CrossRef;
(b) J. Beck and T. Schlörb, Z. Kristallogr., 1999, 214, 780–785 CrossRef CAS.
- M. A. Bonnin, L. Bayarjargal, V. Milman, B. Winkler and C. Feldmann, Inorg. Chem. Front., 2023, 10, 2636–2644 RSC.
- M. A. Bonnin, L. Bayarjargal, S. Wolf, V. Milman, B. Winkler and C. Feldmann, Inorg. Chem., 2021, 60, 15653–15658 CrossRef CAS PubMed.
-
(a) J. Wernet, Z. Anorg. Allg. Chem., 1953, 272, 279–287 CrossRef CAS;
(b) E. Wendling and J. De Lavillandre, Bull. Soc. Chim. Fr., 1967, 2142–2148 CAS.
- B. Buss and B. Krebs, Inorg. Chem., 1971, 10, 2795–2800 CrossRef CAS.
- A. Dawson, A. Parkin, S. Parsons, C. R. Pilham and A. L. C. Young, Acta Crystallogr., Sect. E: Struct. Rep. Online, 2002, 58, i95–i97 CrossRef CAS.
- A. Y. Makarov, I. Y. Bagryanskaya, Y. M. Volkova, M. M. Shakirov and A. V. Zibarev, Eur. J. Inorg. Chem., 2018, 1322–1332 CrossRef CAS.
- S. I. Troyanov and E. Kemnitz, Russ. J. Inorg. Chem., 2001, 46, 1547–1552 Search PubMed.
- N. J. Hawkins and D. R. Carpenter, J. Chem. Phys., 1955, 23, 1700–1702 CrossRef CAS.
- R. Ponsioen and D. J. Stufkens, Recl. Trav. Chim. Pays-Bas, 1971, 90, 521–5287 CrossRef.
-
(a) J. E. Griffiths, J. Chem. Phys., 1968, 49, 642–647 CrossRef CAS;
(b) N. S. Gonçalves and L. K. Noda, J. Mol. Struct., 2017, 1146, 750–754 CrossRef;
(c) A. Kovács and R. J. Konings, J. Mol. Struct., 1997, 410–411, 407–410 CrossRef.
- H. D. Flack, Helv. Chim. Acta, 2003, 86, 905–921 CrossRef CAS.
- S. Kurtz and T. Perry, J. Appl. Phys., 1968, 39, 3798–3813 CrossRef CAS.
- A. Ashkin, G. D. Boyd and D. A. Kleinman, Appl. Phys. Lett., 1965, 6, 179–180 CrossRef CAS.
-
X-RED, Data Reduction Program, Stoe, Darmstadt, Germany, 2001 Search PubMed.
- G. M. Sheldrick, Acta Crystallogr., Sect. A: Found. Adv., 2015, 71, 3–8 CrossRef PubMed.
-
DIAMOND, Crystal and Molecular Structure Visualization, Crystal Impact GbR, Bonn, Germany, 2016 Search PubMed.
|
This journal is © The Royal Society of Chemistry 2024 |