DOI:
10.1039/D3QI01634J
(Review Article)
Inorg. Chem. Front., 2023,
10, 6427-6439
The importance of second sphere interactions on single molecule magnet performance
Received
16th August 2023
, Accepted 22nd September 2023
First published on 23rd September 2023
Abstract
Owing to their potential applications in areas such as information storage, molecular spintronics, and quantum computing among others; the field of single molecule magnets (SMMs) has experienced a surge of interest and advances. One aspect of these systems that is often overlooked is the effect that second-sphere interactions can have on the magnetic performance and viability of SMMs. This review focuses on SMMs that display significant secondary interactions which influence magnetic performance, and highlights the importance of considering these interactions in the design of future SMMs.
Introduction
The discovery that single molecules were capable of retaining magnetisation outside of an applied magnetic field resulted in a burst of interest in the wider field of molecular magnetism. The first reported single molecule magnet (SMM) was the Mn12 cluster [MnIII8MnIV4(OAc)16 (H2O)4O12]·2AcOH·4H2O reported by Sessoli et al.,1 the structure of which was first reported in 1980 by Lis et al.2 Over the next 30 years, researchers have developed SMMs that featured a variety of different transition metals, lanthanides, and a combination of the two. One of the major factors driving research into SMMs and similar materials is their potential technological applications. Owing to their magnetic properties, researchers have proposed that SMMs can be utilised where traditional bulk ferromagnetic materials are currently being used, such as in information storage or processing. Furthermore, since SMMs are discrete molecular species, they have potential applications in advanced technologies such as molecular spintronics and quantum computing.3,4 However, several major hurdles must be overcome before these technological innovations can be implemented. The most significant being that the vast majority of SMMs only work at low temperatures, below the temperature of liquid nitrogen. This limits their viability in information storage or processing technologies as traditional components are able to operate in ambient conditions. Thus, a key focus for researchers is developing SMMs that can retain their magnetisation at ever higher temperatures.
SMMs are often defined by two main parameters: the blocking temperature (TB) and the effective energy barrier for reversal of magnetisation (Ueff).5–7 The blocking temperature TB, being the temperature below which magnetisation is retained, would be the most sensible parameter for determining SMM performance. However, the exact definition of TB often varies between groups with the more commonly utilised definitions being; the maximum temperature at which magnetic hysteresis is observed,8,9 (the temperature corresponding to the maximum zero-field AC susceptibility),10–13 and the temperature at which the relaxation time is equal to 100 seconds.14–16 Furthermore, the exact definition used for TB is not always present in publications, making comparisons of TB between studies difficult.17Ueff is typically an easier to measure parameter and, with respect to the studies covered within this minireview, is the more popular parameter for defining and comparing SMMs.18–20Ueff represents the minimum amount of energy required to overcome the barrier for reversal of magnetisation, and as such is the parameter most directly related to SMM performance. Ueff is obtained by fitting the temperature dependence of the relaxation time to an Arrhenius law.21–23 Thus, Ueff provides information about the exponential relaxation regime, which is dominated by the Orbach process.24,25 However, at lower temperatures significant deviation from the Arrhenius law can be observed, which is often attributed to direct processes and quantum tunnelling of magnetisation (QTM).26,27 Additionally, Raman relaxation and thermally-assisted QTM can operate over a wide temperature range. All this means that simply achieving a high Ueff will not necessarily lead to improved SMM performance.28–32
In conjunction with the typical magnetic analyses, DFT and other computational calculations are popular for interpreting and rationalising SMM behaviour.33,34 These computational methods tend to agree well with the experimental results whilst providing researchers a way to probe how small structural differences between SMMs can affect their magnetic properties.35
Initial attempts to enhance SMM performance were focused on maximising the total ‘spin’ of the system.36,37 However, it was later found that Ueff was independent of the total spin, but instead reliant on the magnetic anisotropy of the magnetic centre,36 thus, researchers focused their efforts on maximising the magnetic anisotropy. Lanthanide ions have large intrinsic single ion magnetic anisotropy, thus are excellent candidates as SMMs.38 The first lanthanide SMM was reported in 2003 by Ishikawa et al. and utilised a sandwich-type coordination where two phthalocyanine ligands are bonded to a central TbIII or DyIII.39 Fitting the experimental data to the Arrhenius law showed energy barriers of 230 K and 28 K respectively.
All elements possess an intrinsic electron distribution that is unique, for lanthanide ions these can be either oblate or prolate.40,41 Matching the ligand field to the type of ion (axial ligand field for oblate ions and equatorial ligand field for prolate anions) reduces electrostatic interactions between the lanthanide ion and the ligand, which maximises the anisotropy thereby enhancing SMM performance.19
A major factor limiting the performance of SMMs is the presence of through-barrier relaxation processes which remove the magnetisation without having to fully overcome the energy barrier, therefore minimising these processes is essential for developing high-end SMMs.42,43 One of the key routes in reducing QTM is by utilising exchange pathways to couple adjacent metal centres together. This can effectively reduce the probability of QTM by requiring the coupled metal centres to both simultaneously lose their magnetisation.44 Lanthanide ions do not possess strong pathways for this exchange coupling, but transition metal ions do. Thus, exchange coupled 3d–4f complexes are an attractive prospect for designing SMMs as this combines the intrinsic magnetism of lanthanide ions with the exchange pathways present in transition metal ions.45–50 Another route to reducing QTM is by controlling the symmetry of the complex. One of the major factors behind QTM in SMMs is the magnitude of transverse crystal fields (CFs). Designing SMMs with a strong ligand field and high symmetry coordination environments (such as C5h, D4hD5h, D4d and D6d) the CFs can be minimised, which in turn suppresses QTM.51–54
Second sphere interactions, or secondary interactions consist of interactions that are not directly involved in the primary coordination sphere. The two most common types of secondary interactions are steric effects and hydrogen bonding. Steric effects are due to electrostatic repulsion which results in molecules and atoms pushing neighbouring species away. Effective use of sterically bulky macrocyclic ligands has been explored by Tang et al., in their quest to elucidate the relaxation mechanisms of SMMs.55–58 These bulky ligands helped isolate the magnetic centres and reduce the CFs. With the addition of electron withdrawing substituents to the macrocycles Tang et al. were able to further weaken the equatorial crystal field, dramatically increasing SMM performance.59 Hydrogen bonding interactions result in an attraction between hydrogen bond donor and acceptor species. This effect is shared by similar interactions such as weaker halogen bonding and π–π stacking interactions.
Effects of secondary interactions
The effects of secondary interactions on SMMs are numerous and varied often involving the interplay of multiple types of interactions. Perhaps the most often mentioned secondary effect in the context of SMMs is the physical isolation of magnetic centres, which prevents interactions between adjacent magnetic centres, resulting in a reduction of the number of relaxation pathways present. This is most easily achieved by electrostatically driven processes where sterically bulky ligands push neighbouring molecules apart and is represented in Fig. 1(A). Similarly to this, utilising secondary interactions to impose rigidity on a complex can result in a decrease in molecular vibrations, which then decreases the number of relaxation pathways.60 Another subcategory of this involves magnetic dilution where the majority of magnetically active ions in a sample are replaced with diamagnetic ions.61 An effect utilising hydrogen bonding is shown in Fig. 1(B), where hydrogen bonds mediate exchange between adjacent metal centres. This can result in an increase or decrease in QTM or even the presence of SMM behaviour. An effect that is often the result of multiple secondary interactions is the reorientation of the magnetic axis of the complex as represented in Fig. 1(C). This is often, but not exclusively, a negative effect and is a result of secondary interactions altering the orientation of the anisotropy axes and the symmetry axis of a molecule. This phenomenon has been thoroughly explored by Sessoli et al., in their work on a series of Ln-DOTA complexes.62–65 The nuclearity of SMMs is a sterically dominated effect. By utilising ligands with similar coordination sites, but differing steric bulk, it is possible to control the number and orientation of magnetic ions present in the complex, as shown in Fig. 1(D). Finally, the electron density of the magnetic centres can be affected by secondary interactions. This effect is the broadest as it often involves the interplay of several secondary interactions and can be either positive or negative, as represented in Fig. 1(E) and (F) respectively, depending on whether the electron density is altered to enhance the intrinsic anisotropy of the magnetic centre or not.
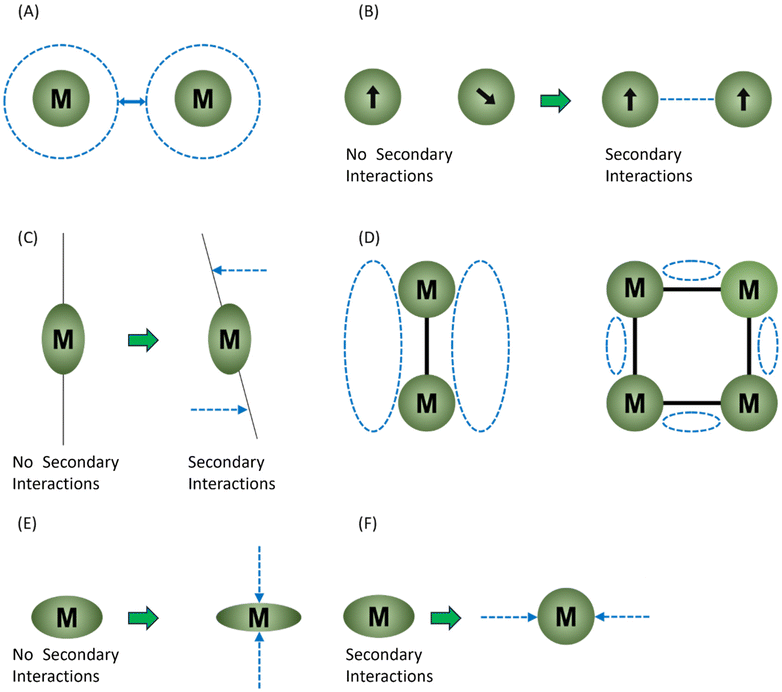 |
| Fig. 1 Representations of secondary effects (dashed lines represent the secondary interactions). (A) Isolation of adjacent magnetic centres. (B) Magnetic exchange mediated by hydrogen bonds. (C) Reorientation of the magnetic axis away from the ideal orientation. (D) Nuclearity of complexes can be determined by secondary interactions, predominantly electrostatic repulsion. (E) Alteration of electron density by secondary interactions into a more favourable distribution. (F) Alteration of electron density by secondary interactions into a less favourable distribution. | |
This mini-review provides a brief overview of cases where the effects of secondary interactions directly influence the magnetic properties of ligand metal systems and are a major focus of the study. The effects are divided into sections depending on how they affect SMM performance. Examples of SMMs displaying significant secondary interactions are highlighted and discussed with regard to the relevant secondary effects. A table listing the reported complexes and their magnetic properties is presented at the end of this article (Table 1).
Table 1 Table containing all the reported complexes in this review
No. |
Formula |
T
B (K) |
U
eff (K) |
Ref. |
|
Positive effects
|
|
|
|
1 |
[DyIII2(DMOMP)2(TFNB)4]·Et2O |
— |
38.9 |
66
|
2 |
[DyIII2(DMOAP)2(TFNB)4] |
— |
74.7 |
|
3 |
[DyIII2(DMOEP)2(TTA)4] |
— |
20.0 |
|
4 |
[DyIII2(DMOEP)2(BTFA)4] |
— |
33.6 |
|
5 |
[DyIII2(DMOEP)2(TFNB)4] |
— |
97.3 |
|
6 |
[CoII2(calix)2]·(Et3NH)2 |
— |
— |
67
|
7 |
[CoII (Himl)2]·CH3OH |
— |
— |
70
|
8 |
[CoII (Himn)2] |
— |
— |
|
9 |
[CoII (Hthp)2] |
— |
— |
|
10 |
[MnIII3O(Me-salox)3(MeOH)3(ClO4)]·MeOH |
— |
58 |
69
|
11 |
[MnIII3O(Ph-salox)3(MeOH)3(ClO4)]·2MeOH |
— |
42 |
|
12 |
[Mn12O12(O2C(C6H4-p-F))16(H2O)4] |
— |
59.3 |
72
|
13 |
[NiII(4-Clbpy)2][(pzTp)FeIII(CN)3]2·4H2O |
— |
— |
73
|
14 |
[NiII(4-Clbpy)2][(Tp*)FeIII(CN)3]2·4CH3OH·2H2O |
1.8 |
62.31 |
|
15 |
[DyIII(L1R)(4-Me-PhO)2](BPh4) |
— |
800 |
74
|
16 |
[DyIII(L1S)(4-Me-PhO)2](BPh4) |
— |
766.9 |
|
17 |
[DyIII2(L2R)2(4-Me-PhO)2(OH)2](BPh4)2 |
— |
198.6 |
|
18 |
[DyIII2(L2S)2(4-Me-PhO)2(OH)2](BPh4)2 |
— |
230.2 |
|
19 |
[LCuIIGdIII(NO3)3]·3.5THF |
— |
— |
75
|
20 |
[LCuIITbIII(NO3)3]·3.5THF |
— |
— |
|
21 |
[LCuIIDyIII(NO3)3]·3.5THF |
— |
— |
|
22 |
[(μ3-C9H3O6)(LCuIIGdIII-(NO3)2)3]·3THF |
— |
— |
|
23 |
[(μ3-C9H3O6)(LCuIITbIII-(NO3)2)3]·3THF |
— |
— |
|
24 |
[(μ3-C9H3O6)(LCuIIDyIII-(NO3)2)3]·3THF |
— |
— |
|
25 |
[DyIII(tmpd)3(4,4′-dmpy)] |
— |
66 |
79
|
26 |
[DyIII(tffb)3(4,4′-dmpy)] |
— |
189 |
|
27 |
[DyIII(tffb)3(5,5′-dmpy)] |
— |
115 |
|
28 |
[DyIII(tmpd)3(5,5′-dmpy)] |
— |
205 |
|
29 |
[CuIIL(H2O)Gd(NO3)2(H2O)2]·NO3 |
— |
— |
80
|
30 |
[CuIIL(MeOH)Tb(NO3)3] |
— |
24.6 |
|
31 |
[CuIIL(MeOH)Dy(NO3)3] |
— |
— |
|
32 |
[CuIIL(H2O)Dy(NO3)3] |
— |
— |
|
|
Negative effects
|
|
|
|
33 |
[DyIII(H2O)5(HMPA)2]Cl3·HMPA·H2O |
∼7 |
460 |
81
|
34 |
[DyIII(H2O)5(HMPA)2]I3·2HMPA |
∼7 |
600 |
|
35 |
([DyIII(L)2(H2O)4]·(6Br))n |
— |
— |
84
|
36 |
([GdIII(L)2(H2O)4]·(6Br))n |
— |
— |
|
37 |
([LaIII(L)2(H2O)4]·(6Br))n |
— |
— |
|
38 |
[((PyPz3)CoII)2(DHBQ)](PF6)2 |
— |
117 |
85
|
39 |
[((PyPz3)CoII)2(CA)](PF6)2 |
— |
40.3 |
|
40 |
[((PyPz3)CoII)2(BA)](PF6)2 |
— |
33.1 |
|
41 |
[DyIII((−)/(+)hfc)3(L)]2·C7H16 |
4 |
— |
86
|
42 |
[[DyIII((−)/(+)hfc)2(L)][BarF]]n·nCH3NO2 |
— |
— |
|
43 |
[DyIII(N-NCS)3(H2O)5]·0.45(KSCN)·(18-crown-6) |
— |
47 |
87
|
44 |
[DyIII(NO3)2(N-NCS)3(H2O)]·(H2O)(NH4)2·2(18-crown-6) |
— |
65.9 |
|
45 |
[DyIII(NO3)3(H2O)3]·(18-crown-6) |
— |
66–71 |
|
46 |
[ErIII(NO3)3(H2O)3]·(18-crown-6) |
— |
21–24 |
|
|
Other effects
|
|
|
|
47 |
[NdIIIL12][Et3NH]·THF |
— |
— |
90
|
48 |
[TbIIIL12][Et3NH]·THF |
— |
— |
|
49 |
[DyIIIL12][Et3NH]·THF |
— |
13.45 |
|
50 |
[NdIIIL22][Et3NH]·THF/H2O |
— |
— |
|
51 |
[TbIIIL22][Et3NH]·THF/H2O |
— |
— |
|
52 |
([Dy(OAc)3(H2O)3][Dy(H2O)3(prop·SMe)3][H2O⊂Cr3Dy6(OAc)12(bda)3(gly)3(ox)3(prop·SMe)3]2(H2O))·12H2O·1.5MeCN |
— |
11.9 |
91
|
53 |
([Tb(OAc)3(H2O)3][Tb(H2O)3(prop·SMe)3][H2O⊂Cr3Tb6(OAc)12(bda)3(gly)3(ox)3(prop·SMe)3]2(H2O))·11.25H2O·1.5MeCN |
— |
4.9 |
|
Positive effects
There have been numerous cases where secondary interactions have been reported to enhance or even cause SMM behaviour.
Magnetic isolation
The most noted benefit of secondary interactions with regards to SMMs, is their ability to isolate the magnetic core, resulting in a decrease of the potential pathways for the relaxation of magnetisation. A prime demonstration of this is presented by Liu and co-workers who reported on a series of five binuclear dysprosium complexes featuring substituents with differing electronic and steric environments.66 They synthesised the complexes, [Dy2(DMOMP)2(TFNB)4]·Et2O (1), [Dy2(DMOAP)2(TFNB)4] (2), [Dy2(DMOEP)2(TTA)4] (3), [Dy2(DMOEP)2(BTFA)4] (4) and [Dy2(DMOEP)2(TFNB)4] (5), where H-DMOMP = 2,6-dimethoxy-4-methylphenol, H-DMOAP = 3,5-dimethoxy-4-hydroxybenzaldehyde, H-DMOEP = methyl 3,5-dimethoxy-4-hydroxybenzoate, TTA = 2-thenoyltrifluoro-acetone, BTFA = benzoyltrifluoroacetone, and TFNB = 4,4,4-trifluoro-1-(2-naphthyl)-1,3-butane-dione. The reported Ueff values of the complexes under the optimal applied field are 38.9 K, 74.7 K, 20.0 K, 33.6 K and 97.3 K for complexes (1), (2), (3), (4) and (5) respectively. The structures of complexes (3), (4) and (5) are identical except for the terminal substituents of the β-diketonate. Liu et al. deduced that the vast difference in Ueff was due to the amount of conjugation and steric hindrance increasing from thiophene (3) to benzene (4) to naphthalene (5) in (Fig. 2).
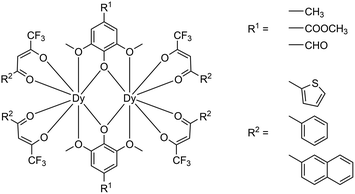 |
| Fig. 2 General structure of complexes reported by Liu et al. (1), R1 = CH3 R2 = naphthalene, (2), R1 = CHO R2 = naphthalene, (3), R1 = COOCH3 R2 = thiophene, (4), R1 = COOCH3 R2 = benzene, (5), R1 = COOCH3 R2 = naphthalene. | |
A similar phenomenon of magnetic isolation was explored by Petit et al. who synthesised the dinuclear cobalt complex [CoII2(calix)2]·(Et3NH)2 (6), where calix = p-tert-butylcalix[8]arene (Fig. 3).67 This study focused on identifying the role the second coordination sphere plays in quantifying the zero-field splitting (ZFS) on the cobalt sites. Through ab initio calculations the authors deduced that the effect of the second coordination sphere on the ZFS of the CoII ions was twice that of the first coordination sphere. Additionally, it was discovered that half of the magnetic anisotropy of the CoII ions is a result of the symmetry lowering effects of the calix[8]arene in the second coordination sphere.
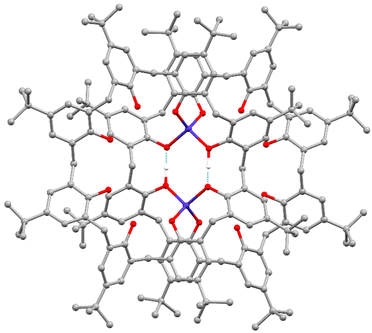 |
| Fig. 3 Structure of the complex (6) reported by Petit et al. Counterions and non-interacting hydrogen atoms have been omitted for clarity. Colour code: Co, O, C and H are purple, red, grey and white respectively. Thermal ellipsoids of metal atoms shown at 30%. | |
Magnetic exchange mediation
The most direct way that secondary interactions can impact SMM performance is by mediating exchange coupling adjacent metal ions. This is most commonly achieved through hydrogen-bonding which is used to couple adjacent metal centres in a manner similar to magnetic superexchange.68 This can create an exchange bias which can alter QTM.69
The direct implications of hydrogen bonding on the magnetic relaxation dynamics of SMMs was investigated by Mitsuhashi et al.70,71 who synthesised a series of three tetracoordinated mononuclear cobalt complexes, [Co(iml)2]·CH3OH (7), [Co(imn)2] (8) and [Co(thp)2] (9), where H-iml = 2-(2-imidazolyl)phenol, H-imn = 2-(2-imidazolinyl)phenol, and H-thp = 2-(1,4,5,6-tetrahydropyrimidin-2-yl)phenol.70 These three complexes differ by slight alterations in the conformation of the N–H groups. They found that the static magnetic properties of the complexes were comparable with axial zero field splitting parameters (D) of −42(11), −38(3), and −35(24) cm−1 for (7), (8), and (9), respectively. The dynamic magnetic properties revealed that only two of the complexes displayed frequency dependent in phase (χ′) and out of phase (χ′′) signals between 1.9–8 K and 1.9–3.5 K for (8) and (9), respectively. Mitsuhashi et al. identified two reasons for this, firstly, (7) formed a two-dimensional hydrogen bonding network whilst (8) and (9) formed one-dimensional chains. The inter-sheet Co–Co distance in (7) (7.51 Å) is much shorter than the interchain distances in (8) and (9) (≥ca. 9 Å). Mitsuhashi et al. surmised that since each CoII ion is surrounded by more magnetic ions, dipolar interactions can enhance the QTM resulting in reduced SMM performance. Secondly the closest Co⋯Co distance between two adjacent molecules for (7) is 7.03 Å whilst it is 6.05 Å and 6.36 Å for (8) and (9), respectively. Thus, the hydrogen-bonded chains of (8) and (9) can be regarded as a chain of dimers, in which the magnetic exchange coupling between SMMs works to suppress QTM.
This same phenomenon was explored by Yang et al. who synthesised two trinuclear manganese complexes [Mn3O(Me-salox)3(MeOH)3(ClO4)]·MeOH (10) and [Mn3O(Ph-salox)3(MeOH)3(ClO4)]·2MeOH (11) where HMe-salox = 2-hydroxyphenylethanone oxime and HPh-salox = (2-hydroxyphenyl)(phenyl)methanone oxime.69 Magnetic measurements revealed that both complexes displayed frequency dependent in phase (χ′) and out of phase (χ′′) signals between 2 K and 7 K, with (10) showing an effective energy barrier of 58 K, whilst complex (11) had an effective energy barrier of 42 K. An interesting difference was found in the stepwise magnetisation hysteresis loops of the complexes where the QTM steps of (11) were observed at fields of −0.52 T, −0.17 T, 0.16 T, 0.52 T, 0.87 T, 1.21 T, and 1.67 T, whereas those for complex (12) were observed at fields of 0 T and 0.7 T. This difference was attributed to the supramolecular environments each individual complex resides in. The less bulky complex (10) conforms to a ‘tail to tail’ arrangement of adjacent units which provides strong hydrogen bonding interactions between the binding ligand and a terminal MeOH ligand of neighbouring units (Fig. 4). Complex (11) meanwhile, adopts a ‘head to tail’ arrangement providing a single hydrogen bonding interaction between an uncoordinated perchlorate anion and a terminal MeOH ligand. The presence of the strong hydrogen bonding interaction in complex (10) mediated a pathway for the exchange bias effect which in turn caused the QTM steps in the hysteresis loop.
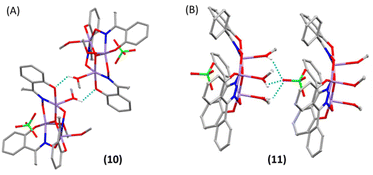 |
| Fig. 4 Structure of the two complexes reported by Yang et al. With the tail–tail arrangement (A) and the head to tail arrangement (B). Intermolecular interactions are shown by dashed cyan lines. Solvent molecules and non-interacting hydrogens have been omitted for clarity. Colour code: Mn, O, N, C, Cl, and H are lilac, red, blue, grey, green, and white, respectively. Thermal ellipsoids shown at 15%. | |
Fournet et al. investigated the hydrogen bond mediated magnetic coupling of a new member of the Mn12 family [Mn12O12(O2C(C6H4-p-F))16(H2O)4] (12) which was the first of its family to display a three-dimensional ferromagnetic network.72 Magnetic measurements of the complex revealed a Ueff of 59.3 K which is typical for the Mn12 family. There are two main types of intermolecular hydrogen bonds, one involving ortho-C–H⋯F units the other involving meta-C–H⋯F units. Fournet et al. further explained that due to the symmetry and spins in the relevant manganese and fluorine orbitals, exchange interactions mediated by the hydrogen bonds were predominantly ferromagnetic for the ortho units and antiferromagnetic for the meta units. The overall result is a net ferromagnetic interaction due to the greater localisation of spin onto the ortho position.
Control over complex nuclearity
A less direct way that second sphere interactions can impact SMM performance is by controlling the nuclearity of resulting complexes. This is often achieved by utilising ligands that provide identical metal binding sites but different steric bulk. In this vein, Jiao et al. reported two complexes in 2018 which showed intramolecular ferromagnetic interactions.73 A trinuclear [NiII(4-Clbpy)2][(pzTp)FeIII(CN)3]2·4H2O complex (13) and a tetranuclear [NiII(4-Clbpy)2][(Tp*)FeIII(CN)3]2·4CH3OH·2H2O (14) where Clbpy = 4,4′-dichloro-2,2′-bipyridine, pzTp = tetrakis(pyrazolyl)borate, and Tp* = hydrotris-(3,5-dimethylpyrazol-1-yl)borate. They proposed that the interaction between the bulky Tp* units and the 4-Clbpy ligand resulted in the formation of a tetranuclear square complex. Magnetic studies performed on these two complexes revealed that the trinuclear complex (13) showed no SMM behaviour, however, the tetranuclear complex (14) displayed frequency dependent in phase (χ′) and out of phase (χ′′) signals between 1.9 K and 5 K, and an effective energy barrier of 62.31 K. This difference was put down to three factors: the larger ground spin state owing to the addition of an extra NiII ion, weaker intermolecular interactions removing pathways for magnetic relaxation, and stronger intermolecular ferromagnetic interactions due to the difference in Ni–N–C bond angles.
Zhao and co-workers utilised two chiral pairs of weakly coordinating hexaazamacrocycles, one based on (1R,2R/1S,2S)-1,2-diphenylethylenediamine, and the other, on (1R,2R/1S,2S)-1,2-diaminocyclohexane in their report investigating the effects of steric hindrance on the performance of dysprosium single molecule magnets (Fig. 5).74 The mononuclear complexes were synthesised by using the bulkier 1,2-diphenylethylenediamine based hexaazamacrocycle which resulted in two complexes (15) and (16) displaying SMM behaviour with Ueff values of 800.0 K and 766.9 K, respectively. Two dinuclear complexes (17) and (18) which displayed SMM behaviour were synthesised using the less sterically bulky 1,2-diaminocyclohexane based macrocycle. These complexes gave Ueff values of 198.6 K and 230.2 K, respectively. The reason for the large difference between energy barriers was put down to the geometry of the magnetic cores. The mononuclear DyIII ions possess an almost perfect local D6h geometry which enhances the axial crystal field. In contrast, the dinuclear DyIII ions possess a ‘hula-hoop’ geometry which weakens the axial crystal field, thereby reducing Ueff.
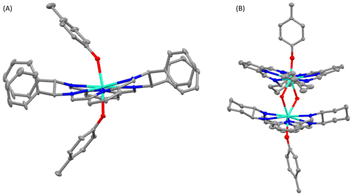 |
| Fig. 5 Structures of the mononuclear (A, 15/16) and dinuclear (B, 17/18) complexes reported by Zhao et al. Hydrogen atoms have been omitted for clarity. Colour code: Dy, O, N, and C are cyan, red, blue, and grey, respectively. Thermal ellipsoids shown at 15%. | |
Reorientation of magnetic axis
An interesting way that secondary interactions have been shown to affect the magnetic properties of SMMs is by reorienting the magnetic axes. An example where this was beneficial is reported by Novitchi et al. The group developed two series of 3d–4f SMMs [L4CuGd(NO3)3]·3.5THF (19), [L4CuTb(NO3)3]·3.5THF (20), and [L4CuDy(NO3)3]·3.5THF (21) [(μ3-C9H3O6)(L4CuGd-(NO3)2)3]·3THF (22), [(μ3-C9H3O6)(L4CuTb-(NO3)2)3]·3THF (23), and [(μ3-C9H3O6)(LCuDy-(NO3)2)3]·3THF (24). Where H2L4 = N,N′-bis(3-hydroxymethyl-5-methylsalicylidene)-1,3-diaminopropane.75 The magnetic properties of the dysprosium complexes, (21) and (24), showed that the dinuclear complex (21) displayed magnetic hysteresis curves of low coercivity, whilst the hexanuclear cluster (24) was much larger; coercivity being a measure of how resistant the complex is to becoming demagnetised by an external magnetic field. Furthermore, the hysteresis curves of (21) were only open at 0.04 K whilst for (24) the hysteresis curves were open at temperatures up to 1.1 K. Previous research done on similar complexes suggested that this difference was not a result of intramolecular magnetic interactions, since these were expected to be negligible.76–78 Novitchi and co-workers deduced that intermolecular π–π stacking and hydrogen bonding interactions between two hexanuclear clusters resulted in a reorientation of the anisotropy axes of the lanthanide ions (Fig. 6). This in turn caused the large change in SMM behaviour.
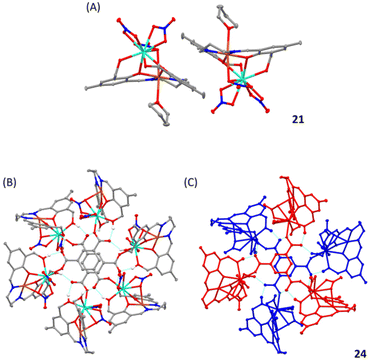 |
| Fig. 6 Structures of two molecules of the dinuclear (A) and hexanuclear (B and C) dysprosium complexes reported by Novitchi et al. Solvent molecules and non-interacting hydrogen atoms have been omitted for clarity. Hydrogen bonding interactions are shown by dashed cyan lines. Colour code: Dy, Cu, O, N, C, and H are cyan, orange, red, blue, grey, and white, respectively. Thermal ellipsoids shown at 15%. | |
Changing electron density
Recent advances in SMM research have revealed that matching the electron density of the magnetic ion with an appropriate ligand field is essential to enhancing the magnetic properties of SMMs, especially those that are lanthanide based. A key example of this was shown by Zhang et al. who developed a series of four mononuclear dysprosium complexes [Dy(tmpd)3(4,4′-dmpy)] (25), [Dy(tffb)3(4,4′-dmpy)] (26), [Dy(tffb)3(5,5′-dmpy)] (27), and [Dy(tmpd)3(5,5′-dmpy)] (28), where tmpd = 4,4,4-trifluoro-1-(4-methoxyphenyl)-1,3-butanedione, tffb = 4,4,4-trifluoro-1-(4-fluorophenyl)-1,3-butanedione, 4,4′-dmpy = 4,4′-dimethyl-2,2′-bipyridyl, and 5,5′-dmpy = 5,5′-dimethyl-2,2′-bipyridyl.79 Magnetic measurements on these complexes revealed Ueff values of 66 K (25), 189 K (26), 115 K (27), and 205 K (28). Experimental and theoretical investigations revealed that the symmetry of the electron density distribution surrounding the central DyIII ion is critical in regulating the slow relaxation of magnetisation. It was found that complex (28) had the strongest axial ligand field, which when coupled with the DyIII ion's naturally oblate electron density resulted in the complex with the highest energy barrier to reversal of magnetisation. Furthermore, the lowest energy barrier was displayed by complex (25), which was found to have the shortest intermolecular Dy⋯Dy separation because of the presence of strong π–π stacking interactions. This resulted in stronger dipolar interactions between the molecules which, in this case, increased QTM and thus a lower energy barrier for reversal of magnetisation.
Fellah et al. investigated the effects a non-coordinating alcohol group had on the magnetic properties of a series of four binuclear CuLn complexes, [CuL1(H2O)Gd(NO3)2(H2O)2]·NO3 (29), [CuL1(MeOH)Tb(NO3)3] (30), [CuL1(MeOH)Dy(NO3)3] (31), and [CuL1(H2O)Dy(NO3)3] (32) where H2L1 = 1,3-bis(2-hydroxy-3-methoxybenzylidene)propan-2-ol.80 Of these complexes, only (30) displayed SMM properties with an Ueff barrier of 24.6 K. In all complexes, the alcohol group acts as a hydrogen bond donor for intermolecular interactions and a hydrogen bond acceptor for intramolecular interactions (Fig. 7). The researchers noted that only complexes (30) and (31), which contained a methanol molecule bound to the copper, displayed slow relaxation of magnetisation. Furthermore, they postulated that the intramolecular hydrogen bonding between the non-coordinated alcohol group and the apical ligand (H2O or MeOH) could help stabilise the coordination with respect to the lability of this ligand. This in turn directly affected the magnetic properties of the reported complexes.
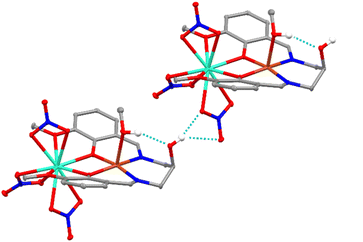 |
| Fig. 7 Structure of the terbium complex (30) reported by Fellah et al. Hydrogen bonding interactions are shown by dashed cyan lines. Non-interacting hydrogen atoms have been omitted for clarity. Colour code: Tb, Cu, O, N, C, and H are cyan, orange, red, blue, grey, and white respectively. Thermal ellipsoids shown at 15%. | |
Negative effects
In this section the impact secondary interactions can have to diminish or prevent SMM behaviour will be discussed. As one might expect, the negative effects of secondary interactions are far less commonly reported, with even fewer being the focus of the reported research.
Changing electron density
Secondary interactions which alter electron density on the magnetic centre can also quench the potential magnetic properties. This happens by either providing various relaxation pathways for the reversal of magnetisation, most notably QTM; or, by reducing the magnetic anisotropy of the metal ion, reducing SMM performance. This effect was thoroughly investigated by Canaj and co-workers who synthesised two dysprosium SMMs [Dy(H2O)5(HMPA)2]3Cl·HMPA·H2O (33) and [Dy(H2O)5(HMPA)2]3I·2HMPA (34), HMPA = hexamethylphosphoramide (Fig. 8).81 Magnetic studies revealed that the energy barrier for the reversal of magnetisation was 460 K (33) and 600 K (34). This difference was attributed to the large computed LoProp (local properties)82 charge of the chloride anion compared to the iodide anion. In practice, this resulted in a larger equatorial electron density for (33) which for a DyIII ion results in a larger transverse ligand field and a resultant increase in QTM. Furthermore, they performed ab initio calculations to identify what effect removing anions/molecules in the second coordination sphere had on the magnetic properties of the complex. The result was an increase in the calculated energy barrier for reversal of magnetisation Ucal to a maximum of approximately 3100 K for the two-coordinate [Dy(HMPA)2]3+ model. This drastic increase was a result of the decreasing equatorial electron density and the increase in the axial crystal field as they moved to lower coordinate models.
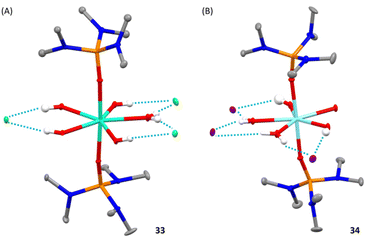 |
| Fig. 8 Diagram of the chlorine containing complex (A) and the iodine containing complex (B) reported by Canaj et al. Solvent molecules and non-interacting hydrogens have been omitted for clarity. Hydrogen bonding interactions are shown as dashed red lines. Colour code: Dy, O, N, C, P, Cl, I, and H are cyan, red, blue, grey, orange, green, violet, and white, respectively. Thermal ellipsoids shown at 15%. | |
A more conclusive example of the detrimental effects of changing the electron density was studied by Ramakant et al. who developed a series of lanthanide based 1D polymers ([Dy(L2)2(H2O)4]·6Br)n (35), ([Gd(L2)2(H2O)4]·6Br)n (36), and ([La(L2)2(H2O)4]·6Br)n (37), where L2 = 3,3′,3′′-((2,4,6-trimethylbenzene-1,3,5-triyl)tris(methylene))tris(1-(carboxymethyl)-benzimidazolium).83 They found that (35) displayed some SMM behaviours whilst (36) and (37) did not, which is not unreasonable since LaIII is diamagnetic and GdIII is typically magnetically isotropic. The observed out-of-phase ac magnetic susceptibility (χ′′) measurements on (35) showed frequency dependence between 1.9 K and 4.2 K however, there was no clear (χ′′) maxima within the experimental temperature and frequency ranges. This absence was a result of fast QTM bypassing the energy barrier. Through theoretical calculations they reasoned that hydrogen bonding interactions between bound water molecules and the bromine anions resulted in an increase in equatorial electron density, which resulted in an unfavourable crystal field and thus negatively affected the slow relaxation of magnetisation.
A case where the magnetic anisotropy of the metal centres was altered is reported by Yao et al. who investigated the effect of substituent size in a series of three dinuclear cobalt(II) SMMs: [((PyPz3)Co)2(DHBQ)](PF6)2 (38), [((PyPz3)Co)2(CA)](PF6)2 (39) and [((PyPz3)Co)2(BA)](PF6)2 (40) (Fig. 9) where PyPz3 = 2-(di(1H-pyrazol-1-yl)methyl)-6-(1H-pyrazol-1-yl)pyridine, dhbq = 2,5-dioxo-1,4-benzoquinone, CA = chloranilate and BA = bromanilate.84 Magnetic measurements revealed the energy barriers for reversal of magnetization to be 117 K (38), 40.3 K (39), and 33.1 K (40). Analysis of the X-ray data revealed that the cobalt centres adopted a distorted trigonal prismatic geometry with this distortion becoming more pronounced with the larger substituents, (38) → (39) → (40) in ascending order. The corresponding magnetic analysis revealed that the more structurally distorted the complex, the lower the effective energy barrier. This was ascribed to the greater distortions increasing the rhombic anisotropy, which promoted QTM mechanisms.
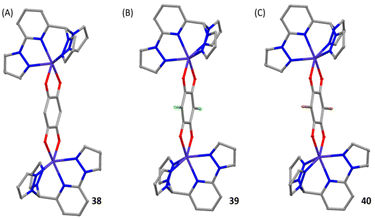 |
| Fig. 9 Structures of the three complexes reported by Yao et al. Counterions and hydrogen atoms have been omitted for clarity. Colour code: Co, O, N, C, Cl, and Br are purple, red, blue, grey, green, and orange, respectively. Thermal ellipsoids shown at 15%. | |
Douib et al. recently reported an investigation on the influence that bulky anions had on the magnetic properties of a pair of chiral dysprosium SMMs.85 The chiral pair of complexes [Dy((∓)hfc)3(L3)]2·C7H16 (41) where hfc = 3-(heptafluoropropylhydroxymethylene)-(±)-camphorate and L3 = 4′-(4′′′-pyridyl-N-oxide)-1,2′:6′,1′′-bis-(pyrazolyl)pyridine, were dinuclear M2L2 type complexes, and possessed eight oxygens occupying the primary coordination sphere of the lanthanide ions. Six oxygens were provided by the coordinated hfc anions and one each by the two bound ligands. Addition of the bulky BarF anion (BarF = tetrakis[3,5-bis(trifluoromethyl)phenyl]borate) resulted in the formation of the chiral pair of 1D coordination polymers [[Dy((∓)hfc)2(L3)][BarF]]n·nCH3NO2 (42). The presence of the bulky anion caused partial dissociation of the hfc anions from the metal centre, which resulted in the lanthanide ion having five oxygen and three nitrogen atoms occupying its coordination sites (Fig. 10). They reported that the dinuclear complex (41) acts as a SMM with a blocking temperature of 4 K whilst the polymeric complex (42) acts as a one-dimensional assembly of field induced SMMs where the magnetic relaxation occurs through Raman processes. They attributed the enhanced SMM properties in the dinuclear complexes to the electronic distribution of the first coordination sphere better matching the oblate DyIII ion when compared to the polymer complex.
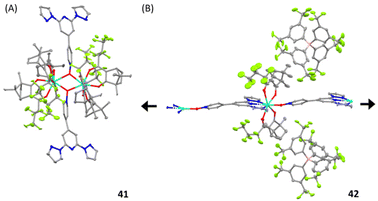 |
| Fig. 10 Structures of the dinuclear complex (A) and polymer complex (B) reported by Douib et al. Solvent molecules and hydrogen atoms have been omitted for clarity. Colour code: Dy, O, N, C, F, and B are cyan, red, blue, grey, lime, and pink, respectively. Thermal ellipsoids shown at 15%. | |
Reorientation of magnetic axis
A combination of secondary interactions can result in the symmetry of the complex no longer aligning with the easy axis of magnetisation, hindering SMM behaviour. Alternatively, secondary effects can also reorient the magnetisation axis itself away from the principal symmetry axis of the molecule. Both result in a lowering of the energy barrier.
An example of the former was presented by Gil et al. who investigated how the secondary interactions caused by 18-crown-6 affected the SMM performance of two mononuclear dysprosium based complexes [Dy(NCS)3(H2O)5]·0.45(KSCN) (18-crown-6) (43) and [Dy(NO3)2(NCS)3(H2O)]·(H2O)(NH4)2·2(18-crown-6) (44).86 The effective energy barriers for reversal of magnetisation were determined to be 47 K for (43) and 65.9 K for (44). They went further and performed a series of theoretical calculations to identify the effects of all secondary interactions resulting from the encapsulation by the crown ether. They found that hydrogen bonding interactions between bound water molecules and the crown ether had a detrimental effect on the magnetic anisotropy due to the water molecules being bound on the equatorial plane of the dysprosium anion. Furthermore, through ab initio calculations they found that by altering the electrostatic potential exerted by the crown ethers to align with the magnetic easy axis, they were able to enhance the magnetic properties. However, the mismatched symmetry axis of the crown ether molecules and the magnetic easy axis of the recorded complexes resulted in less optimal SMM performance.
Exploring the same phenomenon Herchel and co-workers investigated the effect of the second coordination sphere, specifically, the effect that 18-crown-6 had on the magnetic properties of two mononuclear lanthanide complexes [Dy(NO3)3(H2O)3]·(18-crown-6) (45) and [Er(NO3)3(H2O)3]·(18-crown-6) (46).87 Due to the complicated relaxation pathways present, the experimentally determined energy barriers lie in the ranges 66–71 K (45) and 21–24 K (46). Theoretical calculations of each complex with and without the presence of the crown ethers (Fig. 11) indicated that the calculated energy barriers for the complexes without crown ethers present were 143 K (45′) and 42 K (46′) whilst with the crown ethers they were 57 K (45) and 16 K (46). This was attributed to the reorientation of the magnetisation axis, demonstrating that the second coordination sphere has the capability to drastically reduce the magnetic performance of SMMs.
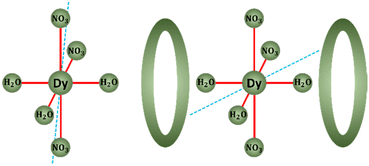 |
| Fig. 11 Effect of 18-crown-6 on the magnetic axis as reported by Herchel et al. blue line represents the magnetic axis. | |
Other effects
Secondary interactions can influence SMMs through ways that don't directly impact the magnetic properties. For example, secondary interactions are the dominant forces behind crystal packing and the formation of supramolecular architectures, where control of these could prove beneficial in the development of future SMMs.88,89
Exploring this, Sushila et al. synthesised five mononuclear lanthanide complexes (NdL52)(Et3NH)·THF (47), (TbL52)(Et3NH)·THF (48), (DyL52)(Et3NH)·THF (49), (NdL62)(Et3NH)·THF/H2O (50), and (TbL62)(Et3NH)·THF/H2O (51). Where H2L5 = 2-[N,N-bis(2-hydroxy-3,5-dichlorobenzyl)aminomethyl]pyridine and H2L6 = 2-[N,N-bis(2-hydroxy-3,5-dibromobenzyl)aminomethyl]pyridine.90 The complexes are essentially isostructural however, only the dysprosium containing complex (49) showed SMM behaviour with Ueff of 13.45 K. A notable trend of this series is that the overall coordination environment of the magnetic centre was invariant to the halogen used. This is a result of the hydrogen/halogen bonding interplay, which controls the overall crystal packing, being largely independent of the halogen present. However, the individual molecules do show subtle variations in order to cope with the stereo-electronic requirements when different halides are present.
Schmitz et al. reported the magnetic properties of two self-assembling supramolecular architectures ([Dy(OAc)3(H2O)3][Dy(H2O)3(prop·SMe)3][H2O⊂Cr3Dy6(OAc)12(H2bda)3(gly)3(ox)3(prop·SM)3]2(H2O))·12H2O·1.5MeCN (52) and ([Tb(OAc)3(H2O)3][Tb(H2O)3(prop·SMe)3] [H2O⊂Cr3Tb6 (OAc)12(H2bda)3(gly)3(ox)3(prop·SMe)3]2(H2O))·11.25H2O·1.5MeCN (53) where H-prop·SMe = 3-(methylthio)propionic acid; H2-bda = N-butyldiethanolamine; H2-ox = oxalic acid; H2-gly = glycolic acid.91 The recorded energy barriers were 11.9 K (52) and 4.9 K (53). The overall structures of each complex have dimensions of ca. 17 × 12 Å, which is comprised of distinct molecules which self-assembled and are maintained by purely intramolecular hydrogen bonds. They postulated that the formation of the supramolecular architecture was likely templated by cooperative hydrogen bonds of a central trio of water molecules in a manner which resembles polyoxovanadate chemistry.92
Conclusions
The biggest challenge facing researchers into SMMs is raising the temperature at which SMMs can retain their magnetisation. Two key routes to achieving this is through raising the effective energy barrier, Ueff, for the reversal of magnetisation or by reducing the number of pathways for through barrier relaxation to occur. In this context we have identified several areas where consideration of the effects of secondary interactions is necessary for the development of high-performance SMMs:
(1) By utilising secondary interactions to control the bond angles and physical positioning of metal centres in 3d–4f SMMs. This would provide a way to modulate the superexchange pathways and identify the ideal conditions to maximise SMM performance.
(2) Intermolecular hydrogen bonding interactions have been reported to facilitate magnetic exchange between adjacent metal centres. Designing SMMs with these interactions present can provide a way to suppress QTM through the exchange bias effect.
(3) Symmetry factors of the secondary coordination sphere can have a significant impact on the magnetic properties of SMMs, notably the use of sterically bulky units to modify the symmetry axis of the complex. Thus, utilising secondary interactions to impose higher order symmetry on metal complexes provides another effective way to suppress QTM.
In summary, we believe that an increased focus on secondary interaction provides an attractive avenue for the development of high-performance SMMs. On the other hand, failing to account for the effects secondary interactions can result in vastly diminished SMM performance.
Author contributions
BEM preparation and writing of the initial draft; ideas and evolution of overarching research goals and aims; PGP initial ideas of research goals and aims; oversight and leadership responsibility for the research activity, revision of the work; GJR and TND supervisory responsibility for the research activity, revision of the work; MED revision of the work.
Conflicts of interest
There are no conflicts to declare.
Acknowledgements
BEM and MED would like to acknowledge the Massey University Doctoral Scholarship for financial support. TND would like to acknowledge the Massey University College of Sciences Māori academic staff development programme.
References
- R. Sessoli, D. Gatteschi, A. Caneschi and M. A. Novak, Magnetic bistability in a metal-ion cluster, Nature, 1993, 365, 141–143 CrossRef CAS
.
- T. Lis, Preparation, structure, and magnetic properties of a dodecanuclear mixed-valence manganese carboxylate, Acta Crystallogr., Sect. B: Struct. Crystallogr. Cryst. Chem., 1980, 36, 2042–2046 CrossRef
.
- L. Bogani and W. Wernsdorfer, Molecular spintronics using single-molecule magnets, Nat. Mater., 2008, 7, 179–186 CrossRef CAS PubMed
.
- M. N. Leuenberger and D. Loss, Quantum computing in molecular magnets, Nature, 2001, 410, 789–793 CrossRef CAS PubMed
.
- E. J. L. McInnes, Introduction to Molecular Magnetism, Angew. Chem., Int. Ed., 2016, 55, 1959–1959 CrossRef CAS
.
-
D. Gatteschi, R. Sessoli and J. Villain, Molecular Nanomagnets, 2006 Search PubMed
.
- Z. Zhu and J. Tang, Metal–metal bond in lanthanide single-molecule magnets, Chem. Soc. Rev., 2022, 51, 9469–9481 RSC
.
- F.-S. Guo, B. M. Day, Y.-C. Chen, M.-L. Tong, A. Mansikkamäki and R. A. Layfield, Magnetic hysteresis up to 80 kelvin in a dysprosium metallocene single-molecule magnet, Science, 2018, 362, 1400–1403 CrossRef CAS PubMed
.
- C. Wang, Y.-S. Meng, S.-D. Jiang, B.-W. Wang and S. Gao, Approaching the uniaxiality of magnetic anisotropy in single-molecule magnets, Sci. China: Chem., 2023, 66, 683–702 CAS
.
- K. Yang, R. Sun, J. Zhao, C. Deng, B. Wang, S. Gao and W. Huang, A Combined Synthetic, Magnetic, and Theoretical Study on Enhancing Ligand-Field Axiality for Dy(III) Single-Molecule Magnets Supported by Ferrocene Diamide Ligands, Inorg. Chem., 2023, 62(25), 9892–9903 CrossRef CAS PubMed
.
- X.-D. Huang, X.-F. Ma and L.-M. Zheng, Photo-responsive Single-Molecule Magnet Showing 0D to 1D Single-Crystal-to-Single-Crystal Structural Transition and Hysteresis Modulation, Angew. Chem., Int. Ed., 2023, 62, e202300088 CrossRef CAS PubMed
.
- R. Weller, M. Atanasov, S. Demeshko, T.-Y. Chen, I. Mohelsky, E. Bill, M. Orlita, F. Meyer, F. Neese and C. G. Werncke, On the Single-Molecule Magnetic Behavior of Linear Iron(I) Arylsilylamides, Inorg. Chem., 2023, 62, 3153–3161 CrossRef CAS PubMed
.
- T. Xue, Y.-S. Ding, D. Reta, Q.-W. Chen, X. Zhu and Z. Zheng, Closely Related Organometallic Er(III) Single-Molecule Magnets with Sizably Different Relaxation Times of Quantum Tunneling of Magnetization, Cryst. Growth Des., 2023, 23, 565–573 CrossRef CAS
.
- D. Reta, J. G. C. Kragskow and N. F. Chilton, Ab Initio Prediction of High-Temperature Magnetic Relaxation Rates in Single-Molecule Magnets, J. Am. Chem. Soc., 2021, 143, 5943–5950 CrossRef CAS PubMed
.
- A. H. Vincent, Y. L. Whyatt, N. F. Chilton and J. R. Long, Strong Axiality in a Dysprosium(III) Bis(borolide) Complex Leads to Magnetic Blocking at 65 K, J. Am. Chem. Soc., 2023, 145, 1572–1579 CrossRef CAS PubMed
.
- A. Chiesa, F. Cugini, R. Hussain, E. Macaluso, G. Allodi, E. Garlatti, M. Giansiracusa, C. A. P. Goodwin, F. Ortu, D. Reta, J. M. Skelton, T. Guidi, P. Santini, M. Solzi, R. De Renzi, D. P. Mills, N. F. Chilton and S. Carretta, Understanding magnetic relaxation in single-ion magnets with high blocking temperature, Phys. Rev. B, 2020, 101, 174402 CrossRef CAS
.
- A. Castro-Alvarez, Y. Gil, L. Llanos and D. Aravena, High performance single-molecule magnets, Orbach or Raman relaxation suppression?, Inorg. Chem. Front., 2020, 7, 2478–2486 RSC
.
- A. Dey, J. Acharya and V. Chandrasekhar, Heterometallic 3d–4f Complexes as Single-Molecule Magnets, Chem. – Asian J., 2019, 14, 4433–4453 CrossRef CAS PubMed
.
- G. A. Craig and M. Murrie, 3d single-ion magnets, Chem. Soc. Rev., 2015, 44, 2135–2147 RSC
.
- A. Zabala-Lekuona, J. M. Seco and E. Colacio, Single-Molecule Magnets: From Mn12-ac to dysprosium metallocenes, a travel in time, Coord. Chem. Rev., 2021, 441, 213984 CrossRef CAS
.
- N. F. Chilton, Molecular Magnetism, Annu. Rev. Mater. Res., 2022, 52, 79–101 CrossRef
.
- J. Villain, F. Hartman-Boutron, R. Sessoli and A. Rettori, Magnetic Relaxation in Big Magnetic Molecules, Europhys. Lett., 1994, 27, 159 CrossRef CAS
.
- Z. Zhu, M. Guo, X.-L. Li and J. Tang, Molecular magnetism of lanthanide: Advances and perspectives, Coord. Chem. Rev., 2019, 378, 350–364 CrossRef CAS
.
- R. Orbach and B. Bleaney, Spin-lattice relaxation in rare-earth salts, Proc. R. Soc. London, Ser. A, 1961, 264, 458–484 CAS
.
- M. Briganti, F. Santanni, L. Tesi, F. Totti, R. Sessoli and A. Lunghi, A Complete Ab Initio View of Orbach and Raman Spin–Lattice Relaxation in a Dysprosium Coordination Compound, J. Am. Chem. Soc., 2021, 143, 13633–13645 CrossRef CAS PubMed
.
- A. Lunghi, F. Totti, S. Sanvito and R. Sessoli, Intra-molecular origin of the spin-phonon coupling in slow-relaxing molecular magnets, Chem. Sci., 2017, 8, 6051–6059 RSC
.
- R. J. Blagg, L. Ungur, F. Tuna, J. Speak, P. Comar, D. Collison, W. Wernsdorfer, E. J. L. McInnes, L. F. Chibotaru and R. E. P. Winpenny, Magnetic relaxation pathways in lanthanide single-molecule magnets, Nat. Chem., 2013, 5, 673–678 CrossRef CAS PubMed
.
- K. R. Vignesh, S. K. Langley, K. S. Murray and G. Rajaraman, Quenching the Quantum Tunneling of Magnetization in Heterometallic Octanuclear {TMIII4DyIII4} (TM=Co and Cr) Single-Molecule Magnets by Modification of the Bridging Ligands and Enhancing the Magnetic Exchange Coupling, Chem. – Eur. J., 2017, 23, 1654–1666 CrossRef CAS PubMed
.
- M. J. Giansiracusa, A. K. Kostopoulos, D. Collison, R. E. P. Winpenny and N. F. Chilton, Correlating blocking temperatures with relaxation mechanisms in monometallic single-molecule magnets with high energy barriers (Ueff > 600 K), Chem. Commun., 2019, 55, 7025–7028 RSC
.
- L. Escalera-Moreno, J. J. Baldoví, A. Gaita-Ariño and E. Coronado, Spin states, vibrations and spin relaxation in molecular nanomagnets and spin qubits: a critical perspective, Chem. Sci., 2018, 9, 3265–3275 RSC
.
- K. S. Pedersen, J. Dreiser, H. Weihe, R. Sibille, H. V. Johannesen, M. A. Sørensen, B. E. Nielsen, M. Sigrist, H. Mutka, S. Rols, J. Bendix and S. Piligkos, Design of Single-Molecule Magnets: Insufficiency of the Anisotropy Barrier as the Sole Criterion, Inorg. Chem., 2015, 54, 7600–7606 CrossRef CAS PubMed
.
- Z. Zhu and J. Tang, Lanthanide single-molecule magnets with high anisotropy barrier: where to from here?, Natl. Sci. Rev., 2022, 9, nwac194 CrossRef PubMed
.
- L. Belkhiri, B. Le Guennic and A. Boucekkine, DFT Investigations of the Magnetic Properties of Actinide Complexes, Magnetochemistry, 2019, 5, 15 CrossRef CAS
.
- Q.-C. Luo and Y.-Z. Zheng, Methods and Models of Theoretical Calculation for Single-Molecule Magnets, Magnetochemistry, 2021, 7, 107 CrossRef CAS
.
- S. S. Woodhouse, T. N. Dais, E. H. Payne, M. K. Singh, E. K. Brechin and P. G. Plieger, The structural manipulation of a series of Ni4 defective dicubanes: Synthesis, X-ray Structures, Magnetic and Computational analyses, Dalton Trans., 2021, 50, 5318–5326 RSC
.
- F. Neese and D. A. Pantazis, What is not required to make a single molecule magnet, Faraday Discuss., 2011, 148, 229–238 RSC
; discussion 299–314.
- A. M. Ako, I. J. Hewitt, V. Mereacre, R. Clérac, W. Wernsdorfer, C. E. Anson and A. K. Powell, A Ferromagnetically Coupled Mn19 Aggregate with a Record S=83/2 Ground Spin State, Angew. Chem., Int. Ed., 2006, 45, 4926–4929 CrossRef CAS PubMed
.
- D. N. Woodruff, R. E. Winpenny and R. A. Layfield, Lanthanide single-molecule magnets, Chem. Rev., 2013, 113, 5110–5148 CrossRef CAS PubMed
.
- N. Ishikawa, M. Sugita, T. Ishikawa, S.-y. Koshihara and Y. Kaizu, Lanthanide Double-Decker Complexes Functioning as Magnets at the Single-Molecular Level, J. Am. Chem. Soc., 2003, 125, 8694–8695 CrossRef CAS PubMed
.
- J. D. Rinehart and J. R. Long, Exploiting single-ion anisotropy in the design of f-element single-molecule magnets, Chem. Sci., 2011, 2, 2078–2085 RSC
.
- J. Sievers, Asphericity of 4f-shells in their Hund's rule ground states, Z. Phys. B: Condens. Matter, 1982, 45, 289–296 CrossRef CAS
.
- H.-D. Li, S.-G. Wu and M.-L. Tong, Lanthanide–radical single-molecule magnets: current status and future challenges, Chem. Commun., 2023, 59, 6159–6170 RSC
.
- S. T. Liddle and J. van Slageren, Improving f-element single molecule magnets, Chem. Soc. Rev., 2015, 44, 6655–6669 RSC
.
- W. Wernsdorfer, N. Aliaga-Alcalde, D. N. Hendrickson and G. Christou, Exchange-biased quantum tunnelling in a supramolecular dimer of single-molecule magnets, Nature, 2002, 416, 406–409 CrossRef PubMed
.
- A. Dey, J. Acharya and V. Chandrasekhar, Heterometallic 3d-4f Complexes as Single-Molecule Magnets, Chem. – Asian J., 2019, 14, 4433–4453 CrossRef CAS PubMed
.
- X. Tan, X. Ji and J.-M. Zheng, Heterometallic tetranuclear 3d–4f complexes: Syntheses, structures and magnetic properties, Inorg. Chem. Commun., 2015, 60, 27–32 CrossRef CAS
.
- K. Liu, W. Shi and P. Cheng, Toward heterometallic single-molecule magnets: Synthetic strategy, structures and properties of 3d–4f discrete complexes, Coord. Chem. Rev., 2015, 289–290, 74–122 CrossRef CAS
.
- L. Rosado Piquer and E. Carolina Sañudo, Heterometallic 3d-4f single-molecule magnets, Dalton Trans., 2015, 44, 8771–8780 RSC
.
- S. Osa, T. Kido, N. Matsumoto, N. Re, A. Pochaba and J. Mrozinski, A Tetranuclear 3d−4f Single Molecule Magnet: [CuIILTbIII(hfac)2]2, J. Am. Chem. Soc., 2004, 126, 420–421 CrossRef CAS PubMed
.
- M. Andruh, The exceptionally rich coordination chemistry generated by Schiff-base ligands derived from o-vanillin, Dalton Trans., 2015, 44, 16633–16653 RSC
.
- N. A. Bonde, J. B. Petersen, M. A. Sørensen, U. G. Nielsen, B. Fåk, S. Rols, J. Ollivier, H. Weihe, J. Bendix and M. Perfetti, Importance of Axial Symmetry in Elucidating Lanthanide–Transition Metal Interactions, Inorg. Chem., 2020, 59, 235–243 CrossRef CAS PubMed
.
- P. Kumar Sahu, R. Kharel, S. Shome, S. Goswami and S. Konar, Understanding the unceasing evolution of Co(II) based single-ion magnets, Coord. Chem. Rev., 2023, 475, 214871 CrossRef CAS
.
- P. Kumar Sahu, A. Mondal and S. Konar, Significant Control on Zero-Field Quantum Tunneling of Magnetization in Dysprosium Based Single-Molecule Magnets via Orientation of the Anilato Ligand, Chem. – Eur. J., 2023, 29, e202203664 CrossRef CAS PubMed
.
- J.-L. Liu, Y.-C. Chen and M.-L. Tong, Symmetry strategies for high performance lanthanide-based single-molecule magnets, Chem. Soc. Rev., 2018, 47, 2431–2453 RSC
.
- S.-Y. Lin, Y.-N. Guo, Y. Guo, L. Zhao, P. Zhang, H. Ke and J. Tang, Macrocyclic ligand encapsulating dysprosium triangles: axial
ligands perturbed magnetic dynamics, Chem. Commun., 2012, 48, 6924–6926 RSC
.
- X. Liu, C. Zhao, J. Wu, Z. Zhu and J. Tang, Air-stable chiral double-decker Dy(III) macrocycles with fluoride ion as the sole axial ligand, Dalton Trans., 2022, 51, 16444–16447 RSC
.
- S.-Y. Lin, C. Wang, L. Zhao, J. Wu and J. Tang, Chiral mononuclear lanthanide complexes and the field-induced single-ion magnet behaviour of a Dy analogue, Dalton Trans., 2015, 44, 223–229 RSC
.
- S. Liu, Y. Gil, C. Zhao, J. Wu, Z. Zhu, X.-L. Li, D. Aravena and J. Tang, A conjugated Schiff-base macrocycle weakens the transverse crystal field of air-stable dysprosium single-molecule magnets, Inorg. Chem. Front., 2022, 9, 4982–4989 RSC
.
- Z. Zhu, C. Zhao, T. Feng, X. Liu, X. Ying, X.-L. Li, Y.-Q. Zhang and J. Tang, Air-Stable Chiral Single-Molecule Magnets with Record Anisotropy Barrier Exceeding 1800 K, J. Am. Chem. Soc., 2021, 143, 10077–10082 CrossRef CAS PubMed
.
- K.-X. Yu, J. G. C. Kragskow, Y.-S. Ding, Y.-Q. Zhai, D. Reta, N. F. Chilton and Y.-Z. Zheng, Enhancing Magnetic Hysteresis in Single-Molecule Magnets by Ligand Functionalization, Chem, 2020, 6, 1777–1793 CAS
.
- M. A. Palacios, J. Nehrkorn, E. A. Suturina, E. Ruiz, S. Gómez-Coca, K. Holldack, A. Schnegg, J. Krzystek, J. M. Moreno and E. Colacio, Analysis of Magnetic Anisotropy and the Role of Magnetic Dilution in Triggering Single-Molecule Magnet (SMM) Behavior in a Family of CoIIYIII Dinuclear Complexes with Easy-Plane Anisotropy, Chem. – Eur. J., 2017, 23, 11649–11661 CrossRef CAS PubMed
.
- P.-E. Car, M. Perfetti, M. Mannini, A. Favre, A. Caneschi and R. Sessoli, Giant field dependence of the low temperature relaxation of the magnetization in a dysprosium(III)–DOTA complex, Chem. Commun., 2011, 47, 3751–3753 RSC
.
- G. Cucinotta, M. Perfetti, J. Luzon, M. Etienne, P.-E. Car, A. Caneschi, G. Calvez, K. Bernot and R. Sessoli, Magnetic Anisotropy in a Dysprosium/DOTA Single-Molecule Magnet: Beyond Simple Magneto-Structural Correlations, Angew. Chem., Int. Ed., 2012, 51, 1606–1610 CrossRef CAS PubMed
.
- M.-E. Boulon, G. Cucinotta, J. Luzon, C. Degl'Innocenti, M. Perfetti, K. Bernot, G. Calvez, A. Caneschi and R. Sessoli, Magnetic Anisotropy and Spin-Parity Effect Along the Series of Lanthanide Complexes with DOTA, Angew. Chem., Int. Ed., 2013, 52, 350–354 CrossRef CAS PubMed
.
- M. Briganti, G. F. Garcia, J. Jung, R. Sessoli, B. Le Guennic and F. Totti, Covalency and magnetic anisotropy in lanthanide single molecule magnets: the DyDOTA archetype, Chem. Sci., 2019, 10, 7233–7245 RSC
.
- X.-W. Liu, Z. Wu, J.-T. Chen, L. Li, P. Chen and W.-B. Sun, Regulating the single-molecule magnetic properties of phenol oxygen-bridged binuclear lanthanide complexes through the electronic and spatial effect of the substituents, Inorg. Chem. Front., 2020, 7, 1229–1238 RSC
.
- S. Petit, G. Pilet, D. Luneau, L. F. Chibotaru and L. Ungur, A dinuclear cobalt(II) complex of calix[8]arenes exibiting strong magnetic anisotropy, Dalton Trans., 2007, 4582–4588 RSC
.
- H. Weihe and H. U. Güdel, Quantitative Interpretation of the Goodenough−Kanamori Rules: A Critical Analysis, Inorg. Chem., 1997, 36, 3632–3639 CrossRef CAS PubMed
.
- C.-I. Yang, K.-H. Cheng, S.-P. Hung, M. Nakano and H.-L. Tsai, Crystal packing effects within [MnIII3O]7+ single-molecule magnets: Controlling intermolecular
antiferromagnetic interactions, Polyhedron, 2011, 30, 3272–3278 CrossRef CAS
.
- R. Mitsuhashi, S. Hosoya, T. Suzuki, Y. Sunatsuki, H. Sakiyama and M. Mikuriya, Hydrogen-bonding interactions and magnetic relaxation dynamics in tetracoordinated cobalt(II) single-ion magnets, Dalton Trans., 2019, 48, 395–399 RSC
.
- R. Mitsuhashi, S. Hosoya, T. Suzuki, Y. Sunatsuki, H. Sakiyama and M. Mikuriya, Zero-field slow relaxation of magnetization in cobalt(II) single-ion magnets: suppression of quantum tunneling of magnetization by tailoring the intermolecular magnetic coupling, RSC Adv., 2020, 10, 43472–43479 RSC
.
- A. D. Fournet, K. J. Mitchell, W. Wernsdorfer, K. A. Abboud and G. Christou, Three-Dimensional (3-D) Ferromagnetic Network of Mn12 Single-Molecule Magnets: Subtle Environmental Effects and Switching to Antiferromagnetic, Inorg. Chem., 2017, 56, 10706–10716 CrossRef CAS PubMed
.
- Y.-S. Jiao, C.-Q. Jiao, Y.-S. Meng, X.-R. Liu, L. Zhao and T. Liu, Controlling assembly and single-molecule magnet behavior of Fe-Ni clusters utilizing steric effect, Inorg. Chem. Commun., 2018, 93, 87–91 CrossRef CAS
.
- C. Zhao, Z. Zhu, X.-L. Li and J. Tang, Air-stable chiral mono- and dinuclear dysprosium single-molecule magnets: steric hindrance of hexaazamacrocycles, Inorg. Chem. Front., 2022, 9, 4049–4055 RSC
.
- G. Novitchi, W. Wernsdorfer, L. F. Chibotaru, J.-P. Costes, C. E. Anson and A. K. Powell, Supramolecular “Double-Propeller” Dimers of Hexanuclear CuII/LnIII Complexes: A (Cu3Dy3)2 Single-Molecule Magnet, Angew. Chem., Int. Ed., 2009, 48, 1614–1619 CrossRef CAS PubMed
.
- J.-P. Costes, S. Shova and W. Wernsdorfer, Tetranuclear [Cu–Ln]2 single molecule magnets: synthesis, structural and magnetic studies, Dalton Trans., 2008, 1843–1849 RSC
.
- T. Hamamatsu, K. Yabe, M. Towatari, S. Osa, N. Matsumoto, N. Re, A. Pochaba, J. Mrozinski, J.-L. Gallani, A. Barla, P. Imperia, C. Paulsen and J.-P. Kappler, Magnetic Interactions in CuII−LnIII Cyclic Tetranuclear Complexes: Is It Possible to Explain the Occurrence of SMM Behavior in CuII−TbIII and CuII−DyIII Complexes?, Inorg. Chem., 2007, 46, 4458–4468 CrossRef CAS PubMed
.
- C. Aronica, G. Pilet, G. Chastanet, W. Wernsdorfer, J.-F. Jacquot and D. Luneau, A Nonanuclear Dysprosium(III)–Copper(II) Complex Exhibiting Single-Molecule Magnet Behavior with Very Slow Zero-Field Relaxation, Angew. Chem., Int. Ed., 2006, 45, 4659–4662 CrossRef CAS PubMed
.
- S. Zhang, W. Mo, J. Zhang, Z. Zhang, B. Yin, D. Hu and S. Chen, Regulation of Substituent Effects on Configurations and Magnetic Performances of Mononuclear DyIII Single-Molecule Magnets, Inorg. Chem., 2019, 58, 15330–15343 CrossRef CAS PubMed
.
- F. Z. Chiboub Fellah, S. Boulefred, A. Chiboub Fellah, B. El Rez, C. Duhayon and J.-P. Sutter, Binuclear CuLn complexes (LnIII=Gd, Tb, Dy) of alcohol-functionalized bicompartmental Schiff-base ligand. Hydrogen bonding and magnetic behaviors, Inorg. Chim. Acta, 2016, 439, 24–29 CrossRef CAS
.
- A. B. Canaj, M. K. Singh, C. Wilson, G. Rajaraman and M. Murrie, Chemical and in silico tuning of the magnetisation reversal barrier in pentagonal bipyramidal Dy(III) single-ion magnets, Chem. Commun., 2018, 54, 8273–8276 RSC
.
- L. Gagliardi, R. Lindh and G. Karlström, Local properties of quantum chemical systems: The LoProp approach, J. Chem. Phys., 2004, 121, 4494–4500 CrossRef CAS PubMed
.
- G. A. Ramakant, N. Ahmed, I. Tarannum, S. Mehta, M. Nandeshwar, A. Mondal, S. K. Singh and G. Prabusankar, LnIII (Ln = La, Gd, and Dy) Benzimidazolium Tricarboxylate
Coordination Polymers with Hydrogen Bonding Modulated Magnetic Relaxation, Cryst. Growth Des., 2022, 22, 6046–6055 CrossRef CAS
.
- B. Yao, Y.-Q. Zhang, Y.-F. Deng, T. Li and Y.-Z. Zhang, Series of Benzoquinone-Bridged Dicobalt(II) Single-Molecule Magnets, Inorg. Chem., 2022, 61(39), 15392–15397 CrossRef CAS PubMed
.
- H. Douib, K. Dhbaibi, B. Lefeuvre, V. Dorcet, T. Guizouarn and F. Pointillart, Bulky anion effect on the architecture of chiral dysprosium single-molecule magnets, Chirality, 2023, 35, 155–164 CrossRef CAS PubMed
.
- Y. Gil, L. Llanos, P. Cancino, P. Fuentealba, A. Vega, E. Spodine and D. Aravena, Effect of Second-Sphere Interactions on the Magnetic Anisotropy of Lanthanide Single-Molecule Magnets: Electrostatic Interactions and Supramolecular Contacts, J. Phys. Chem., 2020, 124, 5308–5320 CAS
.
- R. Herchel, P. Zoufalý and I. Nemec, The effect of the second coordination sphere on the magnetism of [Ln(NO3)3(H2O)3]·(18-crown-6) (Ln = Dy and Er), RSC Adv., 2019, 9, 569–575 RSC
.
- M. Dolai, T. Mistri, A. Panja and M. Ali, Diversity in supramolecular self-assembly through hydrogen-bonding interactions of non-coordinated aliphatic –OH group in a series of heterodinuclear CuIIM (M=NaI, ZnII, HgII, SmIII, BiIII, PbII and CdII), Inorg. Chim. Acta, 2013, 399, 95–104 CrossRef CAS
.
- G. E. Kostakis, I. J. Hewitt, A. M. Ako, V. Mereacre and A. K. Powell, Magnetic coordination clusters and networks: synthesis and topological description, Philos. Trans. R. Soc., A, 2010, 368, 1509–1536 CrossRef CAS PubMed
.
- Sushila, R. Siddiqui, S. Patra, K. Shivam, A. Sil, B. Guchhait, H. Tian, R. Kataria, S. Goswami, P. Venugopalan and R. Patra, Halogen Bond Mediated Self-Assembly of Mononuclear Lanthanide Complexes: Perception of Supramolecular Interactions, Slow Magnetic Relaxation, and Photoluminescence Properties, Inorg. Chem., 2022, 61, 11484–11496 CrossRef CAS PubMed
.
- S. Schmitz, J. van Leusen, N. V. Izarova, Y. Lan, W. Wernsdorfer, P. Kogerler and K. Y. Monakhov, Supramolecular 3d-4f single-molecule magnet architectures, Dalton Trans., 2016, 45, 16148–16152 RSC
.
- K. Y. Monakhov, W. Bensch and P. Kögerler, Semimetal-functionalised polyoxovanadates, Chem. Soc. Rev., 2015, 44, 8443–8483 RSC
.
|
This journal is © the Partner Organisations 2023 |